DOI:
10.1039/C0PY00356E
(Review Article)
Polym. Chem., 2011,
2, 998-1007
Received
31st October 2010
, Accepted 3rd December 2010
First published on 4th January 2011
Abstract
Carbon nanotubes (CNTs) are of increasing interest to scientists because of their unique electronic, chemical and mechanical properties. However, the poor solubility in solvents limits the manipulation of CNTs and hampers their applications in many promising fields. Surface modification of CNTs with polymers is an efficient method to solve this problem. In comparison with linear polymers, dendrimers and hyperbranched polymers are highly branched macromolecules with three-dimensional architecture, and they have good solubility, low melt viscosity, and extremely high density of functional groups at the surface. By surface modification with dendrimers or hyperbranched polymers, the solubility of CNTs could be improved greatly. Moreover, the resultant composites can be endowed with novel properties by further functionalization, which enlarges the applications of CNTs. This review makes emphasis on discussing the methods for functionalization of CNTs with dendrimers or hyperbranched polymers, meanwhile, the unique properties and potential applications of the resultant composites are also summarized in an attempt to facilitate the progress in this interesting area.
 Jiao-Tong Sun | Jiao-Tong Sun was born in 1984 in Jiangsu, China. He received his Bachelor in polymer science and engineering in 2007 from Nanjing University of Science and Technology. He is currently pursuing his PhD at University of Science and Technology of China under the supervision of Professors Chunyan Hong and Caiyuan Pan, and works on synthesis of responsive polymers by controlled radical polymerization. |
 Chun-Yan Hong | Chun-Yan Hong is a professor of University of Science and Technology of China (USTC). She obtained a PhD in chemistry from USTC in 2002. Her research interests include controlled radical polymerization, synthesis of stimuli-responsive polymers and biodegradable polymers, functionalization of nanomaterials, and their applications in drug or gene delivery. |
 Cai-Yuan Pan | Professor Cai-Yuan Pan joined the University of Science and Technology of China as lecturer in 1977, and became full professor in 1989. He is a member of the International Advisory Board of Macromolecular Rapid Communications. His interests include the synthesis and characterization of polymers, especially nonlinear polymers, and the preparation and properties of nanomaterials. |
1 Introduction
During the past two decades, carbon nanotubes (CNTs) have attracted great attention due to their unique structure, high aspect ratio and distinctive electronic, mechanical, optical and chemical properties.1–4 Their promising applications in biosensors, nanomedicine, high-strength composite materials and electronic devices have been widely investigated.5–9 However, low solubility in either water or organic solvents and incident aggregation make them difficult to process, which will undoubtedly hamper their practical applications. Especially, the high hydrophobicity and poor solubility of CNTs in biological medium cause the formation of aggregated particles, leading to heterogenous interaction with cell components, and result in adverse effects on health systems.10,11
In order to overcome this obstacle, small molecules or polymers are employed to modify the CNTs via physical interactions or chemical bonding.4,12–14 Usually, even the degrees of functionalization of CNTs with polymers are not so high, they are sufficient to improve the solubility or dispersibility of CNTs, either single-walled CNTs (SWNTs) or multi-walled CNTs (MWNTs), in solvents or polymer matrixes.15 For the covalent approach, this point appears to be especially important. It is generally admitted that the covalent functionalization on sidewalls of CNTs disrupts the conjugated π-system of the tubes4,16,17 and has an effect on their electronic or optical properties. Thus, grafting polymers onto CNTs based on a limited number of attached functional groups (initiating groups or chemical coupling groups) would reduce the destruction of the CNT structures. The polymer-functionalized CNTs through the noncovalent interactions can also form stable dispersion in solvents and retain the typical electronic characteristics of the nanotubes at the same time, but the relatively weak interactions between CNTs and polymers limit their applications in composites as reinforced fillers.18
Among the polymer materials used for functionalization of CNTs, dendrimers and hyperbranched polymers have drawn more interest because of their interesting architecture and special chemical and physical properties.19–24Dendrimers have unique size, symmetric structure with ideally branching units without any structural defects25,26 while hyperbranched polymers have a less regular structure and broader molar mass distribution. In contrast to the troublesome synthetic procedures of dendrimers, hyperbranched polymers are easily prepared with a single step synthetic process.27Dendrimers and hyperbranched polymers have a highly branched, non-entangled architecture and a large number of terminal groups. They exhibit much lower melt and solution viscosities and higher solubility in comparison with their linear analogues. Hence, dendrimers or hyperbranched polymers-functionalized CNTs even with a low functionalization degree are easier to be solubilized. The lower viscosity of functionalized CNTs in solution or the molten state will favor the manipulation and processing of the nanohybrids. Furthermore, dendrimers and hyperbranched polymers can be used advantageously as functional surface and interfacial materials. The numerous terminal groups facilitate further modification and can be covalently modified with some linear polymers or a broad range of functional groups, for instance, fluorophores, electroactive groups, perfluorinated moieties, and dyes. On the other hand, they can be used to entrap or stabilize various metal, metal oxide and semiconductor nanoparticles. Therefore, dendrimers and hyperbranched polymers are ideal materials for modification of CNTs.28
Excellent reviews15,29 on CNT-polymer composites have been published before, and extended representations of various strategies for fabricating the composites have been given. In this review, the modification of CNTs with dendrimers or hyperbranched polymers is discussed based on the different methods of functionalization as shown in Fig. 1. With the emphasis on recent advances in this area and potential applications of the resultant nanocomposites, some representative and important examples are selected to be covered in detail.
2 Noncovalent modification
In this context, noncovalent CNT modification concerns the physical adsorption of dendrimers or hyperbranched polymers to the surface of the CNTs. Conjugated branched polymers or branched polymers functionalized with conjugated functional groups could wrap the pristine CNTs via π–π-stacking interactions. Some hyperbranched polymers or dendrimers could also be used to modify the pristine CNTs by other physical interactions like physical entanglement or van der Waals interactions, etc. In addition, after being treated in mixed acids, the oxidized CNTs could be functionalized by dendrimers or hyperbranched polymersvia electrostatic adsorption or hydrogen-bonding interactions.
2.1 Modification via π–π-stacking interactions
The derivatives of polycyclic aromatic hydrocarbons such as naphthalene, phenanthrene, and pyrene30–33 have been utilized to functionalize carbon nanotubes noncovalently because they can π-stack to CNTs with high binding energies. To solubilize CNTs at full steam, several groups34–36 functionalized dendritic polymers with the polynuclear aromatic compounds and attached the polymers onto the CNTs surfaces using the functional groups as anchors. Some complex dendrimers like dendritic porphyrin,37phthalocyanines and perylenediimides38 were synthesized and immobilized onto SWNTs through strong π–π interactions, showing versatility on the dissolution of SWNTs. The dendrimer/SWNT electron donor–acceptor hybrids were also characterized by investigating the produced photocurrent after illumination and by the use of spectroscopy, respectively. Compared with pristine SWNTs, these nanohybrids showed unique photoelectrical properties.
Through a strong π–π-stacking interaction with the graphitic sidewalls of CNTs, the linear poly(m-phenylenevinylene-co-2,5-dioctoxy-p-phenylenevinylene) (PmPV)39–41 could wrap themselves around CNTs and solubilize the nanotubes. However, the linear PmPV is reasonably flexible and can wrap themselves around either single nanotubes or nanotube bundles without discriminating them based on their different diameters. In a nano-technological context, single carbon nanotubes are much preferred in comparison with bundles. Thus, by encircling a single nanotube with conjugated π–π interactions, more rigid macromolecules with well-defined cavities are promising to disrupt the van der Waals interactions that cause the aggregation of SWNTs. Star and Stoddart42 prepared the hyperbranched PmPV by Wittig condensation of 1,3,5-tris[(triphenylphosphonio)methyl]benzene tribromide and 2,5-dioctyloxyterephthaldehyde (Scheme 1) and simulated a stilbenoiddendrimer that possesses cavities of defined size by molecular modeling. An all-trans-stilbenedendrimer without any substituents was chosen to simplify the calculations. In the third-generation dendrimer, there were three well-defined pockets with the diameter of about 2 nm, which fitted very well with the diameter (1.4 nm) of (10, 10)-SWNT (Fig. 2A).
 |
| Fig. 2 Molecular modeling of the G-3 of an ideal stilbenoiddendrimer and (10,10)-SWNT (A), AFM images of SWNT/hyperbranched PmPV complex (B) and SWNT/linear PmPV complex (C).40,42 | |
They evaluated whether the hyperbranched PmPV had the capacity to disperse nanotube bundles during the solubilization of SWNTs. A very efficient dispersion of the nanotubes was indicated by the noncontact AFM images of the SWNT/hyperbranched polymer samples, in which a large number of single tubes and small bundles were observed. A typical AFM image is shown in Fig. 2B. The result demonstrates that more single strands of SWNTs are obtained with hyperbranched PmPV, while with the linear PmPV most of SWNTs were solubilized in the form of nanotube bundles (Fig. 2C).40 The key to their success is that the hyperbranched polymer with an appropriate degree of branching provides certain pockets for breaking up nanotube bundles.
2.2 Functionalization via other physical interactions
Recently, Ogoshi et al.43 prepared hyperbranched phenolic polymers (HBPs) by Lewis acid-catalyzed polycondensation of 3,4,5-trimethoxytoluene with 1,3,5-tribromomethyl-2,4,6-trimethoxybenzene, and also prepared linear phenolic polymers (LPs) as control samples for solubilization of SWNTs. The result showed that the hyperbranched polymer had better performance in solubilization of SWNTs than the linear polymer in DMF. Due to the relatively rigid macromolecular structure, the void spaces within the globular structure of HBPs enabled their entanglement with SWNTs (Fig. 3). The homogeneous dispersion of SWNTs was obtained with HBPs in DMF. However, in the case of LPs, no pockets formed and the rigid structures lowered physical interactions between them and SWNTs, hence, solubilization of SWNTs did not occur. The SWNT-HBP nanohybrids composed of SWNTs as conducting wires and HBPs as insulating coatings should have potential applications in the fabrication of nanomachines.
 |
| Fig. 3 Solubilization of SWNTs using linear phenolic polymer and hyperbranched phenolic polymer.43 | |
Ye
et al.
44 reported noncovalent nonspecific functionalization and solubilization of MWNTs with hyperbranched polyethylene (HBPE) in organic solvents. HBPE could effectively solubilize MWNTs at high concentrations in chloroform or tetrahydrofuran. The functionalization resulted from noncovalent adsorption of HBPE on sidewall surfaces of MWNTs due to their attractive interactions. HBPE had a dendrimer-like spherical structure without any functionality. The abundant branch ends of HBPE contributed to strong physical interactions between the hyperbranched polymer and MWNTs, which was confirmed by the blue shift of the absorption peak in the UV-Vis spectra. Adsorption of amine-terminated poly(amidoamine) (PAMAM) dendrimer-encapsulated Ptnanoparticles (Pt-DENs) onto undoped CNT and nitrogen-doped CNT (NCNT) supports for oxygenreduction was reported by Vijayaraghavan and Stevenson.45 The adsorption of Pt-DENs was attributed to the strong van der Waals interaction with edge plane sites of CNTs. The Pt-DEN/NCNT composites exhibited high electrocatalytic activity for the oxygenreduction reaction.
Prato and co-workers46 synthesized Aunanoparticles encapsulated within sixth-generation amine-terminated PAMAM dendrimers, and immobilized the dendrimer-encapsulated Aunanoparticles (Au-DENs) onto the surface of MWNTs via electrostatic adsorption. The hydrophilic or hydrophobic character of MWNTs influenced the aggregation of the attached Au-DENs. The Au-DENs aggregated on the pristine MWNTs surface but could be well-dispersed on the surface of oxidized MWNTs (Scheme 2). Electrostatic interaction was also employed to fix Pt-DENs and glucose oxidase onto MWNTs to fabricate a novel amperometric glucose biosensor based on layer-by-layer adsorption.47 Wang et al. immobilized hyperbranched polyethyleneimine (PEI) on the surface of oxidized MWNTs via electrostatic interactions between the positively charged protonated amines in the polymer and the carboxyl groups on the oxidized MWNTs surface, and then used it as a bio-sorbent for the adsorption of bovine serum albumin.48
 |
| Scheme 2 Schematic representation of attachment of dendrimer-encapsulated Aunanoparticles on the surface of pristine or oxidized MWNTs.46 | |
The shape memory materials based on hyperbranched polyurethane and carboxylated MWNTs were prepared viahydrogen-bonding interaction.49 The shape memory properties of the composites were enhanced with the increase of concentration of MWNTs. In addition, the biocompatibility and biodegradability of the composites made them suitable for some biomedical applications. The CNTs were also packed within the micelles formed by amphiphilic hyperbranched copolymers through noncovalent interactions.50 The hydrophobic polystyrene chains of these polymers wrapped the SWNTs around the sides of the tubes, while the hydrophilic hyperbranched polyester chains interacted with the water to maintain the stabilization.
3 Covalent modification
By establishing strong covalent chemical bonds between CNTs and polymers, polymers are attached onto the surface of CNTs firmly. According to the different ways of building the grafted polymer chains, two main methodologies of “grafting to” and “grafting from” are labeled.15,51
3.1 “Grafting to” approach
In general, the “grafting to” method involves ready-made polymers reacting with the functional groups on surfaces of pristine or pre-treated CNTs.52,53 In most cases, by amidation or esterification reaction with the carboxylic acidgroups or derivated acid chloridegroups on CNTs surface, polymer chains terminated with amino or hydroxyl moieties are attached.54–62 In addition, as an efficient coupling reaction, “click” chemistry is exploited in the approach of grafting dendritic polymers onto CNTs. Although the “grafting to” method has the limitation of a relative low grafting density and demands the reactive groups for polymers, it is proved to be a facile and reliable approach to surface modification of CNTs.
In 2001, Sano et al.63 reported the construction of star-shaped structure in which many SWNTs radiated from a dendrimer center (Fig. 4). Since the acid chloridegroups at the open ends of SWNTs were more reactive than those at the sidewalls, the divergent nanotubes from the core of the amine-terminated tenth-generation PAMAM dendrimer were obtained. These interesting super-structure carbon nanotubes exhibited a peculiar response to electron beams.
Haddleton et al.64 prepared fourth-generation PAMAM dendrons by sequential double Michael additions of methylacrylate to primary amines followed by amidation with excess ethylenediamine, and grafted the PAMAM dendrons to pre-functionalized MWNTs by esterification. The silver nanoparticles deposited exclusively on the surface of MWNTs (Scheme 3), which confirmed the presence of dendrons on the surface of MWNTs. Similar PAMAM-CNT hybrid materials were also used for templating CdS quantum dots57 or stabilizing CdTequantum dots.65 Later, PAMAM dendrimers were anchored on carboxylated carbon nanofibers by amidation reaction to support Pt-Runanoparticles.66 The electrocatalytic activity of the prepared composite electrode for methanol oxidation showed excellent performance, which implied that the resultant composite electrode could be applied in methanolfuelcells. Besides these applications, the fourth-generation amine-terminated PAMAM was grafted onto MWNT-modified glass carbon electrodes for electrochemical determination of nitrite.67
 |
| Scheme 3 Synthesis of PAMAM dendron–MWNT–Ag(0) hybrid materials.64 | |
The CNTs' applications in nanomedicine, especially in drug delivery,68 medical imaging,69 cancer targeting and therapeutics,70 are highlighted in the studies of carbon nanotubes. Recently, Shi et al.71 functionalized amine-terminated G5 PAMAM dendrimers with folic acid (FA) and fluorescein isothiocyanate (FI), and then modified MWNTs with the multifunctional dendrimersvia EDC coupling chemistry. The remaining aminegroups on the dendrimers were acetylated to neutralize the surface charge (Scheme 4). The complex carbon nanotube-based materials provided possibility for cancer cell targeting and imaging due to the covalently attached FA and FI on dendrimers. In vitroflow cytometry and confocal microscopy data showed that the formed composites can specifically target cancer cells that overexpress high-affinity FA receptors. Thereby, these modified CNTs have potential applications in diagnosis and therapeutics.
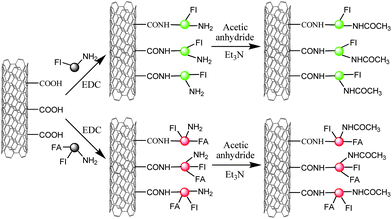 |
| Scheme 4 Schematic representation of reactions to modify MWNTs with dendrimers for cancer cell targeting.71 | |
The CNTs are always thought to be a promising reinforcement material due to their unique mechanical properties. Shi et al.72 reported that the mechanical properties of the CNT-resin composites were greatly enhanced, as indicated by the increases of Young's modulus, fracture toughness, and tensile strength, after hyperbranched polymers were grafted onto the surface of MWNTs via bridging isocyanate.
Take advantage of some efficient covalent chemistry of SWNTs, like nitrene addition,73 aryl diazonium chemistry in conjugation with “click” chemistry,74 and carbodiimide chemistry,75 some dendrons have been successfully attached on the CNT surfaces via “grafting to” approach.
3.2 “Grafting from” approach
The “grafting from” method is generally performed by in situpolymerization of monomers or step-by-step methodology from the attached initiators or reactive groups on the surface of CNTs.76–79 In contrast to “grafting to” approach, it allows high molecular weight polymers to be grafted from the CNT surface with quite high grafting density. Some well-known living polymerizations and polycondensations are utilized to graft a series of dendrimers and hyperbranched polymers from the pre-functionalized CNTs.
3.2.1 Self-condensing vinyl (co)polymerization (SCVP/SCVCP).
Matyjaszewski et al.80 first reported that atom transfer radical polymerization (ATRP) could be applied to the synthesis of hyperbranched polymers by SCVP, and in the polymerizationp-(chloromethyl)styrene (AB*, inimer) acted as both initiator and monomer. The SCVP strategy viaATRP was first employed by our group81 to grow hyperbranched macromolecules on the surfaces of MWNTs (Scheme 5). The ATRPinitiators were fixed on the MWNT surface by reaction of acid chloride-functionalized MWNTs and 2-hydroxyethyl 2′-bromoisobutyrate, and used to initiate the SCVP of 2-((bromobutyryl)oxy)ethyl acrylate (AB*) via the ATRP process. The results demonstrated that hyperbranched polymers were successfully grafted on the nanotubesvia this strategy and the as-prepared MWNT-based conjugates exhibited relatively good dispersibility in organic solvents such as THF and chloroform.
Gao and co-workers82 grafted linear and hyperbranched glycopolymer on MWNTs by the “grafting from” strategy. Via self-condensing vinyl copolymerization (SCVCP) of 3-O-methacryloyl-1,2:5,6-di-O-isopropylidene-D-glucofuranose and 2-(2-bromoisobutyryloxy)ethyl methacrylate (AB*), hyperbranched glycopolymers grew from 2-bromo-2-methylpropionyl-immobilized MWNTs, and the grafting efficiency was lower than that of linear glycopolymer. The kinetics of polymerization and the degree of branching (DB) of the grafted hyperbranched polymers were investigated. When the ratio of monomer to inimer (AB*) increased from 0.5 to 5, the DB of the polymer ranged from 0.49 to 0.21, which was in agreement with the theoretical prediction. After deprotection the multihydroxylhyperbranched polymers were water-soluble and biocompatible, and the resultant polymer-functionalized MWNTs could be applied in bionanotechnology.
3.2.2
Ring-opening polymerization (ROP).
The first example of growing multihydroxylhyperbranched polymers on the surfaces of carbon nanotubes by in situROP was reported by Gao and co-workers.83 The oxidized MWNTs reacted with thionyl chloride and then with an excess of glycol, resulting in hydroxyl-functionalized MWNTs (MWNT-OH). With BF3·OEt2 as catalyst, the MWNT-OH initiated in situ cationic ROP of 3-ethyl-3-(hydroxymethyl)-oxetane, and multihydroxyl hyperbranched polyethers were covalently grafted on the surfaces of MWNTs as illustrated in Scheme 6. TEM images indicated that the MWNTs were enveloped evenly by the hyperbranched polymers. Branched PEI was grafted onto the surface of amine-functionalized MWNTs by cationic ROP of aziridine, and acted as an efficient anchor to immobilize DNA onto the surface of MWNTs. The PEI-g-MWNTs showed good transfection efficiency for DNA delivery.84
Later, Gao et al.85 presented anionic ROP to functionalize CNTs with hyperbranched polyglycerol (HPG), as shown in Scheme 7. To avoid the self-biting side reaction between anions and ester bonds, the initiating groups were introduced on the surface of MWNTs by direct nitrenecycloaddition instead of esterification. With potassium methoxide as catalyst, highly branched polyglycerol was grafted from the nanotubesvia anionic ROP. The functionalized MWNTs exhibited good dispersibility in polar solvents such as water, methanol, DMF, and dimethylsulfoxide (DMSO) due to the grafted polar HPG. The numerous hydroxylgroups of the product reacted with palmitoyl chloride or rhodamine 6B, and the further modification made MWNT-based composites promising for application in drug delivery and bio-imaging. Adeli et al.86 also functionalized MWNTs with biocompatible hyperbranched polyglycerol via anionic ROP using hydroxylgroups on the surface of oxidized nanotubes as initiators. In in vitro cytotoxicity tests and hemolysis assay, no adverse effects were observed on the HT1080cells and red blood cells. Hence, the functionalization of CNTs with hyperbranched polyglycerol decreases in vitro cytotoxicity of carbon nanotubes and makes them promising in nanomedicine.
 |
| Scheme 7 Functionalization of MWNTs with hyperbranched polyglycerol by anionic ROP and modification of the grafted polymer.85 | |
3.2.3
In situ
polycondensation
.
Baek et al.87 grafted hyperbranched poly(ether-ketone)s (HPEKs) from MWNTs by a direct Friedel–Crafts (F–C) acylation reaction (Scheme 8). The polycondensation of trimesic acid (A3) and phenyl ether (B2) was carried out in a mildly acidic medium of optimized phosphoric acid/phosphorus pentoxide (PPA/P2O5). Because of the vast difference in the solubility of two monomers in the reaction medium, gelation was prevented in a self-regulating manner. Through adjusting the feed ratios of A3 and B2 monomers it was possible to alter the end groups of the hyperbranched polymers and vary the polarity of the resulting nanocomposites from highly polar to relatively nonpolar. Due to the globular molecular architecture of hyperbranched polymers, the morphology of the nanocomposites resembled “mushroom-like clusters on MWNT stalks”, as shown in Fig. 5. The resultant nanocomposites could be used as structural additives to polymer matrices. Baek and co-workers also employed this efficient F–C acrylation to prepare HPEK- or hyperbranched poly(phenylene sulfide)-grafted MWNTs via in situpolycondensation of AB2 monomer.88–90 The linear poly(phenylene sulfide) was also grafted from MWNTs with an AB monomer, and the resultant nanocomposite exhibited higher electrical conductivity than the hyperbranched poly(phenylene sulfide)-g-MWNTs, which mostly corresponded to the para-conjugated structure of the former.90
 |
| Scheme 8 Grafting of hyperbranched PEKs onto MWNT from A3 and B2 monomers.87 | |
 |
| Fig. 5
SEM images of as received MWNTs (A) and the prepared HPEK-MWNTs (B). Scale bars are 100 nm.87 | |
The biodegradable hyperbranched poly(citric acid) (HPCA) was grafted onto MWNTs by polycondensation of monohydrate citric acid in the presence of carboxylated MWNTs.91 By elevating reaction temperature and vacuum removing of the water, the esterification was promoted and HPCA was successfully grafted from the functionalized MWNTs. The degree of polymerization of grafted HPCA could be controlled by changing the ratio of MWNTs to citric acid. The grafted HPCA was used for encapsulating silver nanoparticles, and the biocompatibility and antibacterial property of the resultant hybrid make it promising materials for nanomedicine applications.
Gao92 and Xie93–95 synthesized hyperbranched poly(urea-urethane)-functionalized MWNTs (MWNT-HPU) by a one-pot polycondensation of diisocyanate and diethanolamine in the presence of amino- or hydroxyl-functionalized MWNTs, respectively. The novel solution rheology of MWNT-HPU was investigated by steady shear measurements.94 Due to the existence of intra- and intermolecular H-bonds, the rheological behaviors of MWNT-HPU solutions exhibited a strong dependence on temperature and shear rate. Dispersion of MWNT-HPU in polyamide-6 (PA6) were also studied.95 Grafting of HPUs onto the surface of MWNTs improved the compatibility between MWNT-HPU and PA6, and MWNT-HPU was dispersed uniformly in PA6 matrix. Furthermore, the H-bonds between them enhanced their interfacial adhesion. These studies would pave the way to practical applications of carbon nanotubes in composite materials.
Hyperbranched PAMAM-modified MWNTs (MWNT-PAMAM) prepared through a divergent methodology were reported by Wang and co-workers.96 After the acid chloride-terminated MWNTs were changed into amino-terminated MWNTs by reacting with extra diethylene triamine, the mixtures of methyl acrylate and diethylene triamine (molar ratio 1
:
1) were added into the reaction. Hyperbranched PAMAM was in situ grafted from the MWNT surface. Lots of active amino groups of MWNT-PAMAM can be further functionalized. After reacting with the fluorescein isothiocyanate, the modified MWNT-PAMAM showed strong photoluminescent properties.
3.2.4 Step-by-step methodology.
Prato et al.97 grafted PAMAM dendrimers from the SWNTs with the step-by-step method. The amino groups were first anchored on the surface of SWNTs by 1,3-dipolar cycloaddition. Then by repeating the two processes: (1) Michael addition of methyl acrylate to the surface amino groups and (2) amidation of terminal estergroups with ethylenediamine (Scheme 9), they grafted second generation of PAMAM dendrons from the functionalized SWNT surface. The terminal amino groups of the dendrons were further functionalized with derivatized porphyrins. The resulting nanoconjugates were expected to harvest light to give more efficient electron transfers. In addition, the periphery of PAMAM dendrons was derivatized by positively charged tetraalkyl ammonium salts to make the new derivatives efficient vectors for siRNA delivery.98 Chan-Park et al.99 modified SWNTs with dendritic PAMAM in the similar way and prepared anisotropic microsized fibers by reactive spinning using the functionalized SWNTs as filler in thermosetting epoxy. The fibers showed high tensile strength and Young's modulus, which was correlative with the alignment of modified SWNTs. In addition, the PAMAM-modified MWNTs were used for deposition of silver nanoparticles. The resulting nanohybrids showed strong antimicrobial effect against both the representative Gram-negative and Gram-positive bacteria and may be used as effective antimicrobial materials.100 Cui et al.101,102 also grew amine-terminated PAMAM dendrimers on the surface of CNTs with similar method and investigated their application in gene delivery by conjugation with fluorescein isothiocyanate-labeled antisensec-mycoligonucleotides. With generation 5 of the dendrimers, the composites exhibited maximal transfection efficiency and inhibition effects on tumor cells.
 |
| Scheme 9 Schematic graphics of the modification of MWNTs with PAMAM dendrimervia the grafting-from method.97 | |
Recently, You et al.103 grafted hyperbranched PAMAM from the surface of CNTs via a multi-step Michael addition reaction of 1-(2-aminoethyl) piperazine (AEPZ) and N,N′-methylene bisacrylamide (MBA) (Scheme 10). The amido and amine units linked on CNTs could facilitate the assembly of CNTs with other functional polymersviahydrogen bonds. The external ultrasound-stimulus was found to induce the assembly of functionalized MWNTs with linear PAMAM into a smart carbon nanotube gel at room temperature. The sol–gel switching was easily realized by heating and ultrasonicating (Fig. 6). Also, the physically crosslinked network was responsive to some chemical stimuli such as water and acids. The novel multi-responsive carbon nanotube gels supply the possibility of designing and developing ultrasound-driven devices, which will be useful in nanomedicine and sensors.
 |
| Scheme 10 Outline of growing hyperbranched PAMAM from MWNTs.103 | |
 |
| Fig. 6 Photographs and illustration of sol–gel switching through heating and ultrasonicating.103 | |
4 Conclusions
In summary, modification of CNTs with dendrimers or hyperbranched polymers attracts much attention because the branched polymers show great advantages in solubilizing CNTs in comparison with their linear analogues, meanwhile, their globular architecture and lots of terminal groups facilitate further functionalization and enable the resultant nanohybrids to have peculiar properties. Since the solubility and processibility of carbon nanotubes have been improved greatly through functionalization with dendrimers or hyperbranched polymers by both covalent and noncovalent approaches, more research interest has recently been paid to fabricating new nanoconjugates from CNTs and dendrimers or hyperbranched polymers derivatives and pursuing novel characteristics. These novel CNT-based conjugates are potentially applicable in mechanical engineering materials, nanocatalysts, fuelcells, nanomedicine, and sensors, etc. There should be much room for research in this area by chemists, physicists, and biologists. It is expected that this review could demonstrate the development in this area comprehensively and facilitate its further progress.
Acknowledgements
We thank National Natural Science Foundation of China (Grants 20974103 and 21074121) and the Fundamental Research Funds for the Central Universities for financial support.
References
- M. Ouyang, J. L. Huang and C. M. Lieber, Acc. Chem. Res., 2002, 35, 1018–1025 CrossRef CAS.
- J. P. Salvetat, J. M. Bonard, N. H. Thomson, A. J. Kulik, L. Forro, W. Benoit and L. Zuppiroli, Appl. Phys. A: Mater. Sci. Process., 1999, 69, 225–260 CrossRef.
- X. Wan and J. Dong, Phys. Rev. B: Condens. Matter, 1998, 58, 6756–6759 CrossRef CAS.
- D. Tasis, N. Tagmatarchis, A. Bianco and M. Prato, Chem. Rev., 2006, 106, 1105–1136 CrossRef CAS.
- R. Martel, ACS Nano, 2008, 2, 2195–2199 CrossRef CAS.
- J. D. Qiu, W. M. Zhou, J. Guo, R. Wang and R. P. Liang, Anal. Biochem., 2009, 385, 264–269 CrossRef CAS.
- N. W. S. Kam, Z. Liu and H. Dai, J. Am. Chem. Soc., 2005, 127, 12492–12493 CrossRef CAS.
- Y. Wu, J. A. Phillips and H. Liu, ACS Nano, 2008, 2, 2023–2028 CrossRef CAS.
- M. Prato, K. Kostarelos and A. Bianco, Acc. Chem. Res., 2008, 41, 60–68 CrossRef CAS.
- A. D. Maynard, P. A. Baron, M. Foley, A. A. Shvedova, E. R. Kisin and V. Castranova, J. Toxicol. Environ. Health, Part A, 2004, 67, 87–107 Search PubMed.
- L. Ding, J. Stilwell, T. Zhang, O. Elboudwarej, H. Jiang and J. P. Selegue, Nano Lett., 2005, 12, 2448–2464 CrossRef.
- C. Y. Hong and C. Y. Pan, J. Mater. Chem., 2008, 18, 1831–1836 RSC.
- C. Y. Hong, Y. Z. You and C. Y. Pan, Polymer, 2006, 47, 4300–4309 CrossRef CAS.
- Y. Z. You, C. Y. Hong and C. Y. Pan, J. Phys. Chem. C, 2007, 111, 16161–16166 CrossRef CAS.
- Z. Spitalsky, D. Tasis, K. Papagelis and C. Galiotis, Prog. Polym. Sci., 2010, 35, 357–401 CrossRef CAS.
- A. Hirsch, Angew. Chem., Int. Ed., 2002, 41, 1853–1859 CrossRef CAS.
- J. Bahr and J. M. Tour, J. Mater. Chem., 2002, 12, 1952–1958 RSC.
- L. Sun, G. L. Warren, J. Y. O'Reilly, W. N. Everett, S. M. Lee, D. Davis, D. Lagoudas and H. J. Sue, Carbon, 2008, 46, 320–328 CrossRef CAS.
- S. M. Grayson and J. M. J. Frechet, Chem. Rev., 2001, 101, 3819–3868 CrossRef CAS.
- S. Peleshankoa and V. V. Tsukruk, Prog. Polym. Sci., 2008, 33, 523–580 CrossRef CAS.
- D. Wang, Y. Liu, C. Y. Hong and C. Y. Pan, J. Polym. Sci., Part A: Polym. Chem., 2005, 43, 5127–5137 CrossRef CAS.
- C. Y. Hong, Y. Z. You, D. C. Wu, Y. Liu and C. Y. Pan, J. Am. Chem. Soc., 2007, 129, 5354–5355 CrossRef CAS.
- Y. Z. You, C. Y. Hong, C. Y. Pan and P. H. Wang, Adv. Mater., 2004, 16, 1953–1957 CrossRef CAS.
- W. Yang, C. Y. Pan, M. D. Luo and H. B. Zhang, Biomacromolecules, 2010, 11, 1840–1846 CrossRef CAS.
- C. J. Hawker and J. M. J. Frechet, J. Am. Chem. Soc., 1990, 112, 7638–7647 CrossRef CAS.
- O. A. Matthews, A. N. Shipway and J. F. Stoddart, Prog. Polym. Sci., 1998, 23, 1–56 CrossRef.
- C. Gao and D. Yan, Prog. Polym. Sci., 2004, 29, 183–275 CrossRef CAS.
- A. M. Caminade and J. P. Majoralab, Chem. Soc. Rev., 2010, 39, 2034–2047 RSC.
- N. G. Sahooa, S. Ranab, J. W. Chob, L. Li and S. H. Chana, Prog. Polym. Sci., 2010, 35, 837–867 CrossRef.
- F. Wurm, A. M. Hofmann, A. Thomas, C. Dingels and H. Frey, Macromol. Chem. Phys., 2010, 211, 932–939 CAS.
- R. J. Chen, Y. Zhang, D. Wang and H. Dai, J. Am. Chem. Soc., 2001, 123, 3838–3839 CrossRef CAS.
- H. Paloniemi, T. Aaritalo, T. Laiho, H. Liuke, N. Kocharova, K. Haapakka, F. Terzi, R. Seeber and J. Lukkari, J. Phys. Chem. B, 2005, 109, 8634–8642 CrossRef CAS.
- K. Yang, X. Wang, L. Zhu and B. Xing, Environ. Sci. Technol., 2006, 40, 5804–5810 CrossRef CAS.
- L. Valentini, I. Armentano, L. Ricco, J. Alongi, G. Pennelli, A. Mariani, S. Russo and J. M. Kenny, Diamond Relat. Mater., 2006, 15, 95–99 CrossRef CAS.
- L. Valentini, F. Mengoni, I. Armentano, J. M. Kenny, L. Ricco, J. Alongi, M. Trentini, S. Russo and A. Mariani, J. Appl. Phys., 2006, 99, 114305 CrossRef.
- G. J. Bahun and A. Adronov, J. Polym. Sci., Part A: Polym. Chem., 2010, 48, 1016–1028 CrossRef CAS.
- L. Valentini, M. Trentini, F. Mengoni, J. Alongi, I. Armentano, L. Ricco, A. Mariani and J. M. Kenny, Diamond Relat. Mater., 2007, 16, 658–663 CrossRef CAS.
- U. Hahn, S. Engmann, C. Oelsner, C. Ehli, D. M. Guldi and T. Torres, J. Am. Chem. Soc., 2010, 132, 6392–6401 CrossRef CAS.
- S. A. Curran, P. M. Ajayan, W. J. Blau, D. L. Carroll, J. N. Coleman, A. B. Dalton, A. P. Davey, A. Drury, B. McCarthy, S. Maier and A. Strevens, Adv. Mater., 1998, 10, 1091–1093 CrossRef CAS.
- A. Star, J. F. Stoddart, D. Steuerman, M. Diehl, A. Boukai, E. W. Wong, X. Yang, S.-W. Chung, H. Choi and J. R. Heath, Angew. Chem., Int. Ed., 2001, 40, 1721–1725 CrossRef CAS.
- J. N. Coleman, A. B. Dalton, S. Curran, A. Rubio, A. P. Davey, A. Drury, B. McCarthy, B. Lahr, P. M. Ajayan, S. Roth, R. C. Barklie and W. J. Blau, Adv. Mater., 2000, 12, 213–216 CrossRef CAS.
- A. Star and J. F. Stoddart, Macromolecules, 2002, 35, 7516–7520 CrossRef CAS.
- T. Ogoshi, T. Saito, T. Yamagishi and Y. Nakamoto, Carbon, 2009, 47, 117–123 CrossRef CAS.
- L. Xu, Z. Ye, Q. Cui and Z. Gu, Macromol. Chem. Phys., 2009, 210, 2194–2202 CrossRef CAS.
- G. Vijayaraghavan and K. J. Stevenson, Langmuir, 2007, 23, 5279–5282 CrossRef CAS.
- M. A. Herrero, J. Guerra, V. S. Myers, M. V. Gomez, R. M. Crooks and M. Prato, ACS Nano, 2010, 4, 905–912 CrossRef CAS.
- L. Xu, Y. Zhu, L. Tang, X. Yang and C. Li, Electroanalysis, 2007, 19, 717–722 CrossRef CAS.
- M. L. Chen, M. L. Chen, X. W. Chen and J. H. Wang, Macromol. Biosci., 2010, 10, 906–915 CrossRef CAS.
- H. Deka, N. Karak, R. D. Kalita and A. K. Buragohain, Carbon, 2010, 48, 2013–2022 CrossRef CAS.
- W. Zhou, S. Lv and W. Shi, Eur. Polym. J., 2008, 44, 587–601 CrossRef CAS.
- P. Liu, Eur. Polym. J., 2005, 41, 2693–2703 CrossRef CAS.
- Y. Z. You, C. Y. Hong and C. Y. Pan, Adv. Funct. Mater., 2007, 17, 2470–2477 CrossRef CAS.
- Y. Z. You, C. Y. Hong and C. Y. Pan, Macromol. Rapid Commun., 2006, 27, 2001–2006 CrossRef CAS.
- G. Jiang, L. Wang, C. Chen, X. Dong, T. Chen and H. Yu, Mater. Lett., 2005, 59, 2085–2089 CrossRef CAS.
- Q. P. Feng, X. M. Xie, Y. T. Liu, W. Zhao and Y. F. Gao, J. Appl. Polym. Sci., 2007, 106, 2413–2421 CrossRef CAS.
- J. J. Davis, K. S. Coleman, B. R. Azamian, C. B. Bagshaw and M. L. H. Green, Chem.–Eur. J., 2003, 9, 3732–3739 CrossRef CAS.
- S. H. Hwang, C. N. Moorefield, P. Wang, K. U. Jeong, S. Z. D. Cheng, K. K. Kotta and G. R. Newkome, J. Am. Chem. Soc., 2006, 128, 7505–7509 CrossRef CAS.
- X. Wang, H. Liu, Y. Jin and C. Chen, J. Phys. Chem. B, 2006, 110, 10236–10240 CrossRef CAS.
- Y. L. Zeng, Y. F. Huang, J. H. Jiang, X. B. Zhang, C. R. Tang, G. L. Shen and R. Q. Yu, Electrochem. Commun., 2007, 9, 185–190 CrossRef CAS.
- Y. Shen, Q. Xu, H. Gao and N. Zhu, Electrochem. Commun., 2009, 11, 1329–1332 CrossRef CAS.
- J. Zhang, Y. Zheng, P. Yu, S. Mo and R. Wang, Polymer, 2009, 50, 2953–2957 CrossRef CAS.
- N. Zhu, H. Gao, Q. Xu, Y. Lin, L. Su and L. Mao, Biosens. Bioelectron., 2010, 25, 1498–1503 CrossRef CAS.
- M. Sano, A. Kamino and S. Shinkai, Angew. Chem., Int. Ed., 2001, 40, 4661–4663 CrossRef CAS.
- L. Tao, G. Chen, G. Mantovani, S. York and D. M. Haddleton, Chem. Commun., 2006, 4949–4951 RSC.
- Y. Zeng, C. Tang, H. Wang, J. Jiang, M. Tian, G. Shen and R. Yu, Spectrochim. Acta, Part A, 2008, 70, 966–972 CrossRef.
- T. Maiyalagan, J. Solid State Electrochem., 2009, 13, 1561–1566 CrossRef CAS.
- N. Zhu, Q. Xu, S. Li and H. Gao, Electrochem. Commun., 2009, 11, 2308–2311 CrossRef CAS.
- R. Singh, D. Pantarotto, D. McCarthy, O. Chaloin, J. Hoebeke, C. D. Partidos, J. P. Briand, M. Prato, A. Bianco and K. Kostarelos, J. Am. Chem. Soc., 2005, 127, 4388–4396 CrossRef CAS.
- K. Welsher, Z. Liu, D. Daranciang and H. Dai, Nano Lett., 2008, 8, 586–590 CrossRef CAS.
- N. Shao, S. Lu, E. Wickstrom and B. Panchapakesan, Nanotechnology, 2007, 18, 315101 CrossRef.
- X. Shi, S. H. Wang, M. Shen, M. E. Antwerp, X. Chen, C. Li, E. J. Petersen, Q. Huang, W. J. Weber and J. R. Baker, Biomacromolecules, 2009, 10, 1744–1750 CrossRef CAS.
- W. Zhou, J. Xu and W. Shi, Thin Solid Films, 2008, 516, 4076–4082 CrossRef CAS.
- M. Holzinger, J. Abraham, P. Whelan, R. Graupner, L. Ley, F. Hennrich, M. Kappes and A. Hirsch, J. Am. Chem. Soc., 2003, 125, 8566–8580 CrossRef CAS.
- T. Palacin, H. Le Khanh, B. Jousselme, P. Jegou, A. Filoramo, C. Ehli, D. M. Guldi and S. Campidelli, J. Am. Chem. Soc., 2009, 131, 15394–15402 CrossRef CAS.
- A. Garcia, M. A. Herrero, S. Frein, R. Deschenaux, R. Munoz, I. Bustero, F. Toma and M. Prato, Phys. Status Solidi A, 2008, 205, 1402–1407 CrossRef CAS.
- C. Y. Hong, Y. Z. You and C. Y. Pan, Chem. Mater., 2005, 17, 2247–2254 CrossRef CAS.
- C. Y. Hong, Y. Z. You and C. Y. Pan, J. Polym. Sci., Part A: Polym. Chem., 2006, 44, 2419–2427 CrossRef CAS.
- Y. Z. You, C. Y. Hong and C. Y. Pan, Nanotechnology, 2006, 17, 2350–2354 CrossRef CAS.
- L. P. Yang, P. Zou and C. Y. Pan, J. Mater. Chem., 2009, 19, 1843–1849 RSC.
- S. G. Gaynor, S. Edelman and K. Matyjaszewski, Macromolecules, 1996, 29, 1079–1081 CrossRef CAS.
- C. Y. Hong, Y. Z. You, D. C. Wu, Y. Liu and C. Y. Pan, Macromolecules, 2005, 38, 2606–2611 CrossRef CAS.
- C. Gao, S. Muthukrishnan, W. Li, J. Yuan, Y. Xu and A. H. E. Muller, Macromolecules, 2007, 40, 1803–1815 CrossRef CAS.
- Y. Xu, C. Gao, H. Kong, D. Yan, Y. Z. Jin and P. C. P. Watts, Macromolecules, 2004, 37, 8846–8853 CrossRef CAS.
- Y. Liu, D. C. Wu, W. D. Zhang, X. Jiang, C. B. He, T. S. Chung, S. H. Goh and K. W. Leong, Angew. Chem., Int. Ed., 2005, 44, 4782–4785 CrossRef CAS.
- L. Zhou, C. Gao and W. Xu, Macromol. Chem. Phys., 2009, 210, 1011–1018 CrossRef CAS.
- M. Adeli, N. Mirab, M. S. Alavidjeh, Z. Sobhani and F. Atyabi, Polymer, 2009, 50, 3528–3536 CrossRef CAS.
- J. Y. Choi, S. J. Oh, H. J. Lee, D. H. Wang, L. S. Tan and J. B. Baek, Macromolecules, 2007, 40, 4474–4480 CrossRef.
- J. Y. Choi, S. W. Han, W. S. Huh, L. S. Tan and J. B. Baek, Polymer, 2007, 48, 4034–4040 CrossRef CAS.
- I. Y. Jeon, L. S. Tan and J. B. Baek, J. Polym. Sci., Part A: Polym. Chem., 2008, 46, 3471–3481 CrossRef CAS.
- I. Y. Jeon, H. J. Lee, Y. S. Choi, L. S. Tan and J. B. Baek, Macromolecules, 2008, 41, 7423–7432 CrossRef CAS.
- M. Adeli, A. Bahari and H. Hekmatara, Nano, 2008, 3, 1–8 CrossRef.
- C. Gao, Y. Z. Jin, H. Kong, R. L. D. Whitby, S. F. A. Acquah, G. Y. Chen, H. Qian, A. Hartschuh, S. R. P. Silva, S. Henley, P. Fearon, H. W. Kroto and D. R. M. Walton, J. Phys. Chem. B, 2005, 109, 11925–11932 CrossRef CAS.
- Y. K. Yang, X. L. Xie, J. G. Wu, Z. F. Yang, X. T. Wang and Y. W. Mai, Macromol. Rapid Commun., 2006, 27, 1695–1701 CrossRef CAS.
- Y. K. Yang, X. L. Xie, Z. F. Yang, X. T. Wang, W. Cui, J. Y. Yang and Y. W. Mai, Macromolecules, 2007, 40, 5858–5867 CrossRef CAS.
- R. H. Zhang, Y. K. Yang, X. L. Xie and R. K. Y. Li, Composites, Part A, 2010, 41, 670–677 CrossRef.
- L. Cao, W. Yang, J. Yang, C. Wang and S. Fu, Chem. Lett., 2004, 33, 490–491 CrossRef CAS.
- S. Campidelli, C. Sooambar, E. L. Diz, C. Ehli, D. M. Guldi and M. Prato, J. Am. Chem. Soc., 2006, 128, 12544–12552 CrossRef CAS.
- M. A. Herrero, F. M. Toma, K. T. Al-Jamal, K. Kostarelos, A. Bianco, T. D. Ros, F. Bano, L. Casalis, G. Scoles and M. Prato, J. Am. Chem. Soc., 2009, 131, 9843–9848 CrossRef CAS.
- J. Che, W. Yuan, G. Jiang, J. Dai, S. Y. Lim and M. B. Chan-Park, Chem. Mater., 2009, 21, 1471–1479 CrossRef CAS.
- W. Yuan, G. Jiang, J. Che, X. Qi, R. Xu, M. W. Chang, Y. Chen, S. Y. Lim, J. Dai and M. B. Chan-Park, J. Phys. Chem. C, 2008, 112, 18754–18759 CAS.
- B. Pan, D. Cui, F. Gao and R. He, Nanotechnology, 2006, 17, 2483–2489 CrossRef CAS.
- B. Pan, D. Cui, P. Xu, C. Ozkan, F. Gao, M. Ozkan, T. Huang, B. Chu, Q. Li, R. He and G. Hu, Nanotechnology, 2009, 20, 125101 CrossRef.
- Y. Z. You, J. J. Yan, Z. Q. Yu, M. M. Cui, C. Y. Hong and B. J. Qu, J. Mater. Chem., 2009, 19, 7656–7660 RSC.
|
This journal is © The Royal Society of Chemistry 2011 |