Grafting thermally labile molecules on cross-linkable polyimide to design membrane materials for natural gas purification and CO2 capture
Received
19th July 2010
, Accepted 10th September 2010
First published on 20th October 2010
Abstract
A novel strategy to design molecularly the cavity size and free volume of flexible polyimide materials via thermal treatment of rigid and cross-linkable polyimides grafted with thermal liable side beta-cyclodextrin (CD) molecules is demonstrated in this study. The spaces occupied by the labile groups may become microvoids after low-temperature thermal degradation while the rigid polyimide backbone prevails. The thermal induced cross-linking reaction among polyimide chains may create ultra-fine micro-pores that integrally connect with microvoids. As a result, the thermally cured membranes fabricated from dense polyimide precursors show gas separation performance surpassing the trade-off lines, with tough and flexible mechanical properties. Thermal annealing at 425 °C produces polyimide membranes with the best CO2 permeability of 4016 Barrer with reasonable gas pair selectivity.
Broader context
The advantages of membrane technology have been recognized as potentially higher energy efficiency, ease scale-up, small footprint and environmental friendliness. There are many criteria for the selection of membrane materials, the most important ones are high permeation flux and gas pair selectivity. Extensive research to tailor polyimide chemical structures for better gas separation performance has been carried out in the last two decades, but faces a limit as indicated in the trade-off curve describing the relationship between gas permeability and selectivity. In the present study, cyclodextrin, a thermally labile molecule with a large dimensions, was grafted on a high-temperature, rigid, but cross-linkable polyimide with carboxylic acid pendant groups, by esterification. The polyimide membranes with grafted cyclodextrin were subjected to thermal treatment from 300 to 450 °C to decompose the cyclodextrin structure, leaving behind a high pore volume in the membrane matrix of preserved polyimide backbones. Meanwhile, the polyimide containing carboxylic groups undergoes esterification and/or decarboxylation induced cross-linking reactions at high temperatures. As a result, the thermally cured membranes fabricated from dense polyimide precursors show gas separation performance surpassing the trade-off lines, with tough and flexible mechanical properties.
|
1. Introduction
Cryogenic purification, pressure swing adsorption and membrane technology are the technologies used for gas separation.1,2 The advantages of membrane technology have been recognized as potentially higher energy efficiency, ease of scale-up, small footprint and environmental friendliness.3 In particular, from the economic point of view, membrane technology may appear more advantageous for small-to-medium scale separations and non-stringent gas purity requirements, and integrated with other separation methods.4 There are many criteria for the selection of membrane materials, the most important ones are high permeation flux and gas pair selectivity.5–7 Other factors may include mechanical and thermal stability, manufacturing reproducibility, tolerance to contaminants and economical aspects. Among many polymeric materials, aromatic polyimides consisting of rigid chains and containing bulky and sterically-hindered structures are considered to have great potential as emerging membrane materials.8–12 Extensive research to tailor polyimide chemical structures for better gas separation performance has been carried out in the last two decades. However, structural design attempts to further improve performance have apparently approached a limit as indicated in the trade-off curve describing the relationship between gas permeability and selectivity.13–16 In addition, no major breakthroughs in the improvement of plasticization resistance have been achieved on polyimide membranes through backbone chemical modification or introduction of various side groups.
Polyimide modification by cross-linking has received much attention since the resultant membranes show improved selectivity, chemical and plasticization resistance. Cross-linking modifications of polyimides can be induced by several methods, such as ultraviolet (UV) irradiation of benzophenone-containing polyimides,17,18 thermal treatment of polyimides containing acetylene or carboxyl groups,19,20 or cross-linking polyimide chains by small molecules with multiple reactive groups.21–28 The cross-linking modification tends to increase chain packing and inhibits the intra-segmental and inter-segmental mobility, resulting in higher gas selectivity, but sacrificing gas permeability tremendously. Another attempting to enhance polyimide performance is using aromatic polyimides as polymeric precursors for the preparation of carbon molecular sieve membranes under high temperature pyrolysis, which cause the polyimide chain degradation to form a graphite-like structure.29–32 The gas separation factor of carbonized membranes surpasses the upper bound of the trade-off curve for conventional polymer membranes, since the gas transport in the membrane switches from solution-diffusion to a primarily molecular sieving mechanism.33 In addition, carbonized membranes have higher tolerance to harsh environments due to the sluggish chemical reactivity and better thermal resistance. However, their inherent brittleness restricts their practical application.
Recently, high free volume polyimides have been achieved by incorporating the concept of “polymers of intrinsic microporosity” (PIMs) by using a dianhydride with a spiro-center and reacting with a range of diamines.34 The resulted PIMs exhibit very high gas permeability. Prof. Lee and his coworkers suggested the systematic tailoring of an unusual microstructure via a thermally-driven “molecular rearrangement” process.35–37 In this process, the single bond linkages of the polyimide are changed to stiff and rigid chain elements (e.g.benzoxazole-phenylene ring or benzithiazole-phenylene ring). Additionally, the indiscriminant torsional rotation increases the efficiency of free volume formation. Consequently, the gas separation performance of such membranes can surpass the upper bound.
Approaches to prepare polyimide films with high pore volumes include microwave processing,38 ion irradiation,39 incorporation of foaming agents40 and thermal decomposition of labile components in block or graft copolymers.41,42 Those reported polyimide films all exhibit ultra-low-k properties due to their highly porous structure. However, their pore sizes ranging from the nanometre to micrometre scales are too big to be used for gas separation. Islam et al. proposed a low-temperature pyrolysis method to fabricate flexible organic–inorganic intermediate membranes by the cleavage of SO3H groups in the sulfonated polyimides.43 The resultant membranes possess attractive gas separation performance with high degrees of flexibility because the spaces occupied by the labile groups might be kept as voids after the pyrolysis while the polyimide backbone still prevails. In the present study, cyclodextrin, a thermal labile molecule with large dimensions,44 was grafted on a rigid, but cross-linkable polyimide with carboxylic acid pendant groups, by esterification. The polyimide membranes with grafted cyclodextrin were subjected to thermal treatment from 300 to 450 °C to decompose the cyclodextrin structure, leaving behind a high pore volume in the membrane matrix of the preserved polyimide backbones. Meanwhile, the polyimide containing carboxylic groups undergoes esterification and/or decarboxylation induced cross-linking reactions at high temperatures.45 The resultant cross-linked structure provides the polyimide with a very rigid chain structure that prevents pores from collapsing. The evolution scheme from copolyimide, grafted copolyimide to thermally cured grafted copolyimides is shown in Fig. 1. The effects of thermal treatment temperature on gas separation properties of copolyimides containing labile cyclodextrin groups have been investigated. To our best knowledge, this is the first paper combining both thermal labile molecules as the pore forming agent and a thermal cross-linkable polyimide to molecularly design novel membrane materials with much enhanced gas separation performance and better physical/chemical stability.
 |
| Fig. 1 Evolution scheme from copolyimide, CD grafted copolyimide to thermally cured CD grafted copolyimides. | |
2. Experimental
2.1 Reagents
2,2′-Bis(3,4′-dicarboxyphenyl) hexafluoropropane dianhydride (6FDA), supplied by Clariant, and 3,5-diaminobenzoic acid (DABA) supplied by Aldrich were purified by vacuum sublimation before usage. 2,3,5,6-Tetramethyl-1,4-phenylenediamine (durene diamine) supplied by Aldrich, was recrystallized from methanol. NMP (N-methyl-pyrrolidone) purchased from Merck, was distilled at 42 °C under 1 mbar after drying with molecular sieve before use. Other chemicals and solvents such as acetic anhydride, triethylamine, methanol and beta-cyclodextrin were all reagent grade or better from Aldrich and were used without further purification. The purities of all tested pure gases were 99.99%.
2.2 Co-polyimide synthesis and cyclodextrin grafting
Chemical imidization was performed during the synthesis of polyimide. A stoichiometric amount of 6FDA was added to a mixture NMP solution consisting of durene and DABA diamine at a designated molar ratio in a moisture free flask with stirring and nitrogen inlet at room temperature. After reaction for 24 h, a high molecular weight polyamic acid was formed, and then a mixture of acetic anhydride and triethylamine at 4
:
1 molar ratio were slowly added to the polyamic acid solution to induce imidization for 24 h. After precipitation in methanol, the polymer was filtered, washed and dried under 150 °C in vacuum for 24 h. Then the copolyimide was dissolved in NMP with a large excess of beta-cyclodextrin (CD) and catalytic amount of p-toluenesulfonic acid. The esterification was carried out by heating to 120 °C and bubbling with dry nitrogen gas. The resultant polyimide-g-CD product was precipitated, washed and dried.
2.3 Dense membrane preparation
The copolyimide and polyimide-g-CD were prepared as flat dense films with a weight ratio of 1
:
1. 2% (w/w) polymer solutions were prepared by dissolving the readily-soluble polyimide powders in dichloromethane. The polymer solutions were filtered using Whatman's filters (1 μm) to remove insoluble materials and dust particles. Then the solutions were cast onto silicon wafers at ambient temperature. Dense membranes were formed after most of the solvent was allowed to evaporate slowly over a period of about 5 days. The residual solvent was removed by placing the dense membranes in a vacuum oven at 200 °C. All membranes were cut into circles with a diameter of 38 mm. Only the membranes with a thickness of about 50 ± 5 μm were used in the following studies.
2.4 Thermal treatments
The thermal cure was performed using a Centurion™ Neytech Qex vacuum furnace. During thermal curing, the temperature was increased to a set point of 300, 350, 400, 425 and 450 °C at a rate of 10 °C min−1 and held there for 2 h. After completing the thermal treatment process, membranes were cooled naturally in the vacuum furnace to room temperature and stored in a dry box for further studies. All membranes after thermal treatment were flexible and tough enough for the gas permeation measurements. To simplify the sample identification, the cured samples were named as ‘polyimide-curing temperature’, for example PI-g-CD-300.
2.5 Characterizations
The weight loss of the polyimide during post thermal treatment was characterized by thermogravimetric analysis (TGA) with a TGA 2050 Themogravimetric Analyzer (TA Instruments). The analysis was carried out with a ramp of 10 °C min−1 in the temperature range from 50 to 900 °C. The purge gas was N2 and its flow rate was controlled at 50 ml min−1.
The chemical bonds in the polymers were investigated by Fourier Transform Infrared Spectroscopy. The FTIR measurements were performed using an attenuated total reflection mode (FTIR-ATR) with a Perkin-Elmer Spectrum 2000 FTIR spectrometer.
Wide-angle X-ray diffraction (WAXD) was performed to quantitatively measure the interchain spacing of membrane materials with a Bruker X-ray diffractometer (Bruker D8 advanced diffractometer) at room temperature. The d-spacing values are interpreted as the average chain spacing. The measurement was completed in a scan range of 2θ = 2.5 to 65.4° with a step increment of 0.02°. Ni-filtered Cu-Kα radiation with a wavelength of λ = 1.5418 Å was used in the experiments. The average d-spacing was determined based on the Bragg's law.
where
d is the dimension spacing,
ϑ is the diffraction angle,
λ is the X-ray wavelength and
n is an integral number (1, 2, 3…).
Positron annihilation lifetime experiments were performed using a high intense slow positron beam with monoenergy at 5 keV. The resolution of the positron annihilation lifetime spectrometer was determined by measuring 60Co and each PAL spectrum contains 2 million counts. The obtained PAL data were fitted into three lifetime components using the PATFIT program which assumes a Gaussian distribution of the logarithm of the lifetime for each component, and also into continuous lifetime distribution using the MELT program.
2.6 Gas permeation measurements
Pure gas permeabilities were obtained by a constant volume method at 35 °C and 10 atm. The film was mounted onto the permeation cell and vacuumed at 35 °C for more than 24 h before the gas permeation test was carried out. To investigate the CO2-induced plasticization behavior of the films, the testing pressure was intermittently ramped from 2 to 30 atm. The rate of pressure increase (dp/dt) at steady state was used for the calculation of gas permeability. The permeability of each gas was obtained from the average value of at least 3 tests with differences among them smaller than 1%. The ideal selectivity is defined as follows:
where PA and PB are the permeability of gases A and B, respectively.
Binary CO2–CH4 (1
:
1) gas permeation tests were conducted by a modified constant volume permeation system in which an addition valve at the upstream segment is included to adjust the stage cut and another valve at the downstream port is installed to introduce the accumulated permeate gas to an Agilent 7890 gas chromatography (GC) for the analysis of gas composition. For easy comparison with pure gas tests, the testing pressure of mix gas permeation tests is 20 atm.
3. Results and discussion
3.1 Characterizations
Fig. 2 shows the TGA curve and the simultaneous IR spectra of evolved gases of PI-g-CD membranes during pyrolysis. In Fig. 2, the X axis represents the thermal treatment temperature and the Y axis consists of the weight percentage for TGA and the wave numbers for FTIR spectra. Three stages of decomposition are observed. In the first stage, the around 10% weight loss occurring in the range of 200–400 °C is mainly due to the release of compounds with IR adsorption at 1700 cm−1, which may be characteristic bands of aldehydes, ketones or organic acids. This investigation is consistent with other reports about the decomposition of cyclodextrin structure.44 In addition, no other IR peaks are detected during this temperature range. Therefore, it could be concluded that only the CD structure decomposition contributes to the weight loss of the polyimide during 200–400 °C thermal treatment. The second weight loss stage of around 5% is observed from 400–450 °C. This temperature corresponds to the evolution of CO2, which shows a characteristic IR peak at 2350 cm−1. The release of CO2 mainly comes from the decarboxylation of the DABA moiety of the copolyimide.45,46 On the other hand, the IR spectrum of evolved gases exhibit a peak starting at 1149 cm−1 which is indicative of CFx evolution when temperature is increased to 450 °C. In the last stage, a further 30% weight loss occurs in the range of around 500–700 °C accompanied by significant evolution of H2O (characteristic peaks at 3700–3900 cm−1), CO2 (characteristic peak at 2350 cm−1), CO (characteristic peaks at 2170 and 2110 cm−1), C–N (characteristic peak at 1360 cm−1), CFx (characteristic peak at 1149 cm−1) and C–O (characteristic peak at 1050 cm−1). The released gases arise mainly from the imide and 6FDA groups, which construct polymer backbones.
The evolution of chemical changes of CD grafted polyimides during pyrolysis was monitored by ATR-FTIR, as shown in Fig. 3. Compared with the original copolyimide, the polyimide film grafted by CD shows a stronger intensity of the characteristic peaks of OH groups of CD at 1620 and 3650 cm−1, indicating the successful esterification between polyimide and the CD structure. The intensities of these CD characteristic perks decrease with increasing thermal treatment temperature to 400 °C, consistent with the finding of TGA that CD structure is decomposed during thermal treatment. The intensities of the peaks assigned to a symmetric C
O stretch (1776 cm−1), asymmetric C
O stretch (1720 cm−1), C–N stretch (1353 cm−1), and bending of C
O (741 cm−1) are constant until the membranes are thermally treated at 400 °C. This result demonstrates that the imide groups of polyimide are stable until 400 °C and the fact that the decomposition of CD structure may not have a significant influence on the imide groups in the polyimide when the thermal treatment temperature is below 400 °C. Once a thermal curing temperature of 425 °C is attained, the intensities of peaks at 1600–1580 cm−1, representing C–C stretching vibrations in the aromatic ring, are increased. This may be explained by the formation of new covalent bonds between benzene rings. According to TGA results, decarboxylation occurs and form radical DABA groups when thermal temperature increases over 400 °C. It may be possible for two radical DABA groups to form linkages with the corresponding biphenyl.46 For the membranes cured at 450 °C, the absorbance of imide groups is reduced, since the imide ring begins to decompose at this temperature. On the other hand, the absorption bands assigned to aromatic C–C stretching vibrations increase, indicating the stronger cross-linking reaction between aromatic rings.
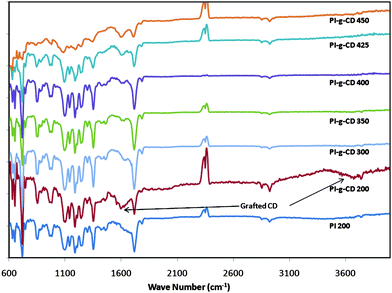 |
| Fig. 3
FTIR-ATR
spectra of copolyimide, CD grafted copolyimide and thermally cured CD grafted copolyimides. | |
Wide angle X-ray diffraction patterns of these polyimides are illustrated in Fig. 4. They are all broad, indicating that they are all amorphous structure. The most prominent WAXD peak in the amorphous glassy polymer spectrum is often used to estimate the average interchain spacing distance (d-spacing). These values were calculated using the Bragg equation and are notated in Fig. 4. There is an obvious trend of increasing d-spacing with increasing thermal treatment temperature to 400 °C. This can readily be explained by the decomposition of CD structure, by which a lot of voids and channels remain between polyimide chains. On the other hand, it shows a surprising decrease in d-spacing after increasing the heat treatment temperature to 425 °C. This confirms that the polymer chains undergo cross-linking reactions at this high temperature, by which consequently promotes chain packing. Interestingly, following a further increase in thermal treatment temperature to 450 °C, the peak moves to in the larger theta angle direction. It is amply evident that an ordered structure is gradually constructed in the matrix and the distance between chains becomes smaller during the curing process. The peak position is near 20°, which corresponds to a d-spacing of 4.3 Å. Although this value is greater than interlayer spacing, d002, of graphite crystal,47 it can still be attributed to the average spacing between neighboring two hexagonal layers, indicating the membrane matrix may transfer to an intermediate stage between polymer and carbon structure.
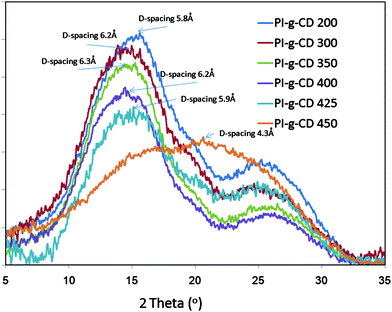 |
| Fig. 4
WAXD
spectra of copolyimide, CD grafted copolyimide and thermally cured CD grafted copolyimides. | |
3.2 Gas permeability and selectivity
Table 1 summarizes the gas permeabilities (P) of pure O2, N2, CH4, CO2, C3H6 and C3H8 through original grafted polyimide and thermally cured polyimides. The permeability of thermally cured polyimides monotonically increases with thermal treatment temperature. In particular, the permeability of PI-g-CD 450 is around 200 times higher than the original PI-g-CD 200. This increase in gas permeability with the aid of thermal treatment is mainly attributed to the decomposition of CD structure. As mentioned before, the membranes lost CD at the temperature range from 300 to 400 °C and the spaces occupied by CD might be kept as micro-pores after thermal treatment if the polymer is sufficiently rigid. Consistent with WXAD results where the d-spacing of polymer chains increases with temperature, the micro-pores are attributable to the significant enhancement in gas permeability. However, the gas permeability continuously increases when thermal treatment temperature is over 400 °C even though the WXAD spectra show a better packing behavior of polymer chains.
Table 1 Pure gas permeabilities and selectivities of original and thermally cured CD grafted copolyimides
Membranes
|
Permeability/Barrera |
Permoselectivity |
O2 |
N2 |
CH4 |
CO2 |
C3H6 |
C3H8 |
O2/N2 |
CO2/CH4 |
C3H6/C3H8 |
Barrer = 10−10 cm3 (STP) cm cm−2s−1 cm Hg−1.
|
PI-g-CD 200 |
11.5 |
2.6 |
1.8 |
56 |
— |
— |
4.4 |
31 |
— |
PI-g-CD 300 |
56 |
13.6 |
8.4 |
189 |
— |
— |
4.1 |
23 |
— |
PI-g-CD 350 |
102 |
26.6 |
18 |
357 |
15.7 |
1.3 |
3.8 |
20 |
12 |
PI-g-CD 400 |
262 |
78 |
58 |
921 |
67 |
6.3 |
3.4 |
16 |
11 |
PI-g-CD 425 |
1399 |
377 |
247 |
4016 |
635 |
45.3 |
3.7 |
16 |
14 |
PI-g-CD 450 |
2707 |
523 |
463 |
8000 |
472 |
26.2 |
5.2 |
17 |
18 |
In order to have a clearer picture of the pore structure, free volume distributions of original and thermally cured PI-g-CD membranes could be probed using PALS.48,49 The PAL data fitting curves are shown in Fig. 5. The peak between 2 and 3.5 ns is attributed to the o-Ps annihilation (τ3) in the amorphous region of the polymeric films.50 Thus τ3 is a useful parameter for the determination of average cavity size in the polymer. The PALS analysis reveals an increase in o-Ps lifetime as the temperature increases from 200 to 425 °C. In generally, a longer o-PS lifetime indicates a larger cavity size. The mean cavity radius can be calculated using a semi-empirical equation based on a spherical infinite potential well model as shown by the following equation:
where Δ
r is an empirical constant (1.656 Å) obtained by fitting well-defined cavities with known dimensions. In other words, the cavity radius of polyimide, which is centered at about 3.4 Å, increases to 3.8 Å, and the intensity also increases as the thermal treatment temperature increases to 425 °C. A larger cavity will definitely contribute to a higher rate of gas transport.
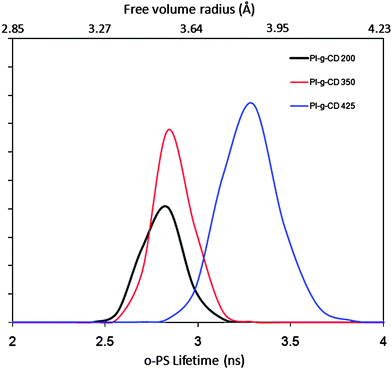 |
| Fig. 5
PAL
spectra of original and thermally cured CD grafted copolyimides. | |
It is noticed that the gas selectivity does not continuously decrease with an increase in permeability. It increases when the thermal treatment temperature increases to 425 °C, especially for the C3H6/C3H8 pair, in which the selectivity increases by 30% compared with that cured at 400 °C. This phenomenon is in agreement with the WAXD results, indicating that polymer chains undergo cross-linking reactions at 425 °C and consequently promotes chain packing. Therefore, it is possible that two kinds of pores; namely, ultra-fine micro-pores and micro-pores, are formed in the polymer membrane cured at high temperatures. The dimensions of the ultra-fine micro-pores are close to the d-spacing of the polyimide precursor (5–6 Å), while the size of micro-pores is larger than 7 Å because they are formed by the decomposition of thermally reliable groups. The micro-pores are integrally connected by the ultra-fine micro-pores, as a dumbbell shape, but the dimensions and shape of the ultra-fine micro-pores determine gas selectivity. Since the dimensions of the ultra-fine micro-pores decreases when polymer chains are cross-linked at higher temperatures, their neck openings are able to discern the gas pair sizes. As a result, the gas pair selectivity increases if the thermal treatment takes place at 425 °C.
Fig. 6 illustrates the CO2-plasticization curves of these membranes as a function of heat treatment temperature. The original PI-g-CD membrane is easily swollen by CO2 and the onset of CO2-induced plasticization is at 10 atm. A higher thermal treatment temperature can effectively suppress this undesirable effect. The 425 °C cured PI-g-CD membrane does not exhibit plasticization up to 25 atm. Clearly, the high-temperature induced cross-linking reactions restrict chain movement and enhance polymer chains from CO2-induced plasticization.
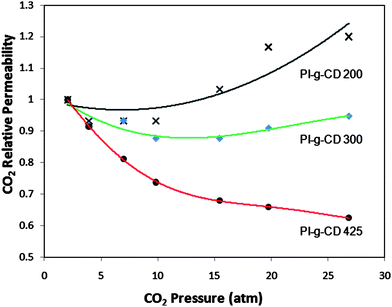 |
| Fig. 6
CO2 plasticization behavior of original and thermally cured CD grafted copolyimides. | |
3.3 Mixed gas tests
Mixed gas tests were performed using a binary feed mixture of 50/50% CO2–CH4 over a range of −10 °C to 25 °C. Table 2 compares the gas transport performance between pure gas and mixed gas tests. The permeability values in mixed gas tests are lower than those in pure gas tests by a value of 40–50% for PI-g-CD 300 and PI-g-CD 400 membranes. In addition to a 10 °C difference in testing temperature, the decrease in permeability is mainly due to the competitive sorption in membranes. The gas permeability decreases and CO2/CH4 selectivity increases with decreasing temperature in all PI-g-CD membranes, except for the case of CO2 permeability in PI-g-CD 400. This phenomenon arises from the fact that both the mobility of polymer chains and gas diffusivity decrease at low temperatures, as a result, permeabilities of all gases decrease. However, CO2 is a highly condensable gas that increases solubility coefficient with decreasing temperature. As a consequence, the CO2/CH4 selectivity increases at lower temperatures. This enhancement is particularly vivid for CO2 transport in the PI-g-CD 400 membrane because it has a negative apparent activation energy of transportation as shown in Table 2. In the solution-diffusion mechanism, the permeability (P) of a gas across the membrane could be given as the product of solubility (S) and diffusivity (D): P = DS. Solubility can be represented by the Arrhenius type equation which is dependent on sorption enthalpy: S = S0 exp(−ΔH/RT). Diffusivity can also be expressed in the similar form which is related to diffusion activation energy: D = D0 exp(−ED/RT). Therefore, the permeability can be written as the Arrhenius type equation: P = D0S0 exp(−(ED + ΔH)/RT) and the apparent activation energy EA = ED + ΔH. The diffusion activation energy ED is dependent on polymer chain stiffness and the average distance between polymer chains.14 When the thermal curing temperature is increased to 400 °C, the decomposition of CD creates many gas permeation channels, making ED lower, while the sorption enthalpy is always negative. As the result, the apparent activation energy shows a negative value and the CO2 permeability of PI-g-CD 400 increases with decreasing temperature. When thermal curing temperature is 425 °C, the thermally induced cross-linking will increase polymer chain stiffness and promote polymer chain packing. Therefore, ED increases and the apparent activation energy changes back to a positive value.
Table 2 Mixed gas permeabilities and selectivities of thermally cured CD grafted copolyimides
Membrane
ID
|
T/°C |
PCO2/Barrera |
PCH4/Barrera |
PCO2/PCH4 |
Activation Energy for CO2 Permeability Ep/kJ mol−1 |
Barrer = 10−10 cm3 (STP) cm cm−2s−1 cm Hg−1.** Pure gas permeability data. |
PI-g-CD 300 |
35** |
189 |
8.4 |
23 |
4.7 |
25 |
110 |
3.2 |
34 |
10 |
109 |
2.6 |
42 |
0 |
104 |
2.1 |
50 |
−10 |
84 |
1.5 |
54 |
PI-g-CD 400 |
35** |
921 |
58 |
16 |
−2.6 |
25 |
520 |
29 |
23 |
10 |
552 |
23 |
24 |
0 |
575 |
21 |
28 |
−10 |
597 |
16 |
38 |
PI-g-CD 425 |
35** |
4016 |
247 |
16 |
4.2 |
25 |
4289 |
286 |
16 |
10 |
4057 |
264 |
17 |
0 |
3696 |
182 |
20 |
−10 |
3454 |
142 |
24 |
4. Conclusions
We have demonstrated a new approach to prepare flexible and highly permeable polyimide membranes for gas separation by thermally curing polyimides consisting of thermal labile CD molecules and thermal cross-linkable polyimide. The following conclusions can be drawn:
1) The cavities formed by the decomposition of CD groups were micro-pores in the polymer matrix, resulting in a high permeability.
2) The gas selectivity of thermally treated PI-g-CD membranes at 425 °C increases because the cross-linking reactions caused by decarboxylation tighten and rigidify polymer chains, thus create ultra-fine micro-pores that integrally connect with micro-pores. The dimension of ultra-fine micro-pores in the neck-like structure determines the gas selectivity.
3) Thermally treated PI-g-CD membranes demonstrate excellent O2/N2, CO2/CH4 and C3H6/C3H8 separation performance, surpassing the trade-off line,15,43 as shown in Fig. 7. For O2/N2 separation, PI-g-CD 425 shows better performance than high permeable PIM polymer. For C3H6/C3H8 separation, not only can thermally cured PI-g-CD match in excellence carbon molecular sieve membranes, but they also exhibit much better toughness and flexibility as shown in Fig. 8. Since the main released gases from CD decomposition are C2/C3 compounds, the channels connected with micro-pores may possess the right characteristics to discriminate C3H6 and C3H8.
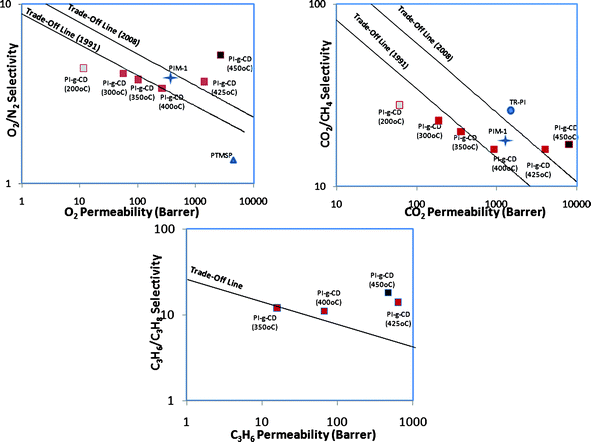 |
| Fig. 7 Tradeoff lines of O2/N2, CO2/CH4 and C3H6/C3H8. | |
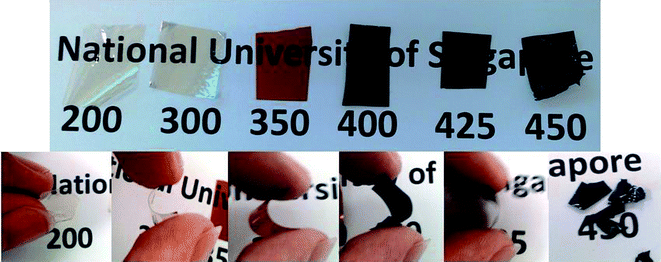 |
| Fig. 8 Mechanical strength and flexibility of CD grafted copolyimide and thermally cured CD grafted copolyimides. | |
The newly proposed strategy provides many advantages for facile tuning of the average interchain spacing and free volume size to achieve high gas separation performance for natural gas purification, CO2 capture and C3hydrocarbon separation.
Acknowledgements
The authors would like to thank the Singapore National Research Foundation (NRF) for the support of the Competitive Research Programme for the project entitled “Molecular Engineering of Membrane Materials: Research and Technology for Energy Development of Hydrogen, Natural Gas and Syngas” with the grant number of R-279-000-261-281. The authors also thank Ms B. T. Low and Mr H. Z. Chen for giving assistance to this work.
References
- B. D. Bhide, A. Voskericyan and S. A. Stern, Hybrid processes for the removal of acid gases from natural gas, J. Membr. Sci., 1998, 140, 27 CrossRef CAS.
-
A. L. Kohl and R. Nielson, Gas Purification, Gulf Publishing, Houston, Texas, 1997 Search PubMed.
- R. W. Baker, Future directions of membrane gas separation technology, Ind. Eng. Chem. Res., 2002, 41, 1393 CrossRef CAS.
- G. Chiappetta, G. Clarizia and E. Drioli, Design of an integrated membrane system for a high level hydrogen purification, Chem. Eng. J., 2006, 124, 29 CrossRef CAS.
-
D. R. Paul and Y. P. Yampolskii, Polymeric Gas Separation Membranes, CRC, Boca Raton, 1994 Search PubMed.
- P. Bernardo, E. Drioli and G. Golemme, Membrane gas separation: A review/state of the art, Ind. Eng. Chem. Res., 2009, 48, 4638 CrossRef CAS.
- V. Abets, T. Brinkmann, M. Dijkstra, K. Ebert, D. Fritsch, K. Ohlrogge, D. Paul, K.-V. Peinemann, S. P. Nunes, N. Scharnagl and M. Schossig, Developments in membrane research: From material via process design to industrial application, Adv. Eng. Mater., 2006, 8, 328 CrossRef CAS.
- Y. Xiao, B. T. Low, S. S. Hosseini, T. S. Chung and D. R. Paul, The strategies of molecular architecture and modification of polyimide-based membranes for CO2 removal from natural gas—a review, Prog. Polym. Sci., 2009, 34, 561 CrossRef CAS.
- Y. Hirayama, T. Yoshinaga, Y. Kusuki, K. Ninomiya, T. Sakakibara and T. Tamari, Relation of gas permeability with structure of aromatic polyimides I, J. Membr. Sci., 1996, 111, 169 CrossRef CAS.
- S. Akira, M. Tsukasa, M. Masatoshi and I. Kenichi, Relationships between the chemical structures and the solubility, diffusivity, and permselectivity of propylene and propane in 6FDA-based polyimides, J. Polym. Sci., Part B: Polym. Phys., 2000, 38, 2525 CrossRef.
- T. H. Kim, W. J. Koros, G. R. Husk and K. C. O'Brien, Relationship between gas separation properties and chemical structure in a series of aromatic polyimides, J. Membr. Sci., 1988, 37, 45 CrossRef CAS.
- Y. C. Wang, S. H. Huang, C. C. Hu, C. L. Li, K. R. Lee, D. J. Liaw and J. Y. Lai, Sorption and transport properties of gases in aromatic polyimide membranes, J. Membr. Sci., 2005, 248, 15 CrossRef CAS.
- L. M. Robeson, Correlation of separation factor versus permeability for polymeric membranes, J. Membr. Sci., 1991, 62, 165 CrossRef CAS.
- B. D. Freeman, Basis of permeability/selectivity tradeoff relations in polymeric gas separation membranes, Macromolecules, 1999, 32, 375 CrossRef CAS.
- L. M. Robeson, The upper bound revisited, J. Membr. Sci., 2008, 320, 390 CrossRef CAS.
- M. L. Cecopieri-Gomes, J. Palacios-Alquisira and J. M. Dominguez, On the limits of gas separation in CO2/CH4, N2/CH4 and CO2/N2 binary mixtures using polyimide membranes, J. Membr. Sci., 2007, 293, 53 CrossRef CAS.
- H. Kita, T. Inada, K. Tanaka and K. Okamoto, Effect of photocrosslinking on permeability and permoselectivity of gases through benzophenone-containing polyimide, J. Membr. Sci., 1994, 87, 139 CrossRef CAS.
- M. S. McCaig and D. R. Paul, Effect of UV cross-linking and physical aging on the gas permeability of thin glassy polyarylate films, Polymer, 1999, 40, 7209 CrossRef CAS.
- Y. Xiao, T. S. Chung, H. M. Guan and M. D. Guiver, Synthesis, Cross-linking and carbonization of Co-polyimides containing internal acetylene units, J. Membr. Sci., 2007, 302, 254 CrossRef CAS.
- G. M. Punt Bos, M. Wessling and H. Strathmann, Suppression of CO2-plasticization by semi-interpenetrating polymer network formation, J. Polym. Sci., Part B: Polym. Phys., 1998, 36, 1547 CrossRef.
- C. Staudt-Bickel and W. J. Koros, Improvement of CO2/CH4 separation characteristic of polyimides by chemical crosslinking, J. Membr. Sci., 1999, 155, 145 CrossRef CAS.
- J. H. Kim, W. J. Koros and D. R. Paul, Effects of CO2 exposure and physical aging on the gas permeability of thin 6FDA-based polyimide membrane – Part 2. With crosslinking, J. Membr. Sci., 2006, 282, 32 CrossRef CAS.
- Y. Liu, R. Wang and T. S. Chung, Chemical cross-linking modification of polyimide membranes for gas separation, J. Membr. Sci., 2001, 189, 231 CrossRef CAS.
- P. S. Tin, T. S. Chung, R. Wang, Y. Liu, S. L. Liu and K. P. Pramoda, Effects of cross-linking modification on gas separation performance of Matrimid membranes, J. Membr. Sci., 2003, 225, 77 CrossRef CAS.
- Y. C. Xiao, T. S. Chung and M. L. Chng, Surface characterization, modification chemistry and separation performance of polyimide and PAMAM dendrimer composites, Langmuir, 2004, 20, 8230 CrossRef CAS.
- B. T. Low, Y. Xiao and T. S. Chung, Amplifying the molecular sieving capability of polyimide membranes via coupling of diamine networking and molecular architecture, Polymer, 2009, 50, 3250 CrossRef CAS.
- C. E. Powell, X. J. Duthie, S. E. Kentish, G. G. Qiao and G. W. Stevens, Reversible diamine cross-linking of polyimide membranes, J. Membr. Sci., 2007, 291, 199 CrossRef CAS.
- C. M. Aberg, A. E. Ozcam, J. M. Majikes, M. A. Seyam and R. J. Spontak, Extended chemical crosslinking of a thermoplastic polyimide: Macroscopic and microscopic property development, Macromol. Rapid Commun., 2008, 29, 1461 CrossRef CAS.
- P. S. Tin, Y. Xiao and T. S. Chung, Polyimide-carbonized membranes for gas separation: structural, composition, and morphological control of precursors, Sep. Purif. Rev., 2006, 35, 285 CrossRef CAS.
- L. Shao, T. S. Chung and K. P. Pramoda, The evolution of physicochemical and transport properties of 6FDA-durene toward carbon membranes; from polymer, intermediate to carbon, Microporous Mesoporous Mater., 2005, 84, 59 CrossRef.
- M. B. Hagg, J. A. Lie and A. Lindbrathen, Carbon molecular sieve membranes: a promising alternative for selected industrial applications, Ann. N. Y. Acad. Sci., 2003, 984, 329 CrossRef CAS.
- S. M. Saufi and A. F. Ismail, Fabrication of carbon membranes for gas separation—a review, Carbon, 2004, 42, 241 CrossRef CAS.
- A. Singh and W. J. Koros, Significance of entropic selectivity for advanced gas separation membranes, Ind. Eng. Chem. Res., 1996, 35, 1231 CrossRef CAS.
- P. M. Budd and N. B. Mckeown, Highly permeable polymers for gas separation membranes, Polym. Chem., 2010, 1, 63 RSC.
- H. B. Park, C. H. Jung, Y. M. Lee, A. J. Hill, S. J. Pas, S. T. Mudie, E. V. Wagner, B. D. Freeman and D. J. Cookson, Polymers with cavities tuned for fast selective transport of small molecules and ions, Science, 2007, 318, 254 CrossRef CAS.
- J. I. Choi, C. H. Jung, S. H. Han, H. B. Park and Y. M. Lee, Thermally rearranged (TR) poly(benzoxazole-co-pyrrolone) membranes tuned for high gas permeability and selectivity, J. Membr. Sci., 2010, 349, 358 CrossRef CAS.
- C. H. Jung, J. E. Lee, S. H. Han, H. B. Park and Y. M. Lee, Highly permeable and selective poly(benzoxazole-co-imide) membranes for gas separation, J. Membr. Sci., 2010, 350, 301 CrossRef CAS.
- J. Gagliani and D. E. Supkis, Non-flammable polyimide materials for aircraft and spacecraft applications, Acta Astronaut., 1980, 7, 653 CrossRef CAS.
- W. Ensinger, R. Sudowe, R. Brandt and R. Neumann, Gas separation in nanoporous membranes formed by ethching ion irradiated polymer foils, RadiatPhys. Chem., 2010, 79, 25.
- R. A. Meyers, Polymerization of pyromellitic dianhydride with diphenylmethane diisocyanate, J. Polym. Sci., Part A-1, 1969, 7, 2757 CrossRef.
- J. L. Hedrick, C. J. Hawker, R. DiPietro, R. Jerome and Y. Charlier, The use of styrenic copolymers to generate polyimide nanofoams, Polymer, 1995, 36, 4855 CAS.
- W. C. Wang, R. H. Vora, E. T. Kang, K. G. Neoh, C. K. Ong and L. F. Chen, Nanoporous ultra-low-k films prepared from fluorinated polyimide with grafted poly(acrylic acid) side chains, Adv. Mater., 2004, 16, 54 CrossRef CAS.
- M. N. Islam, W. Zhou, T. Honda, K. Tanaka, H. Kita and K. Okamoto, Preparation and gas separation performance of flexible pyrolytic membranes by low-temperature pyrolysis of sulfonated polyimides, J. Membr. Sci., 2005, 261, 17 CrossRef CAS.
- L. X. Song and P. Xu, A comparative study on the thermal decomposition behaviors between β-cyclodextrin and its inclusion complexes of organic amines, J. Phys. Chem. A, 2008, 112, 11341 CrossRef CAS.
- A. M. Kratochvil and W. J. Koros, Decarboxylation-induced cross-linking of a polyimide for enhanced CO2 plasticization resistance, Macromolecules, 2008, 41, 7920 CrossRef CAS.
- T. P. Eskay, P. F. Britt and A. C. Buchanan III, Does decarboxylation lead to cross-linking in low-rank coals?, Energy Fuels, 1996, 10, 1257 CrossRef CAS.
- Y. Hishiyama and M. Nakamura, X-Ray diffraction in oriented carbon films with turbostratic structure, Carbon, 1995, 33, 1399 CrossRef CAS.
- C. L. Staiger, S. J. Pas, A. J. Hill and C. J. Cornelius, Gas separation, free volume distribution, and physical aging of a highly microporous spirobisindane polymer, Chem. Mater., 2008, 20, 2606 CrossRef CAS.
- B. W. Rowe, S. J. Pas, A. J. Hill, R. Suzuki, B. D. Freeman and D. R. Paul, A variable energy positron annihilation lifetime spectroscopy study of physical aging in thin glassy polymer films, Polymer, 2009, 50, 6149 CrossRef CAS.
- B. T. Low, T. S. Chung, H. Chen, Y. C. Jean and K. P. Pramoda, Tuning the free volume cavities of polyimide membranes via the construction of pseudo-interpenetrating networks for enhanced gas separation performance, Macromolecules, 2009, 42, 7042 CrossRef CAS.
|
This journal is © The Royal Society of Chemistry 2011 |
Click here to see how this site uses Cookies. View our privacy policy here.