DOI:
10.1039/C0NR00501K
(Communication)
Nanoscale, 2010,
2, 2561-2564
Highly fluorescent magnetic quantum dot probe with superior colloidal stability†
Received
14th July 2010
, Accepted 13th August 2010
First published on
24th September 2010
Abstract
A magnetic quantum dot (MQD) based cellular nanoprobe, composed of a magnetic oxide nanoparticle component and a quantum dot component, has been synthesized and used for both imaging and separation. The successful synthesis is based on a reverse micelle based polyacrylate coating in the presence of component nanoparticles, followed by their functionalization via conjugation chemistry.
Introduction
Multifunctional nanoparticles are considered to be a new class of material with interesting potential applications such as multimodal imaging, imaging and separation or imaging and detection.1,2 Magnetic quantum dots (MQDs) with one fluorescent and one magnetic nanocomponent are considered as a powerful class of multifunctional probes for both fluorescence and magnetic resonance imaging (MRI) as well as magnetic separation.2 However, the synthesis of highly fluorescent MQD with small hydrodynamic diameter and their functionalization with different affinity molecules are challenging problems.2 Common synthetic strategies involve (i) the seeding growth method where one of the component particles is used as a seed to grow the other particle,2e,i,j,k (ii) synthesis of component particles separately followed by their incorporation into a micron size porous bead2b,c or polymeric micelle,2f,h and (iii) the ligand exchange approach where ligand cell of one component particle is replaced by another core–shell particle.2l However, the seeding growth method produces MQD with low quantum yield (<5%) due to the quenching effect by a magnetic component adjacent to the quantum dot2b,e,i,j and other methods produce larger size particles with poor functionalization and labeling scope. In addition, an appropriate coating is required in order to preserve the high quantum yield of MQDs and it assists in deriving various functional MQDs.2l Here we report water soluble MQD probes <100 nm in size with high colloidal stability and 8–20% fluorescence quantum yield. The successful synthesis is based on exploiting the synthetic condition of a high quality component nanoparticle3,4 and by selecting an optimized coating method from among all those we have developed (e.g. silica, polyacrylate, polyimidazole, chitosan oligosaccharide and polyaspartic acid).5 The MQD probes are composed of γ-Fe2O3 as the magnetic component and ZnS-capped CdSe (CdSe–ZnS) quantum dot as the fluorescent component and coated with a cross-linked polyacrylate shell having polyethylene glycol and an affinity molecule.
MQD synthesis
The synthesis involves the preparation of monodispersed γ-Fe2O3 and CdSe–ZnS separately, followed by a reverse micelle based in situ polyacrylate coating in the presence of the two component particles (Scheme 1).5a Hydrophobic γ-Fe2O3 and CdSe–ZnS are first prepared by a high temperature organometallic synthetic route in octadecene solvent.3,4 Then they are purified from free surfactants by conventional ethanol based precipitation and chloroform based redispersion method and finally dissolved in cyclohexane. Next, purified component nanoparticles and polymer-forming monomer precursors are introduced into an Igepal–cyclohexane reverse micelle medium and polymerization is initiated in an oxygen free atmosphere. Igepal produces 1–10 nm size spherical reverse micelles in cyclohexane and assists in solubilizing both the hydrophobic nanoparticles and the polar polymer precursor so that polymerization starts in homogeneous solution.6 After polymerization, particles are precipitated by ethanol addition, separated, washed and dissolved in water.
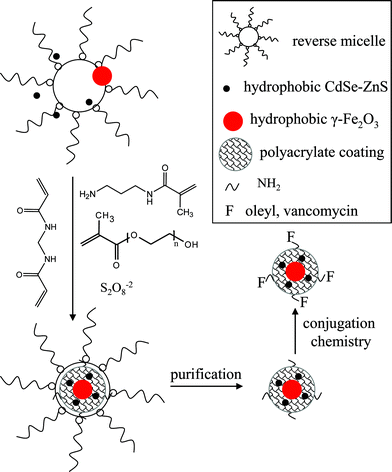 |
| Scheme 1 Reverse micelle based coating chemistry strategy in producing MQD probe. | |
An optimized polymerization condition has been selected based on our earlier study, in order to get the most stable colloidal solution.5a These conditions include the selection of the right acryl monomers, their concentration with respect to nanoparticles and the reaction time. We have used a mixture of three acryl monomers such as poly(ethylene glycol) methacrylate (PEG-methacrylate, Mn ∼ 360), N-(3-aminopropyl) methacrylamide and N,N′-methylenebisacrylamide (in 5–10 mol %). The PEG-methacrylate provides PEG functionality, N-(3-aminopropyl) methacrylamide provides primary amine functionality and N,N′-methylenebisacrylamide provides cross-linking in the polymer backbone. All three acryl monomers are highly water soluble and thus their polymeric coating assists in the high water solubility of the coated particle. We have shown earlier that if only N-(3-aminopropyl) methacrylamide is used for polymerization, the coated particles become highly water soluble but precipitate in basic solutions; when this monomer is used along with PEG-methacrylate, the water solubility of the particles occurs over a wider pH range.5a,c In the polymerization condition acryl monomers are used in high concentration so that they can efficiently replace the hydrophobic surfactant from the nanoparticle surface during the polymerization. In addition the polymerization was stopped before completion (within one hour) in order to prevent particle aggregation and gel formation.
MQD characterization
The as-synthesized γ-Fe2O3 and CdSe–ZnS nanoparticles have a hydrophobic surfactant coating that makes them water insoluble. However, water soluble nanoparticles are produced after the polyacrylate coating step. FTIR study has been performed before and after coating which shows that the original hydrophobic surfactants from the γ-Fe2O3 and CdSe–ZnS nanoparticle surface are replaced by acrylate polymer.† In order to determine the composite nature of particle, TEM study has been performed after coating. It shows the presence of both γ-Fe2O3 and CdSe–ZnS, predominantly discrete γ-Fe2O3 particles surrounded by several CdSe–ZnS, suggesting the formation of the desired MQD structures (Fig. 1). However, some isolated γ-Fe2O3/CdSe–ZnS and non-uniform MQDs are also observed, suggesting that MQD organization deviates from the idealized structure shown in Scheme 1. This deviation is due to random occupation/distribution of component particles in the reverse micelle during MQD formation steps. The hydrodynamic diameter of these MQD as determined by dynamic light scattering (DLS) study shows the overall size between 50–80 nm. The large hydrodynamic diameter compared to TEM size is due to non-uniform coating along with dangling polymer chain ends in an aqueous medium.
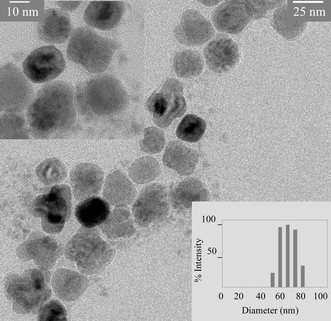 |
| Fig. 1 A representative transmission electron microscopic (TEM) image of MQD structure showing the presence of both 25 nm size γ-Fe2O3 and 3 nm size green flourescent CdSe–ZnS components. Inset: high resolution TEM image and DLS size of MQD. | |
In order to know the magnetic properties of γ-Fe2O3 in composite nanoparticle, the MQD system has been examined in detail.† The hysteresis curve for the MQD and hydrophobic γ-Fe2O3 show superparamagnetic properties at room temperature and exhibit hysteresis at 5 K with coercivities of 879 Oe and 232 Oe, respectively. At 300 K, the saturation magnetization values for MQD and hydrophobic γ-Fe2O3 are 1.36 emu g−1 and 49.67 emu g−1, respectively. The relatively low magnetization value for MQD is attributed to the presence of polymers and QD. The zero field cooled (ZFC) and field cooled (FC) magnetization curves show distinct blocking temperatures of 54 K and 48 K (measured at 100 Oe) for MQD and hydrophobic γ-Fe2O3, respectively, which are the characteristics of superparamagnetism.
The aqueous dispersion of MQD shows the presence of strong fluorescence and magnetic property, further suggesting that they have both CdSe–ZnS and γ-Fe2O3 components (Fig. 2). Depending on the size of CdSe used, MQDs show green, yellow or red fluorescence and the fluorescence quantum yield (QY) varies between 8–20%. In general, the QY of CdSe–ZnS decreases by 20–30% after it becomes a component of MQD. Although this type of quenching effect is commonly reported,2b,e,i,j it is much lower in the present case due to the ZnS shell around CdSe as well as a polymer layer around each component particle that increases the separation distance.
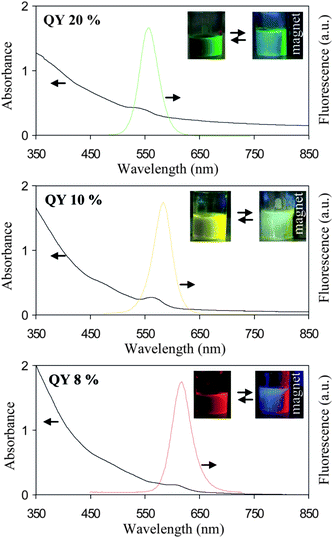 |
| Fig. 2 Absorption and fluorescence spectra of three different types of aqueous MQD solutions composed of 10 nm γ-Fe2O3 and three different sizes of CdSe–ZnS. Inset: typical digital picture of stable colloidal solution and their magnetic response in presence of excesses Na2HPO4. The reversible sign (⇆) indicates that salt induced aggregation is reversible i.e. the precipitate can be redispersed in fresh water. | |
Colloidal stability of MQDs and their functionalization
Although MQDs are highly water soluble they precipitate at high basic pH (>11) or in high salt concentration and precipitated particles are attracted by a magnet. Magnetically separated MQDs can be redispersed in water after adjusting the pH or removing salt by dialysis. This type of reversible precipitation–redispersion can be repeated more than 10 times, suggesting that polymer coated MQDs structures are very robust in nature. The colloidal stability of MQDs is further tested in different aqueous buffer solutions of varied pH (Fig. 3). The result shows that coated MQDs are stable in common buffers for more than a month. This superior colloidal stability of MQDs results from the properties of polyacrylate and the optimized polymerization condition used for coating.
Polymer coated MQDs can be further functionalized with desired chemical/biochemical materials using the primary amine present at their surface. The presence of primary amine on MQD surface has been confirmed by fluorescamine titration.†7 The functionalization of MQD has been achieved via conventional conjugation chemistries. For example, oleyl amine functionalized MQD has been synthesized using glutaraldehyde based conjugation chemistry that covalently links amine terminated MQD and oleylamine.5a Similarly, vancomycin functionalized MQD has been synthesized via EDC coupling chemistry that links a primary amine terminated MQD and a carboxylic acid group of vancomycin.8 The oleyl and vancomycin functionalized MQDs retained the fluorescence and magnetic properties and also retain good colloidal stability. This result shows that polyacrylate coated MQDs exhibit good protection toward adverse chemical (e.g. bioconjugation) and physical environments (e.g. separation, buffer stability) and can be used to prepare other functional MQDs.
In order to explore the multifunctional application potential of functionalized MQDs, they have been tested as labeling probes for different cells. It is found that functionalized MQDs can be very efficient probes for cellular labeling and labeled cells can be separated magnetically. Fig. 4 shows representative results of MQD based cellular labeling, imaging and separation. It is well known that oleyl groups target the cell membrane9 and in our earlier work we have used oleyl functionalized nanoparticles to label different cells.5 In the present case, oleyl functionalization of MQD induces the cellular targeting and uptake of MQD, and the labeling of MQDs is observed via fluorescence microscopic imaging (Fig. 4). Similarly, vancomycin functionalized MQDs selectively label gram-positive bacteria Bacillus subtilis, as vancomycin has binding selectivity to bacterial cell wall and labeling can be followed via fluorescence imaging. In all cases the labeled cells/bacteria can be separated using a laboratory based bar magnet, implying that MQDs can also be used for separation. Control samples having no oleyl or vancomycin do not show such labeling, suggesting that affinity ligands induce the labeling specificity without a non-specific binding problem.† Although non-specific cellular uptake of nanoparticles is commonly observed, the lower uptake of the MQDs is due to their smaller size,10 PEGylated surface11 and the use of a lower dose5c during the labeling study. Thus a small hydrodynamic diameter with PEG coating on the MQD surface helps to achieve efficient and selective cellular labeling while the strong fluorescence and magnetic properties are helpful for fluorescence imaging and magnetic separation.
Conclusion
In conclusion we report water soluble MQD probes which have high fluorescence, small hydrodynamic diameter and high colloidal stability and which are useful for fluorescence based cell imaging and magnetic cell separation applications. The key feature is the utilization of the most advanced synthetic methods in preparing high quality component magnetic and fluorescent nanomaterials separately and the exploitation of optimized in situ polyacrylate coating to derive the MQD structure. The synthetic approach is simple and applicable to milligram scale synthesis of functional MQDs of different emission colours. Different functional MQDs have been derived and used for cellular labeling and magnetic separation, demonstrating that a variety of other functional MQDs can be synthesized using this approach.
Acknowledgements
This work is financially supported by DST and DBT, Government of India. S.B. and A.S. acknowledge CSIR, India, for providing fellowships. The authors would like to thank Dr Nihar R. Jana of National Brain Research Centre (NBRC), Manesar, India, for providing a cellular imaging facility.
References
-
(a) K. T. Shimizu, W. K. Woo, B. R. Fisher, H. J. Eisler and M. G. Bawendi, Phys. Rev. Lett., 2002, 89, 117401 CrossRef CAS;
(b) A. H. Fu, C. M. Micheel, J. Cha, H. Chang, H. Yang and A. P. Alivisatos, J. Am. Chem. Soc., 2004, 126, 10832–10833 CrossRef CAS;
(c) N. Liu, B. S. Prall and V. I. Klimov, J. Am. Chem. Soc., 2006, 128, 15362–15363 CrossRef CAS;
(d) S. I. Stoeva, J.-S. Lee, J. E. Smith, S. T. Rosen and C. A. Mirkin, J. Am. Chem. Soc., 2006, 128, 8378–8379 CrossRef CAS;
(e) K. Ray, R. Badugu and J. R. Lakowicz, J. Am. Chem. Soc., 2006, 128, 8998–8999 CrossRef CAS;
(f) T. Pons, I. L. Medintz, K. E. Sapsford, S. Higashiya, A. F. Grimes, D. S. English and H. Mattoussi, Nano Lett., 2007, 7, 3157–3164 CrossRef CAS;
(g) J. Jiang, H. Gu, H. Shao, E. Devlin, G. C. Papaefthymiou and J. Y. Ying, Adv. Mater., 2008, 20, 4403–4407 CrossRef CAS;
(h) A. Gole, N. Agarwal, P. Nagaria, M. D. Wyatt and C. J. Murphy, Chem. Commun., 2008, 6140–6142 RSC;
(i) Y. Fu, J. Zhang and J. R. Lakowicz, Chem. Commun., 2009, 313–315 RSC;
(j) M. Spuch-Calvar, L. Rodriguez-Lorenzo, M. P. Morales, R. A. Alvarez-Puebla and L. M. Liz-Marzan, J. Phys. Chem. C, 2009, 113, 3373–3377 CrossRef CAS;
(k) Q. Huo, J. Liu, L. Q. Wang, Y. Jiang, T. N. Lambert and E. Fang, J. Am. Chem. Soc., 2006, 128, 6447–6453 CrossRef CAS;
(l) D. Niu, Y. Li, Z. Ma, H. Diao, J. Gu, H. Chen, W. Zhao, M. Ruan, Y. Zhang and J. Shi, Adv. Funct. Mater., 2010, 20, 773–780 CrossRef CAS.
-
(a) D. Wang, J. He, N. Rosenzweig and Z. Rosenzweig, Nano Lett., 2004, 4, 409–413 CrossRef CAS;
(b) D. K. Yi, S. T. Selvan, S. S. Lee, G. C. Papaefthymiou, D. Kundaliya and J. Y. Ying, J. Am. Chem. Soc., 2005, 127, 4990–4991 CrossRef CAS;
(c) T. R. Sathe, A. Agrawal and S. Nie, Anal. Chem., 2006, 78, 5627–5632 CrossRef CAS;
(d) S. T. Selvan, P. K. Patra, C. Y. Ang and J. Y. Ying, Angew. Chem., Int. Ed., 2007, 46, 2448–2452 CrossRef CAS;
(e) J. Gao, B. Zhang, Y. Gao, Y. Pan, X. Zhang and B. Xu, J. Am. Chem. Soc., 2007, 129, 11928–11935 CrossRef CAS;
(f) J.-H. Park, G. von Maltzahn, E. Ruoslahti, S. N. Bhatia and M. J. Sailor, Angew. Chem., Int. Ed., 2008, 47, 7284–7288 CrossRef CAS;
(g) J. Kim, H. S. Kim, N. Lee, T. Kim, H. Kim, T. Yu, I. C. Song, W. K. Moon and T. Hyeon, Angew. Chem., Int. Ed., 2008, 47, 8438–8441 CrossRef CAS;
(h) V. Roullier, F. Grasset, F. Boulmedais, F. Artzner, O. Cardor and V. Marchi-Artzner, Chem. Mater., 2008, 20, 6657–6665 CrossRef CAS;
(i) S. Deka, A. Falqui, G. Bertoni, C. Sangregorio, G. Poneti, G. Morello, M. D. Giorgi, C. Giannini, R. Cingolani, L. Manna and P. D. Cozzoli, J. Am. Chem. Soc., 2009, 131, 12817–12828 CrossRef CAS;
(j) Z.-Q. Tian, Z.-L. Zhang, J. Gao, B.-H. Huang, H.-Y. Xie, M. Xie, H. D. Abruna and D.-W. Pang, Chem. Commun., 2009, 4025–4027 RSC;
(k) C. Y. Ang, L. Giam, Z. M. Chan, A. W. H. Lin, H. Gu, E. Devlin, G. C. Papaefthymiou, S. T. Selvan and J. Y. Ying, Adv. Mater., 2009, 21, 869–873 CrossRef CAS;
(l) A. Saha, S. Basiruddin, N. Pradhan and N. R. Jana, Langmuir, 2010, 26, 4351–4356 CrossRef CAS.
- J. J. Li, Y. A. Wang, W. Guo, J. C. Keay, T. D. Mishima, M. B. Johnson and X. Peng, J. Am. Chem. Soc., 2003, 125, 12567–12575 CrossRef CAS.
- N. R. Jana, Y. Chen and X. Peng, Chem. Mater., 2004, 16, 3931–3935 CrossRef CAS.
-
(a) A. Saha, S. Basiruddin, R. Sarkar, N. Pradhan and N. R. Jana, J. Phys. Chem. C, 2009, 113, 18492–18498 CrossRef CAS;
(b) S. Basiruddin, A. Saha, N. Pradhan and N. R. Jana, J. Phys. Chem. C, 2010, 114, 11009–11017 CrossRef CAS;
(c) S. J. Tan, N. R. Jana, S. Gao, P. K. Patra and J. Y. Ying, Chem. Mater., 2010, 22, 2239–2247 CrossRef CAS.
- S. Lipgens, D. Schubel, L. Schlicht, J.-H. Spilgies and G. Ilgenfritz, Langmuir, 1998, 14, 1041–1049 CrossRef CAS.
- S. Udenfriend, S. Stein, P. Bohlen, W. Dairman, W. Leimgruber and M. Weigele, Science, 1972, 178, 871–872 CAS.
- A. J. Kell, G. Stewart, S. Ryan, R. Peytavi, M. Boissinot, A. Huletsky, M. G. Bergeron and B. Simard, ACS Nano, 2008, 2, 1777–1788 CrossRef CAS.
-
(a) S. Pujals, J. Fernández-Carneado, C. López-Iglesias, M. J. Kogan and E. Giralt, Biochim. Biophys. Acta, Biomembr., 2006, 1758, 264–279 CrossRef CAS;
(b) L. Wasungu and D. Hoekstra, J. Controlled Release, 2006, 116, 255–264 CrossRef CAS.
-
(a) F. Osaki, T. Kanamori, S. Sando, T. Sera and Y. Aoyama, J. Am. Chem. Soc., 2004, 126, 6520–6521 CrossRef CAS;
(b) J. Rejman, V. Oberle, I. S. Zuhorn and D. Hoekstra, Biochem. J., 2004, 377, 159–169 CrossRef CAS;
(c) C. J. Ackerson, P. D. Jadzinsky, G. J. Jensen and R. D. Kornberg, J. Am. Chem. Soc., 2006, 128, 2635–2640 CrossRef CAS;
(d) H. S. Choi, B. I. Ipe, P. Misra, J. H. Lee, M. G. Bawendi and J. V. Frangioni, Nano Lett., 2009, 9, 2354–2359 CrossRef CAS.
- E. L. Bentzen, I. D. Tomlinson, J. Mason, P. Gresch, M. R. D. Warnement, D. Wright, E. Sanders-Bush, R. Blakely and S. J. Rosenthal, Bioconjugate Chem., 2005, 16, 1488–1494 CrossRef CAS.
|
This journal is © The Royal Society of Chemistry 2010 |