DOI:
10.1039/B9NR00336C
(Paper)
Nanoscale, 2010,
2, 806-810
Received
(in Hong Kong, China)
4th November 2009
, Accepted 24th January 2010
First published on
17th March 2010
Abstract
A disposable and sensitive biosensor has been fabricated for the detection of the organophosphorous (OP) compound paraoxon using an amperometric technique. For the measurements, gold nanoparticles dispersed on the outer surface of multiwalled carbon nanotubes (Au-MWNTs) has been used as the electrode material, as it possesses high electron transfer rates and provides large immobilization sites for the bioenzymes, which combines with the high electrocatalytic activity of MWNTs for thiocholine oxidation at low potential. Au-MWNTs have been synthesized by chemically reducing Au salt over functionalized MWNTs, and the same has been characterized by X-ray diffraction (XRD), transmission electron microscopy (TEM) and high resolution transmission electron microscopic (HRTEM) techniques. The ability of the Au-MWNTs nanocomposite-based biosensor has been demonstrated to reliably measure the concentration of paraoxon in the nanomolar range.
1. Introduction
Acetylcholine esterase (AChE) is one of the important serine enzymes, necessary for the normal functioning of the peripheral and central nervous systems of living organisms. It converts the neurotransmitter acetylcholine (ACh) substrate to choline and acetate after the transmission of a nerve impulse,1,2 and hence, helps in maintaining proper functioning of the muscular system. Its activity can be drastically affected by organophosphorous (OP) compounds present in the environment, which may result in acute toxicity, cholinergic dysfunction and death.2 OP compounds are commonly used in agriculture, the medicines industry and chemical warfare agents (CWA).3,4 As a result, simple, sensitive and reliable determination of these compounds is necessary. Commonly used methods for the detection of thiocholine are colorimetric methods, spectrophotometry, conductivity variation etc,5–10 which are reliable but time consuming, expensive, and need highly trained personnel and complicated sample pretreatment(s). Irreversible inhibition of enzymes by OP compounds leads to the need to develop cheap and disposable biosensors. In recent years, electrochemistry has developed as a powerful tool for the development of such single use disposable biosensors. Electrochemical strategies involve the measurement of thiocholine produced by the enzymatic hydrolysis of acetylthiocholine iodide (ASChI).11 OP compounds inhibit the activity of AChE, and thus limit the production of thiocholine. Hence, reduction in thiocholine oxidative current is directly related to the enzyme inhibition, which in turn is monitored by amperometric techniques (eqn 1–3). | ASChI → TCh + acetic acid + I | (1) |
| TCh → TCh(ox) + 2H+ + 2e− | (2) |
It is a well established practice to immobilize enzymes over nanomaterial surfaces to improve the response and stability of trace pesticide detection. The commonly used nanomaterial matrices are carbon nanotubes,12–18 quantum dots and Au nanoparticles.19–22 Carbon nanotubes (CNT) are an important class of materials with unique and interesting properties, like high mechanical strength, high aspect ratio, high electrical and thermal conductivity, metallic and structural characteristics. These properties provide good grounds for biological sensing systems.23,24 Liu et al. also illustrated that MWNTs-modified glassy carbon (GC) electrodes offer highly stable, sensitive and repeatable detection of enzymatically generated thiocholine product at low potential, compared to other electrodes like carbon paste and Au electrodes.25 The functional groups like –COOH,
CO and –OH attached to the surface of MWNTs not only provide anchoring sites for biological molecules12,13 but also for metal nanoparticles. Au nanoparticles have also been reported to provide immobilization sites for the enzymes retaining their biocatalytic activity. They also permit direct and fast electron transfer between redox species and the electrode. These properties make Au nanoparticles an attractive material for sensing biological reactions.26 Shulga and Kirchhoff developed a Au nanoparticle-based biosensor where irreversible oxidation of enzymatic product takes place at 680 mV vs. Ag/AgCl.27 However, this high potential is undesirable for biosensing applications. To overcome this problem, several mediators like CoPC,28,29 prussian blue30 and tetracyanoquinodimethane31,32 have been used. But, to the best of our knowledge, not much attention has been paid towards utilizing the effect of both Au nanoparticles and CNTs for OP sensing. Hence, in the present work, we propose the application of Au nanoparticles dispersed over MWNTs, Au-MWNTs nanocomposite, as an electrode material for amperometric measurements, and hence, for OP detection.
2. Experimental
2.1. Reagents, materials and instruments
AChE (Type VI-S from electric eel, 2 K U), ASChI, diethyl p-nitrophenylphosphate (approximately 90%, paraoxon) and N,N-dimethylformamide were from Sigma Aldrich. All other chemicals were of analytical grade and prepared using deionized (DI) water. Thiocholine solution was prepared by enzymatic reaction of AChE and ASChI solution for 30 min in phosphate buffer (pH 7.4). Phosphate buffer solution (0.18 M) was prepared using K2HPO4 and KH2PO4 salts in 0.1 M KCl. The substrate solution of acetylthiocholine iodide (ASChI) solution was prepared in phosphate buffer at a final concentration of 0.01 M and stored at −20 °C. The actual concentration of thiocholine was measured using the absorption spectroscopy technique after reaction with 5,5′-dithiobis(2-nitrobenzoic acid), as described by Ellman's technique,8 which was calculated to be 0.3 mM.
MWNTs were prepared by a single furnace catalytic CVD technique using YNi3 hydride as catalyst and acetylene as carbon precursor. As-grown MWNTs were further purified for the removal of amorphous carbon, other allotropes of carbon and catalytic impurities by air oxidation and acid treatment.33
Amperometric measurements were performed using an electrochemical analyzer CH 660 (CH Instruments, Austin, TX) connected to a personal computer. A three electrode configuration was used with the working volume of 5 ml (phosphate buffer pH 7.4 containing KCl). A modified GC electrode was employed as the working electrode, while Ag/AgCl (3 M KCl) and platinum wire serve as reference and auxiliary electrodes, respectively. The working electrode was operated at the desired potential and transient currents were allowed to decay to a steady value. A magnetic stirrer and magnetic bar were employed for convection.
2.2. Synthesis, purification and functionalization of MWNTs
MWNTs were synthesized over hydrogen decrepitated YNi3 alloy particles using single furnace CVD technique. YNi3 alloy hydride catalyst particles were prepared by the hydrogen decrepitation route, which transforms the alloy into small micro-size particles and exposes fresh surfaces for the pyrolysis of acetylene. As-grown CNTs contain impurities like amorphous carbon, catalytic impurities and some different allotropes of carbon. Hence, they were purified by air oxidation and acid treatment techniques. MWNTs were then further functionalized with hydrophilic functional groups like –OH, –COOH and
CO by additional acid treatment in concentrated nitric acid.34 The functional groups provide anchoring sites for metal nanoparticles as well as biological enzymes.
MWNTs’ outer surfaces were decorated with Au nanoparticles, which are expected to enhance enzyme immobilization and the electron transfer rate between reaction sites and the electrode. Au-MWNTs nanocomposite was prepared by chemically reducing HAuCl4 salt precursor over hydrophilic functionalized MWNTs. About 0.2 g of purified MWNTs was ultrasonicated in 20 ml of DI water for 1 h, and then magnetically stirred for 24 h after the addition of 1 wt% HAuCl4 solution. The Au salt was reduced by the slow addition of a reducing solution, which was a mixture of 0.1 M NaBH4 and 1 M NaOH. Once the reaction was complete, the solution was washed with copious amount of DI water and filtered. The recovered Au-loaded MWNTs were dried at 80 °C for 3 h. Nearly 20 wt% of Au nanoparticles was dispersed on MWNTs surface.
The GC electrode was cleaned by polishing with alumina slurries and rinsed with DI water. 20 mg of Au-MWNTs nanocomposite was ultrasonicated in 1 ml N,N-dimethylformamide (DMF) to obtain a black suspension. 10 μl of this suspension was cast as a thin film over the clean shining surface of the GC, followed by drying at room temperature to evaporate the solvent. After drying, the surface was washed by DI water to remove unbound material.
2.4. Enzyme immobilization
12 μl of AChE solution (0.288 U) was dropped onto the modified GC electrodes and allowed to dry at room temperature under atmospheric conditions. The dried electrode was then rinsed with phosphate buffer having pH 7.4 to remove loosely bound enzymes and MWNTs from the GC. The prepared Au-MWNTs/GC electrode was stored at 4 °C when not in use.
2.5. Inhibition measurement
The OP compound, paraoxon, is structurally similar to other OP compounds like sarin and soman. Hence, in the present work it has been chosen as a standard pesticide for the AChE inhibition. Enzyme AChE-immobilized Au-MWNTs/GC working electrode was immersed in 5 ml of phosphate buffer. This was followed by the addition of substrate ASChI to the phosphate buffer, so that the final concentration of substrate reaches 2 mM, and the current was measured. This value of current, before introduction of paraoxon, corresponds to I0. Now, a known concentration of paraoxon is added onto the electrode, left for incubation for nearly 30 min, followed by washing with buffer solution. After washing, current response was measured again and this value corresponds to Ii, inhibited current. Inhibition % was measured using the following equation:
3. Results and discussion
3.1 Characterization of Au-MWNTs
The synthesized Au-MWNTs nanocomposite has been characterized using powder X-ray diffraction (XRD), transmission electron microscopy (TEM) and high resolution TEM (HRTEM) techniques. Fig. 1 compares the XRD pattern of MWNTs and Au-MWNTs. Fig. 1a shows peaks corresponding to the graphitic hexagonal planes (002) and (101) of MWNTs at 2θ values 26.4 and 44.8 degrees respectively. The diffraction pattern of Au-MWNTs shows major peaks around 2θ values of 40.1, 46.4, 68.0 and 81.7 degrees, corresponding to fcc planes (111), (200), (220) and (311) of Au nanoparticles, respectively. Fig. 2a shows TEM images of the nanocomposite with a homogenous distribution of nanoparticles on the MWNTs’ surface, having outer and inner diameters of approximately 30 and 20 nm, respectively. It also shows that the particle sizes of Au nanoparticles are in the range of 5–10 nm. Lattice planes of the nanoparticles are clearly visible in high resolution TEM image, Fig. 2b.
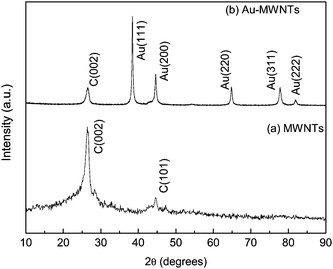 |
| Fig. 1 X-ray diffractograms of (a) MWNTs and (b) Au-MWNTs. | |
3.2. Cyclic voltammetric (CV) characteristics of thiocholine
The CV characteristics of enzymatically produced thiocholine have been studied using the nanocomposite-modified GC electrode as the working electrode in the potential range of 0–1 V and at the scan rate 100 mV s−1 in phosphate buffer (Fig. 3). A bare GC electrode, MWNTs and Au-MWNTs have been studied for the comparative study of thiocholine oxidation. The bare GC electrode fails to give any peak, which confirms that it does not play any role in catalyzing thiocholine oxidation (Fig. 3a). An oxidation peak with small oxidative current is obtained in the case of the MWNTs/GC electrode, which confirms catalysed oxidation of thiocholine by MWNTs. A broad oxidation peak with a very high value of current range is observed with Au-MWNTs/GC at 0.437 V. This is due to the good electron transfer rate of the Au-MWNTs/GC electrode, large working surface area and high aspect ratio of the nanocomposite (Fig. 3b).
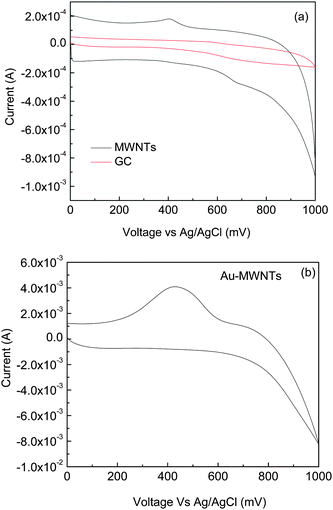 |
| Fig. 3 Cyclic voltammogram of (a) GC and MWNT/GC and (b) Au-MWNTs in thiocholine solution of 0.3 mM. Scanning potential range: 0–1.0 V; potential scanning rate: 100 mV s−1. | |
3.3. Amperometric response
The determination of the optimum potential for the amperometric detection was measured by hydrodynamic voltammetry. This study has been carried out using 5 ml thiocholine with the working electrode at the desired potential in stirring conditions, and transient currents were allowed to decay to a steady value. The response of the plain buffer solution was subtracted from the response in the presence of thiocholine. As shown in Fig. 4, significant values of current enhancement have been observed up to 0.15 V, and it levels off after 0.25 V. The operation of the OP sensor at low electropotential is desirable to reduce the electrochemical interferences for high selectivity, hence a low potential of 150 mV was selected for sensor operation.
3.4. Substrate response
The enzymatic substrate reaction follows zero order kinetics, hence [S] ≫ Km, where [S] denotes substrate concentration and Km is the Michaelis–Menten constant.35 A calibration plot for the sensor has been obtained by the successive addition of substrate in the range 0.2–2.6 mM and measuring the corresponding current, as shown in Fig. 5. A linear relationship has been obtained between the current and concentration of substrate below 2 mM. It follows the linear equation 3.67 × 10−7 + 1.56 × 10−7[S], with a correlation coefficient of 0.99. The Michaelis–Menten constant can be calculated from the electrochemical Eadie–Hofstee form of the Michaelis–Menten equation:
where I is the steady state current and Imax is maximum current. Km has been calculated from the slope of this graph (Fig. 5b) and is 0.53 mM and Imax is 8.3 × 10−7 A. The value of Km reported by Joshi et al. is 0.66 mM.12 This reduction in Km might be due to better adhesion of enzymes to the nanocomposite-modified electrode. Du et al. reported 0.14 μM for galanthamine and 0.19 μM for neostigmine, indicating galanthamine exhibited higher inhibition efficiency to AChE.36 Shulga and Kirchoff reported 0.18 mM for Km for a Au nanoparticles-based electrode.27
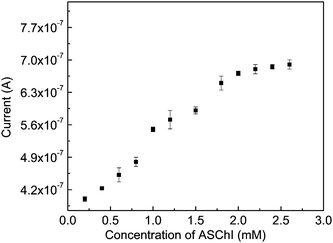 |
| Fig. 5 Calibration plot of substrate for AChE immobilized on a modified GC electrode. Applied potential: 150 mV. | |
The biosensor was then employed for the detection of paraoxon analyte under substrate saturation conditions. Thus, current response depends only on the enzyme-inhibitor reaction and not on the mass transfer of substrate or inhibitor from the electrolyte solution to the electrode–electrolyte interface. Fig. 6 is the inhibition curve which has been obtained by plotting inhibition percentage (I %) versus paraoxon concentration. This inhibition plot of AChE shows high linearity in the range measured from 0.1 to 7 nM. The developed sensor has detected paraoxon up to 0.1 nM (0.025 ppb). If the detection limit is defined as 10% inhibition of the enzyme, then in the present case, the limit of detection obtained is 1 nM. This value is better than other reported values.37 Joshi et al.12 reported the detection limit of 0.5 nM using a thick film strip electrode for the paraoxon sensing. Laschi et al. obtained the detection limit of 4.9 × 10−10 M for carbofuran on the same AChE-based amperometric biosensor.38 Zhang and Malhotra39 developed a biosensor using an ionic liquid technique, where they measured the kinetics with a minimum concentration of 2.75 nM. Recently, Lin et al. studied the electrochemiluminescent (ECL) behavior of lucigenin on MWNTs/nano-Au/GC electrode, and reported a detection limit of 2.5 × 10−8 mol L−1.40 In this case, high sensitivity can be attributed to the presence of Au nanoparticles on the surface of functionalized MWNTs, which provide high anchoring sites for the enzymes, as well as a good electron transfer coefficient.
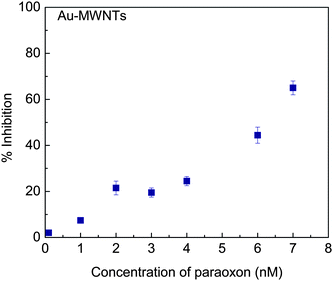 |
| Fig. 6 Inhibition plot of immobilized AChE by paraoxon after 30 min incubation with 2 mM AChI. | |
4. Conclusions
Au-MWNTs-modified GC electrode was used as an electrode material for the quantification of paraoxon. The properties of Au nanoparticles, like high electron transfer rate and large immobilization sites for bioenzymes, are combined with the high electrocatalytic activity of MWNTs towards thiocholine electroxidation at low potential in this nanocomposite. Au-MWNTs allow low potential amperometric detection of paraoxon without the use of a mediating redox potential. The sensor possesses high sensitivity and has been employed to measure concentrations up to 0.1 nM. The developed sensor can also be employed for the detection of other OP compounds.
Acknowledgements
We are thankful to DST, DRDE and DRDO Govt. of India, for the support of this work. One of the authors (Neetu Jha) is grateful to CSIR for financial assistance.
References
- D. M. Quinn, Chem. Rev., 1987, 87, 955–979 CrossRef
.
-
D. Voet and J. G. Voet, Biochemistry, Wiley, New York, 1990 Search PubMed
.
- F. Sanchez-Santed, F. Canada, P. Flores, M. Lopez-Grancha and D. Cardona, Neurotoxicol. Teratol., 2004, 26, 305–317 CrossRef CAS
.
- D. Noort, H. P. Benchop and R. M. Black, Toxicol. Appl. Pharmacol., 2002, 184, 116–126 CrossRef CAS
.
- M. Yamada, Y. Marui, C. Hayashi, Y. Miki and S. T. Clin, Clin. Chem., 2001, 47, 1962–1966 CAS
.
- A. Dietz, H. Rubinstein and T. Lubrano, Clin. Chem., 1973, 19, 1309–1313 CAS
.
- A. Dietz and H. Rubinstein, Clin. Biochem., 1972, 5, 136–138 CrossRef CAS
.
- G. Ellman, Arch. Biochem. Biophys., 1958, 74, 443–450 CrossRef CAS
.
- G. Ellman, Arch. Biochem. Biophys., 1959, 82, 70–77 CrossRef CAS
.
- N. Liu, X. Cai, Y. Lei, Q. Zhang, M. B. Chan-Park, C. Li, W. Chen and A. Mulchandani, Electroanalysis, 2007, 19, 616–619 CrossRef CAS
.
- T. H. Ridgway and H. B. Mark Jr., Anal. Biochem., 1965, 12, 357–366 CrossRef CAS
.
- K. A. Joshi, J. Tang, R. Haddon, W. Joseph, W. Chen and A. Mulchandani, Electroanalysis, 2005, 17, 54–58 CrossRef CAS
.
- N. Jha and S. Ramaprabhu, J. Nanosci. Nanotechnol., 2009, 9, 5676–5680 CrossRef CAS
.
- G. Liu, S. L. Riechers, M. C. Mellen and Y. Lin, Electrochem. Commun., 2005, 7, 1163–1169 CrossRef CAS
.
- G. Liu and Y. Lin, Anal. Chem., 2006, 78, 835–843 CrossRef CAS
.
- D. Du, X. Huang, J. Cai, A. Zhang, J. Ding and S. Chen, Anal. Bioanal. Chem., 2007, 387, 1059–1065 CrossRef CAS
.
- D. Du, X. Huang, J. Cai and A. Zhang, Sens. Actuators, B, 2007, 127, 531–535 CrossRef
.
- D. Du, J. Cai, D. Song and A. Zhang, J. Appl. Electrochem., 2007, 37, 893–898 CrossRef CAS
.
- T. L. Lin, K. T. Huang and C. Y. Liu, Biosens. Bioelectron., 2006, 22, 513–518 CrossRef CAS
.
- D. Du, J. Ding, J. Cai and A. Zhang, Sens. Actuators, B, 2007, 127, 317–322 CrossRef
.
- D. Du, S. Chen, J. Cai and A. Zhang, Biosens. Bioelectron., 2007, 23, 130–134 CrossRef CAS
.
- D. Du, S. Chen, D. Songa, H. Li and X. Chen, Biosens. Bioelectron., 2008, 24, 475–479 CrossRef CAS
.
- R. H. Baughman, A. A. Zakhidov and Walt A. de Heer, Science, 2002, 297, 787–792 CrossRef CAS
.
- D. R. S. Jeykumari and S. S. Narayanan, Carbon, 2009, 47, 957–966 CrossRef CAS
.
- G. Liu, S. L. Riechers, M. C. Mellen and Y. Lin, Electrochem. Commun., 2005, 7, 1163–1169 CrossRef CAS
.
- J. M. Pingarron, P. Yanez-Sedeno and A. Gonzales-Cortes, Electrochim. Acta, 2008, 53, 5848–5866 CrossRef CAS
.
- O. Shulga and J. R. Kirchhoff, Electrochem. Commun., 2007, 9, 935–940 CrossRef CAS
.
- P. Skladal, Anal. Chim. Acta, 1991, 252, 11–15 CrossRef CAS
.
- M. K. Halbert and R. P. Baldwin, Anal. Chem., 1985, 57, 591–595 CrossRef CAS
.
- F. Ricci, F. Arduini, A. Amine, D. Moscone and G. Palleschi, J. Electroanal. Chem., 2004, 563, 229–237 CrossRef CAS
.
- D. Martorell, F. Cespedes, E. M. Fabreagas and S. Alegret, Anal. Chim. Acta, 1997, 337, 305–313 CrossRef CAS
.
- J. Kulys and E. D'costa, Biosens. Bioelectron., 1991, 6, 109–115 CrossRef CAS
.
- M. M. Shaijumon, N. Rajalakshmi, H. Ryu and S. Ramaprabhu, Nanotechnology, 2005, 16, 518–524 CrossRef CAS
.
- N. Jha, A. Leela Mohana Reddy, M. M. Shaijumon, N. Rajalakshmi and S. Ramaprabhu, Int. J. Hydrogen Energy, 2008, 33, 427–433 CrossRef CAS
.
- S. Hernandez, I. Palchetti and M. Mascini, Int. J. Environ. Anal. Chem., 2000, 78, 263–278 CrossRef CAS
.
- D. Du, S. Chen, J. Cai and D. Song, J. Electroanal. Chem., 2007, 611, 60–66 CrossRef CAS
.
- A. Amine, A. Mohammadi, I. Bourais and G. Palleschi, Biosens. Bioelectron., 2006, 21, 1405–1423 CrossRef CAS
.
- S. Laschi, D. Ogonczyk, I. Palchetti and M. Mascini, Enzyme Microb. Technol., 2007, 40, 485–489 CrossRef CAS
.
- C. Zhang and S. V. Malhotra, Talanta, 2005, 67, 560–563 CrossRef CAS
.
- Z. Lin, L. Huang, Y. Liu, J. M. Lin, Y. Chi and G. Chen, Electrochem. Commun., 2008, 10, 1708–1711 CrossRef CAS
.
|
This journal is © The Royal Society of Chemistry 2010 |
Click here to see how this site uses Cookies. View our privacy policy here.