DOI:
10.1039/B9NR00341J
(Review Article)
Nanoscale, 2010,
2, 458-467
LbL multilayer capsules: recent progress and future outlook for their use in life sciences
Received
(in Zürich, Switzerland)
6th November 2009
, Accepted 28th December 2009
First published on
10th February 2010
Abstract
In this review we provide an overview of the recent progress in designing composite polymer capsules based on the Layer-by-Layer (LbL) technology demonstrated so far in material science, focusing on their potential applications in medicine, drug delivery and catalysis. The benefits and limits of current systems are discussed and the perspectives on emerging strategies for designing novel classes of therapeutic vehicles are highlighted.
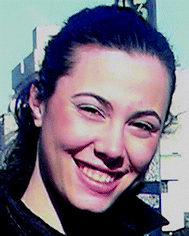 Loretta L. del Mercato | Dr Loretta Laureana del Mercato received her MSc in Biotechnology from the University “Federico II” of Naples (Italy) in 2004. In 2007 she obtained her Ph.D. in Innovative Materials and Technologies from the National Nanotechnology Laboratory (NNL) of CNR-INFM, Lecce (Italy) with a thesis on “Nanoscale characterization of synthetic polypeptides for nanobiotechnology applications”. Since February 2008, she has been a postdoctoral researcher in the Biophotonics group of Professor Wolfgang J. Parak in the Physics Department of the Philipps Universität of Marburg (Germany). Her current research interests include the synthesis, functionalization and characterization of carrier systems based on polyelectrolyte capsules for applications in the biomedical field. |
Introduction
In the last three decades nanotechnology has attracted great interest in nanomedicine. Recent reviews describe the current impact and future prospects of nanotechnology with respect to drug/gene delivery and other fields of nanomedicine such as biosensing.1–4 The main objectives in developing controlled release are avoidance of biological barriers, increase of the in vivo efficiency of drugs and targeted drug administration.1,5 In addition the development of sensitive, specific and stable sensors, which allow for real-time measurements of physiological levels of important molecular species directly in the site of disease, is highly desirable.6,7 For these purposes, a wide variety of carriers based on different methods of preparation have been developed ranging from nano-materials (such as carbon nanotubes,8,9 nanoparticles,10–13 and nanocomposites14) to biomaterials (such as dendrimers,15 liposomes,16,17 block co-polymer micelles,18 bio-degradable polymers19). These materials show some attractive properties such as small size (1 to 100 nm), chemically tailorable physical properties, tunable shape and structural robustness. Nonetheless, in the last years great attention has been focused on the development of novel “multifunctional” platforms4,20–22 which combine a variety of properties allowing for the simultaneous or sequential performance of multiple functions in single cells, including enzymatic catalysis, controlled release, directed drug delivery and sensing. The most important requirements of a multifunctional system include (i) increased longevity and stability of the carrier in the circulation, (ii) targeting to the site of the disease via both non-specific and specific mechanisms, (iii) stimuli sensitivity to the local environment of the pathological site (such as pH or temperature) or to externally applied stimuli (such as magnetic field, ultrasound, laser irradiation), (iv) enhanced intracellular delivery of the cargo/drug, (v) contrast agents for both intra-cellular imaging of the carrier and real-time measurement of certain analytes in the body.20 In this context polyelectrolyte multilayer capsules fabricated via the Layer-by-Layer (LbL) technique23 have emerged as an interesting platform for the assembly of multifunctional carrier systems.24 LbL-based hollow multilayer polyelectrolyte microcapsules consist of two distinct compartments: the multilayer shell and the cavity. The shell is built up through the consecutive adsorption of oppositely charged species around a charged spherical template and is held together due to the strong electrostatic forces that take place between each component layer.23,25 The cavity, which is obtained after removal of the sacrificial template, represents the main volume of the capsules in which chemical reactions can be performed26,27 and in which a range of materials from small molecules28,29 to macromolecules30–32 can be encapsulated, thus protecting unstable cargo from the surrounding hostile environment, beside increasing their biodistribution and solubility. Thanks to the high versatility of the LbL technique, the two compartments can be easily manipulated to create different types of active systems with respect to specific application requirements. The general properties of LbL microcapsules regarding their synthesis, loading and release,33–36 physicochemical36,37 and mechanical38 properties as well as their permeability,33,34,39 have been comprehensively reviewed by many articles in the past few years. Therefore, in this review, we first provide a short overview of the main steps involved in the preparation and functionalization of LbL-derived capsules, then we give an overview about some of the very recent progress achieved by several groups on the design of novel composite microcapsules, focusing on their use as drug delivery vehicles, intracellular sensors, and micro-reactor containers. Finally, we discuss the benefits and limits of current systems and we try to highlight the perspectives of the emerging strategies based on these systems for designing novel classes of therapeutics vehicles.
LbL-derived capsules: preparation and functionalization
LbL adsorption of oppositely charged species around submicrometre and micrometre-sized charged colloidal particles is a multi-step process which allows the fabrication of multilayer polyelectrolyte capsules for a wide range of applications. The technique is based on LbL adsorption of oppositely charged polymers on colloidal templates, followed by core dissolution.23,25Fig. 1 summarizes the main steps involved in the assembly of a multilayer polyelectrolyte capsule, based on a spherical porous template, in which more components with different functions have been combined at separate regions (cavity, wall and external surface of the capsule) to create a single object capable of performing multiple functions simultaneously.40 As can be observed, the properties of the capsules can be varied at each step employing building blocks with different properties. For example, by using templates of different diameter (from 60 nm to 10 μm), the size of the resulting capsules can be tuned,25,32,37,41 whereas by using different types of component layers, such as synthetic polyelectrolytes,25,42 charged and not charged biopolymers,43–47 the chemical properties of the multilayer shell can be tailored. Moreover the multilayer shell can be modified by simultaneously loading charged inorganic nanoparticles to yield capsules responsive to specific external stimuli.48–51 Finally the surface of the capsules can be decorated to give low-fouling capsules by the adsorption of a poly(ethylene glycol)-based layer52,53 or targeted capsules through the coupling of specific recognition elements (e.g., antibodies).54 In the following the range of materials used so far for each step of fabrication and their main properties are described.
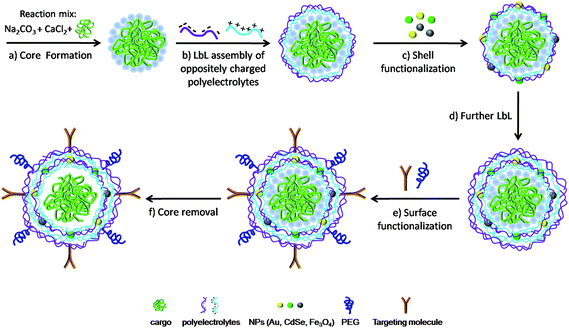 |
| Fig. 1 Schematic illustration of the synthesis of a multifunctional polyelectrolyte capsule via LbL assembly. (a) A spherical CaCO3 porous template is synthesized by mixing two solutions of Na2CO3 and CaCl2 in presence of the cargo molecules (such as drugs, genes or proteins). The cargo molecules are embedded in the pores of the template. (b) The filled CaCO3 particles are then coated via consecutive LbL deposition of oppositely charged polyelectrolytes to grow a multilayer polymer shell around the template. (c) The shell can be functionalized by loading charged NPs (such as metal, fluorescent and magnetic NPs) onto an oppositely charged layer during the LbL assembly. (d) LbL of polyelectrolytes is repeated to obtain a stable multilayer shell. (e) Additional surface functionalization to improve cellular uptake (e.g. targeting moieties) or in vivo longevity (e.g. conjugation of PEG) can be performed via electrostatic or covalent binding of molecules to the outer surface. (f) Finally the spherical template is removed to obtain a multilayer capsule with cargo molecules inside its cavity. Capsules are not drawn to scale. Only few layers of polyelectrolyte and of NPs are shown for sake of clarity. | |
The main classes of decomposable templates used for LbL assembly of capsules have been extensively reviewed in previous articles.37,39,40 Briefly, capsules have been fabricated by using organic (i.e., polystyrene latex,55 melamine formaldehyde,25 silicon dioxide37) or inorganic templates41,56,57 (i.e., MnCO3, CaCO3 CdCO3) dissolvable in acidic or aqueous solvents, respectively. The choice of the initial core influences significantly the properties of the capsules such as their size distribution and the strategy used for loading the active molecules like dyes and drugs inside the cavities.29,58,59 For instance, capsules based on organic cores are typically characterized by a good monodispersity and their cavities can be filled after the dissolution of the core by changing the permeability of the multilayer shell through variations in pH,60–62 solvent polarity,63 ionic strength64,65 or temperature59,64 (post-loading method). Instead capsules based on porous inorganic cores, such as carbonate crystals, can be directly loaded by mixing the cargo molecules with the porous templates, followed by the LbL coating with polyelectrolytes pairs (pre-loading method).32,56 Because after the dissolution process some oligomers of the original core material can partially remain adsorbed within the shell,66 capsules based on biocompatible templates, such as porous CaCO3,58 mesoporous silica32 or polylactides microparticles (PLGA, PLA),67 are typically preferred especially for biological applications.
Beside the choice of the cores, also the choice of the shell components plays a pivotal role in the LbL assembly since it directly influences the biocompatibility and degradability of the capsules inside living organisms. On one hand, capsules made of intracellular biodegradable shell components such as poly-aminoacids (i.e. poly-L-arginine), synthetic polymers (i.e. poly(hydroxypropyl)methacrylamide dimethylaminoethyl (p(HPMA-DMAE))47 or chitosan43,45,68 are very useful for biomedical applications related to the delivery of active compounds such as genes, proteins or drugs inside living organisms.69 On the other hand, biocompatible but not “easily” degradable polyelectrolytes are necessary for other applications like sensing inside cells i.e. determination of the intracellular ion concentration of different organelles.70 For such applications the synthetic bio-incompatible polylelectrolytes sodium poly(styrene sulfonate, sodium salt) (PSS), poly(allylamine hydrochloride) (PAH) and polydiallyl dimethyl ammonium chloride (PDADMAC) have been widely used up to now.59,71 The challenge for these capsule-based systems might rely on the ability of synthesizing biocompatible capsules stable enough in the different environments inside the different organelles so that they can accurately inform about the local environment of the capsules.
The capsule wall can be functionalized to impart optical, magnetic and photothermal properties to microcapsules by introducing during the LbL process inorganic charged nanoparticles (NPs)48–51 through the use of electrostatic interactions (Fig. 2). The shell modification with different types of nanoparticles allows for addressing important functions such as the labelling, the targeting and the controlled opening of the capsules which are essential for using these systems as carriers for drug delivery and sensing applications. For example, by modifying the walls of the capsules with fluorescent nanoparticles non invasive optical detection of the capsules inside living organisms can be performed.72–74 By incorporating magnetic nanoparticles to fluorescent capsules (e.g., functionalized with organic dye molecules or light-emitting quantum dots), capsules with dual-imaging functionalities, magnetic resonance (MR) imaging and luminescent properties, can be produced for their use as biomarkers in vitro and in vivo.75–77 Additionally, magnetic NPs-modified capsules can be externally manipulated using magnetic fields for directing and accumulating capsules to the target region (e.g., cancer cells) before delivering the chemotherapeutic drugs. For instance, by using Fe3O4-particle-modified capsules a specific accumulation and high local concentration of the NPs-modified capsules was observed along a magnetic field gradient and their internalization by breast cancer cells in vitro.78 Finally by embedding gold, silver and magnetic NPs into the capsule walls, the release of encapsulated drug molecules from the cavities can be achieved upon exposure to an external physical trigger such as electromagnetic or sound waves.49,50,79,80 In a recent work, the laser irradiation of gold-modified capsules has been used to locally heat the metal NPs and to perturb the integrity/permeability of the walls of the capsules inducing the release of cargo from the cavities of single capsules to the cytosol of the cells.71 Gold nanorods (Au NRs)81,82 have been also employed as absorbing elements of the capsule walls for light-controlled release of encapsulated material.83 Like Au NPs, charged Au NRs can be embedded within the multilayer shell during the LbL deposition of oppositely charged layers around the sacrificial template84 (Fig. 2b and Fig. 3b). The use of gold nanorods allows producing capsules with near-infrared absorbing properties whose opening can be induced by using laser wavelength which corresponds to the tissue transparency window (800–900 nm). Capsules with such properties might be very promising for biomedical applications. Fig. 3a shows the mechanism of photoactivated release of cargo from the cavity of a polyelectrolyte capsule containing Au NRs in the walls: laser irradiation leads to local heating of the metal nanorods and subsequent opening of small pores within the capsule wall. In Fig. 3c is reported the effect of the laser irradiation of a FITC-dextran loaded capsule with the following multilayer shell (PSS/PAH)3(PSS/AuNRs)(PAH/PSS)2. Before laser illumination, the intact capsule retains the green cargo inside the cavity (phase contrast and green channel images). During laser illumination the irradiated capsule is deformed because of the heating of the Au NRs. After switching-off the laser the capsule shell appears damaged, as it can be observed in the phase contrast image, and the partial release of the fluorescent cargo can be noticed in the corresponding fluorescent channel. Alternatively to laser irradiation methods, ultrasound treatment of gold-doped capsules has been used to mechanically disintegrate the capsule walls.85 In a different approach, high-frequency magnetic field (HFMF) has been proven to trigger the release of drugs from microcapsules prepared by loading Fe3O4 NPs into the walls.86
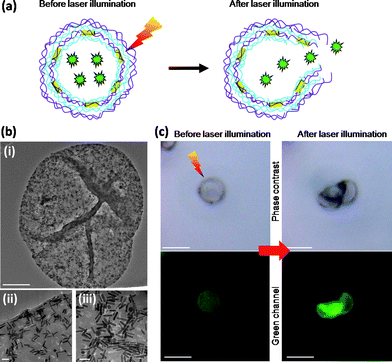 |
| Fig. 3 Laser-opening of FITC-dextran loaded capsule with Au NRs embedded in the capsule wall. (a) Schematic representation of the geometry of a capsule with green dextran as cargo (green stars) encapsulated inside the cavity and Au NRs (yellow rods) embedded in the wall: laser irradiation of Au NRs-capsule (left) leads to local heating of the metal NRs and subsequent rupture of the capsule wall (right). (b) Representative TEM image of a single Au NRs-capsule (i) (scale bar 1 μm). (ii-iii) Two high-resolution images of the multilayer wall of the capsule shown in (i) (Scale bars 50 nm). (c) Effect of near-IR laser irradiation (830 nm) of a single Au NRs-capsule loaded with FITC-dextran. Before laser illumination: the capsule retains the green cargo inside the cavity. After laser illumination: the multilayer wall of the capsule is damaged (phase contrast) and the partial release of the green cargo throughout the small pores of the wall is observed (green channel) (scale bars 5 μm). | |
Capsules made of new components as layers
Besides conventional polyelectrolytes, a variety of substances have been investigated in the last years to construct capsules through LbL assembly. Generally these capsules display improved permeability properties and especially new features such as biocompatibility, degradability and controlled destructability, making them attractive for applications in the fields of pharmacology, medicine and cosmetics etc. In this section we describe some of the recent strategies exploited by several groups to fabricate LbL-derived capsules made of new combinations of building blocks (polymeric micelles, polysaccharides, proteins, liposome and oligonucleotides) as integral components of the capsules wall.
Hollow microcapsules containing polymeric micelles in their walls have been fabricated by alternating assembly of PAH and poly(styrene-b-acrylic acid) (PS-b-PAA) micelles on MnCO3 microparticles.87 In such a system, the micelles serve as hydrophobic reservoirs with a high loading capacity in the shells while the LbL shell provides the micelles with additional support and protection. Besides their potential use as a drug delivery vehicle, these capsules may also provide an alternative option to serve as bioreactors and biosensors.87
Iron-heparin hollow sub-micrometre capsules have been fabricated by alternating deposition of oppositely charged ferric ions(III) and heparin (a highly-sulfated glycosaminoglycan widely used as anticoagulant88) onto the surface of the polystyrene latex (PS) particles, followed by removal of the PS templates by dissolution.89 The resulting capsules displayed longer anticoagulant activity in in vitro and in vivo assays compared with the same dose of an aqueous solution of heparin,89 suggesting their potential use as injectable anticoagulant vehicles in the bloodstream.
Single-component degradable capsules based on poly(methacrylic acid) (PMA) cross-linked via disulfide linkages have been assembled and loaded with the protein transferrin90 and oligonucleotides.91 These capsules undergo a reversible swelling in response to changes in external pH, and degrade in the presence of a physiological concentration of a natural thiol-containing peptide (i.e., glutathione)92 releasing the cargo molecules in a reducing environment.
Disulfide bonds (S–S) have also been employed to cross-link the shell of protein-based microcapsules leading to capsules which are destroyable by organisms and cells. For example, bovine serum albumin (BSA) mono-component hollow microcapsules were fabricated by desolvation of BSA onto MnCO3 microparticles followed by cross-linking with disulfide-containing dithiobis(succinimidylpropionate) (DSP) and subsequent core removal.93 Destruction of the BSA microcapsules was achieved under the treatment with a reductive agent (NaBH4) and with a further treatment of ultrasonication, indicating that these microcapsules are stabilized not only by S–S covalent bonds but also by other non-covalent forces (i.e., hydrophobic forces and hydrogen bonds).93 In another work, hemoglobin (Hb) microcapsules crosslinked by glutaraldehyde (GA) were fabricated through covalent LbL assembly onto MnCO3 microparticles.94 In this approach, the use of GA to crosslink proteins has been shown to lead to significant improvements in the permeability of Hb capsules in contrast to polyelectrolyte capsules.94 In a further work, CF0F1-proteoliposomes, previously prepared by incorporating the chloroplastic F0F1-ATP synthase (CF0F1-ATP) into liposomes, were mixed with a suspension of Hb capsules leading to the adsorption of lipids on the capsule surface and the assembly of CF0F1-ATP synthase onto the capsule shells. The resulting lipid-coated Hb microcapsules were successfully used to synthesize ATP.95
Enzymatic proteins have been also used as layers to grow multilayer shells capable of performing specific reactions. Qi et al., fabricated glucose-sensitive microcapsules from the LbL assembly of Hb and glucose oxidase (GOD) followed by crosslinking of the protein layers with GA.96 The formation of hydrogen peroxide (H2O2) upon processing glucose, catalyzed by GOD and Hb, indicated that the proteins were still enzymatically active after their immobilization in the multilayer. In addition, the author observed a glucose-stimulated enhancement of the wall permeability probably due to the decrease in the local pH and the loosening of the multilayer structure. In a further work, the author employed this pH dependent behaviour to control the release of insulin molecules encapsulated inside glucose-sensitive multilayer shells.97 Briefly, GOD and catalase (CAT) were assembled onto insulin particles alternately via GA cross-linking. As expected, the release ratio of insulin from the protein multilayers was observed to linearly increase in response to addition of external glucose because of the increased permeability of the capsule wall97 (Fig. 4). The described approach is interesting because it represents a proof of concept for potential applications of biocatalytic capsules sensitive to specific analytes for the controlled release of drugs.
![Controlled release of insulin from glucose-sensitive enzyme multilayer shells. (a) Schematic representation of coupled reactions of glucose oxidase (GOD) and catalase (CAT) assembled onto insulin particles followed by the enhanced permeability of the capsule for release of insulin. (b) Top: Release profiles of coated insulin particles before (black line) and after (red line) external application of glucose solution, respectively. Bottom: CLSM images of (CAT/GOD)5 microcapsules mixed with FITC–dextran (2000 kDa): (i) before and (ii) after adding glucose solution. [Adapted from ref. 97.]](/image/article/2010/NR/b9nr00341j/b9nr00341j-f4.gif) |
| Fig. 4 Controlled release of insulin from glucose-sensitive enzyme multilayer shells. (a) Schematic representation of coupled reactions of glucose oxidase (GOD) and catalase (CAT) assembled onto insulin particles followed by the enhanced permeability of the capsule for release of insulin. (b) Top: Release profiles of coated insulin particles before (black line) and after (red line) external application of glucose solution, respectively. Bottom: CLSM images of (CAT/GOD)5 microcapsules mixed with FITC–dextran (2000 kDa): (i) before and (ii) after adding glucose solution. [Adapted from ref. 97.] | |
Recently, the technologies of liposomes and LbL assembly have been combined to fabricate polyelectrolyte-coated magnetic liposomes with the aim of protecting the lipid bilayer with a polyelectrolyte multilayer shell, thus avoiding their fusion. Lately, superparamagnetic nanoparticles were encapsulated in liposomes and the resulting charged liposomes were used as templates for the stepwise LbL adsorption of the polyelectrolytes PAH and PSS. These polyelectrolyte-coated magnetic liposomes could be delivered to living cells and manipulated by applying an external magnetic field.98 In a different approach, Caruso and co-workers combined liposomes and polyelectrolyte capsules to fabricate so-called capsosomes, a hybrid platform in which the properties/advantages of the two systems are merged. The capsosomes were formed through initial coating of silica cores with a precursor of PAH, followed by adsorption of 50 nm-sized DOPC liposomes and subsequent layering of PSS and PAH99 (Fig. 5). However, since electrostatic interactions alone showed to provide insufficient affinity between liposomes and the underlying polymer surface, the authors developed a modified protocol in which cholesterol-modified polymers were used as noncovalent anchors for the loading of liposomes into the capsules. In this work the enzyme β-lactamase has been pre-loaded into the liposomes before their entrapment inside the polymer capsules and the presence of the active enzyme within the as-prepared capsosomes has been confirmed by quantitative enzymatic reaction.100 The main novelty of the presented approach consists on the synthesis of a hybrid microreactor system which contains numerous liposome subcompartments (about 8 × 103) in which different enzymatic reactions might be carried out simultaneously.
![Typical morphology of (PAH/liposomesNBD/(PSS/PAH)4/PSS) capsosome. (a) Scheme of the geometry of a capsosome with liposome embedded in its wall, CLSM image and (b) negative stained TEM image of capsosomes. The black arrows identify structurally intact liposomes, while the white arrows indicate areas where the liposomes have been displaced. The inset shows a complete capsosome. [Adapted from ref. 99.]](/image/article/2010/NR/b9nr00341j/b9nr00341j-f5.gif) |
| Fig. 5 Typical morphology of (PAH/liposomesNBD/(PSS/PAH)4/PSS) capsosome. (a) Scheme of the geometry of a capsosome with liposome embedded in its wall, CLSM image and (b) negative stained TEM image of capsosomes. The black arrows identify structurally intact liposomes, while the white arrows indicate areas where the liposomes have been displaced. The inset shows a complete capsosome. [Adapted from ref. 99.] | |
The use of DNA as shell component has also been demonstrated recently.101 Multilayer DNA shells were formed by alternately depositing diblock oligonucleotides containing two different regions (one of which is complementary to the adsorbed single-stranded block in the film, whereas the second region is free for hybridization in the subsequent layer). In a related work the authors developed a method to encapsulate various nucleic acids inside degradable polyelectrolyte capsules.91 Capsules entirely composed of DNA (as cargo and as shell constituents) could be of particular interest because they are biodegradable, biocompatible, and their physicochemical properties (e.g., size, permeability, structure and shrinkage) can be finely controlled by base pairing of the nucleotides.
Biostability in physiological environments
One of the main problems of charged systems is that they are easily cleared from the organism by serum protein adsorption (opsonization) and subsequent phagocytosis. Covalent linkage of PEG (poly(ethylene glycol)) or other hydrophilic polymers to the surface of several carrier systems have displayed to reduce their non-specific uptake by the cells (including cells from the mononuclear phagocyte system) due to their enhanced low-fouling properties.20,102–104 Since the main stabilizing forces in polyelectrolyte capsules are electrostatic interactions, the efficient binding of a PEG corona to the surface of the capsule has to be carried out by previous linkage of PEG to highly charged substances like polyelectrolytes. In this way, modified PEG can be strongly and stably attached to charged surfaces by electrostatic interactions. Modifying the surface of the polyelectrolyte multilayer capsules by adding polyelectrolyte (i.e. poly(L-lysine))-graft-PEG molecules has been a successful strategy to resist protein adsorption and therefore opsonization.52,105–107 As mentioned before, the main forces that stabilize LbL assembled systems are based on electrostatic interactions. This makes such systems very sensitive to environmental conditions such as changes in the ionic strength of the medium or in the temperature and thus susceptible to disassembly. Although systems assembled in this way can be tailored to take advantage of these properties, for some biomedical applications this can be an important limitation. Recent approaches have developed capsules whose synthesis combines the electrostatic forces via LbL assembly and covalent cross-linking via click chemistry.108 This versatile approach has several advantages: (i) low charged polyelectrolytes can be now incorporated to the LbL (after incorporation of covalent bonds); (ii) linkage groups that remain unreacted can be easily post-functionalized; (iii) due to the mild, non-harmful conditions of the click chemistry, sensitive cargo (easy denaturizable active molecules like protein, nucleic acids) can be incorporated.106 Nevertheless, post-functionalization of the capsule surface is still an important feature under investigation. With click chemistry, all reactions are catalyzed by copper, thus leading to cytotoxic effects,106 although very recently a metal-catalyst-free approach has been developed to introduce functional groups to the multilayer of the capsules.109
Cytotoxicity
Regarding the cytotoxicity of the capsules, although not yet well studied, the main sources for toxicity come obviously from the polyelectrolytes composing the wall as well as from the functionalities embedded in the cavity and/or in the wall.40 The magnitude of the cytotoxic effect of the capsules is primarily concentration- and time-dependent.69,110 Additionally, the intrinsic chemical properties of the positively charged polyelectrolyte (polycation) turned out to make them effective triggers of mitochondrial-mediated cell death (apoptosis/necrosis).111,112 Due to their positive charge, polycations cause cellular membrane damage with subsequent activation of signalling pathways that end up with mitochondrial depolarization and generation of reactive oxygen species resulting in cell death. In this regard, together with the molecular weights, the cationic charge density of the polycations are key parameters for the interaction with cell membrane and cell damage.113,114 Furthermore, polycations containing (poly)amine functionalities may result in an increased interaction with anionic intracellular components that also lead to oxygen-independent cell death.115 In this regard, a reduction of the cytotoxicity could be obtained by using materials that are already present in the cells i.e. lipids and proteins as natural polyions. Owing to their amphiphilic character, cellular lipids can aggregate in aqueous solution into spherically closed bilayer structures due to hydrophobic interactions. When the aqueous solution contains a charged protein, a self-assembly process that combines electrostatic interactions and hydrophobic forces occurs in the immiscible interface. The result is the adsorption of proteins and lipids onto the interface of emulsion droplets and the formation of a multilayer elastic shell.116 Despite the compatibility of these biomimetic capsules and the potential to incorporate molecular functionalities like channels or receptors, the main approach of this technique still relies in controlling the size of the capsules and the unwanted degradation of the system.
Applications in medicine
One of the possible contributions of LbL-derived multilayer capsules to medicine is their use as biocompatible multifunctional composite carrier systems that are sensitive to remote guidance and activation for local release of cargo molecules (i.e. drugs) inside target cells/tissues.40 Due to the high versatility of the LbL technique, not only hydrophilic molecules can be loaded but also hydrophobic molecules (i.e. many therapeutical drugs) have been efficiently encapsulated and released thus overcoming the obstacles of hydrophobicity.117 To date it is a fact that polyelectrolyte multilayer capsules of different sizes (from nanometre to micrometre) are taken up by living cells.69,118 The incorporation of the capsules occurs spontaneously and is non-cell specific. Therefore the addition of low-fouling polymers which posses protein-repellent qualities is required besides targeting features toward the design of novel vehicles for targeted drug delivery in vivo. For instance, microcapsules coated with a layer of PEG-grafted polyelectrolytes have been shown to escape clearance by the mononuclear phagocytic system.52,105,106 As previously described, the release of the transported cargo molecules can occur under exposure to external stimuli (e.g., light- or ultrasound-treatment of nanoparticles modified capsules) or more challenging, by using the conditions of the local environment of the capsules (i.e. intracellularly).68,97 Recent publications have proven the use of biodegradable capsules for the delivery of pro-drugs inside the cells.47,119 Pro-drugs are the non-active form of a medicament that needs to be enzymatically hydrolysed to release the active form of the drug. Fig. 6 shows a capsule-based system sensitive to enzymatic degradation at two distinct positions.119 Both the capsule wall and the encapsulated cargo (in this case a pro-drug) are easily degraded by proteases located inside the cells. The intracellular degradation of the capsule wall leads to the release of the pro-drug and enables activation of the drug through enzymatic cleavage. Furthermore, sustained release of the cargo molecules is obtained by the continuous enzymatic digestion of the capsule membrane.119 In this way, several main requirements for drug delivery are achieved. The incorporation of highly environmental-sensitive cargos (i.e. pro-drug or proteins) inside the capsule protects both the cargo from unwanted degradation and healthy cells from the action of the drug. Since less concentration of the drug could be administered in order to reach therapeutic concentrations, the side effects would be reduced. Thus, the intracellular degradation would enable the controlled release of cargo over a long period of time locally.
![Cargo release and activation mediated by intracellular degradation of capsules. Capsule walls are of DEXS/pARG and the cavity is filled with a fluorogenic substrate for proteases (DQ-OVA™). Upon cellular internalization, the capsule wall is actively degraded enzymatically, this lead specific enzymes to reach the cargo proteins (ovalbumine, OVA). When OVA is structurally intact, the fluorescence of the dyes conjugated to ovalbumine are quenched. On the contrary, when OVA is enzymatically cleavaged the quenching effect is relieved and a bright green fluorescence appears. As a result of the cleavage of OVA, single dye-labeled peptides are released out of the capsule. [Adapted from ref. 119.]](/image/article/2010/NR/b9nr00341j/b9nr00341j-f6.gif) |
| Fig. 6 Cargo release and activation mediated by intracellular degradation of capsules. Capsule walls are of DEXS/pARG and the cavity is filled with a fluorogenic substrate for proteases (DQ-OVA™). Upon cellular internalization, the capsule wall is actively degraded enzymatically, this lead specific enzymes to reach the cargo proteins (ovalbumine, OVA). When OVA is structurally intact, the fluorescence of the dyes conjugated to ovalbumine are quenched. On the contrary, when OVA is enzymatically cleavaged the quenching effect is relieved and a bright green fluorescence appears. As a result of the cleavage of OVA, single dye-labeled peptides are released out of the capsule. [Adapted from ref. 119.] | |
Anticancer drugs such as doxorubicin (DOX) have been demonstrated to be released upon changes in the pH of a solution.120 Since the location of the capsules seems to be in acidic compartments,70 by choosing the right materials that provide a good compromise between swelling and stiffness, drugs can be released from the capsules over time in a controlled manner self-regulated by the intracellular conditions. The low pH of the acidic vesicles where the capsules are transported causes swelling of the multilayer121 with an initial rapid release of the drug due to high differences in the drug concentration between the bulk and the capsule cavity and followed by a plateau. The release can continue over a period of time until degradation of the capsule has been finalized or the entire amount of drug has been released. In vivo studies have demonstrated that this might be possible by using DOX-loaded biodegradable polyelectrolytes alternatively attached to doped CaCO3-carboxylmethyl cellulose (CMS) templates. After chemical removal of the CaCO3, CMS forms a complex with DOX and is stabilized in the capsule cavity. Direct injection of the loaded-capsules to the tumor of a mouse, led to a sustained release of DOX for 4 weeks, probably due to a non-favorable thermodynamic process at low pH, and resulted in a reduction of the tumor.45
The LbL approach appears to be a helpful technique not only for anticancer therapy but also for vaccination. One of the main challenges in vaccination is the efficient delivery of effective doses and the co-delivery of adjuvants with the antigen in order to generate sufficient immune response. Self-exploding microcapsules made of a semi-permeable polyelectrolyte multilayer around a sugar-based hydrophilic microgel core (size around 10 μm) have emerged as a “single shot” delivery system for the release of antigens in multiple pulses.122 Due to the large size of the core, smaller carriers can be incorporated in the gel. Because of the semi-permeable conditions of the wall, water can penetrate causing a strong swelling of the gel that finally leads to the rupture of the microcontainer followed by the “shooting” of the smaller carriers that are able to propagate in water faster than if they were in solution. This is of special importance when the species has to be released in viscous medium. By tuning the density of the microgel, the resistance to disruption can be modulated and the microcontainers are able to release cargo (i.e. vaccines) at different times.
Conclusion/outlook
In this review we have highlighted some of the recent progresses of designing and utilizing novel types of multifunctional LbL-based multilayer capsules. LbL-assembled capsules are very versatile platforms which can be used to encapsulate, to store and to delivery cargo molecules. Their selective permeability allows to load not only different types of molecules, ranging from ions to macromolecules, but also to perform chemical reactions inside the interior. In addition, they can be easily functionalized with various natural molecules or inorganic nanoparticles to create multifunctional materials with hybrid properties.
Nowadays several in vitro45,118,123–125 and in vivo studies45,89 have established the use of these capsules as carriers in living systems. Notably, capsules with different physicochemical properties have been produced for different applications ranging from simultaneous imaging and delivery of biologically active molecules,72–74 to intracellular sensing and bio-reactors.26,27,70,96,126,127 Capsules modified by metal nanoparticles capable of protecting molecules in their cavities and release them by light irradiation, have been fabricated and characterized.71,80 As an alternative to active release mechanisms, biocompatible controlled-release by using capsules made of biodegradable polymer shells which are gradually decomposed within the cellular environment, have been demonstrated.47,119
Nevertheless, there are still some challenges left to be overcome for further development of LbL capsules systems for drug delivery and therapeutic purposes. In particular, the preparations of capsules need to be improved in terms of particles dispersion and aggregation as well as new efficient methods for the large-scale production have to be developed since production of capsules is often time-consuming and at the laboratory scale. In addition there is a need for more generally applicable strategies to efficiently encapsulate water-soluble low molecular weight drugs, in fact most capsule shells show pores size around 10 nm which are often the reason of fast diffusion of the cargo out of the cavities. Regarding this issue, the recent data obtained by Song and colleagues are promising: the authors showed the efficient entrapment of a small drug procainamide hydrochloride (PrH), molecular weight 271.79, inside (PDADMAC/PSS) capsules by heat treatment and shrinking of the capsules.28
The cellular uptake pathway and the degradation/release kinetics have to be intensively investigated for optimization of these systems in biological applications. The cytotoxicity and immune response of capsules need to be evaluated in more detail for clinical application. Also future improvements in developing new molecules and in studying new active targeting and controlled release mechanisms are essential in order to assemble new complex functional systems transferrable to the clinic. For example, the development of capsules with multiple compartments able to simultaneously transport multiple therapeutics agents in pathological sites would be very promising for tumors/cancer therapies. Such systems could then co-deliver anticancer drugs and small interfering RNA (siRNA) to inhibit genetic components of the tumors. It is expected that the cooperation between different scientific communities (bioengineers, chemists, physicists, biologists, bio-nanotechnologists) might be the best approach to overcome the limits of the available current systems by designing novel materials capable of fulfilling the needs of different communities.
Acknowledgements
This work was supported by the BMBF within the ERANET Neuron framework.
References
- R. Langer and D. A. Tirrell, Nature, 2004, 428, 487–492 CrossRef CAS.
- O. C. Farokhzad and R. Langer, ACS Nano, 2009, 3, 16–20 CrossRef CAS.
- M. Ferrari, Nat. Rev. Cancer, 2005, 5, 161–171 CrossRef CAS.
- J. Peteiro-Cartelle, M. Rodríguez-Pedreira, F. Zhang, P. Rivera Gil, L. L. del Mercato and W. J. Parak, Nanomedicine, 2009, 4, 967–979 CrossRef CAS.
- D. Peer, J. M. Karp, S. Hong, O. C. FaroKHzad, R. Margalit and R. Langer, Nat. Nanotechnol., 2007, 2, 751–760 CrossRef CAS.
-
A. Merkoçi, Biosensing Using Nanomaterials, Wiley Series In Nanoscience and Nanotechnology, 2009 Search PubMed.
- R. Freeman, R. Gill, I. Shweky, M. Kotler, U. Banin and I. Willner, Angew. Chem., Int. Ed., 2009, 48, 309–313 CrossRef CAS.
- S. J. Son, X. Bai and S. Lee, Drug Discovery Today, 2007, 12, 657–663 CrossRef CAS.
- S. J. Son, X. Bai and S. B. Lee, Drug Discovery Today, 2007, 12, 650–656 CrossRef CAS.
- E. Boisselier and D. Astruc, Chem. Soc. Rev., 2009, 38, 1759–1782 RSC.
- M. Arruebo, R. Fernandez-Pacheco, M. R. Ibarra and J. Santamaria, Nano Today, 2007, 2, 22–32 CrossRef.
- L. Zhang, F. X. Gu, J. M. Chan, A. Z. Wang, R. S. Langer and O. C. Farokhzad, Clin. Pharmacol. Ther., 2008, 83, 761–769 CrossRef CAS.
- R. A. Sperling, P. Rivera_Gil, F. Zhang, M. Zanella and W. J. Parak, Chem. Soc. Rev., 2008, 37, 1896–1908 RSC.
- J. H. Adair, T. Li, T. Kido, K. Havey, J. Moon, J. Mecholsky, A. Morrone, D. R. Talham, M. H. Ludwig and L. Wang, Mater. Sci. Eng., R, 1998, 23, 139–242 CrossRef.
- H. L. Crampton and E. E. Simanek, Polym. Int., 2007, 56, 489–496 CrossRef CAS.
- Y. Malam, M. Loizidou and A. M. Seifalian, Trends Pharmacol. Sci., 2009, 30, 592–599 CrossRef CAS.
- A. Samad, Y. Sultana and M. Aqil, Curr. Drug Delivery, 2007, 4, 297–305 Search PubMed.
- K. Kataoka, A. Harada and Y. Nagasaki, Adv. Drug Delivery Rev., 2001, 47, 113–131 CrossRef CAS.
- Y. Yang, W. Jia, X. Qi, C. Yang, L. Liu, Z. Zhang, J. Ma, S. Zhou and X. Li, Macromol. Biosci., 2004, 4, 1113–1117 CrossRef CAS.
- V. Torchilin, Eur. J. Pharm. Biopharm., 2009, 71, 431–444 CrossRef CAS.
- S. A. Corr, Y. P. Rakovich and Y. K. Gun'ko, Nanoscale Res. Lett., 2008, 3, 87–104 Search PubMed.
- E. Tasciotti, X. W. Liu, R. Bhavane, K. Plant, A. D. Leonard, B. K. Price, M. M. C. Cheng, P. Decuzzi, J. M. Tour, F. Robertson and M. Ferrari, Nat. Nanotechnol., 2008, 3, 151–157 CrossRef CAS.
- G. Decher, Science, 1997, 277, 1232–1237 CrossRef CAS.
- G. B. Sukhorukov, A. L. Rogach, M. Garstka, S. Springer, W. J. Parak, A. Muñoz-Javier, Oliver Kreft, A. G. Skirtach, A. S. Susha, Y. Ramaye, R. Palankar and M. Winterhalter, Small, 2007, 3, 944–955 CrossRef CAS.
- E. Donath, G. B. Sukhorukov, F. Caruso, S. A. Davis and H. Möhwald, Angew. Chem., Int. Ed., 1998, 37, 2201–2205 CrossRef.
- L. Dähne, S. Leporatti, E. Donath and H. Mohwald, J. Am. Chem. Soc., 2001, 123, 5431–5436 CrossRef CAS.
- D. G. Shchukin and G. B. Sukhorukov, Adv. Mater., 2004, 16, 671–682 CrossRef CAS.
- W. Song, Q. He, H. Möhwald, Y. Yang and J. i. L, J. Controlled Release, 2009, 139, 160–166 CrossRef CAS.
- F. Caruso, W. J. Yang, D. Trau and R. Renneberg, Langmuir, 2000, 16, 8932–8936 CrossRef CAS.
- R. Georgieva, S. Moya, M. Hin, R. Mitlohner, E. Donath, H. Kiesewetter, H. Mohwald and H. Baumler, Biomacromolecules, 2002, 3, 517–524 CrossRef CAS.
- F. Caruso, D. Trau, H. Möhwald and R. Renneberg, Langmuir, 2000, 16, 1485–1488 CrossRef CAS.
- A. M. Yu, Y. J. Wang, E. Barlow and F. Caruso, Adv. Mater., 2005, 17, 1737 CrossRef CAS.
- G. B. Sukhorukov, A. Fery, M. Brumen and H. Mohwald, Phys. Chem. Chem. Phys., 2004, 6, 4078–4089 RSC.
- G. Sukhorukov, A. Fery and H. Möhwald, Prog. Polym. Sci., 2005, 30, 885–897 CrossRef CAS.
- Bruno G. De Geest, Niek N. Sanders, Gleb B. Sukhorukov, Joseph Demeestera and S. C. D. Smedt, Chem. Soc. Rev., 2007, 36, 636–649 RSC.
- A. P. R. Johnston, C. Cortez, A. S. Angelatos and F. Caruso, Curr. Opin. Colloid Interface Sci., 2006, 11, 203–209 CrossRef CAS.
- C. S. Peyratout and L. Dähne, Angew. Chem., Int. Ed., 2004, 43, 3762–3783 CrossRef.
- A. Fery and R. Weinkamer, Polymer, 2007, 48, 7221–7235 CrossRef CAS.
- A. Antipov and G. B. Sukhorukov, Adv. Colloid Interface Sci., 2004, 111, 49–61 CrossRef CAS.
- P. Rivera Gil, L. L. del Mercato, P. del Pino, A. Munoz Javier and W. J. Parak, Nano Today, 2008, 3, 12–21 CrossRef CAS.
- G. B. Sukhorukov, D. V. Volodkin, A. M. Günther, A. I. Petrov, D. B. Shenoy and H. Möhwald, J. Mater. Chem., 2004, 14, 2073–2081 RSC.
- W. J. Tong and C. Y. Gao, Polym. Adv. Technol., 2005, 16, 827–833 CrossRef CAS.
- G. Berth, A. Voigt, H. Dautzenberg, E. Donath and H. Mohwald, Biomacromolecules, 2002, 3, 579–590 CrossRef CAS.
- X. P. Qiu, S. Leporatti, E. Donath and H. Mohwald, Langmuir, 2001, 17, 5375–5380 CrossRef CAS.
- Q. Zhao, B. Han, Z. Wang, C. Gao, C. Peng and J. Shen, Nanomedicine, 2007, 3, 63–74 CAS.
- A. M. Yu, I. R. Gentle and G. Q. Lu, J. Colloid Interface Sci., 2009, 333, 341–345 CrossRef CAS.
- B. G. De Geest, R. E. Vandenbroucke, A. M. Guenther, G. B. Sukhorukov, W. E. Hennink, N. N. Sanders, J. Demeester and S. C. d. Smedt, Adv. Mater., 2006, 18, 1005–1009 CrossRef.
- F. Caruso, A. S. Susha, M. Giersig and H. Möhwald, Adv. Mater., 1999, 11, 950–953 CrossRef CAS.
- A. G. Skirtach, A. A. Antipov, D. G. Shchukin and G. B. Sukhorukov, Langmuir, 2004, 20, 6988–6992 CrossRef CAS.
- A. S. Angelatos, B. Radt and F. Caruso, J. Phys. Chem. B, 2005, 109, 3071–3076 CrossRef CAS.
- Z. Lu, M. D. Prouty, Z. Guo, V. O. Golub, C. S. Kumar and Y. M. Lvov, Langmuir, 2005, 21, 2042–2050 CrossRef CAS.
- R. Heuberger, G. Sukhorukov, J. Vörös, M. Textor and H. Möhwald, Adv. Funct. Mater., 2005, 15, 357–366 CrossRef CAS.
- C. R. Kinnane, K. Wark, G. K. Such, A. P. R. Johnston and F. Caruso, Small, 2009, 5, 444–448 CrossRef CAS.
- C. Cortez, E. Tomaskovic-Crook, A. P. R. Johnston, B. Radt, S. H. Cody, A. M. Scott, E. C. Nice, J. K. Heath and F. Caruso, Adv. Mater., 2006, 18, 1998–2003 CrossRef CAS.
- F. Caruso, Chem.–Eur. J., 2000, 6, 413–419 CrossRef CAS.
- D. V. Volodkin, N. I. Larionova and G. B. Sukhorukov, Biomacromolecules, 2004, 5, 1962–1972 CrossRef CAS.
- A. A. Antipov, G. B. Sukhorukov, S. Leporatti, I. L. Radtchenko, E. Donath and H. Möhwald, Colloids Surf., A, 2002, 198–200, 535–541 CrossRef CAS.
- D. V. Volodkin, A. I. Petrov, M. Prevot and G. B. Sukhorukov, Langmuir, 2004, 20, 3398–3406 CrossRef CAS.
- K. Köhler and G. B. Sukhorukov, Adv. Funct. Mater., 2007, 17, 2053–2061 CrossRef.
- T. Mauser, C. Dejugnat and G. B. Sukhorukov, Macromol. Rapid Commun., 2004, 25, 1781–1785 CrossRef CAS.
- Z. An, H. Möhwald and J. Li, Biomacromolecules, 2006, 7, 580–585 CrossRef CAS.
- G. B. Sukhorukov, A. A. Antipov, A. Voigt, E. Donath and H. Mohwald, Macromol. Rapid Commun., 2001, 22, 44–46 CrossRef CAS.
- Y. Lvov, A. A. Antipov, A. Mamedov, H. Möhwald and G. B. Sukhorukov, Nano Lett., 2001, 1, 125–128 CrossRef CAS.
- C. Y. Gao, S. Leporatti, S. Moya, E. Donath and H. Mohwald, Chem.–Eur. J., 2003, 9, 915–920 CrossRef CAS.
- A. A. Antipov, G. B. Sukhorukov and H. Mohwald, Langmuir, 2003, 19, 2444–2448 CrossRef CAS.
- C. Y. Gao, S. Moya, H. Lichtenfeld, A. Casoli, H. Fiedler, E. Donath and H. Möhwald, Macromol. Mater. Eng., 2001, 286, 355–361 CrossRef CAS.
- D. B. Shenoy, A. A. Antipov, G. B. Sukhorukov and H. Mohwald, Biomacromolecules, 2003, 4, 265–272 CrossRef CAS.
- Y. Itoh, M. Matsusaki, T. Kida and M. Akashi, Biomacromolecules, 2008, 9, 2202–2206 CrossRef CAS.
- S. De Koker, B. G. De Geest, C. Cuvelier, L. Ferdinande, W. Deckers, W. E. Hennink, S. De Smedt and N. Mertens, Adv. Funct. Mater., 2007, 17, 3754–3763 CrossRef CAS.
- O. Kreft, A. Muñoz_Javier, G. B. Sukhorukov and W. J. Parak, J. Mater. Chem., 2007, 17, 4471–4476 RSC.
- A. Muñoz Javier, P. d. Pino, M. Bedard, A. G. Skirtach, D. Ho, G. Sukhorukov, C. Plank and W. J. Parak, Langmuir, 2008, 24, 12517–12520 CrossRef.
- A. Rogach, A. Susha, F. Caruso, G. Sukhorukov, A. Kornowski, S. Kershaw, H. Möhwald, A. Eychmüller and H. Weller, Adv. Mater., 2000, 12, 333–337 CrossRef CAS.
- N. Gaponik, I. L. Radtchenko, M. R. Gerstenberger, Y. A. Fedutik, G. B. Sukhorukov and A. L. Rogach, Nano Lett., 2003, 3, 369–372 CrossRef CAS.
- N. Gaponik, I. L. Radtchenko, G. B. Sukhorukov, H. Weller and A. L. Rogach, Adv. Mater., 2002, 14, 879–882 CrossRef CAS.
- S. Mornet, S. Vasseur, F. Grasset and E. Duguet, J. Mater. Chem., 2004, 14, 2161–2175 RSC.
- C. Corot, P. Robert, J. M. Idee and M. Port, Adv. Drug Delivery Rev., 2006, 58, 1471–1504 CrossRef CAS.
- V. I. Shubayev, T. R. Pisanic and S. H. Jin, Adv. Drug Delivery Rev., 2009, 61, 467–477 CrossRef CAS.
- B. Zebli, A. S. Susha, G. B. Sukhorukov, A. L. Rogach and W. J. Parak, Langmuir, 2005, 21, 4262–4265 CrossRef CAS.
- A. G. Skirtach, C. Dejugnat, D. Braun, A. S. Susha, W. J. Parak, H. Möhwald and G. B. Sukhorukov, Nano Lett., 2005, 5, 1371–1377 CrossRef CAS.
- M. F. Bédard, D. Braun, G. B. Sukhorukov and A. G. Skirtach, ACS Nano, 2008, 2, 1807–1816 CrossRef CAS.
- C. Sönnichsen, T. Franzl, T. Wilk, G. von Plessen, J. Feldmann, O. Wilson and P. Mulvaney, Phys. Rev. Lett., 2002, 88, 077402 CrossRef CAS.
- A. Henkel, A. Jakab, G. Brunklaus and C. Sonnichsen, J. Phys. Chem. C, 2009, 113, 2200–2204 CrossRef CAS.
- A. G. Skirtach, P. Karageorgiev, B. G. De_Geest, N. Pazos-Perez, D. Braun and G. B. Sukhorukov, Adv. Mater., 2008, 20, 506–510 CrossRef CAS.
- T. V. Bukreeva, B. V. Parakhonskiy, I. V. Marchenko, B. N. Khlebtsov, N. G. Khlebtsov, O. V. Dementieva, M. N. Savvateev, L. A. Feigin and a. M. V. Kovalchuk, Nanotechnol. Russ., 2008, 3, 85–93 Search PubMed.
- A. G. Skirtach, B. G. De_Geest, A. Mamedov, A. A. Antipov, N. A. Kotov and G. B. Sukhorukov, J. Mater. Chem., 2007, 17, 1050–1054 RSC.
- S. H. Hu, C. H. Tsai, C. F. Liao, D. M. Liu and S. Y. Chen, Langmuir, 2008, 24, 11811–11818 CrossRef CAS.
- Y. Zhu, W. J. Tong, C. Y. Gao and H. Mohwald, Langmuir, 2008, 24, 7810–7816 CrossRef CAS.
- R. Lever and C. P. Page, Nat. Rev. Drug Discovery, 2002, 1, 140–148 CrossRef CAS.
- L. Yu, Y. G. Gao, X. L. Yue, S. Q. Liu and Z. F. Dai, Langmuir, 2008, 24, 13723–13729 CrossRef CAS.
- A. N. Zelikin, J. F. Quinn and F. Caruso, Biomacromolecules, 2006, 7, 27–30 CrossRef CAS.
- A. N. Zelikin, Q. Li and F. Caruso, Angew. Chem., Int. Ed., 2006, 45, 7743–7745 CrossRef CAS.
- A. N. Zelikin, Q. Li and F. Caruso, Chem. Mater., 2008, 20, 2655–2661 CrossRef CAS.
- Y. Zhu, W. J. Tong, C. Y. Gao and H. Mohwald, J. Mater. Chem., 2008, 18, 1153–1158 RSC.
- L. Duan, Q. He, X. H. Yan, Y. Cui, K. W. Wang and J. B. Li, Biochem. Biophys. Res. Commun., 2007, 354, 357–362 CrossRef CAS.
- W. Qi, L. Duan, K. W. Wang, X. H. Yan, Y. Citi, Q. He and J. B. Li, Adv. Mater., 2008, 20, 601–605 CrossRef CAS.
- W. Qi, X. Yan, L. Duan, Y. Cui, Y. Yang and J. Li, Biomacromolecules, 2009, 10, 1212–1216 CrossRef CAS.
- W. Qi, X. H. Yan, J. B. Fei, A. H. Wang, Y. Cui and J. B. Li, Biomaterials, 2009, 30, 2799–2806 CrossRef CAS.
- J. F. Pereira da Silva Gomes, A. Rank, A. Kronenberger, J. Fritz, M. Winterhalter and Y. Ramaye, Langmuir, 2009, 25, 6793–6799 CrossRef.
- B. Städler, R. Chandrawati, K. Goldie and F. Caruso, Langmuir, 2009, 25, 6725–6732 CrossRef CAS.
- B. Städler, R. Chandrawati, A. D. Price, S. F. Chong, K. Breheney, A. Postma, L. A. Connal, A. N. Zelikin and F. Caruso, Angew. Chem., Int. Ed., 2009, 48, 4359–4362 CrossRef CAS.
- A. P. R. Johnston, E. S. Read and F. Caruso, Nano Lett., 2005, 5, 953–956 CrossRef CAS.
- L. van Vlerken, T. Vyas and M. Amiji, Pharm. Res., 2007, 24, 1405 CrossRef CAS.
- G. Prencipe, S. M. Tabakman, K. Welsher, Z. Liu, A. P. Goodwin, L. Zhang, J. Henry and H. J. Dai, J. Am. Chem. Soc., 2009, 131, 4783–4787 CrossRef CAS.
- V. C. F. Mosqueira, P. Legrand, A. Gulik, O. Bourdon, R. Gref, D. Labarre and G. Barratt, Biomaterials, 2001, 22, 2967–2979 CrossRef CAS.
- U. Wattendorf, O. Kreft, M. Textor, G. B. Sukhorukov and H. P. Merkle, Biomacromolecules, 2008, 9, 100–108 CrossRef CAS.
- C. J. Ochs, G. K. Such, B. Stadler and F. Caruso, Biomacromolecules, 2008, 9, 3389–3396 CrossRef CAS.
- A. J. Khopade and F. Caruso, Langmuir, 2003, 19, 6219–6225 CrossRef CAS.
- B. G. De Geest, W. Van Camp, F. E. Du Prez, S. C. De Smedt, J. Demeester and W. E. Hennink, Chem. Commun., 2008, 190–192 RSC.
- L. A. Connal, C. R. Kinnane, A. N. Zelikin and F. Caruso, Chem. Mater., 2009, 21, 576–578 CrossRef CAS.
- C. Kirchner, A. M. Javier, A. S. Susha, A. L. Rogach, O. Kreft, G. B. Sukhorukov and W. J. Parak, Talanta, 2005, 67, 486–491 CrossRef CAS.
- A. C. Hunter, Adv. Drug Delivery Rev., 2006, 58, 1523–1531 CrossRef CAS.
- D. Fischer, Y. X. Li, B. Ahlemeyer, J. Krieglstein and T. Kissel, Biomaterials, 2003, 24, 1121–1131 CrossRef CAS.
- T. Bieber, W. Meissner, S. Kostin, A. Niemann and H. P. Elsasser, J. Controlled Release, 2002, 82, 441–454 CrossRef CAS.
- W. T. Godbey, K. K. Wu and A. G. Mikos, J. Biomed. Mater. Res., 1999, 45, 268–275 CrossRef CAS.
- N. Seiler and F. Raul, J. Cell. Mol. Med., 2005, 9, 623–642 CrossRef CAS.
- J. B. Li, H. Mohwald, Z. H. An and G. Lu, Soft Matter, 2005, 1, 259–264 RSC.
- K. Wang, Q. He, X. Yan, Y. Cui, W. Qi, L. Duana and J. Li, J. Mater. Chem., 2007, 17, 4018–4021 RSC.
- A. Muñoz Javier, O. Kreft, M. Semmling, S. Kempter, A. G. Skirtach, O. Bruns, P. del Pino, M. F. Bedard, J. Rädler, J. Käs, C. Plank, G. Sukhorukov and W. J. Parak, Adv. Mater., 2008, 20, 4281–4287 CrossRef CAS.
- P. Rivera-Gil, S. De Koker, B. G. De Geest and W. J. Parak, Nano Lett., 2009, 9, 4398–4402 CrossRef CAS.
- X. L. Yang, X. Han and Y. H. Zhu, Colloids Surf., A, 2005, 264, 49–54 CrossRef CAS.
- C. Déjugnat and G. B. Sukhorukov, Langmuir, 2004, 20, 7265–7269 CrossRef CAS.
- B. G. De Geest, M. J. McShane, J. Demeester, S. C. De Smedt and W. E. Hennink, J. Am. Chem. Soc., 2008, 130, 14480–14482 CrossRef.
- Y. J. Wang, V. Bansal, A. N. Zelikin and F. Caruso, Nano Lett., 2008, 8, 1741–1745 CrossRef CAS.
- Z. H. An, K. Kavanoor, M. L. Choy and L. J. Kaufman, Colloids Surf., B, 2009, 70, 114–123 CrossRef CAS.
- M. Semmling, O. Kreft, A. Muñoz Javier, G. B. Sukhorukov, J. Käs and W. J. Parak, Small, 2008, 4, 1763–1768 CrossRef.
- M. J. McShane, J. Q. Brown, K. B. Guice and Y. M. Lvov, J. Nanosci. Nanotechnol., 2002, 2, 411–416 CrossRef CAS.
- U. Reibetanz, D. Haloan, M. Brumen and E. Donath, Biomacromolecules, 2007, 8, 1927–1933 CrossRef CAS.
|
This journal is © The Royal Society of Chemistry 2010 |
Click here to see how this site uses Cookies. View our privacy policy here.