Non-aggregated Ga(III)-phthalocyanines in the photodynamic inactivation of planktonic and biofilm cultures of pathogenic microorganisms
Received
2nd November 2009
, Accepted 5th October 2010
First published on 29th October 2010
Abstract
Visible light-absorbing cationic water-soluble gallium(III) phthalocyanines (GaPcs) peripherally substituted with four and eight methylpyridyloxy groups were synthesized and investigated as antimicrobial photodynamic sensitizers. The inserted large gallium ion in the phthalocyanine ligand is axially substituted by one hydroxyl group which prevents aggregation of the complexes in aqueous solution. The cellular uptake and the photodynamic activity for the representative strains of the Gram positive bacteria methicillin-resistant Staphylococcus aureus(MRSA) and Enterococcus faecalis, of the Gram negative bacterium Pseudomonas aeruginosa and of the fungus Candida albicans in planktonic phase were studied. The tetra-methylpyridyloxy substituted GaPc1 showed lower cellular uptake compared to the octa-methylpyridyloxy substituted GaPc2. The photodynamic activity of the GaPcs was studied in comparison to methylene blue (MB) and a photodynamically active Zn(II)-phthalocyanine with the same substitution (ZnPcMe). Photodynamic treatment with 3.0 μM GaPc1 at mild light conditions (50 J cm−2, 60 mW cm−2) resulted in a high photoinactivation of the microorganisms in the planktonic phase nevertheless the dark toxicity of GaPc1 towards MRSA and E. faecalis. GaPcs against fungal biofilm grown on polymethylmethacrylate (PMMC) resin showed a complete inactivation at a higher concentration of GaPc2 (6.0 μM) and of the referent sensitizer ZnPcMe. However, the bacterial biofilms were not susceptible to treatment of GaPcs with only 1–2 log reduction of the biofilm. The bacterial biofilm E. faecalis was effectively inactivated only with MB. The water-soluble octa-methylpyridyloxy substituted GaPc2 has a potential value for photodynamic treatment of C. albicans biofilms formed on denture acrylic resin.
Introduction
Photodynamic therapy (PDT) is an innovative therapeutic modality for treatment of infection diseases caused from pathogenic microorganisms.1 The ability of PDT to inactivate bacteria was first discovered more than a century ago (O. Raab and H. von Tappeiner, 1900).2 The further development of antimicrobial PDT passed slowly through the golden age of antibiotic drugs to the comprehensive study during the last decade.3 This approach for inactivation of pathogens has an emerging role due to the high bacterial resistance to conventional therapy and the extended population of bacteria causing acute infections.4 Presently, PDT is well accepted as a promising therapeutic method for local infections of the oral cavity, especially for those caused by multiresistant pathogens.5
PDT is based on the visible light activation of a photosensitizer (PS) molecule in the presence of molecular oxygen which results in generation of reactive oxygen species (ROSs). The mechanisms of photosensitization involve generation of singlet oxygenvia energy transfer from the triplet excited state of PS to the molecular oxygen (Type II) or viaelectron transfer from the triplet excited state of PS to surrounding molecules (Type I). Known macrocyclic metal complex photosensitizers with a metal ion of closed d or p electron configuration in the core of the ligand exhibit high singlet oxygen quantum yields of ∼0.5.6 Reactions of the generated ROSs with the surrounding pathogenic cells, specifically with cellular components such as unsaturated lipids, peptides or nucleic acids, take place. As a result, oxidative damage of the living cells and cell death occur.
Water-soluble photodynamic sensitizers or photosensitizers which can be bound to a functional water-dispersible carrier system are suitable for applications in PDT.7 Among them are metallophthalocyanines (MPcs), which have the disadvantage of easy aggregation in water solution. This property influences the photophysical characteristics negatively and leads to a lowering of the photodynamic effect. Bulky axial substituents at the metal ion and peripheral substituents at the ligand can reduce the molecular aggregation and can increase the solubility due to the change to electronic structure of the complex.8 Only a few papers report the synthesis and photophysical properties of water-soluble Ga(III)-phthalocyanine derivatives.9,10 A large ion such as gallium(III) enhances the intersystem crossing, which improves the triplet quantum yields of the complex.11 The substituent attached to the gallium(III) can lead to an increase in water solubility and to a reduction of molecular aggregation. In addition, the limitation of the aggregation can be effectively achieved by the uses of the carrier system or other formulations as a vehicle of photodynamic sensitizers.12
Gram-positive bacteria are sensitive to the photodynamic action of photosensitizers independent of their charge and hydrophobicity, whereas Gram-negative bacteria and fungi are resistant to anionic and neutral phthalocyanines.13,14 Gram-negative bacteria were efficiently photoinactivated by several cationic porphyrins, phthalocyanines and their analogues.15,16 The photodynamic effect is strongly influenced by the cellular localization and the binding sites of the photosensitizer, which is affected by the molecular structure, the nature and the number of charges of the sensitizers.17,18 The cationic phthalocyanines exhibit a better adhesion to the cellular membrane due to an enhancement of the electrostatic binding at the outer membrane of Gram-negative bacteria.19,20 Therefore primary damage of the cell wall occurs which promotes drug penetration and intracellular photodynamic actions.21
In vitro studies showed that most bacteria and fungi are susceptible to antibacterial agents when they grow as planktonic (liquid) cultures compared to their biofilm counterparts.22–24 Observations on a variety of natural habitats have established that the most microbes persist attached to biological or artificial surfaces as a structured biofilm.25 Development and characterization of Candida albicans biofilms on PMMC, a material used in construction of dentures, have been well described.26Biofilm formation occurs in three phases, which proceeds through early (0–11 h), intermediate (12–30 h) and maturation (38–72 h) phases with a highly complex procedure. Projection analysis and vertical sectioning of 3-D images revealed that aged C. albicans biofilms have a heterogeneous matrix structure (25–30 μm thick), with thin areas of metabolically active cells. Similar distinct developmental phases of biofilm development were described for the bacterial biofilm formation.27
Biofilms associated with dental practice were reported to be susceptible to PDT with a wide range of photodynamic sensitizers with the phenothiazine, porphyrin and phthalocyanine chemical structures.28 Recently, we reported a promising photodynamic effect on an early stage fungus biofilm treated with a water-soluble tetra-methylpyridyloxy-substituted Zn(II)-phthalocyanine (ZnPcMe).29,30 The present work studied two water-soluble Ga(III) phthalocyanines bearing four or eight positively charged methylpyridyloxy functional groups at the ligand and one axial hydroxyl group at the gallium(III) in the core of the ligand (GaPc1 and GaPc2; Scheme 1a, b). The synthesis of octa-methylpyridyloxy-substituted GaPc2 was not described in the literature.
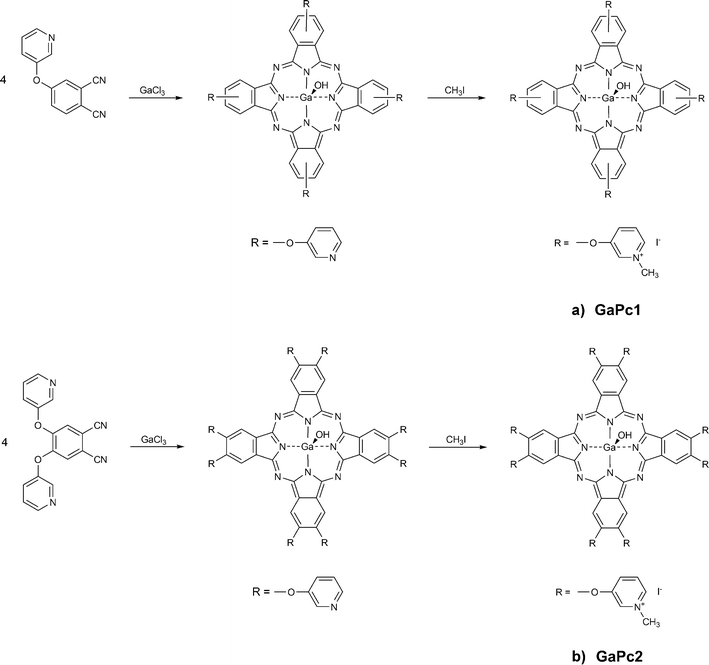 |
| Scheme 1 Synthetic pathways of preparation of tetra- and octa-methylpyridyloxy-substituted Ga(III) phthalocyanines: GaPc1 (a) and GaPc2 (b). | |
The PDT was carried out on four representative strains: two Gram-positive bacteria, methicillin-resistant Staphylococcus aureus and Enterococcus faecalis, one Gram-negative bacterium, Pseudomonas aeruginosa, and a fungus C. albicans. The cellular uptake of GaPcs into pathogenic cells with different cell densities was evaluated. The photodynamic responses on the bacteria and fungus cells as suspensions and as biofilms in an intermediate phase were studied. Biofilms were formed on PMMC resin and were characterized for their thickness and the penetration depth of sensitizers. The GaPcs were studied in comparison to known photodynamic sensitizers (ZnPcMe and MB).
Experimental
Chemicals
Chemicals for synthesis and spectroscopy were purchased from Sigma–Aldrich and Fluka. Spectrophotometric experiments were carried out in dimethylsulfoxide (DMSO), dimethylformamide (DMF) and tetrahydrofuran (THF) of spectroscopic grade, and in doubly distilled water (Labsystems, Ltd.). The syntheses of the GaPcs were carried out in quinoline, which was distilled twice over CaH2. The other solvents such as dichloromethane (DCM), acetone (Ac), ethanol and pyridine were of analytical grade and dried or distilled. Chemicals for preparation of phthalocyanines such as 4-hydroxypyridine, 4-nitrophthalonitrile, potassium carbonate, methyl iodide and GaCl3 were used as supplied. Both precursor chemicals 4-(3-pyridyloxy)phthalonitrile and 4,5-bis(3-pyridyloxy)phthalonitrile were synthesized and purified as described previously.31 All reactions were carried out under a dry nitrogen or argon atmosphere. Stock solutions of GaPc1 and GaPc2 in DMSO (1–3 mM) were freshly prepared and stored in the dark. All dilutions were performed in sterile 0.01 M phosphate-buffered saline (PBS) to the final concentrations needed (1–10 μM).
Apparatus
Electronic absorption spectra were recorded on a spectrophotometer Shimadzu UV–Vis 3000 (Japan) in the spectral range of 300–800 nm at room temperatures (RT). The steady-state fluorescence measurements were carried out on Fluorolog-3 fluorimeter (Horiba Jobin Yvon) using 1 cm pathlength cuvette at RT. FT-IR spectra (KBr pellets) were obtained using a BIORAD SPC-3200 FTS7 spectrometer. 1H NMR (600 MHz) spectra were acquired on an AVANCE AV600 II+ NMR spectrometer. Mass spectra were measured using a Bruker Esquire LC for ESI spectra.
Syntheses
Hydroxygallium(III) 2,9,16,23-tetrakis-(3-pyridyloxy)-phthalocyanine (mixture of regioisomers).
The reaction mixture of 4-(3-pyridyloxy)phthalonitrile (1.5 g, 6.78 mmol) and anhydrous gallium(III) chloride (0.600 g, 3.4 mmol) in quinoline (5 mL, freshly distilled over CaH2) was flushed with nitrogen and heated at 180 °C for 6 h. A green precipitate was obtained. After cooling 30 mL of a mixture ammonia hydroxide (25%) and pyridine (1
:
1, v/v) was added, and the mixture was stirred overnight at 20 °C. After precipitation with water the reaction product was collected by filtration and washed with water, DMF : water (1
:
3), water and then Ac. The product was purified by preparative column chromatography on silica gel starting with tetrahydrofuran (THF) and then with THF : Ac (1
:
1) as elution system. The green solid was dried under vacuum over phosphorous pentoxide. Yield: 0.86 g (53%). UV-Vis (DMSO) λmax/nm (ε/105): 351 (0.32), 619 (0.23), 686 (1.38). IR [(KBr) νmax/cm−1]: 3402 (Ar–CH), 2924 (CH), 1611 (C–O–C), 1573 (C
C), 1504, 1472, 1422, 1340, 1234, 1083 (C–O–C), 1052, 949, 806, 748. ESI-MS (positive ion mode) m/z: 953 [M+], 1923 [2M − OH−]+.
Hydroxygallium(III) 2,9,16,23-tetrakis-[3-(N-methyl)pyridyloxy]-phthalocyanine tetraiodide (GaPc1) (mixture of regioisomers).
Hydroxygallium 2,9,16,23-tetrakis-(3-pyridyloxy)phthalocyanine (0.100 g, 0.1 mmol) was dissolved in dry DMF (5 mL) and an excess of methyl iodide (5 mL, 80 mmol) was added. The solution was stirred at 40 °C under nitrogen for 48 h. Then the solvent was evaporated and the reaction product was precipitated with dichloromethane (DCM), filtrated and washed with DCM, ethanol and Ac. The green solid was dried under vacuum over phosphorous pentoxide. Yield: 0.11 g (72%). UV-Vis (DMSO) λmax/nm (ε/105): 351 (0.47), 615 (0.32), 681 (1.78). IR [(KBr) νmax/cm−1]: 3041 (Ar–CH), 1581 (C
C), 1498, 1402, 1274 (C–O–C), 1084, 1051, 943, 747. 1H NMR (600 MHz, DMSO-d6) δ (ppm): 9.80–9.83 (m, 2H), 9.75–9.78 (m, 2H), 9.47–9.52 (m, 8H), 9.02–9.05 (m, 4H), 8.72–8.75 (m, 2H), 8.68–8.71 (m, 2H), 8.39–8.45 (m, 4H), 8.33–8.38 (m, 4H), 4.52 (s, 6H), 4.51 (s, 6H). ESI-MS (positive ion mode) m/z: 257 [M − 4I−]4+, 285 [M − OH− − 3I−]4+, 423 [M − OH− − 2I−]3+, 697 [M − OH− − I−]2+, 1521 [M − OH−]+. Fluorescence, λexc/nm: 630; λmax/nm: 696 (DMSO), 692 (H2O).
Hydroxygallium(III) 2,3,9,10,16,17,23,24-octakis-(3-pyridyloxy)-phthalocyanine (mixture of regioisomers).
The mixture of 4,5-bis(3-pyridyloxy)phthalonitrile (1.5 g, 4.77 mmol), anhydrous gallium chloride (0.450 g, 2.56 mmol) and quinoline (5 mL, freshly distilled over CaH2) was flushed with nitrogen and stirred at 180 °C for 6 h. Then a mixture of NH4OH (25%) and pyridine (1
:
1) was added and the mixture was stirred over night. The green product was isolated and purified as described for tetra-GaPc. Yield: 0.780 g (59%). UV-Vis (DMSO) λmax/nm (ε/105): 356 (0.79), 620 (0.36), 678 (1.26). IR [(KBr) νmax/cm−1]: 3401 (Ar–CH), 1612 (C–O–C), 1574 (C
C), 1475, 1457, 1409, 1281, 1213, 1088 (C–O–C), 1031, 893, 801, 748, 704. ESI-MS (positive ion mode) m/z: 1325 [M+], 1532 [2M − OH−]+.
Hydroxygallium(III) 2,3,9,10,16,17,23,24-octakis-[3-(N-methyl)pyridyloxy]-phthalocyanine octaiodide (GaPc2) (mixture of regioisomers).
The methylated complex was synthesized and purified following the procedure for preparation of GaPc1. Briefly, hydroxygallium(III) 2,3,9,10,16,17,23,24-octakis-(3-pyridyloxy)-phthalocyanine (0.110 g, 0.083 mmol), methyl iodide (5 mL, 80 mmol) were mixed in dry DMF (5 mL) and stirred at 40 °C under nitrogen for 48 h. The green product was obtained after precipitation from DCM and further it was washed with DCM, ethanol and then Ac. The product was dried under vacuum over phosphorous pentoxide. Yield: 0.230 g (96%). UV-Vis (DMSO) λmax/nm (ε/105): 353 (0.83), 615 (0.42), 680 (2.04). IR [(KBr) νmax/cm−1]: 3400 (Ar–CH), 2994 (CH), 1654 (C–O–C), 1585 (C
C), 1501, 1452, 1412, 1347, 1286, 1266, 1174 (C–O–C), 1091, 1041, 957, 884, 749, 712. 1H NMR (600 MHz, DMSO-d6) δ (ppm): 9.88 (s, 8H), 9.49 (s, 8H), 8.99 (d, J = 6.2 Hz, 8H), 8.81 (dd, J = 8.8, J = 1.8 Hz, 8H), 8.32 (dd, J = 8.8, J = 6.00 Hz, 8H), 4.47 (s, 24H). ESI-MS (positive ion mode) m/z: 2382 [M+]. Fluorescence λexc/nm: 630; λmax/nm: 691 (DMSO), 687 (H2O).
Fluorescence study
The fluorescence emission spectra were measured for excitation wavelength 630 nm in the spectral range of 640–850 nm. The Rayleigh scattering of the excitation light (630 nm) was observed in all used solvents (DMSO, THF and H2O). The fluorescence quantum yields (ΦF) were determined for DMSO and H2O on the basis of absorption and fluorescence spectra according to the comparative method.32Eqn (1) was employed for the calculations: | 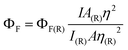 | (1) |
where ΦF(R) is the fluorescence yield of the reference standard (ZnPc, ΦF(R) = 0.18 in DMSO), I and I(R) are the integrated fluorescence of the sample (GaPc1 or GaPc2) and the reference, A and A(R) are the absorbance of the sample and the reference, and η and η(R) are the refractive indices of the solvents employed in calculating fluorescence quantum yields. The absorbance of the sample and the reference standard at the excitation wavelength was kept under 0.05.
Cell cultures
Calf trachea cell line, MDBK cell line, Vero cell line, Madin–Darby Canine Kidney Cells and primary tissue culture of chick embryo fibroblast were used for testing the studied GaPcs for dark toxicity. The cells were cultured at 37 °C in a humidified sterile atmosphere of 95% air and 5% CO2. DMEM (Dulbecco Modified Eagles's Medium, Gibco BRL, USA) supplemented with fetal calf serum (5% v/v) and 10 mM HEPES was used. The cell cultures were incubated at 37 °C and 5% CO2 environment.
Dark toxicity
The toxicity of GaPcs on the cell viability in the absence of light was determined on monolayer cell cultures by the MTT colorimetric assay. Cells were seeded in 96 well plates and cultured to 80–85% confluence. Further incubation in the dark (37 °C) with 0.2 to 10 μM GaPcs for three time intervals (15 min, 24 h and 48 h) was performed. PBS was used for washing and 200 μL DMEM with 5 mg MTT mL−1 was added to each well. Cell cultures were incubated for 4 h at 37 °C and 5% CO2, then medium was discarded, the plates were dried and 0.1 mL of cold isopropanol was added. Plates were mildly shaken for 10 min and the absorbance was read on spectrophotometer. The 50% cytotoxic concentration (CC50) was calculated in comparison to the control by regression analysis using Origin 6.1 programme. The experiments were carried out in triplicate and the values were statistically validated.
Pathogens
The model strains used were as follows: the Gram-positive Staphylococcus aureus 1337, methicillin-resistant strain (MRSA), and Enterococcus faecalis, from the Collection of the Institute of Microbiology, Bulgarian Academy of Sciences, Sofia; the Gram-negative Pseudomonas aeruginosa 1390, and the fungus Candida albicans 74 from the National Bank for Industrial Microorganisms and Cell Cultures (NBIMCC) Bulgaria. Brain heart infusion broth (Difco, BD Diagnostic Systems, and Sparks, MD) was used for S. aureus, E. faecalis and P. aeruginosa. YM medium (Difco) was used for C. albicans. All strains were grown aerobically overnight at 37 °C. Cells were harvested by centrifugation and were suspended in sterile PBS. Absorbance of cell suspension was measured to be 0.490 at 600 nm using spectrophotometer (Unico 2100UV, Canada), which corresponded to 109 CFU mL−1. Prior each experiment, the cells were diluted to the needed density (108–105 CFU mL−1).
Photosensitizer uptake
Cellular suspensions with densities of 109, 108, 107, 106 and 105 CFU mL−1 were incubated with GaPcs (1.0 μM) for 15 min at 25 °C by gentle stirring in the dark. The supernatants were removed and stored for fluorescent measurements. The experimental set-up for evaluation of uptake included a red LED 635 nm as excitation source and a spectrometer for emission registration and was described in detail previously.33 After incubation, the cells were washed with PBS in triplicate. The extraction was carried out in THF : aqueous 2% SDS (1
:
9) for 30 min by mild stirring in the dark. Then the samples were centrifuged, and the collected extracts were examined by fluorescence analysis. The results are presented as number of sensitizer molecules per cell by processing the obtained values of fluorescence intensities and referring to the calibration curves taken for pure compounds in the extraction solvent mixture.
Biofilm assay
Biofilm assay was performed on PMMC acrylic discs, which were designed with a diameter of 10 mm and a thickness of 1.5 mm. The acrylic discs were placed in commercial pre-sterilized, polystyrene flat bottomed 12 well cell culture test plates (Switzerland). A standard bacterial suspension (1 mL, 107 CFU mL−1) prepared after serial dilutions was applied onto the surface of the discs placed in each well of the plate followed by incubation for 1.5 h at 37 °C to promote cellular adherence to surface of the discs. The blank control wells with the discs at same conditions but without bacterial cells were inoculated. After the initial adhesion phase, the cell suspensions were aspirated and the discs were gently washed with PBS to remove loosely adherent cells. In the biofilm phase formation of C. albicans an addition of 4 mL Tryptic soy broth (Difco Laboratories, MD, USA) was placed in each well. The plates were covered and incubated for 18 h at 37 °C to form the microbial biofilm.
The biofilms of bacteria or fungal cells were developed for 18 h incubation at 37 °C by using coverslips covered with PMMC resin. The biofilms were observed by confocal scanning laser microscope from Leica Microsystems (CLSM, Leica TCS SPE). The images were processed via the Leica LAS AF software provided with the CLSM. The oil immersion with 63× objective (NA = 1.23) was used. The bacterial biofilms were characterized with a BacLight Live-Dead bacterial viability kit, L13152 (Molecular Probes, Europe BV). The kit contains two fluorescence stains: SYTO9 and propidium iodide. The preparation and application of the dyes were according to the product information list. The viable cells fluoresce green from the SYTO9 stain (exc. 488 nm, em. 525 nm). The dead cells fluoresce red from the propidium iodide (exc. 520 nm, em. 620 nm). Images of a 0.150 μm slices were taken by scanning the biofilms of bacteria species (MRSA and E. faecalis). The biofilm of C. albicans was evaluated by the fluorescence of fungus cells (exc. 488 nm, em. 500–580 nm) and by the transmission of 635 nm light. Both photosensitizers were evaluated for their penetration depth into microbial biofilms. The biofilms of C. albicans were washed in PBS and covered with a coverslip. The green autofluorescence of the cells into the formed biofilms were detected by excitation at 488 nm and emission between 500–580 nm. The fluorescence of GaPcs inside the biofilms was imaged with excitation by a 635 nm laser and emission between 650–740 nm. The whole biofilm was scanned in slices of 0.150 μm to picture the red fluorescence of GaPcs. The transmission channel of the 635 nm laser radiation was also used to determine the exact thickness of the biofilm in comparison to the sensitizer penetration depth.
In vitro photodynamic study
Samples of 1 mL bacterial suspensions were incubated for 15 min with GaPcs from stock solution in DMSO (<0.5% DMSO) to final concentration of 3.0 μM GaPcs. The incubation was carried out in the dark at room temperature and with a magnetic stirrer. Bacterial suspensions with cell densities between 106–107 CFU mL−1 were prepared in PBS before measurement. After the incubation time, the aliquot (200 μL) from the suspension was placed in a standard palette where the irradiation was performed.
The biofilm of bacterial or fungal cells were developed for 18 h incubation at 37 °C by using PMMC resin discs. The samples were washed with PBS and were incubated with 6.0 μM GaPcs for 1.5 h. The samples were washed again after incubation and were positioned for irradiation with 635 nm. Two diode lasers for two identical samples were used. The fluence rates were controlled with photometer (Spectra Physics, USA). The fluence of 60 mW cm−2 and a light dose of 50 J cm−2 were applied. Samples of three control groups of microbial cells were collected: (1) without photosensitizer, but illuminated, (2) with photosensitizer, but no light (dark toxicity), and (3) only bacterial suspension (no photosensitizer, no light). After irradiation, cells (0.1 mL) were taken off and serially diluted (10-fold) with PBS. The same action was repeated for the control samples. Aliquots (0.1 mL) were spread over Trypticase® Soy agar. The number of colonies (CFU) on each plate was counted following 48 h incubation at 25 °C.
Statistics
The uptake and survival experiments were carried out in triplicate and the data are presented as a mean ± standard deviation (SD). The difference between two means was compared by two-tailed unpaired Student's test. Values of P < 0.05 were considered as significant.
Results and discussion
Synthesis
The synthetic pathways of the tetra- and octa-substituted gallium(III) phthalocyanine complexes with methylpyridyloxy groups are presented in Schemes 1a, b. The general procedure of the synthesis of methylpyridyloxy-substituted Zn(II) phthalocyanines is well documented.31 The preparation includes the synthesis of substituted phthalonitriles or 1,3-diimino-1H-isoindolines followed by the reaction of cyclotetramerization to phthalocyanines and finally their alkylation to cationic complexes. Two different synthetic routes are known and followed in the present study. The preparation of 4-pyridyloxyphthalonitrile from 4-hydroxypyridine was carried out by following the known reaction scheme.31
The tetrapyridyloxy-substituted Ga(III)-phthalocyanine was obtained by the cyclo-tetramerization of 4-pyridyloxy-substituted phthalonitriles in the presence of anhydrous GaCl3 (Scheme 1a). The reaction was carried out at high temperature (180 °C), because gallium is a large metal ion and for its coordination a high energy is required. The crude products were purified first by treatment in a Soxhlet extractor with different solvents and then by preparative flash chromatography on silica gel. The tetra-pyridyloxy-substituted Ga(III)-phthalocyanine was employed for the alkylation with an excess of methyl iodide in DMF. The water soluble GaPc1 was obtained in good yield of 72%.
The preparation of the octa-methylpyridyloxy-substituted Ga(III)-phthalocyanine (GaPc2) started from the corresponding bis-substituted phthalonitrile following the above-mentioned reaction conditions (Scheme 1b). The macrocycle was obtained by treatment of the phthalonitrile derivative with anhydrous GaCl3 in freshly distilled quinoline at high temperature (180 °C). The crude product was at first treated with different solvents and then purified by preparative chromatography on silica gel. The next step includes an alkylation procedure. The reaction was carried out with an excess of methyl iodide in DMF, and the water soluble GaPc2 was obtained in high yield of 96%.
The prepared GaPc1 and GaPc2 exhibit good solubility in organic solvents such as DMF, THF, and polar biologically-compatible solvents such as DMSO and water.
The synthesized complexes GaPc1 and GaPc2 were characterized by means of several spectroscopic analytic techniques (UV-Vis, IR, 1H-NMR and ESI MS spectra, and TLC). The analyses are consistent with the predicted structures as shown in the experimental section.
UV-Vis spectra of GaPc1 and GaPc2 were recorded in DMSO and H2O (Fig. 1). The spectra are typical for phthalocyanine dyes with the characteristic Q band absorption peak in the far red region. GaPc1 shows a maximum at 683 nm in DMSO and at 681 nm in H2O. GaPc2 has the Q band at 680 nm in DMSO and at 678 nm in H2O. In addition the B band was observed between 350 and 360 nm with half of the intensity of absorption of the Q band. In order to see the aggregation behavior by following Beer's law the spectra were recorded in the concentration range between 0.5 × 10−6–10−5 mol L−1.
The aggregation behavior of metal phthalocyanines depends on the concentration, on the metal ion into ligand, on the kind of substituent and on the polarity of solvent, and temperature.32 The obtained absorption spectra of GaPc1 and GaPc2 in water showed sharp and single Q bands with molar extinction coefficients (>105 M−1 cm−1) that are typical for the monomer state of phthalocyanines. It may be a monomer in water due to the large Ga(III) in the core of the ligand.9 The hydroxyl group at the axial position of Ga(III) can also contribute to the monomeric state.
The fluorescence emission spectra of GaPc1 and GaPc2 in THF, DMSO and water were recorded (Fig. 2). The fluorescence emission maxima are red shifted to 696 nm for GaPc1 and 691 nm for GaPc2 as compared to the absorption maxima (683 nm and 681 nm) in DMSO. The fluorescence maxima in water are 692 nm for GaPc1 and 687 nm for GaPc2. The fluorescence maxima in THF are 687 nm for GaPc1 and 686 nm for GaPc2. The fluorescence spectra also suggested that both GaPcs are monomeric molecules with no loss of symmetry due to the large gallium atom.
The fluorescence quantum yields (ΦF) of the studied GaPcs are typical for metal phthalocyanine complexes.32 The tetra-substituted GaPc1 shows higher fluorescence quantum yields (0.25 in DMSO and 0.12 in water) than the octa-substituted GaPc2 (0.13 in DMSO and 0.15 in water). As seen, GaPc1 has a value of ΦF in DMSO (0.25) twice as high as that in water (0.12). This can be explained by the observation that in solvents with polarity larger than DMSO such as water, the fluorescence quantum yield decreases due to the strong intramolecular charge transfer.34 The observation that the ΦF value of GaPc1 (0.25) is higher than that of GaPc2 (0.13) in DMSO suggests a quenching of the excited singlet state by the peripheral substitution of GaPc2. On the other hand, the ΦF values of GaPc1 and GaPc2 in water are comparable without any influence of the number of substituents. It was evaluated that the electronic configurations of the ground and excited states of GaPcs were not affected by the excitation wavelengths (380 nm, 630 nm and 660 nm).
Dark toxicity
Survival of the five cell cultures were evaluated after incubation with GaPc1 and GaPc2 under dark conditions. A concentration of 6 μM and incubation times of 2 h were found as optimal conditions to test dark toxicity. It was shown that up to 10 μM GaPc1 and GaPc2 were not toxic for the used five cell lines. The viability was more than 75% for each tested cell line.
Uptake into pathogens
The uptake of GaPcs after incubation of bacterial and fungal cells with different cell densities was studied. The chemical extraction procedure was applied. The measurements of the fluorescence emission spectra of the samples containing GaPcs were carried out (Fig. 3). The uptake capacities towards GaPcs (1 μM) of the four studied microbial species were estimated by the fluorescence spectra recorded for the cell suspensions and for the followed supernatants: (1) in PBS after incubation, (2) in PBS after cell wash (three times), and (3) after extraction with a mixture of 2% SDS : THF (9
:
1). The spectra were recorded in the spectral range 650–800 nm at excitation wavelength of 635 nm by using the set-up for fluorescence measurements which was described in our previous work.33 The bounded molecules of GaPcs are calculated for one bacterium or one fungus cell. The number of positive charges was observed to influence the uptake capacity of GaPcs. The uptake of GaPc1 was about one and a half times lower compared to GaPc2, which suggested a better membrane penetration due to eight positive charges of GaPc2 compared to only four positive charges of GaPc1 (Fig. 4). The uptake of GaPcs decreased with increasing of the cell densities between 105–109 CFU mL−1. The accumulation capacity of GaPcs shows a significant decrease independently of the treated microbial strains (Fig. 4).
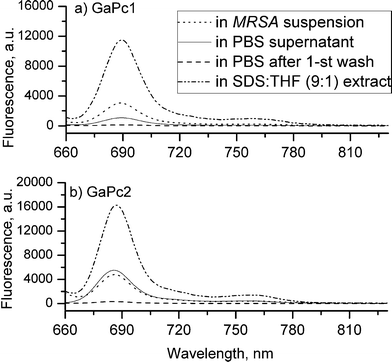 |
| Fig. 3
Fluorescence spectra of samples of MRSA cells incubated for 15 min with GaPc1 (a) and GaPc2 (b), both with concentration of 1.0 μM. The excitation light from a LED 635 nm was applied. | |
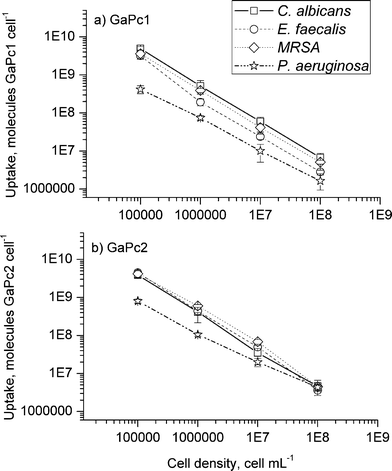 |
| Fig. 4 Uptake of GaPc1 (a) and GaPc2 (b) with concentration of 1.0 μM in dependence on the cell density. The pathogenic bacteria (MRSA, E. faecalis and P. aeruginosa) and fungus (C. albicans) are tested. The data are presented as means ± SD (n = 5). | |
These data follow our previous findings with zinc phthalocyanines with different hydrophobicity and charges.18,19 The binding process occurs via an electrostatic mechanism of interactions and not a covalent binding analogous to our recent results.20 Cationic dyes are more easily taken up in the cells due to the nature of the membrane bilayers. In addition, a higher number of positive charges on the sensitizers results in better accumulation and in drug–cell adhesion, which is essential for the resistant Gram-negative bacterial strains. The phenomenon of an inverse dependence of the number of dye molecule on the cell density was first reported by Demidova and Hamblin for photodynamic sensitizers and bacteria cells.35
The present study contributes to the further understanding of the structure–function relationship between water-soluble and non-aggregated GaPcs as photodynamic sensitizers towards pathogenic cells. Both GaPcs were selected as perspective drugs because of the high accumulation in pathogenic cells from the group of the Gram-positive and the Gram-negative bacteria as well as for the fungus. The uptake results with GaPcs also confirmed that cationic complexes are more tolerable to biological membranes than anionic and non-charged phthalocyanine analogues. Comparison of GaPcs to ZnPcMe shows a negligible difference in favors to GaPc1 for bacterium cells (MRSA).30 This suggests that the coordinated metal ion does not seem to affect significantly the overall charge distribution of MPcs molecules with methylpyridyloxy substituents and in addition the uptake behavior.
Photodynamic study on planktonic cells
The photodynamic antimicrobial activity of tetra- and octa-methylpyridyloxy-substituted Ga(III)-phthalocyanines (GaPc1 and GaPc2) was investigated against model bacterial and fungal strains. Both complexes were studied in vitro on bacterial suspensions with a cell density between 106–107 CFU mL−1 (Fig. 5). The effect was compared to the dark toxicity of 3.0 μM GaPcs and mild irradiation parameters (60 mW cm−2 for 15 min). It can be seen that the dark toxicity of GaPc1 was comparable to the phototoxicity. Only at lower GaPc1 concentration (1.5 μM) a difference of 1 log between samples with irradiation and without light (dark control) was observed. Complete photoinactivation of the Gram-positive bacteria (MRSA and E. faecalis) was observed for 3.0 μM GaPc1 (Fig. 5a). The effect was lower (2 log) for the Gram-negative P. aeruginosa. The octa-substituted GaPc2 showed high photodynamic inactivation towards the studied pathogens, with the exception of MRSA (Fig. 5b).
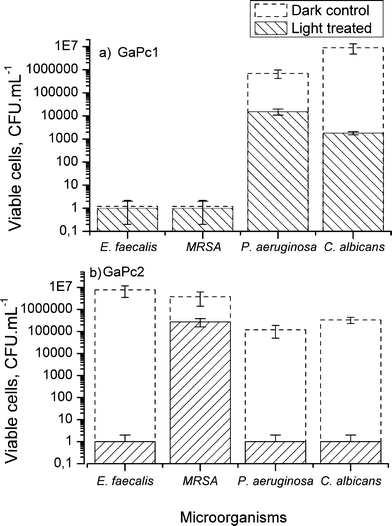 |
| Fig. 5
PDT effect on planktonic cultures of bacteria (MRSA, E. faecalis, P. aeruginosa) and fungus (C. albicans). The data represent the survived cells after treatment with GaPc1 (a) and GaPc2 (b) with concentration of 3 μM and irradiation with a LED 635 nm (60 mW cm−2, 50 J cm−2) and without light (dark control). The data are presented as means ± SD (n = 5). | |
Comparing GaPc1 with ZnPcMe, both bearing the same substituents, inactivation with ZnPcMe resulted in complete cell death for most of the treated pathogenic species.18,19 Replacement of Zn(II) by Ga(III) in the core of the ligand results in an increase in the generated singlet oxygen.29 The singlet oxygen and other ROSs formed under irradiation can directly affect the cellular membrane, as observed for Gram-positive and Gram-negative bacteria.36 On the other hand, sufficient uptake of a photosensitizer into microbial cells facilitates the singlet oxygen action to the membranes due to its diffusion occurs within the membrane constituents.37 The present study suggests that the higher uptake of GaPc2vs.GaPc1 affects slightly the photodynamic response for the selected bacteria and fungus as planktonic cultures (Fig. 4, 5).
Microbial biofilms
The biofilms were analyzed for their thicknesses and the penetration depth of GaPc1 and GaPc2 by a confocal scanning laser microscope (CSLM). Biofilms of bacteria strains MRSA and E. faecalis were analyzed with the stains of the Live-Dead kit and showed thickness between 7–11 μm. The fungus biofilms were measured by their autofluorescence and by light transmission. The selected C. albicans biofilms were with thicknesses between 17–23 μm. Fig. 6 presents the scans of 0.150 μm slices for detection of biofilm of fungus cells by autofluorescence (exc. 488 nm; em. 500–580 nm) and for GaPcs by fluorescence emission (exc. 635 nm; em. 650–740 nm). The microbial biofilms were also characterized by the transmission mode of the excitation light of 635 nm laser (Fig. 7). The images showed that GaPc2 was preferably attached to the cellular wall and also to some extent inside the fungus cells (Fig. 6b, 7b). In contrast, GaPc1 accumulates on the cellular wall (Fig. 6a, 7a). The difference in the intensity of the fluorescence signals of GaPc1 and GaPc2 in the cells was observed (Fig. 6 and 7). The assessments of the scans made by three modes showed that the water-soluble and cationic GaPcs can penetrate into biomass of the selected bacteria and fungus biofilms up to 75% of the total biofilm thickness.
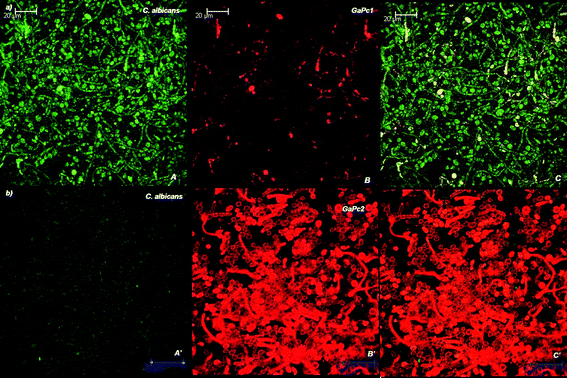 |
| Fig. 6 Confocal images of slices of C. albicans biofilm incubated with 6 μM GaPcs. The biofilm was analyzed by CLSM as described in the Experimental section. The autofluorescence of the cells (exc: 488 nm), panel A; the fluorescence of GaPc1 (exc: 635 nm), panel B; and the overlay of two panels (A and B), panel C (a). The same images for GaPc2 (panels A′, B′ and C′) are presented in (b). Magnification: 63×. | |
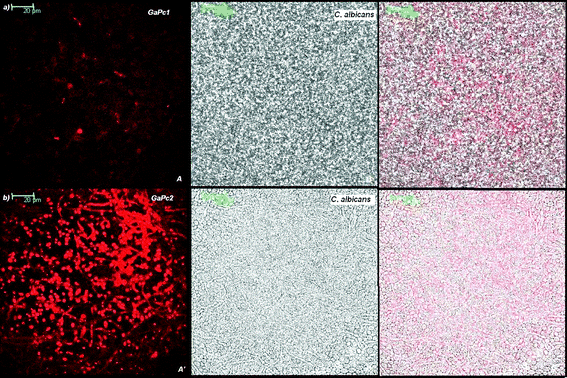 |
| Fig. 7 Confocal images of slices of C. albicans biofilm incubated with 6 μM GaPcs. The fluorescence of GaPc1 (exc: 635 nm), panel A; the image of the cells visualized by transmission of 635 nm light, panel B; and the overlay of two panels, panel C (a). The fluorescence of GaPc2 into biofilm, panel A′, and only cells, panel B′, and the overlay of two panels, C′ (b). Magnification: 63×. | |
Photodynamic study on biofilms
The photodynamic effect of the water-soluble GaPc1 and GaPc2 for inactivation of (18 h) biofilms of pathogenic bacteria MRSA and E. faecalis and a fungus C. albicans were studied in comparison to MB and ZnPcMe (Fig. 8). Complete photodynamic inactivation of C. albicans biofilms with GaPc2 and ZnPcMe and of E. faecalis biofilm with MB (in each case the concentration of 6 μM and incubation time of 1.5 h) was found. In contrast to the planktonic forms of the same pathogenic cells, a concentration of 3 μM of the sensitizers and incubation time of 15 min were necessary for photoinactivation. The decrease in the number of surviving MRSA and E. faecaliscells in biofilms was approximately 0.8–1.5 log for GaPc1. A slight inactivation was obtained for C. albicans biofilm for GaPc1. The photodynamic response on biofilms showed that GaPc2 has an analogous activity to ZnPcMe, which has the same but four methylpyridyloxy functional groups (Fig. 8b,d).
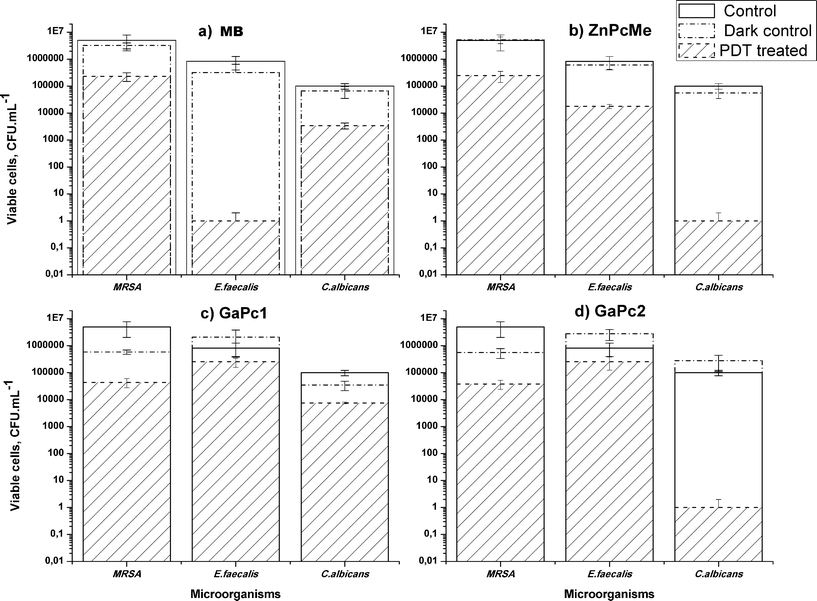 |
| Fig. 8
PDT effect on biofilms of the bacteria MRSA and E. faecalis, and the fungus C. albicans formed on acrylic resin discs. The samples were incubated for 1.5 h with 6 μM PS as follows: MB (a), ZnPcMe (b), GaPc1 (c) and GaPc2 (d) and irradiated with a red LED, 635 nm (60 mW cm−2, 50 J cm−2). The data are presented as means ± SD (n = 5). | |
The different susceptibility of the pathogens to the applied photosensitizers can be explained by several factors. It should be considered that the generation time of the fungus is longer than for the bacterial species.22 The different chemical structure of the phthalocyanines (GaPcs) and the phenothiazine dye (MB) is important. The structural differences between bacterial and fungal biofilms and the stage of the maturity of the studied biofilms are crucial factors for effectiveness of the treatment.26
The present study explores the intermediate biofilms (18 h) of bacteria and fungus cells. The inactivation of an early stage biofilm (4 h) of the pathogens MRSA and E. faecalis grown on acrylic resins treated with ZnPcMe was successful.30 As was mentioned above, the maturity for bacterial and fungal biofilms is different. The assessment of the PDT effect towards different stages of biofilm formation (4 h vs. 18 h) showed that the number of survived cells decreased by 4–5 log to the full cell death in case of 4 h biofilm and respectively increased in the same order in case of 18 h biofilm. This can be explained by the structure of an early stage biofilm, which varies in density forming areas with water channels.26 For that reason, an early stage biofilm exhibit an optimal depth of photosensitizer accumulation and favours further photodynamic mechanism involving ROSs.36 Singlet oxygen is known to directly affect polysaccharides, which are present in the extracellular matrix of a microbial biofilm.37 The molecular oxygen supply seems to be significant in case of 4 hour formed biofilm and an effective destruction of the extracellular matrix of a microbial biofilm can occur. The penetration of the photosensitizer into the biofilms during different stages of biofilm growth is also a very important. The penetration depth of the studied GaPcs and also of the referent ZnPcMe for 18 h biofilms of bacteria and fungus pathogens was similar (∼75%). However, the effect was complete inactivation of C. albicans biofilm with GaPc2 and negligible with GaPc1. This observation can be explained by the higher uptake of GaPc2 in C. albicans biofilms compared to GaPc1 (Fig. 6, 7). The high accumulation of GaPc2 into fungus cells was also observed by CSLM study (Fig. 6b, 7b). The limited susceptibility of bacteria biofilms to photodynamic treatment can be overcome by several combined treatment procedures.38–40
The application of conventional therapy together with PDT improves a limited transfer of antibiotics through the biofilm channels.38 The therapy of vancomycin and PDT with tetra-substituted N-methylpyridylporphine was reported as effective against Staphylococcus epidermidis biofilms.39 Other approaches to improve the PDT effect using MB is the application of the photomechanical wave for drug delivery or nanoparticles with photosensitizer.40In vitro studies suggest that octa-methylpyridyloxy-substituted GaPc2 is a suitable photosensitizer towards fungus C. albicans grown as biofilm on denture resin.
Conclusions
Two water soluble tetra- and octa-methylpyridyloxy-substituted phthalocyanine Ga(III) complexes (GaPc1 and GaPc2) were prepared in good yields. The compounds are non-aggregated in aqueous solution and appear to be a good prospect for photodynamic therapy. Cellular uptake and photoinactivation ability towards different bacteria species, namely the Gram-positive methicillin-resistant S. aureus and E. faecalis, the Gram-negative P. aeruginosa, and, in addition, the fungus C. albicans, all in planktonic phase were studied. Relatively high accumulations of the GaPcs towards the studied bacteria and fungus cells were determined. The uptake capacity of the octa-substituted GaPc2, which has eight positive charges of the methylpyridyloxy group, was slightly higher in comparison to the tetra-substituted GaPc1. The strong dependence of the uptake on the cell density was observed also for GaPcs.
The efficiency of the photodynamic inactivation of pathogenic microorganisms with cationic photosensitizers was confirmed in the present work with GaPcs. For example, the bacteria suspensions treated with 3 μM GaPc2 reduced the survival fraction from 4–5 log to full cell death of the selected bacteria (MRSA and E. faecalis) and of fungus C. albicans. The Gram-negative P. aeruginosa treated with GaPcs showed poor photodynamic response (1–2 log) within a wide range of therapeutic conditions. Comparing the results for the pathogens as biofilm cultures, only the fungus biofilm was susceptible to photodynamic action of GaPc2. The biofilms of MRSA and E. faecalis was slightly inactivated (0.7–1.5 log) with GaPc2. The treatment on C. albicans biofilms with the known photosensitizers (MB and ZnPcMe) showed only 0.5–1.0 log inactivation for MB and full inactivation for ZnPcMe. The susceptibility of C. albicans biofilm to PDT with GaPc2 and ZnPcMe suggests a potential value of the both complexes for treatment of denture associated infections.
Acknowledgements
The work was supported of the National Science Fund, Bulgaria (Grants: B1534/05, DO-02-177/08, DO-02-112/08). VM thanks to the DAAD, Germany (Grant, A/08/01935) and to Dr Günter Schnurpfeil for help in synthesis. The spectrometer Bruker Avance AV600 II+ 600 NMR was purchased with Grant UNA-17/2005 of the National Science Fund, Bulgaria.
References
- T. Dai, Y.-Y. Huang and M. R. Hamblin, Photodynamic therapy for localized infections – State of the art, Photodiagn. Photodyn. Ther., 2009, 6(3–4), 170–188 CrossRef CAS.
-
R. Bonnett, in Chemical aspects in Photodynamic therapy (Advanced chemistry texts), ed. D. Phillips, P. O'Brien and S. Roberts, CRC Press, London 1st edn, 2000, vol. 1, ch. 1, pp. 1–13 Search PubMed.
- G. Jori, C. Fabris, M. Soncin, S. Ferro, O. Coppellotti, D. Dei, L. Fantetti, G. Chiti and G. Roncucci, Photodynamic therapy in the treatment of microbial infections: Basic principles and perspective applications, Lasers Surg. Med., 2006, 38, 468–481 CrossRef.
- M. R. Hamblin and T. Hasan, Photodynamic therapy: a new antimicrobial approach to infection disease?, Photochem. Photobiol. Sci., 2004, 3(5), 436–450 RSC.
- P. Meisel and T. Kocher, Photodynamic therapy for periodontal diseases: state of the art, J. Photochem. Photobiol., B, 2005, 79(2), 159–170 CrossRef CAS.
- W. Spiller, H. Kliesch, D. Wöhrle, S. Hackbarth and B. Roeder, Singlet oxygen quantum yield of different photosensitizers in polar solvents and micellar solutions, J. Porphyrins Phthalocyanines, 1998, 2, 145–158 CrossRef CAS.
- L. B. Josefsen and R. W. Boyle, Photodynamic therapy of the development of metal-based photosensitizers, Met.-Based Drugs, 2008, 2008, 276109 Search PubMed.
- S. Moeno and T. Nyokong, Solvent and central metal effects on the photophysical and photochemical properties of peripherally tetra mercaptopyridine substituted metallophthalocyanines, J. Photochem. Photobiol., A, 2009, 203, 204–210 CrossRef CAS.
- M. Durmus and T. Nyokong, The synthesis, fluorescence behaviour and singlet oxygen studies of new water-soluble cationic gallium(III) phthalocyanine, Inorg. Chem. Commun., 2007, 10, 332–338.
- M. Durmus and T. Nyokong, Synthesis, photophysical and photochemical properties of tetra- and octa-substituted gallium and indium phthalocyanines, Polyhedron, 2007, 26, 3323–3335 CrossRef CAS.
- V. Chauke, A. Ogunsipe, M. Durmus and T. Nyokong, Novel gallium(III) phthalocyanine derivatives -Synthesis, photophysics and photochemistry, Polyhedron, 2007, 26, 2663–2671 CrossRef CAS.
- C. M. Cassidy, M. M. Tunney, P. A. McCarron and R. F. Donnelly, Drug delivery strategies for photodynamic antimicrobial chemotherapy, J. Photochem. Photobiol., B, 2009, 95, 71–80 CrossRef CAS.
- A. Minnock, D. I. Vernon, J. Schofield, J. Griffits, J. H. Parish and T. S. Brown, Photoinactivation of bacteria. Use of cationic water-soluble zinc-phthalocyanine to inactivate both Gram-negative and Gram-positive bacteria, J. Photochem. Photobiol., B, 1996, 32, 159–164 CrossRef CAS.
- G. Bertoloni, F. Rossi, G. Valduga, G. Jori and J. E. Van Lier, Photosensitizing activity of water- and lipid-soluble phthalocyanines on Escherichia coli, FEMS Microbiol. Lett., 1990, 71, 149–156 CrossRef CAS.
- I. Scalise and E. N. Durantini, Synthesis, properties and photodynamic inactivation of Escherichia coli using cationic and a noncharged Zn(II) pyridyloxyphthalocyanine derivatives, Bioorg. Med. Chem., 2005, 13, 3037–3045 CrossRef CAS.
- E. Reddi, M. Ceccon, G. Valduga, G. Jori, J. C. Bommer, F. Elisei, L. Latterini and U. Mazzucato, Photophysical properties and antibacterial activity of meso-substituted cationic porphyrins, Photochem. Photobiol., 2002, 75, 462–470 CrossRef CAS.
- A. Minnock, D. I. Vernon, J. Schofield, J. Griffiths, J. H. Parish and S. B. Brown, Mechanism of uptake of a cationic water-soluble pyridinium zinc phthalocyanine across the outer membrane of Escherichia coli, Antimicrob. Agents Chemother., 2000, 44, 522–527 CrossRef CAS.
- V. Kussovski, V. Mantareva, I. Angelov, P. Orozova, D. Wöhrle, G. Schnurpfeil, E. Borisova and L. Avramov, Photodynamic inactivation of Aeromonas hydrophila by cationic phthalocyanines with different hydrophobicity, FEMS Microbiol. Lett., 2009, 294, 133–140 CrossRef CAS.
- V. Mantareva, V. Kussovski, I. Angelov, E. Borisova, L. Avramov, G. Schnurpfeil and D. Wöhrle, Photodynamic activity of water-soluble phthalocyanine zinc(II) complexes against pathogenic microorganisms, Bioorg. Med. Chem., 2007, 15, 4829–4835 CrossRef CAS.
- I. Angelov, V. Mantareva, V. Kussovski, D. Wöhrle, E. Borisova and L. Avramov, Improved antimicrobial therapy with cationic tetra- and octa-substituted phthalocyanines, Proceedings of SPIE, Lasers Biol. Med., 2008, 7027, 702–717 Search PubMed.
- L. Costa, E. Alves, C. M. B. Carvalho, J. P. C. Tome, M. A. F. Faustino, M. G. P. M. S. Neves, A. C. Tome, J. A. S. Cavaleiro, A. Cunha and A. Almeida, Sewage bacteriophage photoinactivation by cationic porphyrins: a study of charge effect, Photochem. Photobiol. Sci., 2008, 7, 415–422 RSC.
- M. E. Davey and G. A. O'Toole, Microbial biofilms: from ecology to molecular genetics, Microbiol. Mol. Biol. Rev., 2000, 64, 847–867 CrossRef CAS.
- H. Anwar, M. K. Dasgupta and J. W. Costerton, Testing the susceptibility of bacteria in biofilms to antibacterial agents, Antimicrob. Agents Chemother., 1990, 34, 2043–2046 CAS.
- J. Dobson and M. Wilson, Sensitization of oral bacteria in biofilms to killing by light from a low-power laser, Arch. Oral Biol., 1992, 37, 883–887 CrossRef CAS.
- G. O'Tool, H. B. Caplan and R. Kolter, Biofilm formation as microbial development, Annu. Rev. Microbiol., 2000, 54, 49–79 CrossRef.
- J. Chandra, D. M. Kuhn, P. K. Mukherjee, L. L. Hoyer, T. McCormick and M. A. Ghannoum, Biofilm formation by the fungal pathogen Candida albicans: development, architecture, and drug resistance, J. Bacteriol., 2001, 183, 5385–5394 CrossRef CAS.
- C. F. Lee, C. J. Lee, C. T. Chen and C. T. Huang, delta-Aminolaevulinic acid mediated photodynamic antimicrobial therapy on Pseudomonas aeruginosa planktonic and biofilm cultures, J. Photochem. Photobiol., B, 2004, 75, 21–25 CrossRef CAS.
- K. Konopka and T. Goslinski, Photodynamic therapy in dentistry, J. Dent. Res., 2007, 86(8), 694–707 CrossRef CAS.
- V. Mantareva, I. Angelov, V. Kussovski, D. Wöhrle and S. Dimitrov, Metallophthalocyanines as photodynamic sensitizers for treatment of pathogenic bacteria. Synthesis and singlet oxygen formation, C. R. Acad. Bulg. Sci., 2009, 62(12), 1521–1526 Search PubMed.
- V. Mantareva, I. Angelov, V. Kussovski, D. Wöhrle and S. Dimitrov, Metallophthalocyanines as photodynamic sensitizers for treatment of pathogenic bacteria. Uptake and photoinactivation properties, C. R. Acad. Bulg. Sci., 2010, 63(1), 77–84 Search PubMed.
- D. Wöhrle, N. Iskander, G. Graschew, H Sinn, E. A. Friedrich, W. Maier-Borst, J. Stern and P. Schlag, Synthesis of positively charged phthalocyanines and their activity in the photodynamic therapy of cancer cells, Photochem. Photobiol., 1990, 51, 351–356 CAS.
- A. Ogunsipe, D. Maree and T. Nyokong, Solvent effects on the photochemical and fluorescence properties of zinc phthalocyanine derivatives, J. Mol. Struct., 2003, 650, 131–140 CrossRef CAS.
- V. Mantareva, D. Petrova, L. Avramov, I. Angelov, E. Borisova, M. Peeva and D. Wöhrle, Long wavelength absorbing cationic Zn(II)-phthalocyanines as fluorescent contrast agents for B16 pigmented melanoma, J. Porphyrins Phthalocyanines, 2005, 9(1), 47–53 CrossRef CAS.
- Zh. Xu, G. Bai and Ch. Dong, Spectral and photophysical properties of intramolecular charge transfer fluorescence probe: 4-Dimethylamino-2,5-dihydroxychalcone, Spectrochim. Acta, Part A, 2005, 62, 987–990 CrossRef.
- T. N. Demidova and M. R. Hamblin, Effect of cell-photosensitizer binding and cell-density on microbial photoinactivation, Antimicrob. Agents Chemother., 2005, 49, 2329–2335 CrossRef CAS.
- M. Wainwright and K. B. Crossley, Photosensitising agents–circumventing resistance and breaking down biofilms: a review, Int. Biodeterior. Biodegrad., 2004, 53, 119–126 Search PubMed.
- T. Maisch, J. Baier, B. Franz, M. Maier, M. Landthaler, R.-M. Szeimies and W. Baumler, The role of singlet oxygen inactivation of bacteria, Proc. Natl. Acad. Sci. U. S. A., 2007, 104(17), 7223–7228 CrossRef CAS.
- A. Di Poto, M. S. Sbarra, G. Provenza, L. Visai and P. Speziale, The effect of photodynamic treatment combined with antibiotic action or host defence mechanisms on Staphylococcus aureus biofilms, Biomaterials, 2009, 30, 3158–3166 CrossRef CAS.
- M. S. Sbarra, C. R. Arciola, A. Di Poto, E. Saino, H. Rohde, P. Speziale and L. Visai, The photodynamic effect of tetra-substituted N-methyl-pyridyl-porphine combined with the action of vancomycin or host defense mechanisms disrupts Staphylococcus epidermidis biofilms, Int. J. Artif. Organs, 2009, 32(9), 574–583 Search PubMed.
- T. C. Pagonic, J. Chen, C. R. Fontana, H. Devalapally, K. Ruggiero, X. Song, F. Foschi, J. Dunham, Z. Skobe, H. Yamazaki, R. Kent, A. C. Tanner, M. M. Amiji and N. S. Soukos, Nanoparticles-based Endodontic Antimicrobial Photodynamic therapy, J. Endod., 2010, 36(2), 322–328 Search PubMed.
|
This journal is © The Royal Society of Chemistry and Owner Societies 2011 |
Click here to see how this site uses Cookies. View our privacy policy here.