DOI:
10.1039/C0FO00068J
(Paper)
Food Funct., 2011,
2, 124-129
Normalization genes for quantitative RT-PCR in differentiated Caco-2 cells used for food exposure studies
Received
10th July 2010
, Accepted 26th November 2010
First published on 13th December 2010
Abstract
Exposure of food products to small-intestinal-like Caco-2 cells, combined with a gene expression based response analysis can be a valuable tool to classify potential bioactive effects of food homogenates. In order to study changes in gene expression upon food exposure, a robust set of stably expressed genes is required for normalization. Here we present a set of reference genes suitable for RT-qPCR that has been validated for exposure studies with the intestinal-like Caco-2 cell line. This study identified ribosomal phosphoprotein P0 (RPLP0) and glyceraldehyde-3-phosphate dehydrogenase (GAPDH) as best reference genes. The set can be extended with β-2-microglobulin (B2M), splicing factor 3A, subunit 1 (SF3A1), and mitochondrial ribosomal protein L19 (MRPL19). Food homogenates did provoke responses in the Caco-2 cells, as was demonstrated by changed expression of NAD(P)H Quinone dehydrogenase 1 (NQO1), Claudin 4 (CLDN4), Nitric Oxide Synthase 2 (NOS2), and ATP-binding cassette, subfamily B, member 1 (ABCB1) in the same experiment. Results indicate that: i) natural food homogenates can exert effects in Caco-2 cells, and ii) stability in expression of the reference genes is not due to a lack of response of the Caco-2 cells.
Introduction
Fruit and vegetables are considered a healthy food choice and are under intensive study to identify the compounds responsible for the effects on human physiology and the underlying mechanisms. This type of research has led to the identification of many potential health promoting compounds from fruits and vegetables like sulforaphane from broccoli, and quercetin as a health compound from apples and onions.1–3 However, fruit and vegetables are more than just a carrier of a single compound, they are complex products with a wide array of compounds that interact.4 Moreover, most products we consume are processed, prepared at home, or contain all kinds of additives, so we aimed to develop a tool to analyse potential bioactivity of food products instead of individual bioactive compounds.
The gut is exposed to food we consume. These intestinal cells are important for e.g. uptake of food components, provide a barrier to unwanted pathogens and compounds, modification of compounds, and local immune responses.5 It has been shown that these intestinal functions can be modulated by food compounds and that in vitrocell lines can be used to mimic the response.5 One of such small intestine representing cell cultures is the human, colon derived, Caco-2 culture.6,3 Although originating from a colon carcinoma,7 Caco-2 cells show high morphological and physiological homology with small intestinal cells when allowed to differentiate after having formed a confluent monolayer; as they then exhibit villi and express brush border enzymes such as sucrase-isomaltase, alkaline phosphatase, and aminopeptidase N.8,9
The most versatile readout for detecting responses of cells is to determine changes in mRNA abundance, as has been studied with microarrays.10,11 Exposure of Caco-2 cells to food compounds, like quercetin or glycoalkaloids, has resulted in detectable differences in gene expression.11,12 However, analysing bioactive effects of crude food mixtures will urge for a more robust and sensitive method than microarrays, which can be found in a good controlled quantitative RT-qPCR approach with a well validated set of biomarker genes and highly stable reference genes for normalization.13,14 Sets of normalization genes have been published for use with Caco-2 cells, but these are focused on differentiation processes,15 leaving a requirement for a gene set that can be used in food exposure studies. A common way of deciding on reference genes for RT-qPCR is to compare the quantification cycles (Cq values, sometimes indicated as Ct or Cp13) of a gene in different samples, assuming that the genes with most constant Cq value has the most constant expression. This method however, results in a selection of genes which abundance is constant relative to the total amount of RNA used for cDNA synthesis, instead of having a constant expression per se. An improved method has been proposed by Vandesompele et al.,14 which is based on selecting genes having a constant ratio of expression. This method is independent of the amount of mRNA or cDNA used, and will thus give good reference genes when changes in total RNA are expected.
In this study we tested several genes that have been proposed as useful reference genes in Caco-2 cells, like the generally applied β-actin (ACTB),14,15glyceraldehyde-3-phosphate dehydrogenase (GAPDH),14 β-2-microglobulin (B2M),16 and ribosomal phosphoprotein P0 (RPLP0).16 A further expansion of the tested set comes from genes found by Szabo et al.,17 who used public microarray data to find stably expressed genes like proteasome (prosome, macropain) 26S subunit ATPase 4 (PSMC4), mitochondrial ribosomal protein L19 (MRPL19), Pumilio homologue 1 (PUM1), and splicing factor 3A, subunit 1 (SF3A1).
In order to ensure that food based effects can indeed be monitored using the designed exposure and response read out system, a selection of homogenates of crude natural foods were used. Candidate responsive genes were selected and were subsequently used in the RT-qPCR approach to study whether the used food homogenates can still provoke effects in Caco-2 cells. The observed changes in gene expression support the hypothesis that exposure to natural food homogenates did result in responses of the Caco-2 cells and that the selection of stably expressed reference genes is not caused by a lack of response of the cells to food homogenates. The changed expression of the response genes in reaction to the used food homogenates is discussed.
Materials and methods
Cell culture
Caco-2 cells were grown in 12-well tissue culture plates (Greiner bio-one, Alphen a/d Rijn, The Netherlands). Cells were seeded with 8 × 104cells cm−2 per well, and grown in Dulbecco's Modified Eagles Medium (DMEM, with 4.5 g l−1 glucose, 4 mM L-glutamine and 25 mM HEPES, Invitrogen, Paisley, UK) supplemented with 9.1% heat inactivated fetal bovine serum (FBS, Invitrogen, Paisley, UK), at 37 °C and 5% CO2. The cells in the wells reached confluence after 4 days of growth, growth was continued for 25 days when at which they showed increased expression of sucrase-isomaltase and alkaline phosphatase RNA. Medium was replaced three times a week.
Broccoli, apples, tomatoes, and mushrooms were obtained from a local supermarket. The edible parts were mashed, clear supernatant was taken and diluted 1
:
1 with DMEM + FBS medium. pH was adjusted with 1 M NaOH using the phenol red pH indicator in the DMEM. Samples were filter sterilized through 0.45 and 0.2 μm and samples were diluted further to a total of 3 or 5 times. DMEM only and water controls were included. For exposure, medium was removed from the well, and sample/DMEM mixtures were added, cells were incubated at growing conditions for 24 h. Every fruit and vegetable sample was added to three different wells which were subsequently processed and analysed separately, and are therefore considered as biological replicates. Cell and monolayer morphology did not differ between treatments and control samples, as checked by microscopic examination. MTT viability tests were performed under similar conditions as used for the RT-qPCR samples.
RNA
extraction and cDNA synthesis
Medium was removed and cells were harvested with 0.5 ml TriZol (Invitrogen, Paisley, UK). RNA was extracted with TriZol, DNaseI treated (Sigma-Aldrich, Steinheim, Germany), and purified with RNeasy mini columns (Qiagen, Hilden, Germany), all according to the manufacturers protocols. cDNA was synthesized in a volume of 20μl with iScript (Bio-Rad, Hercules, USA), using 0.2 μg total RNA as determined spectrophotometrically with a NanoDrop (NanoDrop, Wilmington, USA). cDNA was diluted 20x before being quantified by qPCR.
Primer design and qPCR
Primers were designed with Clone Manager Professional 9 and checked in silico for specificity with the NCBI primer design tool (http://www.ncbi.nlm.nih.gov/tools/primer-blast/) and UCSCin silico PCR (http://genome.ucsc.edu/). Primer efficiency and gene expression analysis were conducted on a BioRad iCycler iQ1 Real Time PCR machine, with SYBR-Green Supermix (Bio-Rad, Hercules, USA). Primer concentrations were optimized per primer pair and are stated in Table 1 and 2. Primer specificity was tested by examining the dissociation curve made with 1 °C temperature steps, and by running the PCR products on agarose gel. All PCR's used 58 °C as annealing temperature. Every sample was run with technical replicates, and the average was used for calculations with the ddCt method18 and geNorm.14 Expression values of NQO1, CLDN4, NOS2, and ABCB1 were normalized using the geometric average of the Cq values of RPLP0 and GAPDH.
Table 1
Primer sequences and characteristics of the reference genes testeda
Name |
RNA
ID
|
Sequence (5′-3′) |
Tm (°C) |
Am. (bp) |
AE (%) |
Conc. (μM) |
gDNA |
Ref. |
Abbreviations: RNA ID: NCBI mRNA identifier of the sequence used for designing primer. Tm: melting temperature, as calculated by Clone Manager software. Am: amplicon size expected from cDNA, in base pairs. AE: amplification efficiency. Conc.: concentration of primer in reaction mixture. gDNA: length of product expected to result from genomic DNA. Ref.: Source of primer sequence; N-newly designed by authors.
|
RPLP0
|
NM_001002.3 |
GCAATGTTGCCAGTGTCTG |
61 |
142 |
93.5 |
0.1 |
none |
16
|
GCCTTGACCTTTTCAGCAA |
60 |
|
|
|
|
|
B2M
|
NM_181861.1 |
TGCCGTGTGAACCATGTG |
62 |
92 |
89.8 |
0.1 |
none |
N |
GCGGCATCTTCAAACCTC |
60 |
|
|
|
|
|
ACTB
|
NM_001101 |
CTGGAACGGTGAAGGTGACA |
64 |
140 |
93.2 |
0.1 |
var. |
14
|
AAGGGACTTCCTGTAACAATGCA |
64 |
|
|
|
|
|
GAPDH
|
NM_02046
|
TGCACCACCAACTGCTTAGC |
65 |
87 |
96.5 |
0.1 |
87-3750 |
14
|
GGCATGGACTGTGGTCATGAG |
65 |
|
|
|
|
|
PSMC4
|
NM_006503.2 |
GGCATGGACATCCAGAAG |
59 |
190 |
97.3 |
0.1 |
5279 |
17
|
CCACGACCCGGATGAAT |
60 |
|
|
|
|
|
MRPL19 |
NM_005877.4 |
GGGATTTGCATTCAGAGATCAG |
61 |
182 |
96.2 |
0.4 |
2260 |
17
|
GGAAGGGCATCTCGTAAG |
58 |
|
|
|
|
|
PUM1
|
NM_005877.4 |
TGAGGTGTGCACCATGAAC |
62 |
187 |
98 |
0.4 |
3487 |
17
|
CAGAATGTGCTTGCCATAGG |
60 |
|
|
|
|
|
SF3A1
|
NM_005877.4 |
GGAGGATTCTGCACCTTCTAA |
61 |
196 |
101 |
0.4 |
6627 |
17
|
GCGGTAGTAGGCATGGTAA |
60 |
|
|
|
|
|
Table 2
Primer sequences and characteristics of the response genes used to verify effect of exposures to Caco-2 cellsa
Name |
RNA
ID
|
Sequence (5′-3′) |
Tm (°C) |
Am. (bp) |
RE (%) |
Conc. (μM) |
gDNA |
Ref. |
See Table 1 for explanation of abbreviations.
|
NQO1
|
NM_000903.2 |
GGGATCCACGGGGACATGAATG |
67 |
134 |
90.4 |
0.1 |
1880 |
19
|
ATTTGAATTCGGGCGTCTGCTG |
66 |
|
|
|
|
|
NOS2
|
NM_000625.3 |
CATCCTCTTTGCGACAGAGAC |
62 |
118 |
89.8 |
0.1 |
1408 |
N |
GCAGCTCAGCCTGTACTTATC |
62 |
|
|
|
|
|
CLDN4
|
NM_001305.3 |
TTGTCACCTCGCAGACCATC |
64 |
92 |
94.3 |
0.1 |
92 |
N |
CAGCGAGTCGTACACCTTG |
62 |
|
|
|
|
|
ABCB1
|
NM_000927.3 |
GCTCGTGCCCTTGTTAGAC |
62 |
96 |
98.2 |
0.1 |
1543 |
N |
CAGGGCTTCTTGGACAACC |
62 |
|
|
|
|
|
Statistical analysis
Differences in gene expression were analysed on Cq values, with univariate ANOVAs. Dunnett two-sided post-hoc testing was used to identify expression levels different from DMEM control at p < 0.05.
Results
Reference gene primer quality
Reference genes with corresponding primer sequences were selected from literature and were analyzed for their specificity and PCR efficiency (see Table 1). Primer sequences were taken from literature and tested for use at 58 °C annealing temperature to allow all analyses under similar PCR conditions. New primers were developed for B2M due to the formation of two products by the published sequences (data not sown). The primer pairs for the reference genes were expected to give products ranging from 89 to 196 bp, with melting temperatures between 58 and 65 °C. Impact of a potential contamination of the samples with genomic DNA was determined by running in silico PCRs against the human genome. Melting curves showed only one peak, and one band was observed for each primer pair when the qPCR products were run on agarose gel. PCR amplification efficiencies of all primer pairs were between 89.8 and 101.4%.
Reference gene selection
Homogenates of broccoli, apple, tomato, and mushroom were used for determining the best set of reference genes for normalizing qPCR data when analysing responses of Caco-2 cells to food products, with a 3 times dilution, and a 5 times dilution. Cq values of the eight tested reference genes are shown in Fig. 1. GAPDH is the gene with the highest gene abundance, resulting in the lowest Cq values (average 19.7), and PSMC4 had the highest Cq value (average 27.0). MRPL19 had the highest difference between maximum and minimum average Cq values (3.25), SF3A1 had the lowest difference between the extreme Cq values (1.25). Expression data was analyzed using geNorm,14 which calculates the stability of expression (M), and a measure for pairwise variation (V). Gene stability (M) is calculated by determining the ratios of the expression of the indicated gene with all the other genes, and the average standard deviation of the logarithmically transformed ratios is computed. Good reference genes are expected to have a constant expression, so the ratio of the expression of two good reference genes is expected to be constant. The variation of the ratios of these two genes over treatments will therefore be low. By repetitively removing the worst performing gene from the gene pool, and recalculating M, the best performing, least variable pair of genes can be selected. Fig. 2 shows the gene stability measure M for the studied reference genes, using the cyclic approach described. PSMC4 had the highest variation in expression compared to the other genes. Expression of RPLP0 and GAPDH were most similar to each other (Fig. 2).
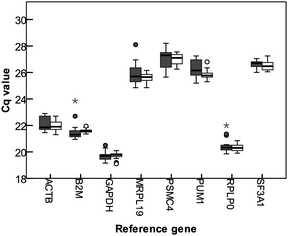 |
| Fig. 1 Box plot showing the quantification cycles (Cq) of tested reference genes in Caco-2 cells upon exposure to different food products homogenates. The boxes represent the median with the 25 and 75 percentiles, the whiskers indicate the 5 and 95 percentiles, outliers are represented by dots, extreme outliers (more than three times the height of the box) are indicated with an asterisk. Dark boxes represent the Cq values for 3 times diluted samples, open boxes represent the Cq values for 5 times diluted samples. | |
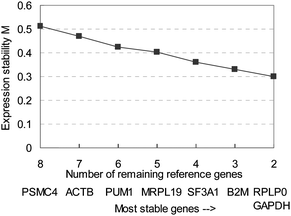 |
| Fig. 2 Average expression stability (M) of tested reference genes, with stepwise exclusion of the least stable gene. The least stable gene in each group is indicated below the axis. The genes are thus ranked in order of increasing stability of expression. | |
Using multiple reference genes for normalization gives a more accurate normalization factor, which is calculated as the geometric mean of the Cq values. A method to determine the number of reference genes to be used is based on how much the normalization value changes by adding an extra (next best) gene to the set. Comparing a reference group of 3 genes (RPLP0, GAPDH, and B2M) with a group of 2 genes (GAPDH and RPLP0) showed a difference in the corresponding normalization factor that resulted in a standard deviation of 0.11 (Fig. 3), a value that is within the same range as has been found for other validated sets of reference genes.14,15 Expression of response genes will be normalized using GAPDH and RPLP0 as reference genes. Addition of extra genes to the set results in more stable normalization factors, as indicated by the descending line in Fig. 3, with the least variation between a set consisting of five genes (RPLP0, GAPDH, B2M,SF3A, and MRPL19) compared with a set of six genes. This set might be used in larger experiments such as RT-qPCR-arrays.
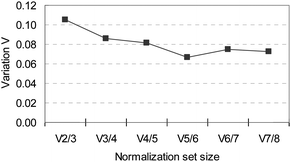 |
| Fig. 3 Variation in normalization factor when different number of genes are included, comparing the normalization factor composed of n genes with the normalization composed of n + 1 (next best gene). | |
Effect of exposure
Primers were designed for genes which expression was be expected to change in Caco-2 cells upon exposure to different foods (see Table 2). Similar quality parameters were met as used for the primes of the reference genes (see above). Altered expression of these genes served as a positive control to show that the cells did indeed react to the different food homogenates. Genes were selected based on reported responsiveness to individual bioactive compounds, since data on whole products is scarce. NAD(P)H Quinone dehydrogenase 1 (NQO1) was selected to be responsive for broccoli as it is generally accepted that broccoli contains glucoraphanin, a glucosinolate that is converted to sulforaphane, which in turn induces NQO1 gene expression.1,20CLDN4 was selected to be responsive to exposure to apple, since apples contain quercetin-glycosides, a phytochemical that has been shown to induce CLDN4 expression.2,21Nitric Oxide Synthase 2 (NOS2), and ATP-binding cassette, subfamily B member 1 (ABCB1) were selected as general responsive genes, involved in nitric oxide signalling and xenobiotic transport.
Exposure of Caco-2 cells to broccoli extract induced an 8-fold increase in NQO1 mRNA abundance compared with medium only (Fig. 4A). Mushroom induced a 2-fold increase in NQO1 expression, with a lower induction at higher dilution. Different dilutions of broccoli did not result in different induction. Exposure to apple and tomato did not result in a changed NQO1 expression indicating that changes in gene expression are not a general exposure response but specific for a food product. CLDN4 expression in Caco-2 cells was enhanced by all food products tested except tomato, with the highest induction by apple and broccoli (Fig. 4B). A dose dependent induction of CLDN4 was observed for apple and mushroom. Expression of NOS2 was enhanced by apple and mushroom in a dose dependent manner, and was reduced by the highest ‘concentration’ of broccoli (Fig. 4C). Expression of ABCB1 in Caco-2 cells was induced by broccoli and mushroom (Fig. 4D).
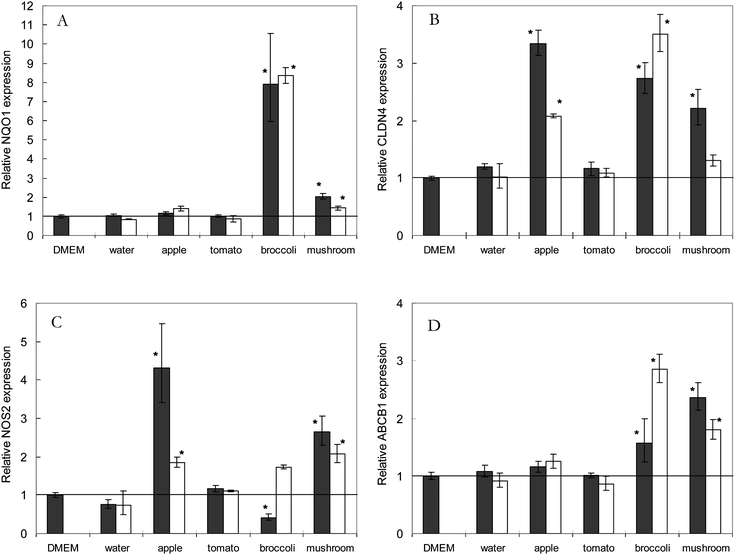 |
| Fig. 4 Relative gene expression of A. NAD(P)H Quinone dehydrogenase 1 (NQO1), B. Claudin 4 (CLDN4), C. Nitric Oxide Synthase 2 (NOS2), and D. ATP-binding cassette, sub-family B, member 1 (ABCB1), in Caco-2 cells upon exposure to different fruit and vegetables homogenates. Expression levels in cells exposed to medium alone (Dulbecco's modified eagle medium, DMEM) was set as 1. Solid bars indicate the relative gene expression of 3 times diluted samples, open bars represent the relative gene expression of 5 times diluted samples. Values mean ± standard error, n = 3. Values that are significantly different from the DMEM control are indicated with *, for p < 0.05. | |
Cell viability was tested in a separate experiment for the 3 times diluted samples, and did not change upon exposure to food homogenates, except for broccoli which induced a decrease in MTT conversion (data not shown). However, total amount of RNA extracted from broccoli exposed cells was similar to the control samples, and reference genes showed exact the same Cq values as for other exposures, arguing that no severe induction of cell death could be held responsible for all effects seen for broccoli.
Discussion
Reference gene selection
Eight putative reference genes were selected from literature and tested for their suitability to be used in systematic Caco-2 exposure studies, including the widely used ACTB and GAPDH. All primers developed and used in this study were specific as they amplified only a single fragment and the PCR efficiency was close to 100%. The expression of 18S was not considered in this study, since that gene is much more abundant than most response genes and the Cq value will not be in the same range. Samples will thus need extra dilution which might be a source of variation. RPLP0 and GAPDH were selected as the best reference couple, using the method as described by VanderSompele et al.14 Since the method is based on finding genes with the best co-expression, care has to be taken not to end up with a gene-set that is part of one biological signalling pathway or that their expression is regulated by a common factor. RPLP0 is a ribosomal protein, and as such involved in protein synthesis.22GAPDH is part of the glycolysis pathway, another major cellular process, and not likely to be closely regulated with RPLP0. Expression patterns of both genes are similar to the other tested reference genes, making it unlikely that this set of reference genes is selected solely based on co-regulation. GAPDH and RPLP0 can be used as reference genes when studying a small number of genes of interest. When larger gene sets are analysed, one might consider to include SF3A1, B2M and MEPL19. Exposure to broccoli might have resulted in toxic stress, but even so, this did not result in large difference in Cq values of the reference genes (Fig. 1). The tested selected normalization genes might therefore also be candidates for conditions where adverse effects are expected. Preliminary results show that RPLP0 and GAPDH can be used as stable reference genes in exposure studies with in vitro digested homogenates and Caco-2 cells grown in transwells as well (data not shown).
Effect of exposure
The 3 and 5 times diluted homogenate of fresh broccoli exposed to the Caco-2 cells induced an 8-fold increase in NQO1 mRNA abundance (Fig. 4A). The lack of concentration dependent response might be indicative that the NQO1 induction has reached a plateau already at the 5 times dilution. Besides exposure to broccoli, exposure to button mushroom extract induced an increase in NQO1 mRNA abundance as well, showing that induction of the phase II detoxification system might be induced by more food products than those in the group of brassica vegetables. Broccoli and button mushroom incited an increased expression of CLDN4 as well, together with apple (Fig. 4B). CLDN4 is a gene coding for a protein that is part of the tight junction complex between cells23 and its expression is known to be induced by polyphenols such as quercetin.21 To our knowledge, this is the first time that it is shown that a food homogenate, containing all phytochemicals at diluted levels and in combination with the food matrix, can up-regulate CLDN4 expression, and that intake of certain food products might regulate the tight junction or barrier function of the gut epithelium like suggested for quercetin.21 Since we aim at studying bioactive effects of whole food products, no attempts have been made at this stage to correlate these cell responses to concentrations of a certain phytochemicals. The responsiveness of Caco-2 cells to the fruit and vegetable homogenates implies that active compounds are present in high enough concentrations to exert an effect, and that the stability in expression of the reference genes is not due to a lack of response of the Caco-2 cells.
Induction of gene expression by apple and mushroom is higher for the 3 times diluted sample than for the 5 times diluted samples, which would be expected for a concentration dependent effect. Induction of genes by broccoli showed a reverse effect on the expression of CLDN4 and ABCB1. These contradicting effects can be explained by taking into account the effect of broccoli homogenates on cell viability, since a 3 times diluted homogenate resulted in a reduced MTT reduction. The higher induction of certain genes with a more diluted sample might be the result of an interplay of product-induced effects, and toxicity-induced effects, of which the latter will be more alleviated at lower concentrations. Exposure of cells to tomato homogenates did not incite a response for any of the tested genes. As only a very limited set of response genes have been studied here, it is not unlikely that crude tomato homogenate can regulates other functional genes in Caco-2 cells. This study shows, however, that whole food homogenates can be used to study effect on gene expression level, but that enough marker genes should be included to be able to analyze the full responses. We would like to state at this point it that a single cell model will never result in a complete bioactivity signature for a food product since different products might effect different organs.
Applications
A good set of reference genes has been selected to be used for normalizing qPCR gene expression analysis in Caco-2 cells upon exposure to food products. The set of response genes, as shown in this paper, can be used for quantifying the effect upon exposure to food homogenates for a very limited number of cellular processes of the small intestinal cells. The set of response genes can be extended to develop a RT-qPCR-array, having indicator genes for many physiological functions that are relevant for intestinal cells, like barrier function, uptake and transporters of food compounds, immune function, contraction, cell proliferation and apoptosis and many more. RT-qPCR analysis allowed the detection of small differences in expression, which might be indicative of a physiological effect induced by a food product. When the expression of multiple genes is quantified upon exposure of Caco-2 cells to homogenates of different food products, a so called food signature can be obtained. Such a signature has already been presented for different small drug based compounds,24 which allowed linkage of gene expression signatures of cultured cells upon exposure to small compounds to the mode of action of tested small drug molecules and diseases. A similar strategy using fruit and vegetables will result in a database with gene expression signatures obtained from Caco-2 cells exposed to a variety of food products. Such an approach might detect similar or different biological effects of food products allowing the classification of products in bioactivity classes. This can facilitate product choice in human or animal intervention studies.
Abbreviations
ACTB
|
β-actin
|
B2M
| β-2-microglobulin |
CLDN4
| claudin 4 |
Cq
| quantification cycle |
DMEM | Dubelco's Modified Eagles Medium |
GAPDH
|
glyceraldehyde-3- phosphate dehydrogenase |
ABCB1
|
ATP-binding cassette, sub-family B, member 1 |
MRPL19
| mitochondrial ribosomal protein L19 |
NQO1
|
NAD(P)H quinone dehydrogenase 1 |
NOS2
|
nitric oxide synthase 2 |
PSMC4
| proteasome (prosome, macropain) 26S subunit, ATPase4 |
PUM1
| pumilio homologue 1 |
RT-qPCR
| quantitative reverse transcriptase polymerase chain reaction |
RPLP0
| ribosomal phosphoprotein P0 |
SF3A1
| splicing factor 3A, subunit 1. |
Acknowledgements
The authors declare that there is no conflict of interest. This work was supported by the Wageningen University and Research Centre IPOP systems biology program.
Literature cited
- J. W. Fahey, Y. Zhang and P. Talalay, Proc. Natl. Acad. Sci. U. S. A., 1997, 94, 10367–10372 CrossRef CAS.
- J. Boyer and R. H. Liu, Nutr. J., 2004, 3, 5 CrossRef.
- J. Boyer, D. Brown and R. H. Liu, Nutr. J., 2005, 4, 1 CrossRef.
- J. Parada and J. M. Aguilera, J. Food Sci., 2007, 72, R21–R32 CrossRef CAS.
- M. Shimizu, Biosci., Biotechnol., Biochem., 2010, 74, 232–241 CrossRef CAS.
- R. P. Glahn, O. A. Lee, A. Yeung, M. I. Goldman and D. D. Miller, J Nutr., 1998, 128, 1555–1561 CAS.
- J. Fogh, J. M. Fogh and T. Orfeo, J Natl Cancer Inst., 1977, 59, 221–225 CAS.
- M. Pinto, S. Robine-Leon, M. D. Appay, M. Kedinger, N. Triadou, E. Dussaulx, B. Lacroix, P. Simon-Assmann, K. Hafen, J. Fogh and A. Zweibaum, Biol Cell., 1983, 47, 323–330.
- A. Zweibaum, H. P. Hauri, E. Sterchi, I. Chantret, K. Haffen, J. Bamat and B. Sordat, Int. J. Cancer, 1984, 34, 591–598 CrossRef CAS.
- M. Traka, A. V. Gasper, J. A. Smith, C. J. Hawkey, Y. Bao and R. F. Mithen, J Nutr., 2005, 135, 1865–1872 CAS.
- A. A. Dihal, C. Tilburgs, M. J. van Erk, I. M. C. M. Rietjens, R. A. Woutersen and R. H. Stierum, Mol. Nutr. Food Res., 2007, 51, 1031–1045 CrossRef CAS.
- T. Mandimika, H. Baykus, Y. Vissers, P. Jeurink, J. Poortman, C. Garza, H. Kuiper and A. Peijnenburg, J. Agric. Food Chem., 2007, 55, 10055–10066 CrossRef CAS.
- S. A. Bustin, V. Benes, J. A. Garson, J. Hellemans, J. Huggett, M. Kubista, R. Mueller, T. Nolan, M. W. Pfaffl, G. L. Shipley, J. Vandesompelel and C. T. Wittwer, Clin. Chem., 2009, 55, 4.
-
J. Vandesompele, K. De Preter, F. Pattyn, B. Poppe, N. Van Roy, A. De Paepe and F. Speleman, GenomeBiology, 2002, 3, R34.1–34.11 Search PubMed.
- C. Piana, M. Wirth, S. Gerbes, H. Vierstein, F. Gabor and S. Toegel, Eur. J. Pharm. Biopharm., 2008, 69, 1187–1192 CrossRef CAS.
- A. B. Dydensborg, E. Herring, J. Auclair, E. Tremblay and J. F. Beaulieu, Am. J. Physiol.: Gastrointest. Liver Physiol., 2006, 290, 1067–1074.
- A. Szabo, C. M. Perou, M. Karaca, L. Perreard, J. F. Quackenbush and P. S. Bernard, GenomeBiology, 2004, 5, R59 CrossRef.
- K. J. Livak and T. D. Schmittgen, Methods, 2001, 25, 402–408 CrossRef CAS.
- F. Pattyn, F. Speleman, A. De Paepe and J. Vandesompele, Nucleic Acids Res., 2003, 31, 122–123 CrossRef CAS.
- T. A. Shapiro, J. W. Fahey, K. L. Wade, K. K. Stephenson and P. Talalay, Cancer Epidemiol. Biomark. Prev., 2003, 7, 1091–1100 Search PubMed.
- M. Amasheh, S. Schlichter, S. Amasheh, J. Mankertz, M. Zeitz, M. Fromm and J. D. Schulzke, J Nutr., 2008, 138, 1067–1073 CAS.
- M. Tchórzewski, D. Krokowski, W. Rzeski, O. G. Issingen and N. Grankowski, Int. J. Biochem. Cell Biol., 2003, 35, 203–211 CrossRef CAS.
- C. M. Van Itallie and J. M. Anderson, Annu. Rev. Physiol., 2006, 68, 403–429 CrossRef CAS.
- J. Lamb, E. D. Crawford, C. Peck, J. W. Modell, I. C. Blat, M. J. Wrobel, J. Lerner, J. P. Brunet, A. Subramanian, K. N. Ross, M. Reich, H. Hieronymus, G. Wei, S. A. Armstrong, S. J. Haggarty, P. A. Clemons, R. Wei, S. A. Carr, E. S. Lander and T. R. Golub, Science, 2006, 313, 1329–1935.
|
This journal is © The Royal Society of Chemistry 2011 |