DOI:
10.1039/C1MD00081K
(Concise Article)
Med. Chem. Commun., 2011,
2, 644-649
Structure–activity relationships of gramicidin S analogs containing (β-3-pyridyl)-α,β-dehydroalanine residues on membrane permeability†
Received
22nd March 2011
, Accepted 18th April 2011
First published on 19th May 2011
Abstract
The synthesis, biological activities and membrane permeability of gramicidin S (GS) analogs containing (Z)-(β-3-pyridyl)-α,β-dehydroalanine (ΔZ3Pal) residues are described. The cationic side chains of the Orn and ΔZ3Pal4,4′ residues in [ΔZ3Pal4,4′]GS (1) are important for dissociating antimicrobial and hemolytic activity.
Introduction
The global spread of multidrug-resistant bacteria is a growing threat to human health.1 Cationic antimicrobial peptides (CAPs) that attack bacterial membranes are promising agents for combating bacterial pathogens.2 Among various CAPs, cyclic decapeptide gramicidin S (GS, cyclo(Val-Orn-Leu-D-Phe-Pro)2) exhibits potent antimicrobial activity against both Gram-positive and Gram-negative bacteria.3–5 GS is generally believed to perturb lipid packing, resulting in the destruction of the cytoplasmic membrane's integrity and enhancement of its permeability.6 Unfortunately, the use of GS for therapeutic purposes has been limited to topical application because of its high toxicity to human red blood cells. Therefore, the structure–activity relationships of GS and related cyclic peptides have been extensively studied in order to dissociate their antimicrobial and hemolytic activities.7
It is believed that a stable antiparallel β-sheet conformation containing two type II′ β-turn moieties are essential for the bioactive conformation of GS.3–5,8,9 Recent breakthroughs in dissociating antimicrobial and hemolytic activities include ring-size modulation of the GS backbone10 and the disruption of amphiphilic structure by a single D-amino acid substitution.11 Mutation of β-turn moieties often reduces unwanted hemolytic activity.4,12–14 For example, [D-Tyr4,4′]GS, a water-soluble analog of GS carrying phenolic substituents at the β-turn moieties, exhibits weaker hemolytic activity than wild-type GS.4 Incorporation of water-soluble β-turn inducers such as γ-amino-Pro derivatives12 or sugar amino acids13 is also effective in reducing hemolytic activity, whereas aryl substituents in the turn regions are indispensable for bactericidal action.13c These results prompted us to incorporate β-turn mimetics carrying hydrophilic aryl substituents into the GS framework.
Among various β-turn mimetics,14 α,β-dehydro amino acids (ΔAAs) have been used in the de novo design of peptides with ordered structure.15,16 Recently, we developed (Z)-(β-3-pyridyl)-α,β-dehydroalanine (ΔZ3Pal), an α,β-unsaturated analog of (β-3-pyridyl)-alanine (3Pal)17 (Fig. 1a). Our preliminary study demonstrated that the 4,4′-ΔZ3Pal analog of GS ([ΔZ3Pal4,4′]GS, 1) scarcely induces hemolysis but exhibits potent antimicrobial activity.18a In addition to our study, some low-hemolytic 10-mer GS analogs with C2-symmerty have been reported.14f,18b Since the mechanisms of action of 1 on bacterial membranes are not fully understood, we explored the structure–activity relationship of ΔZ3Pal-GS on membrane permeability. Various techniques are available for evaluating the permeability of membrane-active drugs. In the present study, a K+-selective electrode was used to evaluate the membrane permeability of ΔZ3Pal-GS. This method enabled us to simultaneously measure changes in the permeability of the cytoplasmic membranes of Gram-positive and Gram-negative bacteria in order to directly evaluate the actions of CAPs in situ.6a,19
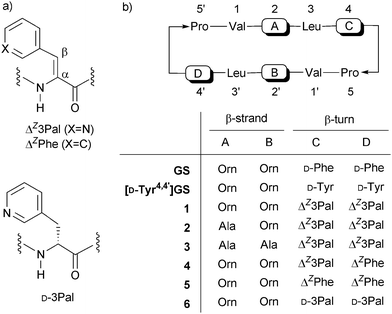 |
| Fig. 1 (a) ΔZ3Pal, ΔZPhe and D-3Pal and (b) GS analogs in this study. | |
Previous studies revealed that the aromatic and conjugated planes in ΔZPhe4,4′ residues play a crucial role in membrane–peptide interactions and/or the permeation of the peptides through phospholipid bilayers, since [Ala2,2′,ΔZPhe4,4′]GS exhibited antimicrobial activity despite its lack of cationic Orn residues.20 Furthermore, this dehydropeptide enhanced K+ efflux from human erythrocytes, resulting in changes in the morphology of the erythrocytes, while inactive [Ala2,2′]GS scarcely induced any changes. Therefore, we synthesized novel analogs of 1 lacking cationic Orn residues, namely [Ala2,ΔZ3Pal4,4′]GS (2) and [Ala2,2′,ΔZ3Pal4,4′]GS (3), and evaluated their membrane permeability. We report the synthesis, biological activities and bacterial membrane permeability of 1–3. In addition, the membrane permeability of previously reported analogs related to 1, namely [ΔZPhe4,ΔZ3Pal4′]GS (4), [ΔZPhe4,4′]GS (5) and [D-3Pal4,4′]GS (6, D-3Pal = (β-3-pyridyl)-D-alanine), were also studied and their characteristics were compared to those of 1–3.
Results and discussion
Synthesis of GS analogs containing ΔZ3Pal residues
The protected tridehydropeptide ester containing a ΔZ3Pal residue was synthesized using the Erlenmeyer azlactone method.16(β-3-Pyridyl)-DL-serine (7) is a key intermediate for synthesizing ΔZ3Pal-containing peptides. In our previous studies, 7 was synthesized by treating COMPOUND LINKS
Read more about this on ChemSpider
Download mol file of compoundglycine with COMPOUND LINKS
Read more about this on ChemSpider
Download mol file of compoundpyridine-3-carboxaldehyde (3.5 equiv.) and NaOEt (3 equiv.), and was used without further purification.16 In the present study, COMPOUND LINKS
Read more about this on ChemSpider
Download mol file of compoundethyl glycinate was used instead of COMPOUND LINKS
Read more about this on ChemSpider
Download mol file of compoundglycine. The desired compound 7 could be isolated in 83% yield and was characterized by 1H NMR, ESI-MS and elemental analysis. Derivatization of 7 to the protected tridehydropeptide, Boc-Leu-ΔZ3Pal-Pro-OMe (8), was carried out essentially as described previously.17
[Ala2,ΔZ3Pal4,4′]GS (2) and [Ala2,2′,ΔZ3Pal4,4′]GS (3), which are analogs of 1 lacking one or two Orn residues, respectively, were synthesized from 8 as previously reported.18aScheme 1 shows the synthesis of 2. The formyl (For) group was employed as a protecting group on the Orn side-chain. Stepwise coupling of Boc-amino acids was carried out using the EDC–HOBt method. Segment condensation between 12 and 13 afforded fully protected linear decapeptide 14 in good yield. After deprotection, the peptide was highly diluted in DMF and head-to-tail cyclization was achieved using HATU in DMF to afford [Ala2,Orn(For)2′, ΔZ3Pal4,4′]GS (16) in 37% yield. The side-chain For group was removed with 10% methanolic HCl to afford the desired GS analog 2. Compound 3 was also synthesized from protected pentadehydropeptide ester 10 in a similar manner (Scheme 2). These peptides and intermediates were characterized by 1H NMR and ESI-MS.
![Solution-phase synthesis of [Ala2,ΔZ3Pal4,4′]GS (2). Reagents and conditions: (a) TFA, rt, (b) 9: Boc-Ala-OH, EDC·HCl, HOBt, CH2Cl2, 0 °C to rt; 10: Boc-Orn(For)-OH, EDC·HCl, HOBt, CH2Cl2, 0 °C to rt, (c) Boc-Val-OH, EDC·HCl, HOBt, CH2Cl2, 0 °C to rt, (d) 1 M NaOH, MeOH : H2O = 1 : 1 (v/v), 0 °C to rt, (e) EDC·HCl, HOBt, CH2Cl2, 0 °C to rt, (f) HATU (1.6 equiv.), DIEA (6.0 equiv.), DMF (peptide conc.: 0.73 mM), 0 °C to rt, (g) 10% methanolic HCl, rt.](/image/article/2011/MD/c1md00081k/c1md00081k-s1.gif) |
| Scheme 1 Solution-phase synthesis of [Ala2,ΔZ3Pal4,4′]GS (2). Reagents and conditions: (a) TFA, rt, (b) 9: COMPOUND LINKS
Read more about this on ChemSpider
Download mol file of compoundBoc-Ala-OH, EDC·HCl, COMPOUND LINKS
Read more about this on ChemSpider
Download mol file of compoundHOBt, CH2Cl2, 0 °C to rt; 10: Boc-Orn(For)-OH, EDC·HCl, COMPOUND LINKS
Read more about this on ChemSpider
Download mol file of compoundHOBt, CH2Cl2, 0 °C to rt, (c) COMPOUND LINKS
Read more about this on ChemSpider
Download mol file of compoundBoc-Val-OH, EDC·HCl, COMPOUND LINKS
Read more about this on ChemSpider
Download mol file of compoundHOBt, CH2Cl2, 0 °C to rt, (d) 1 M COMPOUND LINKS
Read more about this on ChemSpider
Download mol file of compoundNaOH, COMPOUND LINKS
Read more about this on ChemSpider
Download mol file of compoundMeOH : COMPOUND LINKS
Read more about this on ChemSpider
Download mol file of compoundH2O = 1 : 1 (v/v), 0 °C to rt, (e) EDC·HCl, COMPOUND LINKS
Read more about this on ChemSpider
Download mol file of compoundHOBt, CH2Cl2, 0 °C to rt, (f) HATU (1.6 equiv.), DIEA (6.0 equiv.), DMF (peptide conc.: 0.73 mM), 0 °C to rt, (g) 10% methanolic HCl, rt. | |
![Solution-phase synthesis of [Ala2,2′,ΔZ3Pal4,4′]GS (3). Reagents and conditions: (a) 1 M NaOH aq., MeOH : H2O = 1 : 1 (v/v), 0 °C to rt, (b) 13, EDC·HCl, HOBt, NMM, CH2Cl2, 0 °C to rt, (c) TFA, rt, and (d) HATU (1.6 equiv.), DIEA (6.0 equiv.), DMF (peptide conc.: 0.73 mM), 0 °C to rt.](/image/article/2011/MD/c1md00081k/c1md00081k-s2.gif) |
| Scheme 2 Solution-phase synthesis of [Ala2,2′,ΔZ3Pal4,4′]GS (3). Reagents and conditions: (a) 1 M COMPOUND LINKS
Read more about this on ChemSpider
Download mol file of compoundNaOH aq., COMPOUND LINKS
Read more about this on ChemSpider
Download mol file of compoundMeOH : COMPOUND LINKS
Read more about this on ChemSpider
Download mol file of compoundH2O = 1 : 1 (v/v), 0 °C to rt, (b) 13, EDC·HCl, COMPOUND LINKS
Read more about this on ChemSpider
Download mol file of compoundHOBt, NMM, CH2Cl2, 0 °C to rt, (c) TFA, rt, and (d) HATU (1.6 equiv.), DIEA (6.0 equiv.), DMF (peptide conc.: 0.73 mM), 0 °C to rt. | |
The main-chain conformation of GS and analogs has been established as an antiparallel β-sheet conformation with two type II' β-turn by various spectroscopic studies.8 According to Shimohigashi et al.,16 the main-chain conformation of [ΔZPhe4,4′]GS (5) was estimated as a similar β-sheet conformation to that of wild-type GS. Table 1 shows temperature coefficients of the amide NHs and 3JNH–CH coupling constants of 1–3. In all cases, four NαHs of Val1,1′ and Leu3,3′ were of hydrogen-bonded and 3JNH–CH values of Val, Orn and Leu residues (ranging from 8.6 to 9.5 Hz) correspond to β-sheet conformation. CD spectral analysis is also useful in studying conformational change of GS analogs from parent GS. We previously reported that the CD spectrum of 1 in COMPOUND LINKS
Read more about this on ChemSpider
Download mol file of compoundMeOH is quite similar to that of ΔZPhe4,4′ analog 5, indicating 1 adopts GS-like β-sheet conformation.18aCD spectra of the Ala-containing analogs 2 and 3 resemble that of 1 (see Fig. S3 in the ESI†). These data also supported that ΔZ3Pal4,4′ analogs 1–3 essentially adopt a β-sheet conformation similar to that of wild-type GS and 5.
|
Val
|
Orn/Ala |
Leu |
ΔZ3Pal |
3
J
NH–CH values are in the parentheses and are measured at 20 °C.
Upper: Val1-ΔZ3Pal4. Lower: Val1′-ΔZ3Pal4′.
Overlapped with aromatic protons.
Singlet due to no α-proton.
|
GS |
−1.8 (—c) |
−4.8 (9.0) |
−2.8 (9.0) |
−7.2 (3.5) |
1
|
−0.9 (10.0) |
−5.6 (9.5) |
−2.6 (8.5) |
−4.6 (—d) |
2
|
−0.8 (9.2) |
−6.7 (8.5) |
−3.2 (8.2) |
−5.6 (—d) |
3
b
|
−0.8 (10.1), −0.9 (9.3) |
−5.9 (7.8), −6.2 (—) |
−2.7 (8.7), −2.6 (8.9) |
−4.6 (—d), −4.6 (—d) |
Antimicrobial and hemolytic activities
The biological activities of GS analogs 1–6 were evaluated using a method similar to that reported previously.12,19 The minimum inhibitory concentration (MIC) and ED50 (concentration giving 50% hemolysis) values of 1–6 are shown in Table 2. Dose-dependence curves of the hemolysis of human erythrocytes induced by GS and analogs 1–6 are shown in Fig. 2. Analog 1 exhibited antimicrobial activity against S. aureus comparable to that of GS, but its hemolytic ED50 value was much lower than that of GS. Analog 2, which possesses one Orn residue, was active only against Gram-positive Staphylococcus aureus, but its activity was slightly weaker than that of 1. Analog 3, which contains no Orn residues, was inactive against both Gram-positive and Gram-negative bacteria, indicating that at least one cationic side-chain, such as that of an Orn residue, is crucial for the bactericidal action of ΔZ3Pal-containing GS analogs. Although both 2 and 3 strongly induced hemolysis at concentrations over 50 μM, their hemolytic activity was much weaker than that of ΔZPhe-containing GS (4 and 5) and [D-Tyr4,4′]GS.
Table 2 Antimicrobial and hemolytic activities of GS analogsa,d
|
MICb,c |
ED50d/μM |
S. aureus FDA 209P |
E. coli K12 W3110 |
Human erythrocyte |
MIC values were determined by the liquid-broth method using two-fold dilution techniques.
MIC in μg ml−1.
The values in parentheses indicate MIC in μM.
Peptide concentration giving 50% hemolysis.
|
GS |
4 (3) |
16 (13) |
5–10 |
[D-Tyr4,4′]GS |
4 (3) |
32 (26) |
10–20 |
1
|
8 (6) |
32 (26) |
>100 |
2
|
16 (13) |
128 (106) |
50–100 |
3
|
128 (114) |
>128 (>114) |
50–100 |
4
|
4 (3) |
32 (26) |
20–50 |
5
|
4 (3) |
32 (26) |
5–10 |
6
|
64 (50) |
64 (50) |
>100 |
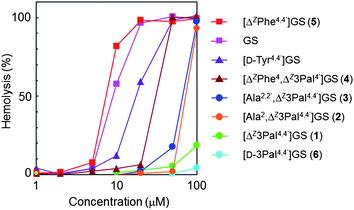 |
| Fig. 2 Dose-dependence curves of hemolysis induced by GS and analogs (1–6). | |
Bacterial membranes are composed of acidic phospholipids and hence are more negatively charged than membranes in mammalian cells, which are mainly composed of zwitterionic phospholipids and COMPOUND LINKS
Read more about this on ChemSpider
Download mol file of compoundcholesterol. The selectivity of CAPs toward biomembranes is governed by the net positive charge and amphiphilicity of the peptide.21 Although substitution of Orn residues in 1 by Ala enhanced hemolytic activity (analogs 2 and 3), the activities of 2 and 3 at concentrations below 50 μM were much weaker than those of GS and [D-Tyr4,4′]GS, even though GS and [D-Tyr4,4′]GS lack a cationic Orn residue. Hemolysis was also suppressed by the introduction of one ΔZ3Pal residue (analog 4) or two D-3Pal residues (analog 6). It appears that hydrophilic pyridyl groups at β-turn moieties significantly weaken the interactions between GS analogs and erythrocyte membranes. As a result, the erythrocyte cells are not destroyed.22
Effect of ΔZ3Pal residues on the permeability of bacterial membranes
Disruption of cytoplasmic membranes induced by GS leads to the efflux of K+.19 To evaluate the effect of ΔZ3Pal residues on membrane permeability, changes in K+ efflux upon treatment with GS analogs 1–6 were measured using a K+-selective electrode.19,23 Typical results for wild-type GS and 1 are shown in Fig. 3. Both peptides induced a change in the K+ permeability of Gram-positive S. aureus in a dose-dependent manner. The decrease in cell viability of S. aureus was closely associated with the increase in K+ permeability. As summarized in Table 3, the ED50 (peptide concentration giving 50% K+ efflux) and LC50 (peptide concentration giving 50% decrease in cell survival) values of 1 and 2 toward S. aureus were similar to those of wild-type GS. In contrast, analog 3, which lacks Orn residues, scarcely induced K+ permeability in S. aureus and therefore exhibited much weaker activity than [Ala2,2′,ΔZPhe4,4′]GS or the other analogs investigated in this study. The antimicrobial and hemolytic activities of analog 4 were comparable to those of [D-Tyr4,4′]GS, indicating that the introduction of one ΔZ3Pal residue into the β-turn moiety reduces affinity for zwitterionic erythrocyte membranes. [D-3Pal4,4′]GS (6), a saturated analog of 1, exhibited similar antimicrobial activity and membrane permeability toward Gram-negative E. coli, but much weaker activity toward Gram-positive S. aureus. These results suggest that replacing the phenylalanine side-chain by hydrophilic aromatics at the β-turn moiety reduces affinity not only to erythrocytes, but also to Gram-positive bacteria. However, it is notable that the aromatic and conjugated planes in ΔZ3Pal4,4′ residues significantly aid permeability through the cytoplasmic membrane of Gram-positive bacteria. In summary, substitution of one or two Orn residues by Ala significantly reduced K+ efflux through E. colimembranes, indicating that cationic amino acid residues in ΔZ3Pal-GS are indispensable for bactericidal action, especially toward Gram-negative bacteria.
![Dose-dependence curves of K+ efflux and cell viability of bacteria upon addition of (a) [ΔZ3Pal4,4′]GS (1) and (b) wild-type GS.](/image/article/2011/MD/c1md00081k/c1md00081k-f3.gif) |
| Fig. 3 Dose-dependence curves of K+ efflux and cell viability of bacteria upon addition of (a) [ΔZ3Pal4,4′]GS (1) and (b) wild-type GS. | |
Table 3
ED50 (the concentration required for 50% K+ release from bacteria) and LC50 (the concentration required for 50% decrease in cell survival) of GS and analogsa
|
S. aureus FDA 209P |
E. coli K12 W3110 |
ED50a/μM |
LC50a/μM |
ED50a/μM |
LC50a/μM |
Cells were incubated with a peptide for 30 min at 37 °C.
|
GS |
2–5 |
5 |
20–50 |
20–50 |
1
|
5–10 |
10–20 |
50–100 |
50–100 |
2
|
10 |
10–20 |
>100 |
>100 |
3
|
50–100 |
50–100 |
>100 |
>100 |
4
|
5–10 |
10 |
50 |
50 |
5
|
5–10 |
5–10 |
20–50 |
20–50 |
6
|
20–50 |
20–50 |
50–100 |
100 |
The action of ΔZ3Pal-GS on the outer and cytoplasmic membranes of E. coli was investigated according to a previously published methodology.24,25 The outer membrane (OM) of Gram-negative bacteria plays an important role as a protective permeability barrier for the cell. Polyamine compounds such as polymyxin B (PMB) and its analogs act as OM destabilizers, enabling hydrophobic antibiotics to permeate through the inner membranes.24,25 To evaluate the permeabilizing action of 1 toward the outer and cytoplasmic membranes of E. coli, we measured the antimicrobial activity of 1 in the presence of polymyxin B nonapeptide (PMBN), using COMPOUND LINKS
Read more about this on ChemSpider
Download mol file of compoundnovobiocin (NB) and wild-type GS as control antibiotics. The MICs of NB, 1 and wild-type GS in the presence of PMBN are depicted in Fig. 4. As expected, NB and wild-type GS inhibited the growth of E. coli upon the addition of PMBN. As the concentration of PMBN was increased, NB exhibited higher activity, confirming that PMBN can strongly destabilize the outer membrane of E. coli. In the case of wild-type GS, a synergistic bactericidal effect was observed (the MIC value changed from 16 μg ml−1 to 4 μg ml−1). In contrast, 1 had little effect on improving antimicrobial activity against E. coli even as the concentration of PMBN was increased. These results suggest that both wild-type GS and 1 can permeate across both the outer and cytoplasmic membrane of E. coli, but the ability of 1 to perturb the cytoplasmic membrane is weaker than that of wild-type GS.
Conclusions
The synthesis and membrane permeability of gramicidin S (GS) analogs containing (Z)-(β-3-pyridyl)-α,β-dehydroalanine (ΔZ3Pal) residues were described. The replacement of two phenyl groups in [Ala2,2′,ΔZPhe4,4′] by 3-pyridyl groups resulted in the loss of antimicrobial activity, whereas [Ala2,2′,ΔZPhe4,4′]GS was active. These results indicate that the cationic side-chains of Orn and ΔZ3Pal4,4′ residues in [ΔZ3Pal4,4′]GS (1) play important roles in membrane selectivity and permeability. Evaluation of the membrane permeability of ΔZ3Pal-containing GS analogs revealed that these analogs can permeate through the cytoplasmic membranes of Gram-positive bacteria, but their permeability of the inner membranes of Gram-negative bacteria is slightly lower. Our results provide useful information for the rational design of CAPs with low cytotoxicity.
Acknowledgements
We are grateful to Mr Kenta Uehata and Mr Koji Miyoshi for technical assistance. This work was supported by a Grant-in-Aid for Scientific Research (KAKENHI 22590036) from the Japan Society for the Promotion of Science.
References
- H. C. Neu, Science, 1992, 257, 1064 CrossRef CAS.
- M. Zasloff, Nature, 2002, 415, 389 CrossRef CAS.
-
N. Izumiya, T. Kato, H. Aoyagi, M. Waki and M. Kondo, Synthetic Aspects of Biologically Active Cyclic Peptides: Gramicidin S and Tyrocidines, Wiley, New York, 1979 Search PubMed.
-
M. Waki and N. Izumiya, Biochemistry of Peptide Antibiotics, ed. H. Kleinkauf and H. von Dhren, Walter de Gruyter, Berlin, 1990, pp. 205–244 Search PubMed.
- L. H. Kondejewski, S. W. Farmer, D. S. Wishart, R. E. W. Hancock and R. S. Hodges, Int. J. Pept. Protein Res., 1996, 47, 460 CAS.
-
(a) T. Katsu, H. Kobayashi, H. Hirota, Y. Fujita, K. Sato and U. Nagai, Biochim. Biophys. Acta, 1987, 899, 159 Search PubMed;
(b) E. J. Prenner, R. N. A. H. Lewis and R. N. McElhaney, Biochim. Biophys. Acta, 1999, 1462, 201 CrossRef CAS;
(c) M. Ashrafuzzaman, O. S. Andersen and R. N. McElhaney, Biochim. Biophys. Acta, 2008, 1778, 2814 Search PubMed;
(d) M. Jelokhani-Niaraki, R. S. Hodges, J. E. Meissner, U. E. Hassenstein and L. Wheaton, Biophys. J., 2008, 95, 3306 CrossRef CAS.
- D. L. Lee and R. S. Hodges, Biopolymers, 2003, 71, 28 CrossRef CAS.
-
(a) A. Stern, W. A. Gibbons and L. C. Craig., Proc. Natl. Acad. Sci. U. S. A., 1968, 61, 734 CAS;
(b) S. E. Hull, R. Karlsson, P. Main, M. M. Woolfson and E. J. Dodson, Nature, 1978, 275, 206 CAS;
(c) K. Yamada, M. Unno, K. Kobayashi, H. Oku, H. Yamamura, S. Araki, H. Matsumoto, R. Katakai and M. Kawai, J. Am. Chem. Soc., 2002, 124, 12684 CrossRef CAS.
- M. Kawai and U. Nagai, Biopolymers, 1978, 17, 1549 CAS.
-
(a) S. Ando, H. Nishikawa, H. Takiguchi, S. Lee and G. Sugihara, Biochim. Biophys. Acta, 1993, 1147, 42 Search PubMed;
(b) L. H. Kondejewski, S. W. Farmer, D. S. Wishart, C. M. Kay, R. E. W. Hanncock and R. S. Hodges, J. Biol. Chem., 1996, 271, 25261 CrossRef CAS;
(c) M. Kiricsi, E. J. Prenner, M. Jelokhani-Niaraki, R. N. A. H. Lewis, R. S. Hodges and R. N. McElhaney, Eur. J. Biochem., 2002, 269, 5911 Search PubMed;
(d) M. Tamaki, R. Ishii, S. Kikuchi and E. Watanabe, J. Antibiot., 2005, 58, 293 Search PubMed;
(e) M. Tamaki, K. Sawa, S. Kikuchi, M. Shindo, M. Kimura and Y. Uchida, J. Antibiot., 2006, 59, 370 Search PubMed;
(f) M. Tamaki, I. Sasaki, Y. Nakao, M. Shindo, M. Kimura and Y. Uchida, J. Antibiot., 2009, 62, 597 Search PubMed;
(g) M. Jelokhani-Niaraki, L. H. Kondejewski, L. C. Wheaton and R. S. Hodges, J. Med. Chem., 2009, 52, 2090 Search PubMed;
(h) M. Tamaki, I. Sasaki, M. Kokuno, M. Shindo, M. Kimura and Y. Uchida, Org. Biomol. Chem., 2010, 8, 1791 RSC.
-
(a) L. H. Kondejewski, D. L. Lee, M. Jelokhani-Niaraki, S. W. Farmer, R. E. W. Hancock and R. S. Hodges, J. Biol. Chem., 2002, 277, 67 Search PubMed;
(b) D. L. Lee, J.-P. S. Powers, K. Pflegerl, M. L. Vasil, R. E. W. Hancock and R. S. Hodges, J. Pept. Res., 2004, 63, 69 Search PubMed.
-
(a) M. Kawai, R. Tanaka, H. Yamamura, K. Yasuda, S. Narita, H. Umemoto, S. Ando and T. Katsu, Chem. Commun., 2003, 1264 RSC;
(b) G. M. Grotenbreg, G. E. Spalburg, A. J. De Neeling, G. A. Van der Marel, H. S. Overkleeft, J. H. van Boom and M. Overhand, Bioorg. Med. Chem., 2003, 11, 2835 CrossRef CAS;
(c) M. Kawai, H. Yamamura, R. Tanaka, H. Umemoto, C. Ohmizo, S. Higuchi and T. Katsu, J. Pept. Res., 2005, 65, 98 CrossRef CAS.
-
(a) G. M. Grotenbreg, M. S. M. Timmer, A. L. Llamas-Saiz, M. Verdoes, G. A. van der Marel, M. J. van Raaij, H. S. Overkleeft and M. Overhand, J. Am. Chem. Soc., 2004, 126, 3444 CrossRef CAS;
(b) G. M. Grotenbreg, M. Kronemeijer, M. S. M. Timmer, F. E. Oualid, R. M. van Well, M. Verdoes, E. Spalburg, P. A. V. van Hooft, A. J. de Neeling, D. Noort, J. H. van Boom, G. A. van der Marel, H. S. Overkleeft and M. Overhand, J. Org. Chem., 2004, 69, 7851 CrossRef CAS;
(c) G. M. Grotenbreg, A. E. M. Buizert, A. L. E. Llamas-Seiz, P. A. V. Spalburg, P. A. V. van Hooft, A. J. de Neeling, D. Noort, M. J. van Raaij, G. A. van der Marel, H. S. Overkleeft and M. Overhand, J. Am. Chem. Soc., 2006, 128, 7559 CrossRef CAS.
- For the use of β-turn mimetics in studies on GS. See:
(a) K. Sato and U. Nagai, J. Chem. Soc., Perkin Trans. 1, 1986, 1231 RSC;
(b) A. C. Bach, II, J. A. Markwalder and W. C. Ripka, Int. J. Pept. Protein Res., 1991, 38, 314;
(c) D. Andreu, S. Ruiz, C. Carrero, J. Alsina, F. Albericio, M. A. Jiménez, N. de la Figuera, R. Herranz, M. T. García-López and R. González-Muñiz, J. Am. Chem. Soc., 1997, 119, 10579 CrossRef CAS;
(d) G. Jeannotte and W. D. Lubell, J. Am. Chem. Soc., 2004, 126, 14334 CrossRef CAS;
(e) J. Xiao, B. Weisblum and P. Wipf, Org. Lett., 2006, 8, 4731 CrossRef CAS;
(f) C. Solanas, B. G. de la Torre, M. Fernández-Reyes, C. M. Santiveri, M. Jiménez, L. Rivas, A. I. Jiménez, D. Andreu and C. Cativiela, J. Med. Chem., 2009, 52, 664 CrossRef CAS.
-
(a) P. Mathur, S. Ramakumar and V. S. Chauhan, Biopolymers, 2004, 76, 150 CrossRef CAS;
(b) P. Mathur, N. R. Jagannathan and V. S. Chauhan, J. Pept. Sci., 2007, 13, 253 Search PubMed;
(c) Y. Inai, H. Komori and N. Ousaka, Chem. Rec., 2007, 7, 191 CrossRef CAS.
-
(a) Y. Shimohigashi, H. Kodama, S. Imazu, H. Horimoto, K. Sakaguchi, M. Waki, H. Uchida, M. Kondo, T. Kato and N. Izumiya, FEBS Lett., 1987, 222, 251 Search PubMed;
(b) S. Imazu, Y. Shimohigashi, H. Kodama, K. Sakaguchi, M. Waki, T. Kato and N. Izumiya, Int. J. Pept. Protein Res., 1988, 32, 298 Search PubMed.
- K. Yamada, H. Oku, S. Shinoda and R. Katakai, J. Pept. Res., 2005, 65, 167 Search PubMed.
-
(a) K. Yamada, S. Shinoda, H. Oku, K. Komagoe, T. Katsu and R. Katakai, J. Med. Chem., 2006, 49, 7592 Search PubMed;
(b) V. V. Kapoerchan, A. D. Knijnenburg, M. Niamat, E. Spalburg, A. J. de Neeling, P. H. Nibbering, R. H. Mars-Groenendijk, D. Noort, J. M. Otero, A. L. Llamas-Saiz, M. J. van Raaij, G. A. van der Marel, H. S. Overkleeft and M. Overhand, Chem.–Eur. J., 2010, 16, 12174 CrossRef CAS.
-
(a) T. Katsu, H. Kobayashi and Y. Fujita, Biochim. Biophys. Acta, 1986, 860, 608 CrossRef CAS;
(b) T. Katsu, M. Kuroko, T. Morikawa, K. Sanchika, Y. Fujita, H. Yamamura and M. Uda, Biochim. Biophys. Acta, 1989, 983, 135 CAS.
- T. Katsu, K. Sanchika, M. Takahashi, Y. Kishimoto, Y. Fujita, H. Yoshitomi, M. Waki and Y. Shimohigashi, Chem. Pharm. Bull., 1990, 38, 2880 Search PubMed.
-
(a) K. Matsuzaki, Biochim. Biophys. Acta, 2009, 1788, 1687 Search PubMed;
(b) S. Nakao, K. Komagoe, T. Inoue and T. Katsu, Biochim. Biophys. Acta, 2011, 1808, 490 Search PubMed.
- E. J. Prenner, R. N. A. H. Lewis, M. Jelokhani-Niaraki, R. S. Hodges and R. N. McElhaney, Biochim. Biophys. Acta, 2001, 1510, 83–92 Search PubMed.
-
(a) C. Ohmizo, M. Yata and T. Katsu, J. Microbiol. Methods, 2004, 59, 173 CrossRef CAS;
(b) K. Komagoe, H. Kato, T. Inoue and T. Katsu, Photochem. Photobiol. Sci., 2011, 11 10.1039/c0pp00376j , in press.
- M. Vaara and T. Vaara, Antimicrob. Agents Chemother., 1983, 24, 107 CAS.
- H. Urakawa, K. Yamada, K. Komagoe, S. Ando, H. Oku, T. Katsu and I. Matsuo, Bioorg. Med. Chem. Lett., 2010, 20, 1771 Search PubMed.
Footnote |
† Electronic supplementary information (ESI) available. See DOI: 10.1039/c1md00081k |
|
This journal is © The Royal Society of Chemistry 2011 |