DOI:
10.1039/C0AN00444H
(Paper)
Analyst, 2011,
136, 205-210
High electrochemiluminescence of a new water-soluble iridium(III) complex for determination of antibiotics†
Received
25th June 2010
, Accepted 21st September 2010
First published on 13th October 2010
Abstract
A new water-soluble iridium(III) diimine complex with appended sugar was synthesized and characterized. The electrochemiluminescent behavior of the new complex in aqueous buffer was first studied and the ECL signal was found to be much higher than that of [Ru(bpy)3]2+ at a Pt working electrode. Tri-n-propylamine (TPA) and antibiotics were determined by the ECL of the iridium(III) complex in aqueous buffer at the Pt electrode and the method was found to show good sensitivity and reproducibility. The new iridium(III) complex was found to display good solubility in aqueous solution and a strong ECL signal at the Pt electrode, which might open up the possibility of its application in analysis.
Introduction
Electrochemiluminescence (ECL) has been paid considerable attention during the past several decades because it offers lower background noise, higher detection sensitivity, a wide dynamic linear range and requires simple and inexpensive instrumentation in comparison with other methods.1 Among luminescent d6 transition-metal complexes, tris(2,2′-bypyridine)ruthenium(II) ([Ru(bpy)3]2+) has become the most thoroughly studied ECL active molecule in both aqueous and nonaqueous solution since the first report in 1972 by Bard,2 and it has been applied for the detection of amino acids,3oxalate,4antibiotics,5 clinical medicines,6DNA7 and so on.1 Glassy carbon (GC), gold, and platinum have been the working electrodes of choice for ECL studies.8 Although the ECL signal of [Ru(bpy)3]2+ was relatively weak at a Pt electrode usually employed as a working electrode in the analytical studies, it provides good reproducibility and is easy to prepare.9 Thus it is urgent to develop new ECL probes to improve the ECL sensitivity at a Pt electrode in aqueous buffer for analytical applications.
Recently iridium(III) cyclometalated derivatives with high photoluminescence efficiency have attracted considerable interest.10 In comparison to the most commonly used ruthenium(II) complexes (ϕem = 0.042, τ = 0.64 μs for [Ru(bpy)3]2+ in deaerated aqueous solution),11 they possess relatively long excited-state lifetimes, much higher emission efficiency and sensitivity, making them promising materials for electricity-to-light conversion in ECL reactions.12 There are some reports on the ECL of iridium(III) cyclometalated complexes in nonaqueous solution, and it was found that the ECL efficiency of iridium(III) cyclometalated complexes was much higher than that of the most commonly used ruthenium(II) complexes in organic solvent,12b,13 but to our knowledge, no investigation has previously been reported on the ECL behavior and application of iridium(III) complexes in aqueous solution because most iridium(III) complexes are only soluble in organic solvent.
In this paper, a new water-soluble iridium(III) complex (Scheme 1) with appended sugar was synthesized and characterized. The ECL behavior of the complex in aqueous buffer was studied and the ECL signal was found to be much higher than that of [Ru(bpy)3]2+ at the Pt electrode. Tri-n-propylamine (TPA) and antibiotics were determined by the ECL of the iridium(III) complex in aqueous buffer at the Pt electrode and the method showed good sensitivity and reproducibility. These results provided information for future ECL studies and an efficient ECL system for analytical applications.
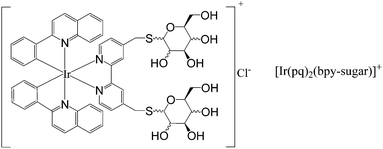 |
| Scheme 1 Chemical structure of the new water-soluble iridium(III) complex | |
Experimental
Materials
Anhydrous iridium(III) chloride was purchased from Alfa Aesar Chemical Co. 1-Thio-beta-D-glucose tetraacetate was purchased from J&K Chemical Co. 2-Phenylquinoline (pq) and TPA were purchased from Aldrich Chemical Co. Ampicillin and erythrocin (98%) were purchased from Aladdin Chemical Co. Other chemicals were of analytical grade and used as received. The bpy-sugar ligand was prepared according to the literature procedures.14 The stock solutions were stored in a refrigerator and diluted to a series of concentrations with double-distilled water (DDW) before use. The pH of the phosphate buffer solution (PBS) was adjusted with concentrated NaOH or phosphoric acid.
Synthesis of [Ir(pq)2(bpy-sugar)]Cl
A mixture of 2-ethoxyethanol and water (3
:
1, v/v) was added to a flask containing IrCl3 (1.0 eq) and pq (2.5 eq). The mixture was heated to reflux for 24 h. After cooling, the solid precipitate was filtered to give the crude cyclometalated iridium(III) chloro-bridged dimer. The solution of cyclometalated iridium(III) chloro-bridged dimer (1.0 eq) and bpy-sugar (2.0 eq) in CH2Cl2–MeOH (30 mL, 1
:
1 (v/v)) was heated to reflux for 12 h under N2. The solution was then evaporated to dryness under reduced pressure, after which the residue was repeatedly dissolved in about 5 mL ethanol and the white precipitation was filtered off to give the orange-yellow solid (yield 61%). The identities of the complex were confirmed by satisfactory 1H NMR spectroscopy, elemental analysis and ESI mass spectrometry. 1H NMR (400 MHz, DMSO): δH 8.56 (m, 4H, quinoline), 8.41 (d, 1H, J = 9.0 Hz, quinoline), 8.35 (s, 1H, quinoline), 8.28 (t, 2H, J = 6.4 Hz, bipyridyl), 7.99 (t, 2H, J = 5.8 Hz, bipyridyl), 7.94 (q, 2H, benzene), 7.70 (d, 1H, J = 5.7 Hz, quinoline), 7.57 (d, 1H, J = 5.6 Hz, quinoline), 7.43 (m, 2H, quinoline), 7.16 (m, 6H, benzene), 6.82 (t, 2H, J = 6.4 Hz, quinoline), 6.43 (t, 2H, J = 8.2 Hz, bipyridyl), 5.29–4.93 (b, 7H, OH), 4.67 (s, 1H, OH), 3.98, 3.95 (d, J = 13.4 Hz, 2H, alkyl), 3.83 (m, 2H, alkyl), 3.76 (d, 1H, J = 9.1 Hz, alkyl), 3.67 (m, 2H, alkyl), 3.58 (d, 1H, J = 9.1 Hz, alkyl), 3.45–3.40 (2H, overlap with solvent peak, alkyl), 3.02 (m, 8H, alkyl). ESI-MS: m/z (%): 1173.3 (100) [(M–Cl)+]. Anal. Calcd (%) for C54H52ClIrN4O10S2·2.5H2O: C 51.73, H 4.47, N 4.58. Found: C 51.73, H 4.70, N 4.29.
Methods
Cyclic voltammetry (CV) was performed with a Model CHI 660 electrochemical analyzer (Shanghai Chenhua Apparatus Company, China), using a three-electrode cell consisting of a 2 mm-diameter Pt, Au or GC working electrode (Shanghai Chenhua Apparatus Company, China), a Ag/AgCl reference electrode and a Pt wire counter electrode. ECL signals were recorded by a Model MCDR-A multifunctional chemistry analytical processing system (Xi'an Remex Electronic and Technological Co., Xi'an, China). All electrochemical and ECL experiments were referenced with respect to a Ag/AgCl electrode (0.20 V vs.NHE). A voltage of −600 V was supplied to the photomultiplier tube (PMT). The ECL spectrum was collected on a Sanco 970 CRT spectrofluorometer by applying a static potential of 1.2 V. Before each experiment, the Pt working electrode was subjected to repeated scanning in the potential range of −0.65 to 1.2 V (vs.SCE) (for a Au electrode, the potential range is from −0.5 to +1.4 V (vs.SCE)) in a phosphate buffer (0.1 M), until reproducible voltammograms were obtained, and was then rinsed with DDW. The glassy carbon electrode was treated prior to use by polishing with 0.3 μm R-alumina (Linde), followed by rinsing with DDW and sonication for about 1 min, and was then finally rinsed under a stream of DDW. The ferrocenium–ferrocene couple (FeCp2+/0) was used as the internal reference for the electrochemical studies of the new complex.15 Emission lifetime measurements were performed using a conventional nanosecond pulsed laser system. The excitation source used was the 355 nm output (third harmonic, 8 ns) from a Spectra-Physics Quanta-Ray Q-switched GCR-150-10 pulsed Nd-YAG laser (10 Hz). Luminescence decay signals were detected by a Hamamatsu R928 photomultiplier tube and recorded on a Tektronix Model TDS-620A (500 MHz, 2 GS s−1) digital oscilloscope.
Results and discussion
Absorption and photoluminescence
The newly synthesized [Ir(pq)2(bpy-sugar)]Cl has a good solubility in water. The electronic absorption spectra of the complex in water were mainly dominated by very intense high-energy absorption bands at ca. 266 and 319 nm, and a comparatively less intense low-energy band at ca. 336 and 432 nm, which tailed off to ca. 510 nm (Fig. 1). With reference to previous spectroscopic studies on related cyclometalated iridium(III) diimine systems,16 these absorption bands with extinction coefficients in the order of 104 dm3·mol−1 cm−1 were ascribed to spin-allowed π → π* intraligand (1IL) transitions of the cyclometalating ligands or substituted bpy ligand. The less intense low-energy absorption bands were likely to originate from the dπ(Ir) → π*(ligand) spin-allowed metal-to-ligand charge-transfer (1MLCT) transition. Similar assignments for the related cyclometalated iridium(III) diimine systems are also reported in the literature.16 The weak absorption tails at ca. 510 nm were tentatively assigned as a spin-forbidden 3MLCT dπ(Ir) → π*(ligand) transition, characteristic of the cyclometalated iridium(III) diimine systems.16 There were no obvious changes in the electronic absorption spectra of the new complex in water stored in the dark for 24 h, which showed that the stability of the new iridium(III) complex in aqueous solution was good.
[Ir(pq)2(bpy-sugar)]Cl was found to emit strongly at room temperature upon excitation with both UV and visible light in aqueous solution with emission maxima at ca. 557 nm (Fig. 2). With reference to previous spectroscopic studies on other related cyclometalated iridium(III)-pq systems,17 the excited state of this complex was likely to possess 3IL (π → π*)(pq−) character and perhaps some 3MLCT (dπ(Ir) → π*(pq−)) character as well. [Ir(pq)2(bpy-sugar)]Cl exhibited a long emission lifetime (τ = 1.0 μs) in deaerated fluid aqueous solution at 298 K, indicating that its emissive state should involve predominant 3IL (π → π*)(pq−) character. Similar features have been observed in related iridium(III)-pq systems.17 The luminescence quantum efficiency of the complex in deaerated and aerated aqueous solution was about three times higher than [Ru(bpy)3]Cl2 (φRu(II) = 1.0), which would make it more sensitive in analytical and biochemical applications.
![Emission spectrum of [Ir(pq)2(bpy-sugar)]Cl in aqueous solution (solid line), ECL spectrum obtained by applying a static potential of 1.2 V (dashed line) and ECL signals obtained by CV scan (0–1.4 V, scan rate: 0.1 V s−1) using filters (circle symbols) of 10 μM complex and 20 mM TPA in 0.15 M PBS (pH 7.5).](/image/article/2011/AN/c0an00444h/c0an00444h-f2.gif) |
| Fig. 2
Emission spectrum of [Ir(pq)2(bpy-sugar)]Cl in aqueous solution (solid line), ECL spectrum obtained by applying a static potential of 1.2 V (dashed line) and ECL signals obtained by CV scan (0–1.4 V, scan rate: 0.1 V s−1) using filters (circle symbols) of 10 μM complex and 20 mM TPA in 0.15 M PBS (pH 7.5). | |
Electrochemistry of [Ir(pq)2(bpy-sugar)]+
Due to the low solubility of [Ir(pq)2(bpy-sugar)]Cl in acetonitrile, a metathesis reaction afforded the iridium(III) complex as the PF6− salt. The study of the electrochemical properties of the new iridium(III) diimine complex was then conducted at a GC electrode in acetonitrile solution (0.1 M nBu4NPF6) by cyclic voltammetry. The complex showed rich electrochemical behaviour with redox couples in the cyclic voltammogram (Fig. 3). The new iridium(III) complex showed an oxidation couple at ca. +1.21 V which was assigned as the oxidation of iridium(III), similar to other related iridium(III) complexes.18 The oxidation couple was a little lower than the Ru2+/3+redox couple of [Ru(bpy)3]2+ (Ep = 1.26 V vs.Ag/AgCl).19 The redox chemistry showed that the peak current ratio (ipa/ipc) and peak separation (ΔEp) were 1.95 and 88 mV at a scan rate of 0.1 V s−1, this indicated a quasi-reversible one-electron-transfer system. The complex exhibited three quasi-reversible reduction couples at around −1.57 V, −1.82 V and −2.07 V, as shown in Fig. 3. It was likely that these waves were associated with the reduction of the diimine and cyclometalating ligands with reference to previous studies.16 Under the experimental conditions, the reversible couple FeCp2+/0 gave a ipa/ipc of 1 and ΔEp of 65 mV irrespective of the scan rate.
Electrochemiluminescence of [Ir(pq)2(bpy-sugar)]+/TPA
Like the [Ru(bpy)3]2+/TPA system,20 the [Ir(pq)2(bpy-sugar)]+/TPA system in phosphate buffer also generated ECL signals at a Pt electrode as shown in Fig. 4. The ECL signal was found to occur at about 1.0 V and increase with increasing potential, finally forming a peak at about +1.2 V where the iridium(III) complex was oxidized, and then the signal decreased. The ECL signal generated from the present system was mainly produced by the reaction of the oxidized complex, [IrIV(pq)2(bpy-sugar)]2+, with the TPA radical. This indicated that the mechanism of the ECL waves followed the classic oxidative reduction coreactant mechanism, where oxidation of TPA generates a strongly reducing species TPA˙.4a The emission excited state was produced from the reaction of directly oxidized iridium(III) complex at the electrode surface with the TPA˙ radical. The obtained ECL spectrum of the [Ir(pq)2(bpy-sugar)]+/TPA system at the Pt electrode by applying static potential was characterized by a broad emission band as shown in Fig. 2. The ECL signals were also recorded by CV scans with filters (Fig. 2). The ECL wavelength maximum for both the two methods was about 571 nm. Compared to the luminescence, the ECL spectrum was shown to be slightly broader in shape with a small red shift of 14 nm for λmax. This was probably due to an inner filter effect as a result of the high concentration of complex needed to obtain measurable ECL in addition to the difference in resolution between the two instruments where the spectra were collected.21
![Cyclic voltammograms (B) and corresponding ECL signals (A) of [Ir(pq)2(bpy-sugar)]+ (a) and [Ru(bpy)3]2+ (b) at a Pt electrode in 0.15 M PBS (pH 7.5) containing 10 μM complex and 20 mM TPA. Scan rate: 0.1 V s−1. Inset: ECL peak intensity of [Ir(pq)2(bpy-sugar)]+ as a function of pH.](/image/article/2011/AN/c0an00444h/c0an00444h-f4.gif) |
| Fig. 4
Cyclic voltammograms (B) and corresponding ECL signals (A) of [Ir(pq)2(bpy-sugar)]+ (a) and [Ru(bpy)3]2+ (b) at a Pt electrode in 0.15 M PBS (pH 7.5) containing 10 μM complex and 20 mM TPA. Scan rate: 0.1 V s−1. Inset: ECL peak intensity of [Ir(pq)2(bpy-sugar)]+ as a function of pH. | |
The effect of pH on the ECL intensity was investigated using a Pt working electrode as shown in the inset of Fig. 4. The concentration of the iridium(III) complex was 10 μM, and the concentration of TPA was 20 mM. The effect of pH on the ECL intensity was studied from pH 6.0 to 9.5. As the pH of buffer solution was increased, the ECL intensity of the iridium(III) complex was found to increase first and reach a maximum value at about pH 7.5, then decrease gradually. The ECL intensity of the iridium(III) complex at pH 7.5 was found to be almost three times higher than that at pH 6.0 as shown in Fig. 4. The profile was similar to a previous report for the [Ru(bpy)3]2+/TPA system.22 The solution pH must be sufficiently high to promote the deprotonation of TPAH and TPA˙+ and lower than 9 to prevent the effect of hydroxide ions. Hence, pH 7.5 was employed as the optimal pH value for both the new iridium(III) complex and [Ru(bpy)3]2+.
Fig. 5 shows the dependence of ECL intensities on the concentrations of TPA from 0.001 mM to 20 mM. The ECL intensity of the iridium(III) complex was found to increase with increasing the TPA concentration. At the same conditions, the ECL intensity of the iridium(III) complex was much larger than that of [Ru(bpy)3]2+ within the studied concentration range of TPA. When the TPA concentration was 20 mM, the ECL intensity of the iridium(III) complex was about 5 times higher than that of [Ru(bpy)3]2+ as shown in Fig. 4. This was probably due to the more hydrophilic properties of the new iridium(III) complex with the appended sugar group. Because the hydrophilic head is adsorbed on the Pt electrode surface,23 an enhancement of oxidation current of the complex and ECL intensity will occur (Fig. 4). The log-log plot for the iridium(III) complex and [Ru(bpy)3]2+ at the studied concentration range of TPA were logI = 5.883 + 0.703logC (R = 0.997) and logI = 4.963 + 0.622logC (R = 0.998), respectively. The results show that the slope and intercept of the iridium(III) complex are larger than that of [Ru(bpy)3]2+, which demonstrates that the ECL of the new iridium(III) complex is more sensitive to TPA than [Ru(bpy)3]2+.
![The dependence of ECL intensities on the concentrations of TPA at a Pt electrode. Solution: 0.15 M PBS (pH 7.5) containing 10 μM complex and different concentrations of TPA. Scan rate: 0.1 V s−1. a, [Ir(pq)2(bpy-sugar)]+; b, [Ru(bpy)3]2+. Inset: logIECL as a function of log[TPA].](/image/article/2011/AN/c0an00444h/c0an00444h-f5.gif) |
| Fig. 5 The dependence of ECL intensities on the concentrations of TPA at a Pt electrode. Solution: 0.15 M PBS (pH 7.5) containing 10 μM complex and different concentrations of TPA. Scan rate: 0.1 V s−1. a, [Ir(pq)2(bpy-sugar)]+; b, [Ru(bpy)3]2+. Inset: logIECL as a function of log[TPA]. | |
Meanwhile, the effect of complex concentration on the ECL intensity was studied in the presence of 20 mM TPA at the Pt working electrode. The ECL intensity of both the iridium(III) complex and [Ru(bpy)3]2+ was found to increase with increasing complex concentration. The iridium(III) complex showed a stronger ECL signal than [Ru(bpy)3]2+ at the studied concentrations of complex from 1 μM to 50 μM, and the ECL intensity of the iridium(III) complex was found to be about 5 times stronger than that of [Ru(bpy)3]2+ as the concentration of complexes was 10 μM. The results demonstrate that the new iridium(III) complex showed a much stronger ECL signal than the classical [Ru(bpy)3]2+/TPA system at the Pt working electrode, which would make it more sensitive in analytical applications.
ECL behavior of the [Ir(pq)2(bpy-sugar)]+/TPA system in aqueous buffer at the GC electrode was also investigated, and the ECL intensity was found to be about two times higher than that of [Ru(bpy)3]2+/TPA. The ECL intensity of the [Ir(pq)2(bpy-sugar)]+/TPA system in aqueous buffer at a Au electrode showed no obvious advantages over the [Ru(bpy)3]2+/TPA system. The above results suggest a higher effectivity of Pt compared to a Au electrode. This is mainly because Pt is usually a better catalyst for the oxidation of organic species such as TPA in acidic or neutral media. The adsorption of the hydrophilic head of the complex on the electrode surface would not only facilitate the oxidation of the complex, but also inhibit the growth of the oxide layer, meaning that the catalytic effect of a Pt electrode was significant for the oxidation of TPA.23,24
Determination of TPA
Since Leland and Powell have suggested tripropylamine (TPA) as a co-reactant,22b extensive research has been focused on the sensitive determination of TPA.25Fig. 6 illustrates a typical log-log calibration plot for the detection of TPA based on the ECL of [Ir(pq)2(bpy-sugar)]+ in phosphate buffer at a Pt electrode. The linear concentration range was found to extend from 1 nM to 1 μM (R = 0.991) and the limit of determination (LOD) was found to be 0.1 nM with a signal-to-noise ratio of 3. The RSD of ECL peak height (n = 5) was 4.3%, which showed that the reproducibility of ECL signal based on a [Ir(pq)2(bpy-sugar)]+ probe in phosphate buffer at a Pt electrode for the detection of TPA was good. The results demonstrate that the determination of TPA using ECL of the new iridium(III) complex is much more sensitive than [Ru(bpy)3]2+ in aqueous buffer at a Pt electrode previously reported to have a LOD of 20 nM.25a
![Calibration plot for the detection of TPA with a [Ir(pq)2(bpy-sugar)]+ ECL probe at a Pt electrode. Solution: 50 mM PBS (pH 7.5) containing 0.1 mM [Ir(pq)2(bpy-sugar)]+ and different concentrations of TPA. Scan rate: 0.1 V s−1.](/image/article/2011/AN/c0an00444h/c0an00444h-f6.gif) |
| Fig. 6 Calibration plot for the detection of TPA with a [Ir(pq)2(bpy-sugar)]+ ECL probe at a Pt electrode. Solution: 50 mM PBS (pH 7.5) containing 0.1 mM [Ir(pq)2(bpy-sugar)]+ and different concentrations of TPA. Scan rate: 0.1 V s−1. | |
Erythromycin is an important macrolide antibiotic used in clinical applications for the treatment of bacterial infections. It has been paid extensive attention to achieve a sensitive determination and [Ru(bpy)3]2+ has been used as an ECL probe in most of the studies.26 In our study, the limit of detection for erythromycin was found to be 0.2 nM with a signal-to-noise ratio of 3 based on the ECL of [Ir(pq)2(bpy-sugar)]+ (0.05 mM) in 50 mM phosphate buffer (pH 7.5) at a Pt electrode. The calibration curve was linear over the range from 1 nM to 0.5 μM and the RSD of peak height was found to be 2.4% (n = 5). It was more sensitive than the previously reported ECL determination of erythromycin based on [Ru(bpy)3]2+.27 In addition, the method has also been developed for the determination of ampicillin. The detection limit (S/N = 3) was found to be 3 nM with a linear range from 3 nM to 1 mM and the RSD of peak height was 3.3% (n = 5). All data are shown in Table 1. The results show that the determination of TPA and some antibiotics based on the new iridium(III) complex in phosphate buffer is sensitive, and its ECL signal shows good reproducibility at the Pt electrode.
Analytes
|
Linear range |
Correlation coefficient |
LOD (S/N = 3) |
RSD of peak height (n = 5) |
TPA |
1 nM–1 μM |
0.991 |
0.1 nM |
4.3% |
Erythromycin
|
1 nM–0.5 μM |
0.994 |
0.2 nM |
2.4% |
Ampicillin
|
3 nM–1 μM |
0.990 |
1 nM |
3.3% |
Conclusions
In this paper, a new water-soluble iridium(III) complex was synthesized and characterized. ECL behavior of the new iridium(III) complex in phosphate buffer was first investigated and it was found that the ECL intensity of the new iridium(III) complex at the Pt electrode was much stronger than that of [Ru(bpy)3]2+. The sensitivity for TPA was much higher than that previously reported using [Ru(bpy)3]2+ in phosphate buffer. This new water-soluble iridium(III) complex was also successfully tested for the detection of two common antibiotics. The results show that the new iridium(III) complex provides a strong ECL signal with good reproducibility in aqueous buffer, which might open up its analytical application as an ECL probe.
Acknowledgements
This work was supported by the National Scientific Foundation of China (NSFC No. 20801014, 20735002, 20801013), Scientific Foundation of Fujian Province (No. 2009J05026) and the start-up funding from the Fuzhou University (No. 826505). Prof. V. W. W. Yam is acknowledged for access to the equipment for the lifetime measurements.
References
-
A. J. Bard, Ed., Electrogenerated Chemiluminescence, Marcel Dekker, New York, 2004 Search PubMed; W. Miao, Chem. Rev., 2008, 108, 2506–2553 Search PubMed; M. M. Richter, Chem. Rev., 2004, 104, 3003–3036 CrossRef CAS; K. A. Fahnrich, M. Pravda and G. G. Guilbault, Talanta, 2001, 54, 531–559 CrossRef CAS.
- N. Tokel and A. J. Bard, J. Am. Chem. Soc., 1972, 94, 2862–2863 CrossRef CAS.
- J. Li, Q. Yan, Y. Gao and H. Ju, Anal. Chem., 2006, 78, 2694–2699 CrossRef CAS; M. T. Chiang and C. W. Whang, J. Chromatogr., A, 2001, 934, 59–66 CrossRef CAS.
- I. Rubinstein and A. J. Bard, J. Am. Chem. Soc., 1981, 103, 512–516 CrossRef CAS; H. Y. Wang, G. B. Xu and S. J. Dong, Anal. Chim. Acta, 2003, 480, 285–290 CrossRef CAS.
- X. C. Zhao, T. Y. You and E. K. Wang, J. Chromatogr. B, 2004, 810, 137–142 CrossRef CAS.
- J. L. Yan, J. F. Liu, W. D. Cao and E. K. Wang,
et al.
, Microchem. J., 2004, 76, 11–16 CrossRef CAS.
- W. Miao and A. J. Bard, Anal. Chem., 2003, 75, 5825–5834 CrossRef CAS; L. Dennany, R. J. Forster and J. F. Rusling, J. Am. Chem. Soc., 2003, 125, 5213–5218 CrossRef CAS.
- X. Hun and Z. J. Zhang, Electroanalysis, 2008, 20, 874–880 CrossRef CAS; L. H. Shi, X. Q. Liu and G. B. Xua, Electroanalysis, 2006, 18, 1584–1589 CrossRef CAS; X. B. Yin, B. B. Sha and X. H. Zhang, Electroanalysis, 2008, 20, 1085–1091 CrossRef CAS; P. Jiang, L. Yan and D. Xiao, Electroanalysis, 2009, 21, 1611–1616 CrossRef CAS; S. L. Chen, Y. Liu and Y. Z. Zheng, Luminescence, 2006, 21, 20–25 CrossRef CAS; Y. Gao, Q. Xiang and E. K. Wang, Electrophoresis, 2006, 27, 4842–4848 CrossRef CAS.
- M. A. Ghanem, Electrochem. Commun., 2007, 9, 2501–2506 CrossRef CAS; L. Hernhdez and A. Zapardiel, Anal. Chim. Acta, 1996, 336, 85–93 CrossRef CAS; M. Li and S. H. Lee, Luminescence, 2007, 22, 588–593 CrossRef CAS.
- S. Lamansky, P. Djarovich and D. Murphy,
et al.
, J. Am. Chem. Soc., 2001, 123, 4304–4312 CrossRef CAS; M. K. Nazeeruddin, R. Humphry-Baker and D. Berner,
et al.
, J. Am. Chem. Soc., 2003, 125, 8790–8797 CrossRef CAS; A. Tsuboyama, H. Iwawaki and M. Furogori,
et al.
, J. Am. Chem. Soc., 2003, 125, 12971–12979 CrossRef CAS; S. Tokito, T. Iijima, T. Tsuzuki and F. Sato, Appl. Phys. Lett., 2003, 83, 2459–2462 CrossRef CAS.
- J. Van Houten and R. J. Watts, J. Am. Chem. Soc., 1976, 98, 4853–4858 CrossRef CAS.
- E. M. Gross, N. R. Amstrong and R. M. Wightman, J. Electrochem. Soc., 2002, 149, 137–142; D. Bruce and M. M. Richter, Anal. Chem., 2002, 74, 1340–1342 CrossRef CAS; C. Cole, B. D. Muegge and M. M. Richter, Anal. Chem., 2003, 75, 601–604 CrossRef CAS.
- J. I. Kim, I. S. Shin, H. Kim and J. K. Lee, J. Am. Chem. Soc., 2005, 127, 1614–1615 CrossRef CAS; I. S. Shin, J. I. Kim, T. H. Kwon, J. I. Hong, J. K. Lee and H. Kim, J. Phys. Chem. C, 2007, 111, 2280–2286 CrossRef CAS; A. Kapturkiewicz, T.-M. Chen, I. R. Laskar and J. Nowacki, Electrochem. Commun., 2004, 6, 827–831 CrossRef CAS.
- M. Gottschaldt, D. Koth and D. Muller,
et al.
, Chem.–Eur. J., 2007, 13, 10273–10280 CrossRef.
- P. S. Braterman, J. Song and R. D. Peacock, Spectrochim. Acta, Part A, 1992, 48, 899–903 CrossRef.
- K. K. W. Lo, J. S. W. Chan, C. K. Chung, V. W. H. Tsang and N. Zhu, Inorg. Chim. Acta, 2004, 357, 3109–3118 CrossRef CAS; A. P. Wilde and R. J. Watts, J. Phys. Chem., 1991, 95, 622–629 CrossRef CAS; S. Serroni, A. Juris, S. Campagna, M. Venturi, G. Denti and V. Balzani, J. Am. Chem. Soc., 1994, 116, 9086–9091 CrossRef CAS; G. Calogero, G. Giuffrida, S. Serroni, V. Ricevuto and S. Campagna, Inorg. Chem., 1995, 34, 541–545 CrossRef CAS; G. Di Marco, M. Lanza, A. Mamo, I. Stefio, C. Di Pietro, G. Romeo and S. Campagna, Anal. Chem., 1998, 70, 5019–5023 CrossRef CAS; N. Francesco, C. Alessandra, C. Sebastiano and S. Scolastica, Inorg. Chem., 1999, 38, 2250–2258 CrossRef CAS; P. M. Griffiths, F. Loiseau, F. Puntoriero, S. Serroni and S. Campagna, Chem. Commun., 2000, 2297–2298 RSC; F. Neve, A. Crispini, S. Serroni, F. Loiseau and S. Campagna, Inorg. Chem., 2001, 40, 1093–1101 CrossRef CAS; I. Ortmans, P. Didier and A. Kirsch-De Mesmaeker, Inorg. Chem., 1995, 34, 3695–3704 CrossRef CAS; M. Maestri, V. Balzani, C. Deuschel Cornioley and A. Von Zelewsky, Adv. Photochem., 1992, 17, 1–68 CAS.
- S. Lamansky, P. Djurovich, D. Murphy, F. Abdel-Razzaq, R. Kwong, I. Tsyba, M. Bortz, B. Mui, R. Bau and M. E. Thompson, Inorg. Chem., 2001, 40, 1704–1711 CrossRef CAS; K. K.-W. Lo, C.-K. Chung, T. K.-M. Lee, L.-H. Lui, K. H.-K. Tsang and N. Zhu, Inorg. Chem., 2003, 42, 6886–6897 CrossRef CAS; K. K.-W. Lo, K. Yin. Zhang, C.-K. Chung and K. Y. Kwok, Chem.–Eur. J., 2007, 13, 7110–7120 CrossRef CAS.
- Y. Ohsawa, S. Sprouse and R. J. Watts, J. Phys. Chem., 1987, 91, 1047–1054 CrossRef CAS; F. O. Garces, K. A. King and R. J. Watts, Inorg. Chem., 1988, 27, 3464–3471 CrossRef CAS; P. M. Griffiths, F. Loiseau, F. Puntoriero, S. Serroni and S. Campagna, Chem. Commun., 2000, 2297–2299 RSC; F. Neve, M. La Deda, A. Crispini, A. Bellusci, F. Puntoriero and S. Campagna, Organometallics, 2004, 23, 5856–5863 CrossRef CAS.
- C. H. Braunstein, A. D. Baker, T. C. Strekas and H. D. Gafney, Inorg. Chem., 1984, 23, 857–864 CrossRef.
- F. R. F. Fan, D. Cliffel and A. J. Bard, Anal. Chem., 1998, 70, 2941–2948 CrossRef CAS; M. M. Richter, A. J. Bard, W. Kim and R. H. Schmehl, Anal. Chem., 1998, 70, 310–318 CrossRef CAS.
- R. Y. Lai and A. J. Bard, J. Phys. Chem. A, 2003, 107, 3335–3340 CrossRef CAS; M. Bandini, M. Bianchi, G. Valenti, F. Piccinelli, F. Paolucci, M. Monari, A. Umani-Ronchi and M. Marcaccio, Inorg. Chem., 2010, 49(4), 1439–1448 CrossRef CAS.
- A. W. Knight and G. M. Greenway, Analyst, 1996, 121, 101–106 Search PubMed; K. Leland and M. J. Powell, J. Electrochem. Soc., 1990, 137, 3127–3131 CAS.
- F. Li and Y. Zu, Anal. Chem., 2004, 76, 1768–1772 CrossRef CAS.
- Y. Zu and A. J. Bard, Anal. Chem., 2000, 72, 3223–3232 CrossRef CAS.
- M. Sreedhar, Electrophoresis, 2005, 26, 2984–2990 CrossRef CAS; L. Zhang, Z. Guo, Z. Xu and S. J. Dong, J. Electroanal. Chem., 2006, 592, 63–67 CrossRef CAS; H. N. Choi, S. H. Cho and W. Y. Lee, Anal. Chem., 2003, 75, 4250–4256 CrossRef CAS; Y. Zhuang and H. Ju, Electroanalysis, 2004, 16, 1401–1405 CrossRef CAS.
- I. Kanfer, J. Chromatogr., A, 1998, 812, 255–286 CrossRef CAS; L. Dong and M. T. Martin, Anal. Biochem., 1996, 236, 344–347 CrossRef CAS.
- B. Y. Deng, Y. H. Kang, X. F. Li and Q. M. Xu, J. Chromatogr., B: Anal. Technol. Biomed. Life Sci., 2007, 857, 136–141 CrossRef CAS.
|
This journal is © The Royal Society of Chemistry 2011 |