DOI:
10.1039/C1PY00075F
(Paper)
Polym. Chem., 2011,
2, 2193-2203
Red light-emitting hyperbranched fluorene-alt-carbazole copolymers with an iridium complex as the core
Received
13th February 2011
, Accepted 20th June 2011
First published on 8th July 2011
Abstract
Red light-emitting hyperbranched copolymers with an iridium complex as the core and fluorene-alt-carbazole as the branches were synthesized by one-pot Suzuki polycondensation. Efficient energy transfer from the poly(3,6-carbazole-alt-2,7-fluorene) segment to the iridium complex occurs when the Ir complex content is as low as 1 mol% in the copolymer. A maximal external quantum efficiency of 6.1% ph/el and a luminous efficiency of 4.7 cd A−1 were obtained with a CIE coordinate of (0.63, 0.32) for the device made from PFCzPh20NapyIr2 copolymer. The efficiency loss upon the increase of current density is significantly reduced since the highly branched structure could suppress the interchain interaction of polymers and the self quenching of the Ir complex. The integration of a phosphorescent complex into hyperbranched polymers can provide a successful molecular design route for solution-processable polymers for PLED applications.
Introduction
Electrophosphorescent conjugated polymers containing iridium complexes have attracted much attention because of their relatively short phosphorescent lifetime, high luminous efficiency, excellent color tenability and potential applications in large area full-color flat-panel displays and backlights.1–5 The phosphorescent conjugated polymers based on polyfluorene backbone with diketone pendant attached to the C-9 position of fluorene were reported by Chen et al. The device from the graft polymer of 1.3 mol % (btp)2Ir(acac) with a diketone attached to the 9-position of fluorene ring has an external quantum efficiency of 1.59% and a power efficiency of 2.8 cd A−1 at 7.0 V with a luminance of 65 cd m−2 and the peak emission at 610 nm.6 The device from fluorene-alt-carbazole copolymer grafted in the N-position with 1-phenylisoquinoline-Ir complex was reported, with a highest external quantum efficiency of 4.9%.7 A series of yellow and red light-emitting conjugated well-defined oligo- and polyfluorenyl bis-cyclometalated Ir complexes were synthesized with Suzuki homo-polycondensation of A–B-type monomer, and the maximum external quantum efficiencies of 1.5% and 0.12% were obtained respectively by introducing (btp)2Ir(acac) and (ppy)2Ir(acac) complexes covalently into the poly(9,9′-dioctylfluorene) backbone.8 The copolymers with Ir complexes in the backbone of poly(fluorene-alt-carbazole) and polyfluorene were synthesized, with the maximal external quantum efficiencies of 4.6% and 7.0%, respectively.9,10 Recently, Huang et al. reported the synthesis and applications of phosphorescent conjugated polymers containing iridium complexes.11–14 Among them, the most extensive study focused on the linear conjugated polymers, and the hyperbranched electrophosphorescent polymers were much less reported. Since the highly branched and globular features can reduce or eliminate strong intermolecular interaction and aggregation, the electroactive and light-emitting dendrimers and hyperbranched polymers are of current interest for developing efficient electroluminescent devices for displays and lighting and other photonic devices.15–20 Moreover, it is likely that the three-dimensional polymers are advantageous to its linear counterpart in high solubility, good processibility, minimizing unfavorable crystallization, and forming good quality amorphous films.21 Comparing to the dendritic polymers or other well defined polymers,22–24 the hyperbranched polymers are not only easier to synthesize but also possess comparable properties.
Many methods have been developed to prepare hyperbranched polymers, such as “A2 + B3,”25–27 “AB2 + AB,”28 “AB2,”26,29,30 and A2 + A′2 + B331,32 approaches. He et al. synthesized a hyperbranched conjugated polymer with benzene as the core, phenylene vinylene as the connecting groups and dimethylamino benzene as the end groups.25 Liu et al. synthesized a series of hyperbranched alternating copolymers by either Suzuki or Grignard coupling condensation between two AB4-type tetrahedral monomers with AB2-type dibromo or diboronic acid monomers of 9,9-dihexylfluorene or oligothiophene.33Xinet al. synthesized hyperbranched oxadiazole containing polyfluorenes with stable blue light emission even in the air at the elevated temperatures.34 Bo et al. synthesized porphyrin-cored star polymers with fluorenyl and triphenylamine as the capping groups, which exhibit good solubility, film-forming ability and pure saturated red light emission.35 Peng et al. synthesized a series of hyperbranched polyfluorenes containing triarylpyrazolinecores by the “A2 + A′2 + B3” Suzuki polycondensation.32 The hyperbranched conjugated polymers can effectively suppress the long-wavelength emission usually observed in thermally treated polyfluorenes. The standard PLED based on PFNZ10 emitted stable and pure blue light with an external quantum efficiency of about 1.93%.32 A novel hyperbranched phthalocyanine was constructed by Lee et al. for use as a hole injection layer that is coated on the ITO layer of an OLED.36 Wen et al. synthesized a series of hyperbranched triazine-containing polyfluorenes by an “A2 + A′2 + B3” approach based on Suzuki polycondensation.31 Wang et al. synthesized hyperbranched polymers by incorporating carbazole into polyfluorene to obtain highly stable blue light-emitting conjugated polymers with 9-phenyl-carbazole derivatives as the polymer cores.37
Previously, we prepared green light-emitting phosphorescent hyperbranched polymers with an iridium complex as the core by Yamamoto38 and Suzuki polycondensation.39 And the best device from PCzPy10Irppy3 with a peak external quantum efficiency, a luminous efficiency and a power efficiency of 13.3%, 30.1 cd A−1 and 16.6 lm W−1 at 5.6V, respectively. In this paper, the red light-emitting hyperbranched copolymers based on 2,7-fluorene-alt-3,6-carbazole branch with iridium complex as core were synthesized by one-pot Suzuki polycondensation.
Experimental section
Materials
All manipulations involving air-sensitive reagents were performed under an atmosphere of dry argon. The solvents (THF and toluene) were purified by routine procedures and distilled under dry argon before being used. All reagents, unless otherwise specified, were obtained from Aldrich, Acros, and TCI Chemical Co. and used as received.
Instrumentation
The 1H and 13C NMR spectra were recorded on a Bruker DRX 300 in deuterated chloroform solution. Mass spectrometric detection was performed using Shimadzu LCMS-2010A quadrupole mass spectrometer with electrospray ionization (ESI) interface. The number-average molecular weights (Mn) were determined by a Waters GPC 2410 in tetrahydrofuran (THF) with a calibration curve of polystyrene standards. The element analyses were performed on a Vario EL element analysis instrument (Elementar Co.). The iridium content analyses were determined by using a Philips (Magix PRO) sequential X-ray fluorescence spectrometry (XRF), with a rhodium tube operated at 60 kV and 50 mA, a LiF 200 crystal and a scintillation counter. Tris(acetylacetonate) iridium(III) (Ir content of 38%, from Alfa Aesar Co.) was used as an internal reference specimen. Samples and specimens were pressed into homogeneous tablets (Φ = 30 mm) of compressed (375 MPa) powder of the copolymers. UV-Vis absorption spectra were recorded on an HP 8453 UV-Vis spectrophotometer. Cyclic voltammetry was carried out on a CHI660A electrochemical workstation in a solution of tetrabutylammonium hexafluorophosphate (Bu4NPF6, 0.1M) in acetonitrile (CH3CN) at a scanning rate of 50mV s−1 at room temperature under argon. A platinum electrode coated with a thin polymer film was used as the working electrode. A Pt plate was used as the counter electrode, and a saturated calomel electrode was used as the reference electrode. The thermogravimetric analysis (TGA) of the polymers was performed at a heating rate of 20 °C min−1 with a NETZSCH TGA-209 thermal analyzer under nitrogen atmosphere. The thermal transitional properties of the polymers were measured with a NETZSCH DSC-204 differential scanning calorimeter (DSC) under a nitrogen atmosphere at a heating rate of 20 °C min−1. The photoluminescence (PL) efficiencies were determined in an IS080 integrating sphere (Labsphere) with 325 nm excitation of a HeCd laser (Mells Griot). PL and EL spectra were recorded on an Instaspec IV CCD spectrophotometer (Oriel Co.).
Synthesis
2,7-Bis(4,4,5,5-tetramethyl-1,3,2-dioxaborolan-2-yl)-9,9-dioctylfluorene,401-(4,4,5,5-tetramethyl-1,3,2-dioxaborolan-2-yl)-3,5-dibromobenzene41 and 3,6-dibromo-N-(2-ethylhexyl)-carbazole42 were prepared according to the published procedures.
3-(Pyridin-2-yl)-1H-1,2,4-triazole
43,44(1).
2-Cyanopyridine (6.2 g) and aqueous hydrazine (10 ml, 80%) were added to a three-necked flask and stirred at 40 °C for 6 h. 2-Pyridinamidrazone as a white solid was collected by suction filtration, and after it was washed with ether several times, put into the vacuum drier. Formic acid (40 ml) was added to a three-necked flask in an ice bath and then 2-pyridinamidrazone slowly added into the three-necked flask, keeping the reaction temperature below 5 °C. After adding 2-pyridinamidrazone, it reacted at room temperature for 0.5 h, and was then heated to reflux for 3 h. After removed of the extra formic acid and water with a suction rotator, the residue was cooled to room temperature and the white solid was separated out. After neutralized with 10% aqueous Na2CO3, washed with isopropyl ether, filtered with a suction, and dried in the vacuum drier, the crude product was recrystallized in alcohol/ether solution to afford 3-(pyridin-2-yl)-1H-1,2,4-triazole as a white solid (yield: 60%).
1H NMR (300 MHz, CDCl3) δ (ppm):15–14 (br s, 1H), 8.76 (d, J = 4.6 Hz, 1H), 8.24 (d, J = 7.9 Hz, 1H), 8.13 (s, 1H), 7.88 (t, J = 7.6 Hz, 1H), 7.41 (m, 1H). 13C NMR (300 MHz, CDCl3) δ (ppm): 154.75, 151.70, 149.46, 146.44, 137.82, 125.04, 121.94. Calculated for C7H6N4: C, 57.53; H, 4.14; N, 38.34. Found: C, 57.43; H, 4.07; N, 38.20.
3-Bromo-5-(pyridin-2-yl)-1H-1,2,4-triazole
45 (2).
A suspension of 3-(pyridin-2-yl)-1H-1,2,4-triazole (4.4 g, 30 mmol) in H2O (75 mL) was dissolved by slow addition of 10 M NaOH (pH = 12). Subsequently, 3 mL of Br2 (99%; d = 3.199 g mL−1) was added slowly while maintaining a pH of 12 by addition of concentrated NaOH solution. After stirring for 3 h, the solution was acidified to pH 3–4 with 5 M HCl (aq), resulting in precipitation of the brominated ligand, which was collected by suction filtration. Yield: 4.7 g (70%). 1H NMR (300 MHz, d6-DMSO) δ (ppm): 15.21 (s, 1H), 8.71 (s, 1H), 8.03 (s, 2H), 7.55 (s, 1H). Calculated for C7H5BrN4: C, 37.36; H, 2.24; N, 24.90. Found: C, 37.27; H, 2.17; N, 24.78.
5-Bromo-2-(1-naphthalene) pyridine
10 (3).
2,5-Dibromopyridine (2.37 g, 10 mmol), 1-naphthaleneboronic acid (1.72 g, 10 mmol), and tetrakis- (triphenylphosphine)palladium (0.107 g, 0.1 mmol) were dissolved in toluene (15 mL) and ethanol (5 mL). Then an aqueous solution of 2 M Na2CO3 (6 mL) was added to the mixture. The resulting mixture was stirred at 100 °C for 24 h. The reaction mixture was concentrated by evaporation of solvents, and the residue was dissolved in dichloromethane, washed with water, and dried under anhydrous sodium carbonate. After the evaporation of solvent, the obtained product was purified by column chromatography (silica gel, dichloromethane/hexane = 1
:
4) (yield: 70%). ESI-MS: m/z, 284, [m + 1]+. 1H NMR (300 MHz, CDCl3) δ (ppm): 8.87 (d, J = 2.4 Hz, 1H), 8.08–8.05 (m, 1H), 7.97–7.91 (m, 3H), 7.60–7.48 (m, 5H). Calculated for C15H10BrN: C, 63.40; H, 3.55; N, 4.93. Found: C, 63.32; H, 3.45; N, 4.95.
Iridium(III) bis(5-bromo-2-(1-naphthalene)pyridine-C2, N)-3-bromo-5-(pyridine-2 -yl)-1,2,4-triazole [(1-NapyBr)2Ir(BrPytz)] (4).
Iridium trichloride hydrate (178 mg, 0.56 mmol), 5-bromo-2-(1-naphthalene) pyridine (477 mg, 1.68 mmol), 2-ethoxyethanol (15 mL), and water (5 mL) were added into a three-neck flask (100 mL). The mixture was refluxed under an argon atmosphere for 24 h and then cooled to room temperature. A red precipitate was filtered and washed with water and ethanol several times. The resulted red solid chloride-bridged dimer complex was purified by recrystallization in mixture of CH2Cl2 and hexane. Then the dried chloride-bridged dimer complex (476 mg, 0.078 mmol) was mixed with 3-bromo-5-(pyridin-2-yl)-1H-1,2,4-triazole (49 mg, 0.22 mmol) and sodium carbonate (13 mg) in degassed 2-ethoxyethanol (8 mL) in a three-necked flask. The mixture was refluxed in an argon atmosphere for 13 h. After cooling to room temperature the red precipitate was formed. After being filtered and washed with water and methanol, the precipitate was purified by column chromatography (silica gel, dichloromethane) to get the red crystal product (yield: 58%). ESI-MS: m/z, 983.1, [m + 1]+. 1H NMR (300 MHz, d6-DMSO) δ (ppm): 8.62 (t, J = 7.05 Hz, 2H), 8.57 (t, J = 4.86 Hz, 2H), 8.24 (d, J = 2.25 Hz, 1H), 8.21 (d, J = 2.34 Hz, 1H), 8.18–8.09 (m, 2H), 7.80 (t, J = 8.64 Hz, 2H), 7.70 (d, J = 2.19 Hz, 1H), 7.63 (d, J = 2.10 Hz, 1H), 7.76–7.55 (m, 2H), 7.51 (d, J = 5.40 Hz, 1H), 7.45–7.36 (m, 4H), 7.32 (d, J = 8.43 Hz, 1H) 6.43 (d, J = 8.28 Hz, 1H), 6.29 (d, J = 8.37 Hz, 1H). 13C NMR (300 MHz, d-C6H4Cl2) δ (ppm): 128.84, 127.90, 127.56, 127.46, 126.81, 126.50, 126.42, 126.28, 126.20, 126.10, 125.66, 125.47, 125.22, 125.13, 124.26, 124.23, 124.01, 123.76, 123.20, 122.94, 122.68, 122.46, 122.40, 121.34. Calculated for C37H22Br3IrN6: C, 45.23; H, 2.26; N, 8.55. Found: C, 45.11; H, 2.20; N, 8.43.
Iridium(III) bis(5-bromo-2-(1-naphthalene)pyridine-C2,N)-5-(pyridine-2-yl)-1,2,4-triazole [(1-NapyBr)2Ir(Pytz)]
.
Iridium trichloride hydrate (178 mg, 0.56 mmol), 5-bromo-2-(1-naphthalene) pyridine (477 mg, 1.68 mmol), 2-ethoxyethanol (15 mL), and water (5 mL) were added to a three-neck flask (100 mL). The mixture was refluxed under an argon atmosphere for 24 h and then cooled to room temperature. A red precipitate was filtered and washed with water and ethanol several times. The resulted red solid chloride-bridged dimer complex was purified by recrystallization in mixture of CH2Cl2 and hexane. Then the dried chloride-bridged dimer complex (158.7 mg, 0.1 mmol) was mixed with 5-(pyridin-2-yl)-1H-1,2,4-triazole (43.8 mg, 0.3 mmol) and sodium carbonate (10.6 mg, 0.1 mmol) in degassed 2-ethoxyethanol (20 mL) in a three-necked flask. The mixture was refluxed in an argon atmosphere for 13 h. After cooling to room temperature the red precipitate was formed. After being filtered and washed with water and methanol, the precipitate was purified by column chromatography (silica gel, dichloromethane) to get the red crystal product (yield: 64%). ESI-MS: m/z, 903.3, [m + 1]+. 1H NMR (300 MHz, d6-DMSO) δ (ppm): 8.63–8.53 (4H), 8.24–8.11 (4H), 7.79 (2H), 7.68 (1H), 7.61–7.57 (4H), 7.50–7.41 (4H), 7.33 (2H), 6.42 (1H), 6.28 (1H). Calculated for C37H23Br2IrN6: C, 49.18; H, 2.57; N, 9.30. Found: C, 49.11; H, 2.49; N, 9.20.
2-(4,4,5,5-Tetramethyl-1,3,2-dioxaborolan-2-yl)-9,9-dioctylfluorene
41 (5).
To a solution of 2-bromo-9,9-dioctylfluorene (4.69 g, 10 mmol) in 100 ml of dry THF, then cooled to −78 °C. N-Butyllithium (2.5 M, 15 mmol) was added dropwise to the solution, resulting a white viscous slurry. The mixture was stirred for 2 h at this temperature. Then 2-isopropoxy-4,4,5,5-tetramethyl-1,3,2-dioxaboralane (4.1 mL, 20 mmol) was added in one portion. The reaction mixture was stirred for another 1 h at −78 °C, then up to room temperature for another 24 h. After the reaction was complete, the mixture was poured into 300 ml of ice-water, and extracted with dichloromethane three times. The organic layer was combined and washed with water, brine and water, dried over MgSO4. After removal of the solvent, the product was purified by gel column chromatography using petroleum ether-dichloromethane (v/v = 8/2) mixture as eluent to give a white solid (yield: 87%). 1H NMR 300 MHz, CDCl3) δ (ppm): 7.80 (d, J = 7.3 Hz, 1 H), 7.72 (m, 3 H), 7.32 (m, 3 H), 1.97 (m, 4 H), 1.38 (s, 12 H), 1.25–1.09 (m, 20 H), 0.81 (t, J = 6.6 Hz, 6 H), 0.59 (m, 4 H).
Model compound
Iridium(III) bis(5-(fluorene-2-yl)-2-(1-naphthalene)pyridine-C2,N)-3-(fluorene-2-yl)-5-(pyridine-2-yl)-1,2,4-triazole (6).
Under argon atmosphere, a solution of monomer 5 (90 mg, 0.174 mmol), monomer 4 (50 mg, 0.05 mmol), Pd(OAc)2 (22.5 mg), and tricyclohexylphosphine (45 mg) in a mixed solution of toluene-THF (1
:
1, 10 ml) was stirred and heated to 80 °C, the Et4NOH (35%) aqueous solution (0.4 mL) was added. The solution was kept in the range of 80–85 °C with vigorous stirring for 20 h. The product was purified by gel column chromatography using petroleum ether, and then petroleum ether-dichloromethane (v/v = 8/2) mixture as eluent. The eluent was concentrated and the product was washed with hot methanol, then cooled and filtered off to give the targeted compound (yield: 63%). ESI-MS, m/z, 1908.5, [m + 1]+. 1H NMR (300 MHz, CDCl3) δ (ppm): 8.79 (1 H), 8.64–8.61 (m, 3 H), 8.18 (s, 1H), 8.03 (m, 2 H), 7.87–7.55 (m, 14 H), 7.51 (t, J = 7.50 Hz, 2 H), 7.38–7.19 (m, 13 H), 6.97 (m, 2 H), 6.90 (d, J = 7.50 Hz, 1 H), 6.59 (m, 1H), 6.49–6.42 (m, 2H), 6.11 (m, 1H), 1.98 (m, 12 H), 1.20–1.06 (br, 60 H), 0.81 (m, 18 H), 0.64–0.56 (m, 12 H).
General procedures for Suzuki polycondensation, with PFCzPh20NapyIr1 as an example:
2,7-Bis(4,4,5,5-tetramethyl-1,3,2-dioxaborolan-2-yl)-9,9-dioctylfluorene (321.3 mg, 0.5 mmol), 3,6-dibromo-N-(2-ethylhexyl)-carbazole (168.3 mg, 0.385 mmol), 1-(4,4,5,5-tetramethyl-1,3,2- dioxaborolan-2-yl)-3,5-dibromobenzene (72.4 mg, 0.2 mmol), (1-NapyBr)2Ir(BrPytz) (9.8 mg, 0.01 mmol), tricyclehexylphosphor (4 mg) and palladium(II) acetate (2 mg) were dissolved in a mixed solution of toluene/THF (1
:
1, 12 ml), stirred for 0.5 h under argon atmosphere, and then deionized water (2 ml) and Et4NOH (35%) aqueous solution (1 ml) were added. The mixture was refluxed with vigorous stirring for 2 days under argon. Then the polymer was capped by adding benzeneboronic acid (20 mg) by continuous stirring for 12 h, and then bromobenzene (0.2 ml) followed by continuously reacting for another 12 h. The whole mixture was poured into methanol. The precipitated polymer was recovered by filtration and purified by neutral alumina column chromatography with toluene to remove the small molecular complex and catalyst residue. Then the copolymer in toluene was poured into methanol and then filtrated to gain the slightly yellow solid (yield: 56%).1H NMR (300 MHz, CDCl3) δ (ppm): 8.52, 7.84–7.75, 7.52, 4.28, 2.16, 1.44–1.28, 1.14, 0.93–0.77. 13C NMR (300 MHz, CDCl3) δ (ppm): 151.72, 140.90, 140.84, 139.58, 128.76, 126.17, 125.51, 123.61, 121.67, 119.91, 118.89, 109.36, 55.38, 40.68, 39.61, 31.82, 31.14, 30.13, 29.72, 29.27, 28.98, 24.51, 23.92, 23.11, 22.61, 14.04, 10.95.
PFCzNapyIr1.
2,7-Bis(4,4,5,5-tetramethyl-1,3,2-dioxaborolan-2-yl)-9,9-dioctylfluorene (321.3mg, 0.5 mmol), 3,6-dibromo-N-(2-ethylhexyl)-carbazole (212.0 mg, 0.485 mmol), (1-NapyBr)2Ir(BrPytz) (9.8 mg, 0.01 mmol) were used in the polymerization (yield: 67%). 1H NMR (300 MHz, CDCl3) δ (ppm): 8.53, 7.88–7.53, 4.28, 2.17, 1.45–0.77. 13C NMR (300 MHz, CDCl3) δ (ppm): 151.71, 140.90, 140.83, 139.57, 133.05, 126.16, 125.52, 123.61, 121.66, 119.90, 118.87, 109.34, 55.37, 47.72, 40.68, 39.60, 31.82, 31.13, 30.13, 29.70, 29.26, 28.97, 24.49, 23.92, 23.11, 22.60, 14.08, 14.04, 10.95
PFCzNapyIr2.
2,7-Bis(4,4,5,5-tetramethyl-1,3,2-dioxaborolan-2-yl)-9,9-dioctylfluorene (321.3 mg, 0.5 mmol), 3,6-dibromo-N-(2-ethylhexyl)-carbazole (205.5 mg, 0.47 mmol), (1-NapyBr)2Ir(BrPytz) (19.6 mg, 0.02 mmol) were used in the polymerization (yield: 65%). 1H NMR (300 MHz, CDCl3) δ (ppm): 8.53, 7.88–7.75, 7.55–7.53, 4.28, 2.17, 1.46–1.28, 1.14, 1.02–0.77. 13C NMR (300 MHz, CDCl3) δ (ppm): 151.70, 140.88, 140.82, 139.56, 133.04, 126.15, 125.51, 123.60, 121.64, 119.89, 118.87, 109.34, 55.36, 47.70, 40.65, 39.58, 31.81, 31.12, 30.12, 29.71, 29.25, 28.96, 24.49, 23.91, 23.10, 22.60, 14.07, 14.03, 10.94.
PFCzNapyIr3.
2,7-Bis(4,4,5,5-tetramethyl-1,3,2-dioxaborolan-2-yl)-9,9-dioctylfluorene (321.3mg, 0.5 mmol), 3,6-dibromo-N-(2-ethylhexyl)-carbazole (198.9 mg, 0.455 mmol), (1-NapyBr)2Ir(BrPytz) (29.5mg, 0.03 mmol) were used in the polymerization (yield: 63%). 1H NMR (300 MHz, CDCl3) δ (ppm): 8.53, 7.88–7.75, 7.55–7.53, 4.28, 2.17, 1.46–1.28, 1.14, 1.02–0.76. 13C NMR (300 MHz, CDCl3) δ (ppm): 151.71, 140.90, 140.84, 139.58, 133.06, 126.16, 125.50, 123.61, 121.66, 119.91, 118.87, 109.34, 55.38, 47.74, 40.66, 39.60, 31.82, 31.14, 30.13, 29.72, 29.26, 28.97, 24.50, 23.92, 23.11, 22.61, 14.08, 14.04, 10.95.
PFCzPh10NapyIr1.
2,7-Bis(4,4,5,5-tetramethyl-1,3,2-dioxaborolan-2-yl)-9,9-dioctylfluorene (321.3 mg, 0.5 mmol), 3,6-dibromo-N-(2-ethylhexyl)-carbazole (190.2 mg, 0.435 mmol), 1-(4,4,5,5-tetramethyl-1,3,2-dioxaborolan-2-yl)-3,5-dibromo-benzene (36.2 mg, 0.1 mmol), (1-NapyBr)2Ir(BrPytz) (9.8 mg, 0.01 mmol) were used in the polymerization (yield: 60%). 1H NMR (300 MHz, CDCl3) δ (ppm): 8.53, 7.88–7.76, 7.56–7.53, 4.28, 2.18, 1.47–1.28, 1.14, 1.02–0.92, 0.84–0.77. 13C NMR (300 MHz, CDCl3) δ (ppm): 151.69, 140.88, 140.81, 139.56, 133.04, 126.14, 125.50, 123.59, 121.64, 119.88, 118.86, 109.34, 55.35, 40.65, 39.60, 31.81, 31.12, 30.12, 29.71, 29.25, 28.96, 24.45, 23.90, 23.10, 22.59, 14.03, 10.94
PFCzPh20NapyIr2.
2,7-Bis(4,4,5,5-tetramethyl-1,3,2-dioxaborolan-2-yl)-9,9-dioctylfluorene (321.3mg, 0.5 mmol), 3,6-dibromo-N- (2-ethylhexyl)-carbazole (161.8mg, 0.37 mmol), 1-(4,4,5,5-tetramethyl-1,3,2-dioxaborolan-2-yl) -3,5-dibromo- benzene (72.4mg, 0.2 mmol),(1-NapyBr)2Ir(BrPytz) (19.6mg, 0.02 mmol) were used in the polymerization(yield: 54%). 1H NMR (300 MHz, CDCl3) δ (ppm): 8.52, 7.85–7.74, 7.55–7.53, 4.26, 2.15, 1.44–1.27, 1.13, 1.03–0.76. 13C NMR (300 MHz, CDCl3) δ (ppm):151.71, 140.90, 140.84, 139.58, 133.07, 126.16, 125.53, 123.62, 121.66, 119.91, 118.89, 109.36, 55.38, 40.67, 39.57, 31.82, 31.14, 30.13, 29.72, 29.27, 28.98, 24.50, 23.93, 23.11, 22.61, 14.04, 10.95.
PFCzPh20NapyIr3.
2,7-Bis(4,4,5,5-tetramethyl-1,3,2-dioxaborolan-2-yl)-9,9-dioctylfluorene (321.3 mg, 0.5 mmol), 3,6-dibromo-N-(2-ethylhexyl)-carbazole (155.2 mg, 0.355mmol), 1-(4,4,5,5-tetramethyl-1,3,2-dioxaborolan-2-yl)-3,5-dibromo-benzene (72.4 mg, 0.2 mmol), (1-NapyBr)2Ir(BrPytz) (29.5mg, 0.03 mmol) were used in the polymerization (yield: 45%). 1H NMR (300 MHz, CDCl3) δ (ppm): 8.51, 7.84–7.51, 4.26, 2.15, 1.54–1.27, 1.12, 0.91–0.77. 13C NMR (300 MHz, CDCl3) δ (ppm): 151.70, 140.90, 139.57, 133.05, 126.15, 123.61, 121.64, 119.90, 118.85, 109.35, 67.97, 55.37, 40.62, 31.81, 31.14, 30.11, 29.25, 28.96, 25.62, 24.49, 23.91, 23.10, 22.60, 14.03, 10.94.
L-PFCzNapyIr2
.
2,7-Bis(4,4,5,5-tetramethyl-1,3,2-dioxaborolan-2-yl)-9,9-dioctylfluorene (321.3 mg, 0.5 mmol), 3,6-dibromo-N-(2-ethylhexyl)-carbazole (205.5 mg, 0.47 mmol), (1-NapyBr)2Ir(Pytz) (18.1 mg, 0.02 mmol) were used in the polymerization (yield: 62%). 1H NMR (300 MHz, CDCl3) δ (ppm): 8.51, 7.86–7.84, 7.78–7.73, 7.61–7.51, 4.26, 2.15, 1.66, 1.55, 1.44–1.42, 1.34–1.26, 1.12, 0.99–0.89, 0.79–0.74. 13C NMR (300 MHz, CDCl3) δ (ppm): 151.71, 140.89–140.83, 139.58, 133.05, 126.17, 125.53, 123.61, 121.65, 119.90, 118.88, 109.34, 55.37, 40.68, 39.59, 31.82, 31.13, 30.13, 29.26, 28.97, 24.49, 23.92, 23.11, 22.61, 14.08, 14.04, 10.95.
LED fabrication and characterization
The PLEDs were fabricated on ITO-covered glass substrates. Patterned indium tin oxide (ITO)-coated glass substrates were cleaned with acetone, detergent, distilled water, and isopropyl alcohol, subsequently in an ultrasonic bath. After treatment with oxygen plasma, 50 nm of poly(3,4-ethylenedioxythiophene) (PEDOT) doped with poly(styrenesulfonic acid) (PSS; Batron-P 4083, Bayer AG) was spin-coated onto the ITO substrate followed by drying in a vacuum oven at 80 °C for 8 h. The polymers were dissolved in toluene and filtered through a 0.45 μm filter. A thin film of polymer was coated onto the anode by spin-casting inside a dry box. The film thickness of the active layers was around 80 nm, as measured with an Alfa Step 500 surface profiler (Tencor). A thin layer Ba (4 nm), and a layer of Al (120 nm) were subsequently vacuum-evaporated onto the top of the EL polymer layer under vacuum of 1 × 10−4 Pa. Device performances were measured inside a dry box. Current–voltage (I–V) characteristics were recorded by a Keithley 236 source meter. EL spectra were recorded by Oriel Instaspec IV CCD spectrograph. Luminance was measured by a PR 705 photometer (Photo Research). The external quantum efficiencies were determined by a Si photodiode with calibration in an integrating sphere (IS080, Labsphere).
Results and discussion
Synthesis of complexes and copolymers
The synthetic routes for the iridium complex monomers and the model compound are depicted in Scheme 1. 5-Bromo-2-(1-naphthalene) pyridine was synthesized via a Suzuki coupling reaction of 2,5-dibromopyridine and 1-naphthaleneboronic acid in high yield. The iridium complex (4) was synthesized from chloride-bridged iridium dimer and 3-bromo-5-(pyridin-2-yl)-1H-1,2,4-triazole ligand. Three bromines in the ligands of the complex (4) were used for polymerization to obtain the hyperbranched copolymers. However, it should be noted that the reactivity of the two bromines attached to the 2-(1-naphthalene) pyridine ligand and the one bromine attached to the 5-(pyridin-2-yl)-1H-1,2,4-triazole ligand in the iridium complex (4) might potentially not be equivalent, thus if there are only two equivalent bromines attached to the pyridine or only the bromine on the triazole moiety reacted in the polymerization, there would be no polymer with hyperbranched architecture. To illustrate this point, we prepared the model compound (6) which was synthesized by using three bromines substituted iridium complex (4) and fluorene mono-boronic pinacol ester (5). It was found that the model compound (6) could be fabricated in comparatively high yield via Suzuki coupling reaction. The fact indicated that the potential differences between the reactivity of the three bromines in iridium complex (4) have little effect on the Suzuki coupling procedure, thus it is reasonable to expect that the employed iridium complex (4) could act as an effective core for the designed hyperbranched polymers.
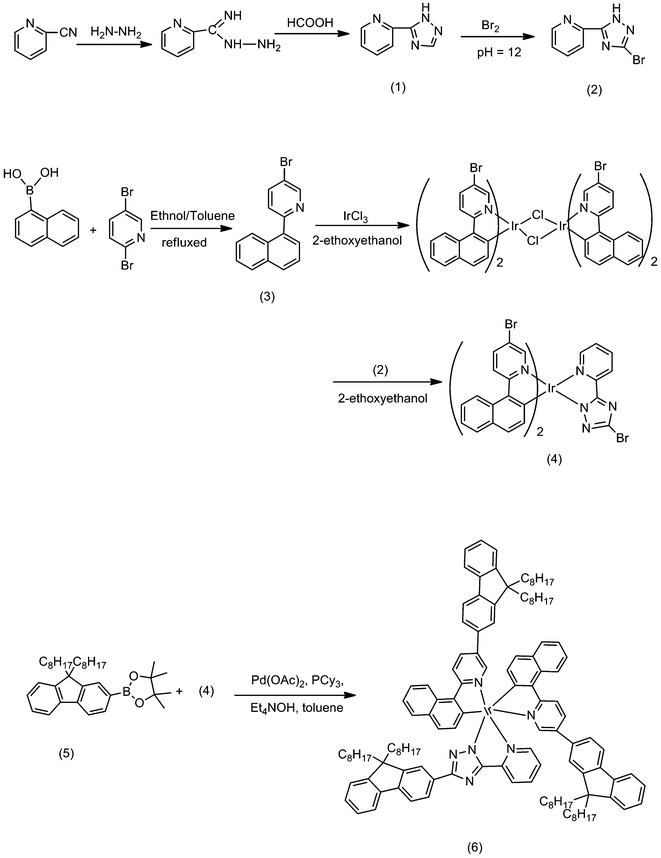 |
| Scheme 1 Synthetic routes for monomers and model compound. | |
The hyperbranched copolymers were synthesized from 2,7-bis(4,4,5,5-tetramethyl-1,3,2-dioxaborolan-2-yl)-9,9-dioctylfluorene, 3,6-dibromo-N-(2-ethylhexyl)-carbazole,1-(4,4,5,5-tetramethyl-1,3,2- dioxaborolan-2-yl)-3,5-dibromobenzene, and the (1-NapyBr)2Ir(BrPytz) complex by one-pot Suzuki polycondensation (Scheme 2). The feed ratios of complexes in the polycondensation were 1, 2 and 3 mol% and 1-(4,4,5,5-tetramethyl-1,3,2-dioxaborolan-2-yl)-3,5-dibromobenzene were 0, 10 and 20 mol%, and the corresponding copolymers were named PFCzNapyIr1, PFCzNapyIr2, PFCzNapyIr3, PFCzPh10NapyIr1, PFCzPh20NapyIr1, PFCzPh20NapyIr2 and PFCzPh20NapyIr3, respectively. For comparing with the hyperbranched copolymers, the linear polymer derived from monomers 2,7-bis(4,4,5,5-tetramethyl-1,3,2-dioxaborolan-2-yl)-9,9-dioctylfluorene, 3,6-dibromo-N-(2- ethylhexyl)-carbazole and (1-NapyBr)2Ir(Pytz) was prepared by Suzuki polycondensation with a feed ratio of 50
:
47
:
2, and the resulted polymer was named L-PFCzNapyIr2.
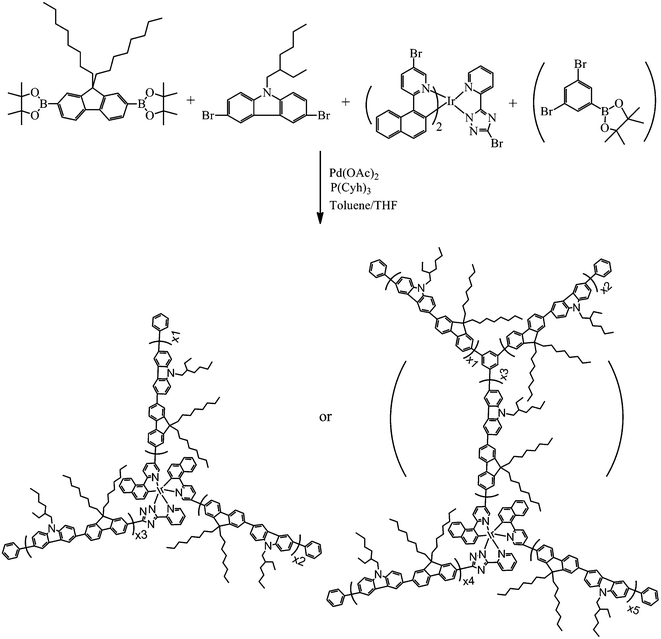 |
| Scheme 2 Synthetic route for hyperbranched copolymers. | |
All synthesized hyperbranched polymers are soluble in common organic solvents, such as chloroform, toluene and 1,2-dichloroethane. In order to avoid producing insoluble cross-linked copolymers in the step-growth polymerization, the reaction conditions such as temperature, reaction time, concentration and solvent should be considered. Suzuki polycondensation was carried out at relatively low temperature and the 3,6-carbazole-alt-2,7-fluorene unit was used as the branch. So insoluble cross-linked copolymers could be avoided.26,34 The monomers 2,7-bis(4,4,5,5-tetramethyl-1,3,2-dioxaborolan-2-yl)-9,9-dioctylfluorene, 3,6-dibromo-N-(2-ethylhexyl)-carbazole, 1-(4,4,5,5-tetramethyl-1,3,2-dioxaborolan-2-yl)-3,5-dibromobenzene, and (1-NapyBr)2Ir(BrPytz) in different molar ratios and tricyclohexyl phosphine (4 mg) and palladium(II) acetate (2 mg) were added in toluene/THF (1
:
1, 12 ml) over 0.5 h under argon atmosphere, and then deionized water (2 ml) and Et4NOH (35%) aqueous solution (1 ml) were added. The mixture was heated slowly to reflux with vigorous stirring, and then reacted for 2 days under argon. Because the hyperbranched copolymers synthesized by one-pot Suzuki approach have a large number of bromo- and boronic ester-end groups, which are detrimental for light-emitting applications, monobromo- and monoboronic acid-substituted monomers were therefore used to cap the end groups. The copolymers was capped by adding benzeneboronic acid under stirring for 6 h, and then bromobenzene followed by continuously reacting for another 6 h after the mixture were reacted for 2 days. The copolymers with slightly yellow color were obtained.
The iridium contents in the copolymers were estimated by X-ray fluorescence spectrometry (XRF), and the molar ratios of Ir complexes incorporated into the copolymers were calculated by XRF data (Table 1). The results indicate that the actual Ir complex contents in the copolymers are substantially lower than the feed ratios of complex monomers, and increase with the increasing feed ratios. Meanwhile, the Ir complex contents in these copolymers are also different even at the same feed ratios of complex monomers.46
Table 1 Molecular weight and thermal properties of the copolymers
Polymer
|
M
n × 104 |
PDI |
Ir complex in (mol%) |
T
g (°C) |
T
d (°C) |
feed ratio |
polymer
|
PFCzNapyIr1 |
1.8 |
1.30 |
1 |
0.68 |
130 |
440 |
PFCzNapyIr2 |
1.85 |
1.32 |
2 |
1.01 |
129 |
440 |
PFCzNapyIr3 |
2.58 |
1.62 |
3 |
1.53 |
134 |
440 |
PFCzPh10NapyIr1 |
11.9 |
1.68 |
1 |
0.55 |
141 |
428 |
PFCzPh20NapyIr1 |
20.5 |
2.42 |
1 |
0.52 |
— |
432 |
PFCzPh20NapyIr2 |
19.6 |
2.30 |
2 |
1.23 |
— |
443 |
PFCzPh20NapyIr3 |
12.9 |
1.93 |
3 |
1.63 |
— |
430 |
L-PFCzNapyIr2
|
2.65 |
1.45 |
2 |
1.17 |
132 |
426 |
The number-average molecule weights (Mn) of the synthesized hyperbranched copolymers determined by gel permeation chromatography (GPC) are from 18,200 to 205,000 with a polydispersity index (PDI) from 1.30 to 2.42 (Table 1). The thermogravimetric analysis (TGA) and differential scanning calorimetry (DSC) are shown in Fig. 1. The glass transition temperatures (Tg) of the hyperbranched copolymers are from 129 to 134 °C. TGA reveals high thermal stabilities of these copolymers with the onset decomposition temperature (Td) of a 5% weight loss are from 428 to 440 °C under nitrogen.
Photophysical properties
The UV-vis absorption of the (1-NapyBr)2Ir(BrPytz) complex and the PL spectra of fluorene-alt-carbazole copolymer (PFCz) are shown in Fig. 2. The intense absorption peaks below 300 nm are attributed to the spin-allowed singlet state 1(π–π*) transition of cyclometalated ligands and the weak absorption peaks around 335 nm can be assigned to the spin-allowed singlet metal-to-ligand charge-transfer (1MLCT) transition. The absorption peaks around 470 nm are due to the triplet metal-to-ligand charge-transfer (3MLCT) transition for the complex. The spin-forbidden 3MLCT transition is gained by mixing it with the higher spin-allowed 1MLCT transition through the strong spin–orbit coupling of iridium. Fig. 2 indicates that the PL emission of the host copolymer (PFCz) and the absorption spectra of the guest (Ir complex) show good spectra overlap. Therefore, the efficient Förster energy transfer from the PFCz host to the Ir complex guest can be expected.
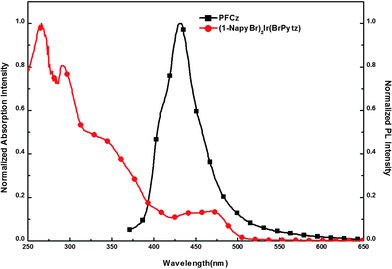 |
| Fig. 2
UV-vis absorption of (1-NapyBr)2Ir(BrPytz) complex in CHCl3 solution and PL spectra of PFCz in film. | |
The UV-vis absorption spectra of the copolymers are shown in Fig. 3. The absorption spectra of the hyperbranched copolymers are dominated by a single peak with an absorbance maximum (λabs) around 350 nm, which can be attributed to the π–π* transitions of PFCz backbones.47 However, no distinct absorption peak corresponding to the Ir complex was found due to the comparatively low content of Ir complex in these copolymers. The absorption spectra peaked at about 350 nm slightly blue-shift by 4–5 nm with the increasing phenyl moieties, which might attribute to the interruption of the delocalization of π-electrons along the copolymer backbones by 1,3,5-phenyl linkages. The result indicated that the absorption maxima are blue-shifted in analogous systems with the increasing contents of kinked or branching units.48 Moreover, it can be seen that the absorption edges of the films are almost identical for all copolymers. As estimated from the band edges of absorption spectra in film, the optical band gaps (Eg) varied slightly from 3.04 to 3.09 eV.
PL spectra of the copolymers are shown in Fig. 4. The photophysical properties of the copolymers are listed in Table 2. As shown in Fig. 4a, PL spectra of the hyperbranched copolymers in CHCl3 solution are strikingly similar to each other, which show a maximum band around 400 nm along with a vibronic band 414 nm. PL spectra of the PFCzPh20NapyIr3 in CHCl3 solution are evidently red-shift compared with other polymers. This may be due to the increase of conjugated length in copolymer. In general, the presence of well-defined vibronic structure in the emission spectra indicates that the copolymers have rigid and well-defined backbone structures.48 The films were excited at 325 nm in which the absorption of 1MLCT and 3MLCT bands of the phosphorescent segment don't fall, therefore, there should be no direct excitation from the phosphorescent segment. As shown in Fig. 4b, the maximum emissions of the hyperbranched copolymers in film are at around 420 nm, as same as PFCz host segment, and 620 nm and 663 nm from the (1-Napy)2Ir(Pytz) complex. The relative intensities at the 420 nm vary with the Ir complex content in the copolymers. With the increase of Ir complex content, the emission intensity of 420 nm from the PFCz segment descends, whilst the emission intensity of Ir complex increases. At more than 2 mol% (actual 1.01 mol%) iridium complex in the copolymers, the emission from complex became stronger than that of PFCz segment. This could be the result of the Förster energy transfer from PFCz segment to the complex core, since the 3MLCT absorption of phosphorescent complex is in good overlap with PL emission of the PFCz segment (Fig. 2). The emission peaked at 420 nm still can be observed for the hyperbranched copolymers with high Ir complex content (1.63 mol %). This implies that the energy transfer from the PFCz main chain to Ir complex is incomplete, probably because the average distance from a photoexcited polymer chain to the nearest Ir complex is too long. For hyperbranched copolymers, the efficiency of energy transfer from PFCz segment to the Ir complex is enhanced with the increase of the content of 1,3,5-linked benzene core in the copolymers. In the dilute CHCl3 solution, energy transfer is exclusively intramolecular, while in film, both intra- and interchain interactions contribute to energy transfer. Significantly, more efficient energy transfer of the copolymers unambiguously indicates that intermolecular energy transfer is a more efficient process. Hyperbranched copolymers can increase PL efficiency, because the concentration quenching and T–T annihilation are greatly decreased. The PL efficiency increases from 34 to 44 by introducing phenyl moiety into the PFCzNapyIr3 copolymer. The values of PL efficiencies and the maximum emission wavelength of the copolymers are listed in Table 2.
Table 2 Photophysical and electrochemical properties of copolymers
Polymer
|
λ
abs,film (nm) |
λ
PL,sol
(nm) |
λ
PL,film (nm) |
Q
PL
(%) |
E
ox
(V) |
HOMO (eV) |
E
g (eV) |
LUMOa (eV) |
Estimated from HOMO levels and the optical band gaps from the absorption spectra.
|
PFCzNapyIr1 |
346 |
403 |
411,616 |
34 |
0.87 |
−5.27 |
3.05 |
−2.22 |
PFCzNapyIr2 |
346 |
402 |
409,617 |
37 |
0.88 |
−5.28 |
3.05 |
−2.23 |
PFCzNapyIr3 |
349 |
404 |
407,618 |
34 |
0.88 |
−5.28 |
3.04 |
−2.24 |
PFCzPh10NapyIr1 |
344 |
400 |
408,613 |
40 |
0.88 |
−5.28 |
3.08 |
−2.20 |
PFCzPh20NapyIr1 |
342 |
401 |
409,609 |
45 |
0.87 |
−5.27 |
3.09 |
−2.18 |
PFCzPh20NapyIr2 |
342 |
401 |
403,616 |
42 |
0.87 |
−5.27 |
3.08 |
−2.19 |
PFCzPh20NapyIr3 |
342 |
418 |
401,612 |
44 |
0.90 |
−5.30 |
3.09 |
−2.21 |
Electrochemical characteristics
The redox behavior of copolymers was investigated by cyclic voltammetry (CV) shown in Fig. 5. All copolymers show a partial reversible oxidation wave with onset ca. 0.87 V (Table 2). The HOMO levels of copolymers were calculated according to the empirical formulas EHOMO = −(Eox + 4.4) (eV).49 The optical band gap was obtained from Eg = 1240/λedge, where the edge is the onset value of the absorption spectrum in film in the long-wavelength direction.41,49–51 From the HOMO levels and the optical gaps, the LUMO levels of copolymers could be determined. The HOMO level of (1-NapyBr)2Ir(BrPytz) was evaluated at −5.4 eV and the LUMO level (−3.0 eV) was obtained from the onset of absorption and HOMO level. On the assumption of no great change in the LUMO level of (1-NapyBr)2Ir(BrPytz) incorporated into host copolymer chain, the Ir complex will function as an electron trap. The band gap (Eg) of PFCzNapyIr1-3 calculated from the onset absorption in film are about 3.05 eV. The HOMO levels of all the hyperbranched copolymers show no essential differences. It is also noted that the HOMO levels of copolymers (from −5.27 to −5.30 eV) are relatively close to the work function of PEDOT (−5.20 eV), thus excellent hole injection ability is expected, while the LUMO values increased to −2.22 eV and −2.18 eV for PFCzNapyIr1 and PFCzPh20NapyIr1, respectively, while the LUMO levels of copolymers (from −2.18 eV to −2.22 eV), are comparatively distant to the work function of Ba (−2.80 eV), indicating that there is a barrier for electron injection.
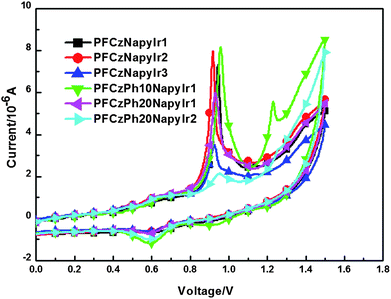 |
| Fig. 5
Cyclic voltammograms of the polymers. | |
Electroluminescent properties
The devices with the configuration of ITO/PEDOT:PSS (50 nm)/polymer + PBD (30 wt%) (75 nm)/TPBI (30 nm)/Ba (4 nm)/Al (120 nm) were fabricated. Here, 2-(4-bisphenylyl)-5-(4-tert-butyl-phenyl)-1,3,4-oxadiazole (PBD) was doped in the polymers to improve the electron-transporting capability,52,53 and 1,3,5-tris (2-N-phenylbenzimidazolyl) benzene (TPBI) was used as electron-transporting/hole-blocking layer.54 The electroluminescent (EL) spectra of the resulting devices are shown in Fig. 6. We can see that the emission from host segment in the copolymers is quenched completely although the Ir complex content is as low as 0.52 mol%, and only emission peaked at 620 nm is dominated by iridium complex, which is almost identical for all copolymers.
The substantial differences in PL and EL spectra imply that different mechanisms should be involved. Under photoexcitation, the singlet excited states are created on the host chain and subsequently transfer to the Ir complex by Förster energy transfer.54 In contrast, the electrons and holes are trapped on the Ir complex after the injection of the electrons from the cathode and the holes from the opposite anode in the device.4,55 In other words, the Ir complexes could function as both hole and electron trapping centers and dominate the EL process. Considering that the branch is connected directly with iridium complex in this hyperbranched framework, thereby the intramolecular energy transfer from the branch to the triplet metal complex would be comparatively efficient. Apart from this point, the branch connected with complex core could also act as spacer, which can increase the mutual distances of the iridium complexes. Therefore, the triplet–triplet (T–T) annihilation of iridium complexes could be well-suppressed in our hyperbranched system.56–58
As can be seen from Table 3, the device performances show a strong dependence on Ir complex content. The performance of PFCzNapyIr3 shows a maximal external quantum efficiency of 6.4%, a luminous efficiency of 4.8 cd A−1, with a CIE coordinate of (0.64, 0.31). We can also see that the external quantum efficiencies enhance from 5.0% for PFCzNapyIr1 to 8.1% for PFCzPh20NapyIr1 with the increase of phenyl core content in the copolymers. Indeed, it is noteworthy that the device performances are remarkably improved when 1,3,5-phenyl moiety as the core is incorporated into the polymer backbone. The best device performances were obtained from PFCzPh20NapyIr2. A maximal external quantum efficiency of 6.1% and a luminous efficiency of 4.7 cd A−1 with a CIE coordinate of (0.63, 0.32) were achieved at a current density of 2.6 mA cm−2. The fluorene-alt-carbazole based linear copolymer L-PFCzNapyIr2 was synthesized for comparison. The device from L-PFCzNapyIr2 exhibits a maximal external quantum efficiency of 2.1% and a luminous efficiency of 2.1 cd A−1, which are obviously lower than that of the hyperbranched copolymer PFCzPh20NapyIr2. The result shows that the hyperbranched structure has more efficient energy transfer and higher device performances compared with the linear polymer for the same system. The turn-on voltages of the devices increase due to the increased LUMO levels of the copolymers and the barrier of electron injection enhanced by introducing the phenyl core into the hyperbranched copolymers (Table 2).
Table 3 Device performances of hyperbranched copolymersa
Polymer
|
V
th (V) |
At the maxima of quantum efficiency |
L
max (cd m−2) |
CIE (x, y) |
V(V) |
J (mA cm−2) |
L (cd m−2) |
LE (cd A−1) |
QEext (%) |
Device structure: ITO/PEDOT/polymer + 30%PBD/TPBI/Ba/Al.
|
PFCzNapyIr1 |
5.8 |
6.6 |
0.78 |
33.2 |
4.3 |
5.0 |
2941 |
0.45, 0.22 |
PFCzNapyIr2 |
5.9 |
6.0 |
0.05 |
2.6 |
5.0 |
6.2 |
3178 |
0.55, 0.27 |
PFCzNapyIr3 |
5.9 |
6.9 |
0.43 |
20.4 |
4.8 |
6.4 |
2147 |
0.64, 0.31 |
PFCzPh10NapyIr1 |
5.9 |
6.0 |
0.04 |
2.2 |
6.2 |
7.8 |
3270 |
0.54, 0.28 |
PFCzPh20NapyIr1 |
6.0 |
6.6 |
0.17 |
11.4 |
6.5 |
8.1 |
2696 |
0.57, 0.30 |
PFCzPh20NapyIr2 |
6.5 |
9.0 |
2.6 |
117 |
4.7 |
6.1 |
2310 |
0.63, 0.32 |
PFCzPh20NapyIr3 |
5.1 |
7.5 |
1.3 |
57 |
4.3 |
6.8 |
1525 |
0.65, 0.34 |
L-PFCzNapyIr2
|
6.1 |
8.2 |
8.0 |
173 |
2.1 |
2.1 |
2054 |
0.60, 0.30 |
Fig. 7 shows the luminous efficiencies and the luminances of the devices as the function of current densities (LE–L–J) for the devices based on hyperbranched copolymers. It is clearly seen that polymers exhibit a gentle efficiency roll-off and the luminance increased readily with the increase of current density. The reduced efficiency roll-off is associated with the reduced concentration quenching of triplet excitons due to avoiding aggregation of the Ir complex in the branched molecular structure. At a high current density, the luminance decreases because of the destruction of the active materials under the high electrical field. Anyway, our results indicate that the strategy using a complex as the core in the hyperbranched polymer is a promising approach.
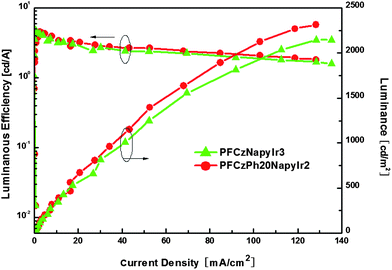 |
| Fig. 7
QE–J–L characteristics of the devices from PFCzNapyIr3 and PFCzPh20NapyIr2. | |
Conclusions
A series of electrophosphorescent hyperbranched 3,6-carbazole-alt-2,7-fluorene copolymers used iridium complex as core were synthesized by Suzuki polycondensation. Efficient energy transfer from 3,6-carbazole-alt-2,7-fluorene segment to iridium complex was observed even the Ir complex content was as low as 1 mol% in the copolymer. A peak external quantum efficiency of 6.1% and a luminous efficiency of 4.7 cd A−1, with a CIE coordinate of (0.63, 0.32) were achieved for the device from PFCzPh20NapyIr2. It is also found that the efficiency loss upon the increase of current density is significantly reduced since the highly branched structures could suppress the self-quenching of the Ir complex due to aggregation.
Acknowledgements
We thank the National Natural Science Foundation of China (Nos.21074038 and U0634003), and State Key Basical Research Project of China (Nos. 2009CB623601 and 2009CB930604) for the financial support.
References
- C. Adachi, M. A. Baldo, S. R. Forrest, S. Lamansky, M. E. Thompson and R. C. Kwong, Appl. Phys. Lett., 2001, 78, 1622 CrossRef CAS.
- C. Adachi, M. A. Baldo, M. E. Thompson and S. R. Forrest, J. Appl. Phys., 2001, 90, 5048 CrossRef CAS.
- S. Lamansky, P. Djurovich, D. Murphy, R. F. Abdel, H. E. Lee, C. Adachi, P. E. Burrows, S. R. Forrest and M. E. Thompson, J. Am. Chem. Soc., 2001, 123, 4304 CrossRef CAS.
- X. Gong, M. R. Robinson, J. C. Ostrowski, D. Moses, G. C. Bazan and A. J. Heeger, Adv. Mater., 2002, 14, 581 CrossRef CAS.
- C. Jiang, W. Yang, J. Peng, S. Xiao and Y. Cao, Adv. Mater., 2004, 16, 537 CrossRef CAS.
- X. Chen, J. L. Liao, Y. Liang, M. O. Ahmed, H. E. Tseng and S. A. Chen, J. Am. Chem. Soc., 2003, 125, 636 CrossRef CAS.
- J. Jiang, C. Jiang, W. Yang, H. Zhen, F. Huang and Y. Cao, Macromolecules, 2005, 38, 4072 CrossRef CAS.
- A. J. Sandee, C. K. Williams, N. R. Evans, J. E. Davies, C. E. Boothby, A. Koehler, R. H. Friend and A. B. Holmes, J. Am. Chem. Soc., 2004, 126, 7041 CrossRef CAS.
- H. Zhen, C. Jiang, W. Yang, J. Jiang, F. Huang and Y. Cao, Chem.–Eur. J., 2005, 11, 5007 CrossRef CAS.
- H. Zhen, C. Luo, W. Yang, W. Song, B. Du, J. Jiang, C. Jiang, Y. Zhang and Y. Cao, Macromolecules, 2006, 39, 1693 CrossRef CAS.
- S. Liu, Q. Zhao, R. Chen, Y. Deng, Q. Fan, F. Li, L. Wang, C. Huang and W. Huang, Chem.–Eur. J., 2006, 12, 4351 CrossRef CAS.
- H. Shi, S. Liu, H. Sun, W. Xu, Z. An, J. Chen, S. Sun, X. Lu, Q. Zhao and W. Huang, Chem.–Eur. J., 2010, 16, 12158 CrossRef CAS.
- S. Liu, W. Xu, T. Ma, Q. Zhao, Q. Fan, Q. Ling and W. Huang, Macromol. Rapid Commun., 2010, 31, 629 CrossRef CAS.
- Q. Zhao, S. Liu and W. Huang, Macromol. Rapid Commun., 2010, 31, 794 CrossRef CAS.
- J. Bettenhausen, M. Greczmiel, M. Jandke and P. Strohriegl, Synth. Met., 1997, 91, 223 CrossRef CAS.
- Y. H. Kim, J. Polym. Sci., Part A: Polym. Chem., 1998, 36, 1685 CrossRef CAS.
- B. J. Voit, J. Polym. Sci., Part A: Polym. Chem., 2000, 38, 2505 CrossRef CAS.
- U. Hahn, M. Gorka, F. Vogtle, V. Vicinelli, P. Ceroni, M. Maestri and V. Balzani, Angew. Chem., Int. Ed., 2002, 41, 3595 CrossRef CAS.
- P. Furuta, J. Brooks, M. E. Thompson and J. M. Frechet, J. Am. Chem. Soc., 2003, 125, 13165 CrossRef CAS.
- N. Satoh, J. S. Cho, M. Higuchi and K. Yamamoto, J. Am. Chem. Soc., 2003, 125, 8104 CrossRef CAS.
- P. W. Wang, Y. J. Liu, C. Devadoss, P. Bharathi and J. S. Moore, Adv. Mater., 1996, 8, 237 CrossRef CAS.
- S. M. Grayson and J. M. Frechet, Chem. Rev., 2001, 101, 3819 CrossRef CAS.
- C. J. Hawker and K. L. Wooley, Science, 2005, 309, 1200 CrossRef CAS.
- M. Adeli and R. Haag, J. Polym. Sci., Part A: Polym. Chem., 2006, 44, 5740 CrossRef CAS.
- Q. He, H. Huang, Q. Sun, H. Lin, J. Yang and F. Bai, Polym. Adv. Technol., 2004, 15, 43 CrossRef CAS.
- C. Gao and D. Yan, Prog. Polym. Sci., 2004, 29, 183 CrossRef CAS.
- L. Tsai and Y. Chen, J. Polym. Sci., Part A: Polym. Chem., 2007, 45, 5541 CrossRef CAS.
- J. Li and Z. Bo, Macromolecules, 2004, 37, 2013 CrossRef CAS.
- G. K. Paul, J. Mwaura, A. A. Argun, P. Taranekar and J. R. Reynolds, Macromolecules, 2006, 39, 7789 CrossRef CAS.
- Z. X. Li, J. H. Liu, S. Y. Yang, S. H. Huang, J. D. Lu and J. L. Pu, J. Polym. Sci., Part A: Polym. Chem., 2006, 44, 5729 CrossRef CAS.
- G. A. Wen, Y. Xin, X. R. Zhu, W. J. Zeng, R. Zhu, J. C. Feng, Y. Cao, L. Zhao, L. H. Wang, W. Wei, B. Peng and W. Huang, Polymer, 2007, 48, 1824 CrossRef CAS.
- Q. Peng, L. Yan, D. Chen, F. Wang, P. Wang and D. Zou, J. Polym. Sci., Part A: Polym. Chem., 2007, 45, 5296 CrossRef CAS.
- X. M. Liu, T. Lin, J. Huang, X. T. Hao, K. S. Ong and C. He, Macromolecules, 2005, 38, 4157 CrossRef CAS.
- Y. Xin, G. A. Wen, W. J. Zeng, L. Zhao, X. R. Zhu, Q. L. Fan, J. C. Feng, L. H. Wang, W. Wei, J. B. Peng, Y. Cao and W. Huang, Macromolecules, 2005, 38, 6755 CrossRef CAS.
- B. Li, X. Xu, M. Sun, Y. Fu, G. Yu, Y. Liu and Z. Bo, Macromolecules, 2006, 39, 456 CrossRef CAS.
- T. W. Lee, Y. Kwon, J. J. Park, L. Pu, T. Hayakawa and M. Kakimoto, Macromol. Rapid Commun., 2007, 28, 1657 CrossRef CAS.
- P. Wang, C. Chai, Q. Yang, F. Wang, Z. Shen, H. Guo, X. Chen, X. Fan, D. Zou and Q. Zhou, J. Polym. Sci., Part A: Polym. Chem., 2008, 46, 5452 CrossRef CAS.
- W. Yang, H. Zhen, C. Jiang, L. Su, J. Jiang, H. Shi and Y. Cao, Synth. Met., 2005, 153, 189 CrossRef CAS.
- R. Guan, Y. Xu, L. Ying, W. Yang, H. Wu, Q. Chen and Y. Cao, J. Mater. Chem., 2009, 19, 531 RSC.
- W. Yang, Q. Hou, C. Liu, Y. Niu, J. Huang, R. Yang and Y. Cao, J. Mater. Chem., 2003, 13, 1351 RSC.
- M. Sun, J. Li, B. Li and Z. Bo, Macromolecules, 2005, 38, 2651 CrossRef CAS.
- J. Huang, Y. Niu, W. Yang, Y. Mo, M. Yuan and Y. Cao, Macromolecules, 2002, 35, 6080 CrossRef CAS.
- J. Roppe, N. D. Smith, D. Huang, L. Tehrani, B. Wang, J. Anderson, J. Brodkin, J. Chung, X. Jiang, C. King, B. Munoz, M. A. Varney, P. Prasit and N. D. P. Cosford, J. Med. Chem., 2004, 47, 4645 CrossRef CAS.
- B. Liang, C. Jiang, Z. Chen, X. Zhang, H. Shi and Y. Cao, J. Mater. Chem., 2006, 16, 1281 RSC.
- C. D. Pietro, S. Serroni, S. Campagna, M. T. Gandolfi, R. Ballardini, S. Fanni, W. R. Browne and J. G. Vos, Inorg. Chem., 2002, 41, 2871 CrossRef.
- H. Zhen, J. Luo, W. Yang, Q. Chen, L. Ying, J. Zou, H. Wu and Y. Cao, J. Mater. Chem., 2007, 17, 2824 RSC.
- Y. Li, J. Ding, M. Day, Y. Tao, J. Lu and M. D'Iorio, Chem. Mater., 2004, 16, 2165 CrossRef CAS.
- C. W. Wu and H. C. Lin, Macromolecules, 2006, 39, 7232 CrossRef CAS.
- J. L. Bredas, R. Silbey, D. S. Boudreaux and R. R. Chance, J. Am. Chem. Soc., 1983, 105, 6555 CrossRef CAS.
- B. Liu, W. Yu, Y. Lai and W. Huang, Chem. Mater., 2001, 13, 1984 CrossRef CAS.
- J. Pei, X. Liu, W. Yu, Y. Lai, Y. Niu and Y. Cao, Macromolecules, 2002, 35, 7274 CrossRef CAS.
- S. Lamansky, P. I. Djurovich, R. F. Abdel, S. Garon, D. L. Murphy and M. E. Thompson, J. Appl. Phys., 2002, 92, 1570 CrossRef CAS.
- B. Du, L. Wang, H. Wu, W. Yang, Y. Zhang, R. Liu, M. Sun, J. Peng and Y. Cao, Chem.–Eur. J., 2007, 13, 7432 CrossRef CAS.
- C. Gu, T. Fei, Y. Lv, T. Feng, S. Xue, D. Lu and Y. Ma, Adv. Mater., 2010, 22, 2702 CrossRef CAS.
- M. D. McGehee, T. Bergstedt, C. Zhang, A. P. Saab, M. B. O'Regan, G. C. Bazan, V. I. Srdanov and A. J. Heeger, Adv. Mater., 1999, 11, 1349 CrossRef CAS.
- J. P. J. Markham, S.-C. Lo, S. W. Magennis, P. L. Burn and I. D. W. Samuel, Appl. Phys. Lett., 2002, 80, 2645 CrossRef CAS.
- J. M. Lupton, I. D. W. Samuel, R. Beavington, M. J. Frampton, P. L. Burn and H. Bassler, Phys. Rev. B: Condens. Matter, 2001, 63, 155206 CrossRef.
- R. N. Bera, N. Cumpstey, P. L. Burn and I. D. W. Samuel, Adv. Funct. Mater., 2007, 17, 1149 CrossRef CAS.
|
This journal is © The Royal Society of Chemistry 2011 |
Click here to see how this site uses Cookies. View our privacy policy here.