DOI:
10.1039/B9NR00407F
(Paper)
Nanoscale, 2010,
2, 755-762
Quantitative evaluation of the effect of poly(amidoamine) dendrimers on the porosity of epithelial monolayers
Received
(in Cambridge, MA, USA)
11th December 2009
, Accepted 23rd January 2010
First published on
17th March 2010
Abstract
Poly(amidoamine) (PAMAM) dendrimers are a family of water-soluble polymers with a characteristic tree-like branching architecture and a large number of surface groups, which have been used to immobilize a variety of therapeutic molecules for targeted drug delivery. Earlier studies showed that small cationic PAMAM-NH2 and selected anionic PAMAM-COOH dendrimers permeate across in vitro models of the small intestinal epithelium by paracellular and transcellular transport mechanisms. The focus of this research is to mathematically calculate the effect of cationic, anionic, and neutral PAMAM dendrimers on the porosity of epithelial tight junctions as a function of dendrimers concentration, incubation time, generation number, and charge density. Results show that the increase in the concentration, incubation time and generation number of cationic G0–G2 PAMAM-NH2 and anionic G2.5 and G3.5 PAMAM-COOH dendrimers caused a corresponding increase in the porosity of Caco-2 cell monolayers. Neutral G2–G4 PAMAM-OH dendrimers had no effect on the porosity of intestinal cells. These results provide quantitative evidence that the observed increase in permeability of PAMAM dendrimers across Caco-2 cell monolayers is due to their effect on the organization of the tight junctions and the associated increase in membrane porosity. Furthermore, these results emphasize the potential of cationic PAMAM-NH2 and anionic PAMAM-COOH dendrimers to function as carriers for controlled oral drug delivery.
1 Introduction
Oral delivery is the most desirable route for drug administration due to higher patient compliance and lower healthcare cost compared to other routes.1 However, oral absorption of polymeric drug delivery systems such as polymeric particles and polymer-drug conjugates remains a significant challenge.2,3 Currently, oral drug delivery using polymeric carriers is limited to mucosal vaccination where natural or synthetic polymers encapsulate different antigens forming stable micro- or nano-particles, which are recognized, internalized, and processed by Peyer's patches in the lower ileum to induce a systemic and/or mucosal immune response.4 To date, there are no polymeric drug delivery systems that are effectively absorbed from the gastrointestinal tract. This can be attributed to the large size and molecular weight of the polymeric carriers, which hinder their diffusion across the intestinal epithelium into the systemic blood circulation.5
Poly(amidoamine) (PAMAM) dendrimers are a family of water-soluble polymers that is characterized by a unique tree-like branching architecture and a compact spherical geometry in solution (Table 1 & Fig. 1).6 The potential of PAMAM dendrimers in controlled drug delivery stems from their large number of surface groups, which can be utilized to immobilize drugs, enzymes, antibodies or other bioactive agents providing a high density of biological molecules in a compact therapeutic system.6,7 For example, phospholipids were conjugated to the NH2 surface groups of generation 4 (G4) to prepare a hydrophilic-hydrophobic core-shell structure that successfully entrapped the 5-fluoronracil anticancer drug.8 This formulation showed a sustained release of the encapsulated drug in vitro and enhanced its oral absorption via the lymphatic system in vivo.8 PAMAM dendrimers have also been used to enhance the oral absorption of drug molecules that are substrates of the P-glycoprotein (P-gp) efflux pump.9,10 Several PAMAM-drug conjugates proved to enhance the aqueous solubility and oral absorption of the incorporated drugs both in vitro and in vivo by escaping the P-gp efflux pump.11,12 Similarly, physical mixtures of doxorubicin with the cationic PAMAM-NH2 (G3) dendrimers showed enhanced uptake by Caco-2 cell monolayers and increased oral bioavailability in vivo.13
Probe |
Molecular Weighta/Da |
Radiusa/Å |
Number of Surface Groupsa |
Surface Groupa |
Valenceb |
Diffusion Coefficientc at 37 °C (×106 cm2s−1) |
Reported by Tomalia et al.6 and Dubin et al.31
Number of ionized surface groups calculated using the Henderson-Hasselbalch equation.26
The diffusion coefficient is calculated based on Stokes–Einstein equation.20
|
Mannitol |
182 |
4.1 |
— |
— |
— |
6.20 |
G0-NH2 |
517 |
15 |
4 |
NH2 |
1 |
1.70 |
G1-NH2 |
1430 |
22 |
8 |
NH2 |
2 |
1.15 |
G2-NH2 |
3256 |
29 |
16 |
NH2 |
4 |
0.88 |
G3-NH2 |
6909 |
36 |
32 |
NH2 |
7 |
0.71 |
G4-NH2 |
14,215 |
45 |
64 |
NH2 |
14 |
0.56 |
G2-OH |
3272 |
29 |
16 |
OH |
— |
0.88 |
G3-OH |
6941 |
36 |
32 |
OH |
— |
0.71 |
G4-OH |
14,279 |
45 |
64 |
OH |
— |
0.56 |
G-0.5-COOH |
436 |
— |
4 |
COOH |
1 |
— |
G0.5-COOH |
1269 |
9.2 |
8 |
COOH |
2 |
2.76 |
G1.5-COOH |
2935 |
12.8 |
16 |
COOH |
4 |
1.98 |
G2.5-COOH |
6267 |
14.7 |
32 |
COOH |
7 |
1.73 |
G3.5-COOH |
12,931 |
24.5 |
64 |
COOH |
14 |
1.04 |
G4.5-COOH |
26,258 |
31.0 |
128 |
COOH |
28 |
0.82 |
 |
| Fig. 1 Schematic drawing showing the tree-like branching architecture of G0–G2 of PAMAM-NH2 dendrimers. | |
Earlier studies by El-Sayed and co-workers established a clear relationship between the size, molecular weight, surface chemistry, and net charge of PAMAM dendrimers and their transport across Caco-2 cell monolayers.14–16 These studies showed that small cationic PAMAM-NH2 dendrimers (G0–G2) exhibit moderate to high permeability across Caco-2 cell monolayers, which increased with the increase in dendrimers concentration, incubation time, and generation number.14 The decline in the transepithelial electrical resistance (TEER) coupled with the increase in mannitol's paracellular permeability across Caco-2 cell monolayers indicated the effect of cationic G0–G2 on the integrity of the epithelial tight junctions.14,15 Anionic G2.5 and G3.5 PAMAM-COOH dendrimers caused an increase in mannitol permeability and a decrease in TEER values across Caco-2 cell monolayers compared to neutral PAMAM-OH dendrimers, which exhibited no effect on the integrity of Caco-2 cells.15 These results indicated that cationic G0–G2 and anionic G2.5 and G3.5 dendrimers can be utilized as polymeric carriers for oral drug delivery. Subsequent studies focused on elucidating the mechanism(s) of transport of PAMAM dendrimers across the intestinal epithelium. Microscopic studies revealed that fluorescently-labeled cationic (G2 and G4) and anionic (G1.5 and G3.5) dendrimers permeate across Caco-2 cell monolayers by a combination of paracellular transport that is associated with reorganization of tight junction proteins16,17 and transcellular transport via endocytosis.17,18 Earlier studies have established the relationship between paracellular permeability and the changes in pore size of intercellular tight junctions using small molecular weight molecules.19–22 In this manuscript, we use a similar approach to mathematically calculate the changes in porosity of Caco-2 cell monolayers as a function of concentration, incubation time, generation number, surface chemistry, and net charge of PAMAM dendrimers to quantitatively delineate the effect of PAMAM dendrimers on paracellular diffusion across intestinal epithelial barriers.
2 Experimental
2.1 Materials
Cationic PAMAM-NH2 (G0–G4), anionic PAMAM–COOH (G-0.5–G4.5), and neutral PAMAM–OH (G2–G4) dendrimers described in this article have an ethylene diamine core and were purchased from Sigma-Aldrich Co. (St. Louis, MO) as reported earlier.14,15,23
2.2 Caco-2 cell culture
The procedures for Caco-2 cell culture and development of confluent monolayers are described in details elsewhere.14,15 Briefly, Caco-2 cells were grown at 37 °C in T-75 flasks in an atmosphere of 5% CO2 and 95% relative humidity using Dulbecco's Modified Eagle's Medium (pH 7.4) supplemented with 10% fetal bovine serum, 1% non-essential amino acids, and 0.05% penicillin–streptomycin with frequent medium change every 48 h. Caco-2 cells (passages 30–70) were seeded at 6.3 × 104 cells cm−2 on polycarbonate 6-well or 12-well Transwell® filters (Corning Costar Corporation, Cambridge, MA) (3.0 mm mean pore size) under the same incubation conditions and used for transport experiments 21–28 days after seeding.
2.3 Effect of PAMAM dendrimers on mannitol permeability across Caco-2 cell monolayers
Collection of the experimental data used in this study was done following published procedures.14,15 Briefly, the apical to basolateral permeability of [14C] mannitol (3.2 mM) across Caco-2 cell monolayers was investigated in triplicate at donor concentrations of 0.1, 1.0, and 10.0 mM PAMAM dendrimers compared to control [14C] mannitol permeability in blank HBSS. Permeability experiments were conducted at pH 7.0, 37 °C, 5% CO2, 95% relative humidity, and shaking at 50 rotations per minute while maintaining sink conditions. Samples were collected at 30, 60, 90, 120, 150, 180, and 210 min where the 30-min sample was discarded to ensure steady-state transport. The integrity of Caco-2 cell monolayers was also monitored by measuring TEER before and after each experiment. Samples were analyzed by liquid scintillation counting (Beckman Coulter, Fullerton, CA). The monolayer permeability (Pm) of [14C] mannitol, in the presence and absence of PAMAM dendrimers, was calculated as previously reported.14,15,23
2.4 Caco-2 permeability of PAMAM dendrimers
The monolayer permeability (Pm) of fluorescently-labeled PAMAM-NH2 dendrimers (G0–G2) used in this study is based on our earlier reports.14 Briefly, apical to basolateral transport of fluorescently-labeled G0–G2 dendrimers across Caco-2 cell monolayers was investigated in triplicate at donor concentrations of 1.0 mM (6-well Transwells®, 4.7 cm2 surface area) and 10.0 mM (12-well Transwells®, 1.0 cm2 surface area). Permeability experiments were conducted at pH 7.0, 37 °C, 5% CO2, 95% relative humidity, and shaking at 50 rotations per minute while maintaining sink conditions. Samples were collected at 30, 90, 150, and 210 min where the 30-min sample was discarded to ensure steady-state transport. The integrity of Caco-2 cell monolayers was monitored by measuring TEER before and after each experiment.
2.5 The relationship between porosity and the paracellular permeability across Caco-2 cell monolayers
Electron micrographs of the paracellular space in Caco-2 monolayers show the presence of two barriers in series; the tight junctions (length < 0.1 μm) followed by a relatively long and tortuous lateral space (∼ 20 μm cell height and ∼75 Å gap width with a tortuosity factor of 2.0–2.5).24 Since the radius of the tight junction is 12 Å and the gap width of the tortuous lateral space is approximately 75 Å, the tight junction is considered the rate-limiting barrier for diffusion of different molecules across the paracellular space.25 We hypothesize that the observed increase in mannitol paracellular permeability across Caco-2 cell monolayers upon incubation with selected cationic and anionic PAMAM dendrimers is due to changes in the conformation of the tight junctions associated with an increase in the size of tight junctional pores. We are using the Renkin function for cylindrical channels (eqn (1)),25 which compares the molecular radius (r) of the diffusing probe to the radius of the paracellular pores (R) to mathematically calculate the changes in tight junctional pore size in response to PAMAM dendrimers. |  | (1) |
Using the Renkin function equation, we calculated the changes in porosity of Caco-2 cell monolayers upon incubation with cationic G0–G4, neutral G2–G4, and anionic G-0.5–G4.5 PAMAM dendrimers reflected by the observed changes in mannitol paracellular permeability.14,15
2.6 Calculation of porosity and Renkin function of Caco-2 cell monolayers
The paracellular permeability coefficient (Pp) of the diffusing mannitol molecules is given by the following equation:20 |  | (2) |
Where ε is the porosity or volume fraction of pores, δ = τ × L is the tortuousity (τ) times the path length across the Caco-2 cell monolayer (L, μm), D (cm2 s−1) is solutes diffusion coefficient in water at 37 °C, and
is the Renkin function. Eqn (2) was reorganized to calculate porosity and Renkin function (eqn (3)). |  | (3) |
We calculated the aqueous diffusion coefficient (D) of mannitol using the Stokes–Einstein relationship (eqn (4)).20 |  | (4) |
Where K is the Boltzmann's constant (
), T is the absolute temperature used for permeability measurements and matches physiological temperature (310 K), η is the viscosity of water at 37 °C (
), which is the main constituent of the HBSS buffer used in the transport experiments, and r is the radius of diffusing mannitol molecules (4.1 × 10−10m). We used our published mannitol permeability data to calculate the changes in porosity and Renkin function of Caco-2 cell monolayers as a function of PAMAM concentration, incubation time, generation number, and net surface charge.14,15
2.7 Calculation of porosity and Renkin function of Caco-2 cell monolayers based on PAMAM-NH2 permeability
The permeability coefficient (P+p) of cationic molecules such as PAMAM-NH2 is given by the following equation: |  | (5) |
By reorganizing eqn (5) to calculate porosity and Renkin function (eqn (6)):
|  | (6) |
We used
eqn (7) to calculate
κ, which is a dimensionless electrochemical energy function across the
tight junction pores.
|  | (7) |
Where
e is the unit charge of an ion (1.602 × 10
−19Coulombs),
z is the valence of the diffusing ions, |Δ
ψ|is the net electrical potential across the
tight junction,
K is the Boltzmann's constant (
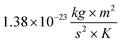
), and
T is the absolute temperature used for permeability measurements and matches physiological temperature (310 K).
To solve for z, we calculated the valence of different PAMAM-NH2 generations using the Henderson-Hasselbalch equation (eqn (8)).26
| 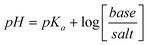 | (8) |
We used the TEER values collected experimentally to calculate |Δψ| using eqn (9):
| |Δψ| = V = IR = I × (TEER × A) | (9) |
Where
V is the voltage (volts),
I is the current (Amp),
R is the resistance (
Ω),
TEER is the transepithelial electrical resistance (Ω.cm
2), and
A is the surface area of Caco-2
cell monolayers (cm
2).
3 Results and discussion
3.1 Calculation of effective porosity of Caco-2 cell monolayers based on mannitol permeability
Our data shows that the effective porosity of Caco-2 cell monolayers, which is the product of Renkin function [
] and the volume fraction of the paracellular pores [
] remained constant for unperturbed monolayers (Fig. 2). In comparison, the incubation of Caco-2 cell monolayers with 0.1 and 1.0 mM G0-NH2 caused an increase in monolayer's effective porosity at an incubation time of 210 min, whereas 10 mM G0-NH2 caused an increase in effective porosity at all incubation time points (p < 0.005) (Fig. 2, Panel A). The incubation of Caco-2 cell monolayers with 0.1, 1.0, and 10.0 mM of G1-NH2 caused an increase in monolayer's effective porosity at all incubation time points compared to baseline porosity values (p < 0.005) (Fig. 2, Panel B). Similarly, the incubation of Caco-2 cell monolayers with 1.0 and 10.0 mM of G2-NH2 resulted in a significant increase (p < 0.005) in monolayer's effective porosity at 150, 180, and 210 min incubation times (Fig. 2, Panel C). G3-NH2 produced a significant increase in the effective porosity of Caco-2 cell monolayers at all concentrations and incubation time points (Fig. 2, Panel D). However, the observed increase in effective porosity at the incubation time point of 210 min may be a result of polymer's toxicity. G4-NH2 produced a significant increase in monolayer's effective porosity at all concentrations and incubation time points, which can be a result of the associated polymer's toxicity (Fig. 2, Panel E). These results collectively indicate that cationic PAMAM-NH2 dendrimers (G0–G4) increase the effective porosity of Caco-2 cell monolayers in a concentration-, incubation time-, and generation-dependent fashion.
![The relationship between the incubation time and the porosity [] of unperturbed Caco-2 cell monolayers (♦) and the monolayers incubated with 0.1 mM (■), 1.0 mM (▲) and 10 mM (●) of G0-NH2 (panel A), G1-NH2 (panel B), G2-NH2 (panel C), G3-NH2 (panel D), and G4-NH2 (panel E) dendrimers. Each data point represents the average ± standard error of the mean of three independent measurements. T denotes the toxicity of PAMAM-NH2 dendrimers at a specific concentration and incubation time point. The porosity of Caco-2 cell monolayers upon incubation with different concentrations of PAMAM-NH2 dendrimers is compared to that of the unperturbed monolayer at the same incubation time point using student t-test where *, **, and *** denote p ≤ 0.05, 0.01, and 0.005, respectively.](/image/article/2010/NR/b9nr00407f/b9nr00407f-f2.gif) |
| Fig. 2 The relationship between the incubation time and the porosity [ ] of unperturbed Caco-2 cell monolayers (♦) and the monolayers incubated with 0.1 mM (■), 1.0 mM (▲) and 10 mM (●) of G0-NH2 (panel A), G1-NH2 (panel B), G2-NH2 (panel C), G3-NH2 (panel D), and G4-NH2 (panel E) dendrimers. Each data point represents the average ± standard error of the mean of three independent measurements. T denotes the toxicity of PAMAM-NH2 dendrimers at a specific concentration and incubation time point. The porosity of Caco-2 cell monolayers upon incubation with different concentrations of PAMAM-NH2 dendrimers is compared to that of the unperturbed monolayer at the same incubation time point using student t-test where *, **, and *** denote p ≤ 0.05, 0.01, and 0.005, respectively. | |
Anionic PAMAM-COOH dendrimers increased the effective porosity of Caco-2 cell monolayers in a concentration- and generation number-dependent fashion (Fig. 3). The smallest PAMAM-COOH dendrimers (G-0.5) caused no change in monolayer's porosity, whereas larger G0.5 and G1.5 caused a statistically significant increase in effective porosity at a concentration 10.0 mM and incubation times 150, 180, and 210 min, which can be attributed to their observed toxicity at these concentration and incubation times (Fig. 3, Panels A–C). G2.5 and G3.5 caused an increase in monolayer's effective porosity at short non-toxic incubation times (60, 90, and 120 min) and to a greater extent at longer incubation times, which proved to be toxic to Caco-2 cells (Fig. 3, Panels D & E). In comparison, G4.5 increased the effective porosity of Caco-2 cell monolayers only at toxic concentrations and incubation time points (Fig. 3, Panel F). The observed increase in effective porosity with anionic G2.5 and G3.5 PAMAM-COOH dendrimers at non-toxic concentrations and incubation time points can be attributed to their negative charge, which allows them to act as efficient Ca+2 ion chelators thus reducing the integrity of the tight junctions.
![Relationship between the incubation time and the porosity [] of unperturbed Caco-2 cell monolayers (♦) and those incubated with 0.1 mM (■), 1.0 mM (▲) and 10 mM (●) of G-0.5-COOH (panel A), G0.5-COOH (panel B), G1.5-COOH (panel C), G2.5-COOH (panel D), G3.5-COOH (panel E), and G4.5-COOH (panel F) dendrimers. Each data point represents the average ± standard error of the mean of three independent measurements. T denotes the toxicity of PAMAM-COOH dendrimers at a specific concentration and incubation time point. The porosity of Caco-2 cell monolayers upon incubation with different concentrations of PAMAM-COOH dendrimers is compared to that of the unperturbed monolayer at the same incubation time point using student t-test where *, **, and *** denote p ≤ 0.05, 0.01, and 0.005, respectively.](/image/article/2010/NR/b9nr00407f/b9nr00407f-f3.gif) |
| Fig. 3 Relationship between the incubation time and the porosity [ ] of unperturbed Caco-2 cell monolayers (♦) and those incubated with 0.1 mM (■), 1.0 mM (▲) and 10 mM (●) of G-0.5-COOH (panel A), G0.5-COOH (panel B), G1.5-COOH (panel C), G2.5-COOH (panel D), G3.5-COOH (panel E), and G4.5-COOH (panel F) dendrimers. Each data point represents the average ± standard error of the mean of three independent measurements. T denotes the toxicity of PAMAM-COOH dendrimers at a specific concentration and incubation time point. The porosity of Caco-2 cell monolayers upon incubation with different concentrations of PAMAM-COOH dendrimers is compared to that of the unperturbed monolayer at the same incubation time point using student t-test where *, **, and *** denote p ≤ 0.05, 0.01, and 0.005, respectively. | |
Surface chemistry and the associated charge density of PAMAM dendrimers affect the effective porosity of Caco-2 cell monolayers. Despite the similarity in size and molecular weight of G2–G4 of PAMAM-OH dendrimers and PAMAM-NH2 dendrimers, the change from NH2 to OH surface groups affects the nature of their interaction with Caco-2 cell monolayers. At physiologic pH of 7.4, approximately 22% of the primary NH2 surface groups will be ionized and carry a positive charge (Table 1) resulting in an electrostatic interaction with the negatively charged epithelium of Caco-2 cells, which results in modulation of the tight junctions and the observed increase in monolayer's effective porosity. In comparison, neutral PAMAM-OH dendrimers (G2–G4) caused no significant changes in the effective porosity of Caco-2 cell monolayers (Fig. 4). These results collectively indicate that the interaction of PAMAM dendrimers with Caco-2 cell monolayers is generation, size, molecular weight, and charge dependent.
![Relationship between the incubation time and the porosity [] of unperturbed Caco-2 cell monolayers (♦) and those incubated with 0.1 mM (■), 1.0 mM (▲) and 10 mM (●) of G2-OH (panel A), G3-OH (panel B), and G4-OH (panel C) dendrimers. Each data point represents the average ± standard error of the mean of three independent measurements. T denotes the toxicity of PAMAM-OH dendrimers at a specific concentration and incubation time point. The porosity of Caco-2 cell monolayers upon incubation with different concentrations of PAMAM-OH dendrimers is compared to that of the unperturbed monolayer at the same incubation time point using student t-test where *, **, and *** denote p ≤ 0.05, 0.01, and 0.005, respectively.](/image/article/2010/NR/b9nr00407f/b9nr00407f-f4.gif) |
| Fig. 4 Relationship between the incubation time and the porosity [ ] of unperturbed Caco-2 cell monolayers (♦) and those incubated with 0.1 mM (■), 1.0 mM (▲) and 10 mM (●) of G2-OH (panel A), G3-OH (panel B), and G4-OH (panel C) dendrimers. Each data point represents the average ± standard error of the mean of three independent measurements. T denotes the toxicity of PAMAM-OH dendrimers at a specific concentration and incubation time point. The porosity of Caco-2 cell monolayers upon incubation with different concentrations of PAMAM-OH dendrimers is compared to that of the unperturbed monolayer at the same incubation time point using student t-test where *, **, and *** denote p ≤ 0.05, 0.01, and 0.005, respectively. | |
3.2 Calculation of porosity of Caco-2 cell monolayers based on permeability of PAMAM-NH2 dendrimers
Results show that the incubation of Caco-2 cell monolayers with 1.0 and 10.0 mM solutions of cationic PAMAM-NH2 dendrimers (G0–G2) increased monolayer's effective porosity in a concentration- and incubation time-dependent fashion (Fig. 5). G0-NH2 caused a significant increase in effective porosity compared to that observed with mannitol, which increased with the increase in dendrimers concentration and incubation time (Fig. 5, Panel A). Similarly, G1-NH2 caused an increase in monolayer's effective porosity that increased with the increase in dendrimers concentration from 1.0 to 10.0 mM (Fig. 5, Panel B). G2-NH2 increased monolayer's effective porosity with the increase in dendrimers concentration and incubation time (Fig. 5, Panel C). By comparing the observed changes in monolayer's effective porosity with cationic G0–G2 dendrimers, the increase in generation number did not result in a parallel increase in effective porosity. This can be attributed to the fact that PAMAM dendrimers permeate across Caco-2 cell monolayers by a combination of paracellular and transcellular transport mechanisms compared to mannitol, which is transported exclusively via the paracellular route.16,18
![Relationship between the incubation time and the porosity [] of unperturbed Caco-2 cell monolayers (♦) and those incubated with 1.0 mM (■) and 10 mM (●) of G0-NH2 (panel A), G1-NH2 (panel B), and G2-NH2 (panel C) dendrimers. Each data point represents the average ± standard error of the mean of three independent measurements. T denotes the toxicity of PAMAM-NH2 dendrimers at a specific concentration and incubation time point. The porosity of Caco-2 cell monolayers upon incubation with different concentrations of PAMAM-NH2 dendrimers is compared to that of the unperturbed monolayer at the same incubation time point using student t-test where *, **, and *** denote p ≤ 0.05, 0.01, and 0.005, respectively.](/image/article/2010/NR/b9nr00407f/b9nr00407f-f5.gif) |
| Fig. 5 Relationship between the incubation time and the porosity [ ] of unperturbed Caco-2 cell monolayers (♦) and those incubated with 1.0 mM (■) and 10 mM (●) of G0-NH2 (panel A), G1-NH2 (panel B), and G2-NH2 (panel C) dendrimers. Each data point represents the average ± standard error of the mean of three independent measurements. T denotes the toxicity of PAMAM-NH2 dendrimers at a specific concentration and incubation time point. The porosity of Caco-2 cell monolayers upon incubation with different concentrations of PAMAM-NH2 dendrimers is compared to that of the unperturbed monolayer at the same incubation time point using student t-test where *, **, and *** denote p ≤ 0.05, 0.01, and 0.005, respectively. | |
Despite the acceptance of Caco-2 cell monolayers as an in vitro model of human small intestinal epithelium, there are multiple anatomical and physiological differences between Caco-2 cell monolayers and the intestinal mucosa. For example, Caco-2 cell monolayers lack the mucus layer that lines the intestinal epithelium and serve as a diffusion barrier.27 In addition, earlier research showed that TEER measurements across Caco-2 cell monolayers are higher than those measured across human colonic epithelia, which is attributed to the narrow paracellular space (12–20 Å) and restrictive tight junctions of Caco-2 cell monolayers.28–30 Currently, the in vivo permeability of PAMAM dendrimers across the small intestinal epithelium has not been established. However, one expects cationic PAMAM-NH2 (G0–G2) and anionic PAMAM-COOH (G1.5 and G2.5) dendrimers to interact with the intestinal epithelium and increase its porosity similar to their established interaction profile with Caco-2 cell monolayers. Interactions of PAMAM dendrimers with the human intestinal epithelium will likely increase its porosity and the associated net paracellular permeability to higher values due to the wider paracellular space and less restrictive tight junctions in vivo. Our results establish a clear relationship between paracellular permeability and porosity of epithelial layers, which allows for a reliable estimation of the porosity of the human intestinal epithelium based on the permeability of marker molecules.
The established ability of selected generations of PAMAM dendrimers to modulate the integrity of the intestinal tight junctions suggests their potential as carriers for controlled oral drug delivery. However, the ability to “loosen” the intestinal tight junctions should be carefully controlled to avoid the penetration of enteric pathogens from the gastrointestinal tract to the systemic circulation. Using PAMAM dendrimers as carriers for oral drug delivery will rely on the ability to “tune” their interaction with the intestinal epithelium to achieve a contact-initiated, transient, and reversible opening of the tight junctions that allows the absorption of drug-loaded carriers while limiting the diffusion of pathogens. One approach to achieve reversible modulation of intestinal tight junctions is to control the cationic and anionic charge density of PAMAM dendrimers, which is currently under investigation.
4 Conclusions
Selected cationic PAMAM-NH2 (G0–G2) and anionic PAMAM-COOH (G2.5 and G3.5) dendrimers proved to increase the effective porosity of Caco-2 cell monolayers in a concentration-, incubation time-, surface charge, and generation-dependent fashion. This increase in monolayer's effective porosity appears to be the primary reason for the observed increase in their permeability across Caco-2 cell monolayers. These studies provide quantitative evidence of the effect of PAMAM dendrimers on the porosity of intestinal epithelial barriers, which further emphasizes their potential as drug carriers for controlled oral delivery.
References
- E. C. Lavelle, Critical Reviews in Therapeutic Drug Carrier Systems, 2001, 18, 341–386 Search PubMed.
- F. Delie and M. J. Blanco-Prieto, Molecules, 2005, 10, 65–80 Search PubMed.
- A. Leone-Bay, D. R. Paton and J. J. Weidner, Med. Res. Rev., 2000, 20, 169–186 CrossRef CAS.
- D. J. Brayden and A. W. Baird, Adv. Drug Delivery Rev., 2004, 56, 721–726 CrossRef CAS.
- D. A. Norris, N. Puri and P. J. Sinko, Adv. Drug Delivery Rev., 1998, 34, 135–154 CrossRef CAS.
- D. A. Tomalia and J. M. J. Frechet, J. Polym. Sci., Part A: Polym. Chem., 2002, 40, 2719–2728 CrossRef CAS.
- S. Svenson and D. A. Tomalia, Adv. Drug Delivery Rev., 2005, 57, 2106–2129 CrossRef CAS.
- P. K. Tripathi, A. J. Khopade, S. Nagaich, S. Shrivastava, S. Jain and N. K. Jain, Pharmazie, 2002, 57, 261–264 CAS.
- A. D'Emanuele, R. Jevprasesphant, J. Penny and D. Attwood, J. Controlled Release, 2004, 95, 447–453 CrossRef CAS.
- M. Najlah, S. Freeman, D. Attwood and A. D'Emanuele, Bioconjugate Chem., 2007, 18, 937–946 CrossRef CAS.
- M. Najlah, S. Freeman, D. Attwood and A. D'Emanuele, Int. J. Pharm., 2006, 308, 175–182 CrossRef CAS.
- M. Najlah, S. Freeman, D. Attwood and A. D'Emanuele, Int. J. Pharm., 2007, 336, 183–190 CrossRef CAS.
- Y. Z. Weilun Ke, Rongqin Huang, Chen Jiang and Yuanying Pei, Journal of Pharmaceutical Sciences, 2007 Search PubMed.
- M. El-Sayed, M. Ginski, C. Rhodes and H. Ghandehari, J. Controlled Release, 2002, 81, 355–365 CrossRef CAS.
- M. El-Sayed, M. Ginski, C. A. Rhodes and H. Ghandehari, J. Bioact. Compat. Polym., 2003, 18, 7–22 CrossRef CAS.
- M. El-Sayed, C. A. Rhodes, M. Ginski and H. Ghandehari, Int. J. Pharm., 2003, 265, 151–157 CrossRef CAS.
- K. M. Kitchens, R. B. Kolhatkar, P. W. Swaan, N. D. Eddington and H. Ghandehari, Pharm. Res., 2006, 23, 2818–2826 CrossRef CAS.
- K. M. Kitchens, R. B. Kolhatkar, P. W. Swaan and H. Ghandehari, Molecular Pharmaceutics, 2008 Search PubMed.
- A. Adson, P. S. Burton, T. J. Ruab, C. L. Barsuhn, L. Audus and N. F. H. Ho, Pharmaceutical Research, 1995, 84, 1197–1203 CAS.
- A. Adson, T. J. Raub, P. S. Burton, C. L. Barsuhn, A. R. Hilgers, K. L. Audus and N. F. H. Ho, Journal of Pharmaceutical Sciences, 1994, 83, 1529–1536 CrossRef CAS.
- G. T. Knipp, N. F. H. Ho, C. L. Barsuhn and R. T. Borchardt, J. Pharm. Sci., 1997, 86, 1105–1110 CrossRef CAS.
- R. Saitoh, K. Sugano, N. Takata, T. Tachibana, A. Higashida, Y. Nabuchi and Y. Aso, Pharm. Res., 2004, 21, 749–755 CrossRef CAS.
- F. Tajarobi, M. El-Sayed, B. D. Rege, J. E. Polli and H. Ghandehari, Int. J. Pharm., 2001, 215, 263–267 CrossRef CAS.
- D. W. Powell, American
Journal of Physiology, 1981, 241, G275–G288 CAS.
-
F. E. Curry, in Handbook of Physiology, Section 2, The Cardiovascular System, Editon edn, 1984, vol. IV, pp. 309–374 Search PubMed.
- M. El-Sayed, M. F. Kiani, M. D. Naimark, A. H. Hikal and H. Ghandehari, Pharm. Res., 2001, 18, 23–28 CrossRef CAS.
- F. Delie and W. Rubas, Critical Reviews in Therapeutic Drug Carrier Systems, 1997, 14, 221–286 Search PubMed.
- P. Artursson, A. L. Ungell and J. E. Lofroth, Pharm. Res., 1993, 10, 1123–1129 CrossRef CAS.
- A. Collett, E. Sims, D. Walker, Y. L. He, J. Ayrton, M. Rowland and G. Warhurst, Pharm. Res., 1996, 13, 216–221 CrossRef CAS.
- A. B. J. Noach, Y. Kurosaki, M. C. M. Blomroosemalen, A. G. Deboer and D. D. Breimer, Int. J. Pharm., 1993, 90, 229–237 CrossRef CAS.
- P. L. Dubin, S. L. Edwards, J. I. Kaplan, M. S. Mehta, D. Tomalia and J. L. Xia, Anal. Chem., 1992, 64, 2344–2347 CrossRef CAS.
|
This journal is © The Royal Society of Chemistry 2010 |