DOI:
10.1039/C1PY00243K
(Review Article)
Polym. Chem., 2011,
2, 2444-2452
Permethylated cyclodextrin-based insulated molecular wires
Received
27th May 2011
, Accepted 22nd June 2011
First published on 29th July 2011
Abstract
Insulated molecular wires (IMWs), in which the π-conjugated polymers are covered by a protective sheath, have attracted considerable attention because of their potential applicability in next-generation mono-molecular electronic devices. We have developed new methods of synthesizing IMWs involving the polymerization of permethylated cyclodextrin (PMCD)-based rotaxane monomers. The obtained IMWs are highly soluble in organic solvents and have a high covering ratio, rigidity, and photoluminescence efficiency; further, they show high charge mobility, even in the solid state. In this review, the synthetic methodologies and characteristic of IMWs are discussed.
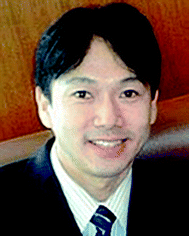 Jun Terao | Jun Terao received his PhD degree (1999) from Osaka University. After working as a postdoctoral fellow at Hokkaido University under Professor Tamotsu Takahashi, he joined the Graduate School of Engineering, the Department of Applied Chemistry, Osaka University, as an Assistant Professor. From 2002 to 2003, he worked at the University of Oxford with Professor Harry L. Anderson as a postdoctoral fellow. He was promoted to Associate Professor in 2008 in the Graduate School of Engineering, the Department of Energy and Hydrocarbon Chemistry, Kyoto University. His research interests are in the areas of supramolecular chemistry and molecular electronics. |
1. Introduction
π-Conjugated polymers are often described as molecular wires because of their high charge mobility. However, crosstalk and short circuiting by internal π–π interactions between these polymers can dramatically decrease their electrical qualities. This imparts interest in the study of insulated molecular wires (IMWs) in which the π-conjugated polymers are covered by a protective sheath, thereby limiting π–π interactions and enhancing their conductivity, solubility, and stability as compared with corresponding π-conjugated polymers. Accordingly, IMWs have attracted considerable attention due to their potential applicability in next-generation mono-molecular electronic devices.1 During the course of our studies on the cross-coupling reaction,2 we grew interested in wiring π-conjugated molecules between nanoscale electrodes using sequential cross-coupling reactions in order to realize molecular electronics. Recently, we developed a method to interconnect molecules between nanoscale electrodes using a simple liquid solution process in which a nanoscale electrode is sequentially dipped into three different solutions to fabricate a nanoscale photoswitching device (Fig. 1).3
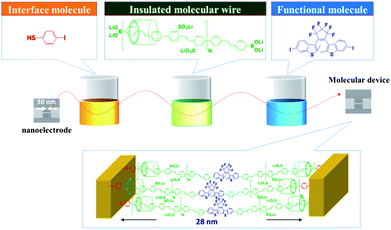 |
| Fig. 1 A new method to interconnect molecules between nanoscale electrodes using a simple liquid solution process.3 | |
These three solutions include p-iodobenzenethiol as an interface-control molecule, π-conjugated polyrotaxane as an IMW, and diarylethene as a functional molecule. These molecules become bound to each other between the nanoscale electrodes through the Suzuki cross-coupling reaction. A key requirement for this method is the use of π-conjugated polyrotaxane as an IMW in order to maintain the linearity and isolation of the π-conjugated polymer chains. However, the reproducibility of this interconnecting method was moderate. This is probably due to the low covering ratio of π-conjugated polyrotaxane prepared by the Suzuki cross-coupling reaction between water-soluble π-conjugated diiodo and diboronic acid derivatives in the presence of cyclodextrin (CD).4 To increase the reproducibility of this method, we are now interested in preparing IMWs with an ideal molecular structure including the following features (Fig. 2): (1) high degree of insulation to isolate the conjugated chain, (2) a rigid polymer chain with high linearity, (3) high solubility in organic solvents to enable in the use of liquid solution processes, (4) high charge mobility, and (5) reaction points at both ends to enable connections to other molecules.
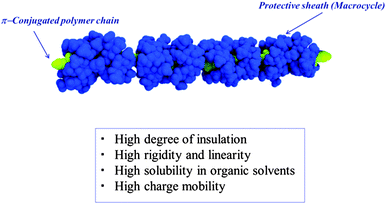 |
| Fig. 2 Ideal structure of insulated molecular wires for molecular electronics. | |
2.
Cyclodextrin-based insulated molecular wires
CD has been widely used as a macrocycle for the synthesis of IMWs because of its commercial availability and the efficacy of the inclusion of organic molecules into CD by hydrophilic–hydrophobic interactions in water.5 One method for the synthesis of IMWs using CD involves threading π-conjugated polymers through CD6 using the same method developed by Harada for the synthesis of a molecular necklace.7 Another method involves the polymerization of pseudo-rotaxane that is formed by the self-inclusion of a conjugated monomer with CD,8 or the copolymerization of the thus-formed pseudo-rotaxane with linker molecules, to form polyrotaxane.4 In general, the polyrotaxanes prepared by these methods do not have high and constant covering ratios. Additionally, these polymers are soluble in water but insoluble in organic solvents due to the hydrophilic CD that covers the conjugated polymer chains.9 There are also many uncovered sites and un-removed water molecules, which are disadvantageous for the use of these IMWs as electronic materials. Therefore, we decided to use permethylated cyclodextrin (PMCD) instead of native CD. The permethylation of all the hydroxyl groups of CD provides PMCD, which has a deeper vacancy and higher solubility in organic solvents as compared to native CD. However, the insolubility of PMCD in water is a disadvantage for the formation of inclusion complexes by self-inclusion via hydrophilic–hydrophobic interactions in water. To overcome this problem, we prepared a guest-branched PMCD that can undergo self-inclusion to form an inclusion complex with a rotaxane structure. This article describes our recent results, focusing on a new method for synthesizing organic-soluble IMWs by polymerization of PMCD-based inclusion complex monomers.
2.1 Synthesis of permethylated cyclodextrin-based insulated molecular wires by the Glaser reaction
Our proposed synthetic route to organic-soluble IMWs is shown in Fig. 3. The self-inclusion of guest-branched PMCD forms pseudo-rotaxane, which is capped with a stopper molecule to afford a linked [2]rotaxane ([1]rotaxane).10Polymerization sites are introduced at both ends of this rotaxane to form a fixed [2]rotaxane monomer. Then, this monomer is polymerized to afford π-conjugated polyrotaxane as an organic-soluble IMW.
Fig. 4 shows the synthesis of a fixed [2]rotaxane.11 The PMα-CD monotosylate 1 was synthesized from CD via an established two-step protocol.12 The reaction of 1 with a phenol derivative quantitatively afforded iodide 2. Sequential Pd-catalyzed Sonogashira cross-coupling reactions of 2 with trimethylsilyl acetylene and 1,4-diiodobenzene afforded tolane derivative 3. It should be noted that 3′ was formed quantitatively by self-inclusion in a 1
:
1 H2O
:
CH3OH solution. This phenomenon has been characterized by 1H NMR by employing different solvents. As shown in Fig. 5, the 1H NMR spectrum of 3 in CDCl3 reveals the exclusion of the tolane moiety from the cavity of the PMCD, whereas the spectrum in CD3OD reveals a mixture of exclusion and inclusion complexes. When a more hydrophilic solvent such as a 1
:
1 D2O
:
CD3OD solution was used, the exclusion complex 3 completely converted into the inclusion complex 3′. The evidence that the NMR spectra of 3′ at different concentrations in 1
:
1 D2O
:
CD3OD showed no new peaks ascribable to oligomeric and/or polymeric supramolecular complexes supports the formation of self-inclusion complex 3′.13 The formation of 3′ resulted in the following up- or down-field shifts of the aromatic protons in 3′: Ha–a′ (−0.25 ppm), Hb–b′ (+0.56 ppm), Hc–c′ (+0.13 ppm), Hd–d′ (+0.49 ppm), and He–e′ (+0.09 ppm). The remarkably large downfield shift of Hd–d′ suggests that these protons are located very close to the α-1,4-glucosidic oxygen atoms of PMCD.13 To fix the pseudo-rotaxane structure of 3′, we carried out a Suzuki coupling reaction using p-aminoboronic acid as a stopper group in a 1
:
1 H2O
:
CH3OH solution. The desired fixed [2]rotaxane 4 was obtained in an 80% isolated yield. The advantage of using PMCD is that all the CD derivatives can be easily purified using conventional column chromatography techniques. No evidence for the formation of exclusion complex 5 was observed. It is known that the decomplexation of [1]rotaxanevia a flipping of D-(+)-glucopyranose units linked through an α-1,4-glycoside bond is often observed due to the relative flexibility of PMCD in comparison to that of CD.14 However, 4 was stable in CDCl3 at room temperature for more than seven days without decomplexation.
As shown in Fig. 6, the structure of the fixed [2]rotaxane 4 was characterized by 2D 1H ROESY NMR; this is because there are obvious NOEs between protons on the tolane moiety and the H3 and H5protons in the interior of the PMCD molecule.
Crystals of 4 were grown from a mixture of DMSO and H2O. The X-ray structure (lateral and top view) of 4 is shown in Fig. 7. It is clear that the PMCD is displaced from the centre of the guest moiety, and the main axis of the guest exhibits a slight torsion.
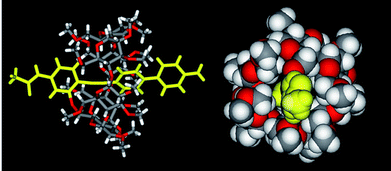 |
| Fig. 7 Lateral (stick model) and top (space-filling model) view of 4.15 | |
An acetamide and the amino groups of 4 were converted into ethynyl groupsvia the Sandmeyer reaction, which was followed by a Sonogashira coupling reaction and deprotection of the silyl group to yield the fixed [2]rotaxane monomer 5 (Fig. 8). According to a space filling model, the covering ratio of PMCD over the π-conjugated backbone was approximately 60%.
Glaser polymerization of 5 was carried out in the presence of Cu(OAc)2 in pyridine at 50 °C. According to the GPC analysis shown in Fig. 9, monomer 5 completely disappeared after 64 h and the formation of PMCD-based IMW 6 was confirmed. The peaks in the 1H NMR spectrum of 6 were broader than those in the 1H NMR spectrum of 5, indicating that the inclusion complex structure was maintained during polymerization. The Mw and PDI of 6 were estimated to be 3.88 × 104 and 2.01, respectively, using polystyrene as the calibration standard. The average degree of polymerization (ñ) was found to be 25.
The MALDI-TOF mass spectrum of IMW 6 provides excellent evidence of its structural authenticity since all of the observed peaks correspond to the expected singly charged molecular ions with more than 20 repeating units (Fig. 10).15
In order to increase the covering ratio and structural regularity of the IMW, we attempted to polymerize [3]rotaxane with a symmetrically structured monomer. The synthetic route to regioregular IMWs is shown in Fig. 11. Sonogashira coupling of 2 with (4-ethynylphenylethynyl)trimethylsilane followed by deprotection of the silyl group gave 7. Dimerization of 8 using Eglinton coupling in H2O
:
CH3OH
:
pyridine (10
:
5
:
1) gave the desired dimer 9. The formation of 9 was inferred from the MALDI-TOF mass spectrum, which displayed a strong signal at m/z 2959 corresponding to the [9 + Na]+ ion.16 Deprotection of the acetamide groups of 9 and treatment with NaNO2 and KI followed by a Sonogashira coupling reaction with trimethylsilylacetylene and deprotection of the silyl group yielded 10. The [3]rotaxane structure of 10 was characterized by 2D ROESY NMR. According to the space-filling model, the coverage of PMCD against the conjugated guest is about 77%. When Eglinton polymerization of 10 was carried out in the presence of Cu(OAc)2 in pyridine at room temperature, regioregular IMW 11 formed after 2 days. The Mn, Mw, and PDI of 11 were estimated to be 1.55 × 104, 9.74 × 104, and 6.28, respectively.17
To examine the insulating effect of PMCDs, the uninsulated monomer 12, which has the same π-conjugated unit as 10, and the corresponding polymer 13 were synthesized as reference compounds (Fig. 12). Unfortunately, 13 was insoluble in all organic solvents because of the strong π–π interactions between the π-conjugated polymer chains. The absorption and emission wavelengths of 10, 11, and 12 in solution are shown in Table 1. The elongation of the π-conjugated backbone from monomer 10 to IMW 11 resulted in a red-shift of about 45 nm. According to the spectra of 11 and 13, slight blue-shifts are observed in both the absorption and the emission spectra of 10. These shifts suggest that the structure of the π-conjugated guest was changed by insulating the polymers using PMCDs.
 |
| Fig. 12 Structures of the uninsulated monomer 12 and the corresponding polymer 13. | |
Table 1 Absorption and emission wavelengths and fluorescence quantum yields of 10–13a
Compound |
Absorption (λmax)/nm |
Emission (λmax)/nm |
Φ
solution
|
Φ
solid
|
The spectra were recorded in THF. The absolute quantum yields were determined using a calibrated integrating-sphere system.
|
10
|
357 |
397, 420 |
0.49 |
0.17 |
11
|
402 |
425, 450 |
0.52 |
0.14 |
12
|
368 |
403, 427 |
0.61 |
0.073 |
13
|
— |
— |
— |
<0.002 |
The photoluminescence quantum yields of 10–13 are summarized in Table 1. As expected, significant fluorescence enhancements are observed in 10 and 11 as compared to the corresponding uninsulated compounds 12 and 13, particularly in the solid state; this indicates that encapsulation of the π-conjugated units by PMCD enhances the fluorescence properties of these π-conjugated units.
To decrease the number of reaction steps and increase coverage, we proposed a simple methodology for the synthesis of regioregular IMWs by polymerization of pseudo-linked [3]rotaxane monomer 15, as shown in Fig. 13. The precursor of 15 was prepared in a 73% yield via three steps including Williamson ether synthesis of hydroquinone with PMCD monotosylate 1 and a Sonogashira coupling reaction with (4-ethynylphenylethynyl)trimethylsilane followed by deprotection. The formation of 16 by sequential intra-molecular self-inclusion of a phenylene-ethynylene oligomer with two PMCDs 15 was confirmed from the 1H NMR spectrum recorded in a CD3OD
:
D2O
:
TMEDA (1
:
1
:
0.1) solution at 50 °C.18 Using the space filling model of 16, the covering ratio was estimated to be approximately 95%. Subsequently, Glaser polymerization of 15 was carried out in the presence of CuI. Although the polymerization of 15 was markedly slower than that of 10 owing to the higher coverage of the former, regioregular IMW 18 (Mw = 5.77 × 104, PDI = 1.51, ñ = 21) was formed after seven days, as confirmed by GPC and MALDI-TOF mass spectroscopy. In order to examine the covering effect of PMCD, an uncovered polymer 17 (Mw = 5.64 × 104, PDI = 1.34, ñ = 21) was synthesized as a reference by the polymerization of 15 in a lipophilic solution (CH2Cl2) instead of a hydrophilic solution (1
:
1 CH3OH
:
H2O).19 The absolute quantum yields of 17 and 18 were determined using a calibrated integrating sphere system. The fluorescence quantum yields of 18 (Φsolution = 0.64, Φsolid = 0.23) revealed that its fluorescence efficiency was better than that of 17 (Φsolution = 0.58, Φsolid = 0.06), particularly in the solid state.
Regioregular IMW 18, in which numerous PMCDs are threaded into the main chain, has the potential to exist in a chiral liquid crystalline (LC) phase because of its rigidity and chirality, which result from the covering effect of the PMCDs. A liquid crystalline form of CD-based IMW derived from main chain rigidity has not been reported until now, although the LC properties of mesogenic pendants covalently linked to a polyrotaxane have been published.20 In order to evaluate the rigidity of 17 and 18, the persistence lengths (q) were estimated from the intrinsic viscosities of the solutions based on the unperturbed worm like cylinder model of the Yamakawa–Fujii–Yoshizaki theory.21 According to Bushin et al.22 and Bohdanecky,23 the solution properties of polymers in this model can be described as analytical functions of the intrinsic viscosity ([η]) and the molecular weight (Mw) if the persistence length (q), the diameter of the cylinder (d), and the molar mass per unit contour length (ML), which eventually leads to the monomer unit height (h), are given. Therefore the relationship between the intrinsic viscosity ([η]) and the absolute molecular weight of 17 and 18 was explored in chloroform by SEC equipped with refractive index and light scattering detectors and a viscometer, and the results were analyzed to estimate the q, d, and ML values in order to investigate the global conformational properties of these polymers (Fig. 14). The solid curves in the plots were calculated using the parameters determined from the fits between the theoretical and experimental [η] values over the studied Mw range. The h values (3.76 and 3.16 nm for 17 and 18, respectively) calculated from the estimated ML values (h = Mw/ML) are inconsistent with the length of the monomer unit (2.4 nm) estimated from molecular modelling. The molecular weight dependencies of the intrinsic viscosities of 17 and 18 were used to estimate the persistence length, q, based on the unperturbed worm like-chain model. The calculation was carried out over the range of the elution volume within which both the concentration and the scattering intensities are greater than 10% of the peak value. The intrinsic viscosity ([η]) is given by the following equation where d is the diameter of the cylinder and ML denotes the molar mass per unit contour length of the polymer.22,23 The viscosity–radius expansion factor for the excluded-volume effect was negligible because it is close to unity in the case of the Kuhn statistical segment number (Mw/(2qML)) < 50.24
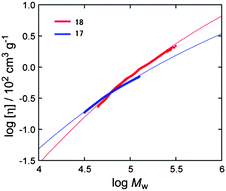 |
| Fig. 14 Double-logarithmic plots of the intrinsic viscosity vs. molecular weight of 17 (blue points) and 18 (red points), taken at 40 °C in chloroform. Solid curves (red and blue lines) were obtained from the worm like cylinder model and fit well with the experimental data. The evaluated parameters are q: 5.7 nm, d: 2.29 nm, ML: 730 nm−1, h: 3.76 nm for 17, and q: 14.4 nm, d: 2.26 nm, ML: 868 nm−1, h: 3.16 nm for 18.15 | |
The above equations indicate that [η] is characterized by q, d, and ML; therefore, a fitting procedure was employed to estimate q, d, and ML. The calculated q values for 17 and 18 were 5.7 nm and 14.4 nm, respectively, indicating that the rigidity of the main chain in 18 could be largely attributed to the intra-molecular inclusion of the polymer main chain into the PMCD cavity.
Fig. 15 shows polarized optical micrographs of 18 in concentrated CHCl3 solutions. It should be noted that 18 forms a cholesteric phase, as confirmed from its indisputably clear fingerprint texture, while 17 forms only an isotropic liquid even at high concentrations. The observed cholesteric phase can be attributed to the presence of PMCD in 18 since a nematic LC phase should be observed if a polymer chain threads through achiral macrocycles. This is the first report of the formation of a cholesteric LC phase whose chirality originates from the inclusion of a polymer main chain into the PMCD cavity. Both 17 and 18 successfully solidified into a film upon evaporation of the solvent from solution (Fig. 16). The striking difference in the birefringence of these films indicates that 18 forms an anisotropic phase in concentrated solutions because of the rigidity of its main chain, which, in turn, is a result of the covering effect of PMCD.
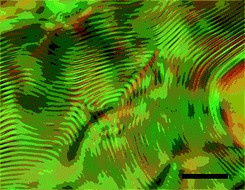 |
| Fig. 15 Polarized optical micrographs of a CHCl3 solution (ca. 15 wt%) of 18 sealed in a glass capillary tube; the micrographs were obtained at 25 °C under crossed polarizers. The LC phase was separated from the isotropic phase in equilibrium and its cholesteric pitch was maintained for more than 3 months. Black scale bar: 50 μm.15 | |
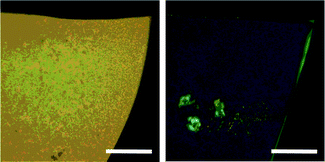 |
| Fig. 16 The films cast from chloroform solutions of 17 (left; Mw = 5.64 × 104, Mw/Mn = 1.34) and 18 (right; Mw = 5.77 × 104, Mw/Mn = 1.51) under crossed polarizers. Scale bars: 200 μm.15 | |
The morphology of 18 was investigated by performing AFM experiments using cleaved mica substrates (Fig. 17). Highly dilute solutions of high molecular weight polymer 18 (Mw = 2.3 × 105, ñ = 84, average length ≈ 200 nm) that was separated by GPC was used to avoid the aggregation of individual assemblies. From the AFM image and a section analysis (Fig. 17a and b), the expected average contour length and height of the polymer chains were determined to be ∼200 nm and ∼1.5 nm, which is equal to the outer diameter of the free PMα-CD calculated using the CPK model, respectively. In addition, the polymers had high linearity. The histogram of the molecular length is constructed from 100 molecules, and the bin width is set at 5 nm. The molecular length is determined from the line profile, as indicated by the arrows in Fig. 17a and b. The histogram is fitted to a Gaussian function that shows a peak value and a full width at half maximum (FWHM) of 175 and 25 nm, respectively. The histogram of the molecular height is constructed from data for 400 molecules, which was obtained from the cross-sectional profiles of 100 molecules, and the bin width is 0.1 nm. The histogram is fitted to a Gaussian function that shows a peak value and FWHM of 1.4 and 0.4 nm, respectively. The statistical analysis of the monomolecules of 18 yielded an average molecular length of 175 nm, which was close to the theoretical value calculated from the molecular weight; the average molecular height was 1.4 nm.
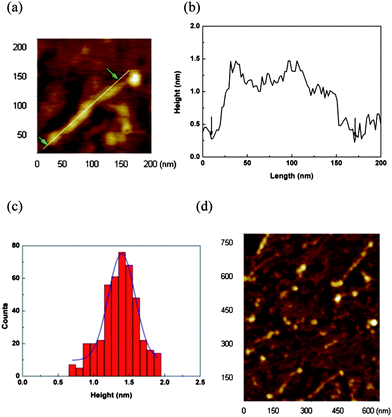 |
| Fig. 17 (a) Tapping-mode AFM topographic image of a molecular wire on a mica substrate. The white line indicates the region from which the line profile is taken. (b) The line profile of the molecular wire from panel (a). The arrows indicate the ends of the molecular wire. (c) Height histogram of 18. The blue lines are Gaussian fits to the histogram data. (d) AFM image of 18.19 | |
The transparent nature of the solid phase of π-conjugated polymers enables both in situflash photolysis time-resolved microwave conductivity (TRMC)25 and transient absorption spectroscopy (TAS) measurements on identical solid films. This makes it possible to carry out a thorough experimental and quantitative analysis of the intra-core charge carrier mobility along the π-conjugated backbones. We then examined the potential for charge mobility of the insulated molecular wire in the solid state by simultaneously performing TRMC and TAS measurements. Complete contactless measurement of the intra-molecular mobility was performed by combining the electrodeless transient conductivity measurement by TRMC with the clear transient optical absorption spectrum of the radical cations of 18. The polyrotaxane structure of 18 is expected to prevent π-stacking of the conjugated main chains even in the solid phase, thereby increasing the lifetime of charged radicals in the conjugated backbone by preventing charge recombination processes. The direct UV (355 nm) excitation of 18 caused charge separation between the polyrotaxane-conjugated core molecules and an oxygen residue with a low quantum yield, thereby generating mobile holes on the conjugated cores (Fig. 18). The observed conductivity transients were not single exponential, but fit well with a double exponential model. It should be noted that a low decay rate constant (k ≈ 4.2 × 103s−1) of 18 was estimated for the first component, which resulted in an extremely long lifetime (τ) of ∼200 μs, although rapid charge recombination (τ < 20 μs) was observed in the case of 17. This suggests that the insulating nature of the PMCD coating protects the holes in the core from being trapped by other molecules, fragments, and/or recombination products. The charge mobility of the polyrotaxanes in the solid state was examined by performing combined TRMC and TAS measurements.
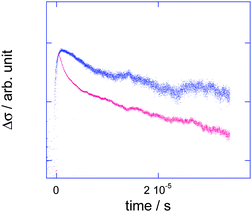 |
| Fig. 18 Normalized transient conductivity observed for thin films of 17 (red) and 18 (blue) on a quartz substrate upon excitation at 355 nm, 2.5 mJ cm−2.19 | |
2.2 Synthesis of permethylated cyclodextrin-based insulated molecular wires by the Sonogashira reaction
To increase the effective conjugation length in the core molecules, a diyne-free analogue of 18, polyrotaxane 19, with only a polyphenylene-ethynylene (PPE) backbone was synthesized by the Sonogashira copolymerization of 16 with 1,4-diiodobenzene instead of the Glaser polymerization of 16 (Fig. 19). The formation of 19 was also confirmed by GPC, MALDI-TOF mass spectrometry, and 1H NMR spectroscopy. The obtained high molecular weight polymer 19 (Mw = 3.2 × 105) was first fractionated by GPC and then analyzed by MALDI-TOF MS and NMR spectroscopy. Using a space filling model of 19, the covering ratio was estimated to be ∼85%.
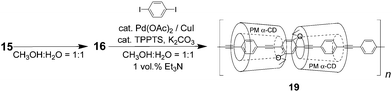 |
| Fig. 19 Synthesis of a diyne-bond-free insulated molecular wire containing polyphenylene-ethynylene as the backbone.19 | |
Fig. 20 shows the observed kinetic traces of the transient conductivity and the optical absorption of 19 upon irradiation by UV light (355 nm). For the quantitative analysis of photogenerated charge carriers, N,N′-bis(2,5-di-tert-butylphenyl)-3,4,9,10-pery-lenedicarboximide (PDCI) was used as an electron acceptor because of its high extinction coefficient in the radical anion form (εPDCI˙− = 7.4 × 104 cm−1 mol−1 dm3).26 The transient absorption spectrum showed a broad maximum at 600 nm, which was enhanced by the addition of PDCI to the film; this was confirmed by the distinct shoulder peak observed at 690 nm, which was attributed to the formation of PDCI radical anions (Fig. 21).
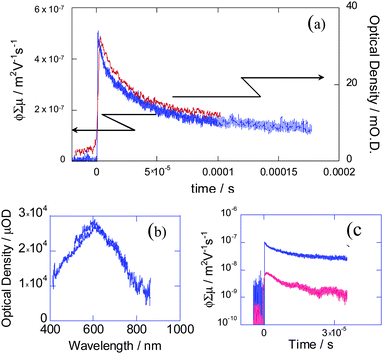 |
| Fig. 20 (a) Conductivity (blue) and optical absorption (red: 600 nm) transients of a thin film of 19 on a quartz substrate upon excitation at 355 nm at 2.5 and 35 mJ cm−2, respectively; (b) transient absorption spectrum of 19 2 μs after pulse exposure; (c) conductivity transients observed for 18 (red) and 19 (blue) upon excitation at 355 nm, 4.6 × 1015photons per cm.19 | |
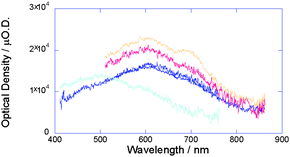 |
| Fig. 21 Transient absorption spectra of solid films of 19 (blue), 19 with 1 mol% PDCI (red), 19 with 2.5 mol% PDCI (orange), and 18 (light blue) on quartz substrates upon excitation at 355 nm, 6.5 × 1016photons per cm2. All the spectra were recorded 2 μs after pulse exposure.19 | |
The absorption maximum was almost identical to that of PDCI˙− in solution suggesting that the PDCI molecules were dispersed in the matrix of 19 without considerable aggregation. From the value of εPDCI˙−, that of 19˙+ (ε5˙+) was estimated to be 3.5 × 105 cm−1 mol−1 dm3. The ratio of the oscillator strength of 19˙+ to that of the singlet exciton transition of 19 at 375 nm was found to be ∼3 by the numerical integration of the spectrum; this value was in good agreement with the ratio estimated by time-dependent density functional theory (TD-DFT) calculations, i.e. 2.5, and that determined by singlet exciton bleaching at 410 nm (Fig. 22). On the basis of the value of ε19˙+, the concentration of [19˙+] was calculated at the pulse end from the TAS transient and the photocarrier generation yield (ϕ) was found to be 3.4 × 10−4 immediately after pulse exposure. The value of ϕ for 19 was also confirmed to be 2 × 10−4 by photocurrent accumulation in a time-of-flight setup.27 Recently, it was reported that self-assembled discotic mesophases based on PDCI core molecules exhibit high charge carrier mobility of both electrons and holes, depending on the molecular packing.28 However, PDCI˙− was observed in an isolated state in transient spectroscopy without contribution from PDCI˙+. The lifetime of the negative charge carriers on π-stacked PDCI (<10 μs) was also more than 10 times shorter than that of the conductivity transient shown in Fig. 20. Thus, the excellent correlation between the two transients in Fig. 20a clearly suggests that the positive charge on the π-conjugated core of the molecules in 19 contributed to the high conductivity of this compound over the entire time range. It is noteworthy that the minimum value of anisotropic hole mobility (μ+) in the core estimated from the maximum value of ϕ (3.4 × 10−4) was 0.5 cm2 V−1s−1, which is 100 times that of 18 (0.0045 cm2 V−1s−1). From the anisotropic hole mobilities, the oscillating displacement of the charge carriers in an electric field (∼102 V cm−1) in a microwave cavity was estimated as ∼5 nm with a turn over period of the microwave of 110 ps. This displacement is almost equivalent to the length of 5–10 repeating monomer units in 19 (1 unit: 0.68 nm from the optimized geometry of 19); the number of repeating units in 18 was found to be <1. This is the first report of the formation of an almost perfectly insulated organic semiconductor wire in which the hole mobility along the π-conjugated polymer chain is extremely high and is comparable to that of amorphous silicon.
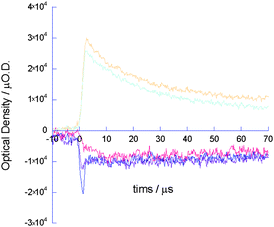 |
| Fig. 22 Transient absorption kinetic traces observed for a solid film of 19 with 2.5 mol% PDCI upon excitation at 355 nm, 6.5 × 1016photons per cm2. The traces are recorded at 600 (orange), 700 (light blue), 430 (blue), 420 (violet), and 410 nm (red).19 | |
We have also developed a new method for the synthesis of highly organic-soluble IMWs with parallel PMCD lines through Sonogashira polymerization of linked [2]rotaxane containing iodo and alkynyl groups at both ends of a π-conjugated guest.29 As shown in Fig. 23, π-conjugated guest-linked PMCD 23 was synthesized from 2-iodo-5-nitrophenolvia five steps. 23 was completely converted to 24 in a 1
:
3 H2O
:
CH3OH solution. Polymerization of 24 in the same solution under Sonogashira coupling conditions gave IMW 25 (Mw = 3.2 × 104, ñ = 22). Complete contactless measurement of the intra-molecular mobility was performed by combining the electrodeless transient conductivity measurement by TRMC with the clear transient optical absorption spectrum of the radical cations of 25. The minimum value of the anisotropic hole mobility in the PPE backbone of this IMW was estimated to be 0.7 cm2 V−1s−1. This value was fairly high and comparable to that in polythiophene-based IMWs.30
Conclusions
This paper highlights a new method for synthesizing IMWs through the polymerization of structurally defined rotaxane monomers. The IMWs thus formed have a high covering ratio, rigidity, and PL efficiency and showed high charge mobility in the solid state; further, they are readily soluble in a variety of organic solvents. The first example of a cholesteric LC phase was observed for polyrotaxanes because of the polymer's high rigidity and structure featuring a π-conjugated polymer chain threaded through chiral macrocycles. A highly insulated organic-semiconductor wire in the solid state was demonstrated with hole mobility along the π-conjugated polymer chain achieving close to that of amorphous silicon. The thus-formed polyrotaxane structure prevents π-stacking of the conjugated main chains even in the solid phase, leading to prolongation of the lifetime of charged radicals on the conjugated backbone by blocking the charge recombination processes. There was significant fluorescence enhancement in the polyrotaxane, especially in the solid state, which suggests that encapsulation of the π-conjugated polymer chains by PMCD is essential to attain efficient fluorescence properties. The present study is attractive from both the synthetic and the material perspectives, especially pertaining to the fields of synthetic and organoelectronic chemistry.
Acknowledgements
I greatly acknowledge the experimental and intellectual contribution of my co-workers. Particular gratitude is directed to Dr Susumu Tsuda, Mr Yuji Tanaka, Mr Keisuke Tsurui, and Mr Kazuhiro Ikai, who provided the experimental foundation for a large portion of this work. Support from the grant from the PRESTO program of the Japan Science and Technology Agency (JST) and the Ministry of Education, Culture, Sports, Science and Technology of Japan is gratefully acknowledged. Additional funding has been provided by the Asahi Glass foundation and the Sumitomo foundation.
Notes and references
- For recent reviews of insulated molecular wires, see:
(a) M. J. Frampton and H. L. Anderson, Angew. Chem., Int. Ed., 2007, 46, 1028–1064 CrossRef CAS
;
(b)
G. Wenz, in Inclusion Polymers, Springer, Verlag, 2009 Search PubMed
.
-
(a) J. Terao, H. Watanabe, A. Ikumi and N. Kambe, J. Am. Chem. Soc., 2002, 124, 4222–4223 CrossRef CAS
;
(b) J. Terao, A. Ikumi, H. Kuniyasu and N. Kambe, J. Am. Chem. Soc., 2003, 125, 5646–5647 CrossRef CAS
;
(c) J. Terao, H. Todo, H. Watanabe and N. Kambe, Angew. Chem., Int. Ed., 2004, 43, 6180–6182 CrossRef CAS
;
(d) J. Terao, H. Todo, S. A. Begum, H. Kuniyasu and N. Kambe, Angew. Chem., Int. Ed., 2007, 46, 2086–2089 CrossRef CAS
;
(e) J. Terao and N. Kambe, Acc. Chem. Res., 2008, 41, 1545–1554 CrossRef CAS
.
- Recently we have developed a molecular interconnecting method between nanoelectrodes by using polyrotaxane as an insulated molecular wire, see: M. Taniguchi, Y. Nojima, K. Yokota, J. Terao, K. Sato, N. Kambe and T. Kawai, J. Am. Chem. Soc., 2006, 128, 15062–15063 CrossRef CAS
.
-
(a) P. N. Taylor, M. J. O'Connell, L. A. McNeill, M. J. Hall, R. T. Aplin and H. L. Anderson, Angew. Chem., Int. Ed., 2000, 39, 3456–3460 CrossRef CAS
;
(b) J. J. Michels, M. J. O'Connell, P. N. Taylor, J. S. Wilson, F. Cacialli and H. L. Anderson, Chem.–Eur. J., 2003, 9, 6167–6176 CrossRef CAS
.
-
(a) G. Wenz, B.-H. Han and A. Müller, Chem. Rev., 2006, 106, 782–817 CrossRef CAS
;
(b) A. Harada, Y. Takashima and H. Yamaguchi, Chem. Soc. Rev., 2009, 38, 875–882 RSC
.
-
(a) K. Yoshida, T. Shimomura, K. Ito and R. Hayakawa, Langmuir, 1999, 15, 910–913 CrossRef CAS
;
(b) I. Yamaguchi, K. Kashiwagi and T. Yamamoto, Macromol. Rapid Commun., 2004, 25, 1163–1166 CrossRef CAS
.
- A. Harada, J. Li and M. Kamachi, Nature, 1992, 356, 325–327 CrossRef CAS
.
- K. Shinohara, T. Suzuki, T. Kitami and S. Yamaguchi, J. Polym. Sci., Part A: Polym. Chem., 2006, 44, 801–809 CrossRef CAS
.
- M. J. Frampton, G. Sforazzini, S. Brovelli, G. Latini, E. Townsend, C. C. Williams, A. Charas, L. Zalewski, N. S. Kaka, M. Sirish, L. J. Parrott, J. S. Wilson, F. Cacialli and H. L. Anderson, Adv. Funct. Mater., 2008, 18, 3367–3376 CrossRef CAS
.
- For nomenclature of rotaxanes, see: A. Yerin, E. S. Wilks, G. P. Moss and A. Harada, Pure Appl. Chem., 2008, 80, 2041–2068 CrossRef CAS
.
- S. Tsuda, J. Terao and N. Kambe, Chem. Lett., 2009, 38, 76–77 CrossRef CAS
.
- For synthetic details, see: T. Kaneda, T. Fujimoto, J. Goto, K. Asano, Y. Yasufuku, J. H. Jung, C. Hosono and Y. Sakata, Chem. Lett., 2002, 31, 514–515 CrossRef
.
- T. Fujimoto, Y. Sakata and T. Kaneda, Chem. Commun., 2000, 2143–2145 RSC
.
-
(a) T. Yamada, G. Fukuhara and T. Kaneda, Chem. Lett., 2003, 32, 53–5354 Search PubMed
;
(b) R. Nishiyabu and K. Kano, Eur. J. Org. Chem., 2004, 4985–4988 CAS
.
- J. Terao, S. Tsuda, Y. Tanaka, K. Okoshi, T. Fujihara, Y. Tsuji and N. Kambe, J. Am. Chem. Soc., 2009, 131, 16604–16605 Search PubMed
.
- S. Tsuda, J. Terao, K. Tsurui and N. Kambe, Chem. Lett., 2009, 38, 190–191 CrossRef CAS
.
- J. Terao, S. Tsuda, K. Tsurui and N. Kambe, Macromol. Symp., 2010, 297, 54–60 CrossRef CAS
.
- S. Tsuda, J. Terao, Y. Tanaka, T. Maekawa and N. Kambe, Tetrahedron Lett., 2009, 50, 1146–1150 CrossRef CAS
.
- J. Terao, Y. Tanaka, S. Tsuda, N. Kambe, M. Taniguchi, T. Kawai, S. Saeki and S. Seki, J. Am. Chem. Soc., 2009, 131, 18046–18047 CrossRef CAS
.
- M. Kidowaki, T. Nakajima, J. Araki, A. Inomata, H. Ishibashi and K. Ito, Macromolecules, 2007, 40, 6859–5862 CrossRef CAS
.
-
(a) H. Yamakawa and M. Fujii, Macromolecules, 1974, 7, 128–135 CrossRef CAS
;
(b) H. Yamakawa and T. Yoshizaki, Macromolecules, 1980, 13, 633–643 CrossRef CAS
.
- S. V. Bushin, V. N.Tsvetkov, E. B. Lysenko and V. N. Emelyanov, Vysokomol. Soedin., Ser. A, 1981, 23, 2494–2503 CAS
.
- M. Bohdanecky, Macromolecules, 1983, 16, 1483–1492 CrossRef CAS
.
-
H. Yamakawa, in Helical Wormlike Chains in Polymer Solutions, Springer, Berlin, 1997, p. 278 Search PubMed
.
-
(a) A. Acharya, S. Seki, A. Saeki, Y. Koizumi and S. Tagawa, Chem. Phys. Lett., 2005, 404, 356–360 CrossRef CAS
;
(b) A. Saeki, S. Seki, T. Takenobu, Y. Iwasa and S. Tagawa, Adv. Mater., 2008, 20, 920–923 CrossRef CAS
;
(c) T. Amaya, T. Moriuchi, K. Nakamoto, T. Nakata, H. Sakane, A. Saeki, S. Tagawa and T. Hirao, J. Am. Chem. Soc., 2009, 131, 408–409 CrossRef CAS
.
-
(a) W. E. Ford, H. Hiratsuka and P. V. Kamat, J. Phys. Chem., 1989, 93, 6692–6696 CrossRef CAS
;
(b) D. Gosztola, M. P.Niemczyk, W. Svec, A. S. Lukas and M. R. Wasielewski, J. Phys. Chem. A, 2000, 104, 6545–6551 CrossRef CAS
.
- S. Seki, Y. Yoshida, S. Tagawa, K. Asai, K. Ishigure, K. Furukawa, M. Fujiki and N. Matsumoto, Philos. Mag. B, 1999, 79, 1631–1646 CrossRef CAS
.
- V. Marcon, D. W. Breiby, W. Pisula, J. Dahl, J. Kirkpatrick, S. Patwardhan, F. C. Grozema and D. Andrienko, J. Am. Chem. Soc., 2009, 131, 11426–11432 CrossRef CAS
.
- J. Terao, K. Ikai, N. Kambe, S. Seki, A. Saeki, K. Ohkoshi, T. Fujihara and Y. Tsuji, Chem. Commun., 2011, 47, 6816–6818 RSC
.
- K. Sugiyasu, Y. Honsho, R. M. Harrison, A. Sato, T. Yasuda, S. Seki and M. Takeuchi, J. Am. Chem. Soc., 2010, 132, 14754–14756 CrossRef CAS
.
|
This journal is © The Royal Society of Chemistry 2011 |