DOI:
10.1039/C0FO00111B
(Review Article)
Food Funct., 2010,
1, 32-59
Review of in vitro digestion models for rapid screening of emulsion-based systems
Received
6th August 2010
, Accepted 7th September 2010
First published on
24th September 2010
Abstract
There is increasing interest in understanding and controlling the digestion of emulsified lipids within the food and pharmaceutical industries. Emulsion-based delivery systems are being developed to encapsulate, protect, and release non-polar lipids, vitamins, nutraceuticals, and drugs. These delivery systems are also being used to control the stability and digestion of lipids within the human gastrointestinal tract so as to create foods that enhance satiety and reduce hunger. In vitro digestion models are therefore needed to test the efficacy of different approaches of controlling lipid digestion under conditions that simulate the human gastrointestinal tract. This article reviews the current status of in vitro digestion models for simulating lipid digestion, with special emphasis on the pH stat method. The pH stat method is particularly useful for the rapid screening of food emulsions and emulsion-based delivery systems with different compositions and structures. Successful candidates can then be tested with more rigorous in vitro digestion models, or using animal or human feeding studies.
1. Introduction
There is growing interest in understanding and controlling the digestibility of lipids within the human gastrointestinal (GI) tract.1–5 The pharmaceutical industry is using this knowledge to design lipid-based delivery systems that either increase the bioavailability of highly lipophilic drugs or that deliver these drugs to specific locations within the GI tract.6,7 The food industry is using a similar approach to design food-grade delivery systems to encapsulate, protect, and release bioactive lipid components, with the aim of either improving their bioavailability or controlling their delivery.4,5,8,9 There are a number of bioactive lipid components that may benefit from encapsulation within this type of delivery system, including ω-3 fatty acids, conjugated linoleic acid, butyrate, phytosterols, carotenoids, antioxidants, coenzyme Q, and vitamins A and D.4,10–14 The availability of effective delivery systems for lipophilic bioactive components could lead to the creation of functional foods specifically designed to maintain or improve human health. Functional foods could be designed to increase the digestibility of lipids in individuals with health conditions that impair the normal digestive process.1,15 Functional foods could be designed to control human satiety, satiation, and hunger by controlling the rate and extent of lipid digestion in different regions of the GI tract.16–20 For example, recent studies show that emulsions that remain stable to gravitational separation in the stomach and/or which have a delayed digestion in the small intestine can stimulate the release of gut hormones that induce satiety and reduce food intake.21,22 Functional foods could be designed to deliver bioactive components to specific locations within the GI tract where they can exhibit their functional attributes, e.g., anticancer components could be released in the colon.23
Analytical tools are needed to screen the efficacy of the various emulsion-based delivery systems that have been designed and developed to control lipid digestion and release. Ultimately, the efficacy of newly designed delivery systems should be tested in animal or human feeding studies, but there are ethical, economic, and practical reasons that make this unrealistic. Some prototype delivery systems may be unsafe or unsuitable for human consumption. Animal feeding studies often involve subjecting animals to uncomfortable conditions and/or sacrifice. Feeding studies are usually expensive, time-consuming, and prone to appreciable subject-to-subject variations. Consequently, there is a need for in vitro analytical tools that can be used to screen delivery systems before more extensive animal or human studies are carried out.4,5 The purpose of this article is to provide an overview of some of the most commonly used in vitro testing methods for studying the digestion of emulsified lipids. We begin by providing a brief overview of the physicochemical and physiological processes that occur when emulsified lipids pass through the human gastrointestinal tract. There have been major advances in this area over the past decade or so, which have greatly facilitated the design of in vitro test methods. Next, we define the concept of lipid bioavailability in the context of the digestion and release of lipophilic components. Finally, we describe a number of in vitro methods that have been developed to test the digestion of emulsified lipids, discussing their relative advantages and disadvantages. In particular, we focus on the pH stat method, which is finding increasing utilization as a convenient tool for rapidly screening different emulsion-based systems.
An understanding of the basic physicochemical and physiological processes that occur as an emulsified lipid passes through the human gastrointestinal (GI) tract is required to develop effective in vitro models that accurately simulate lipid digestion.4,5,8,14,24 After ingestion, emulsified lipids experience a complex series of physical and chemical changes as they pass through the mouth, stomach, small intestine, and large intestine, which affect their ability to be digested and/or absorbed (Fig. 1 and 2). In this section, we provide an overview of the major physicochemical and physiological events that occur in each region of the GI tract. A more detailed description has been given in several recent review articles.4,5,25,26
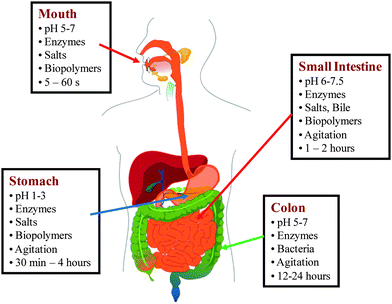 |
| Fig. 1 Schematic diagram of the physicochemical conditions in the different regions of the the human GI tract. Picture of human body was obtained from http://en.wikipedia.org/wiki/Digestive_tract (Copyright free). | |
2.1. Passage through the gastrointestinal tract
We begin by providing an overview of the composition and properties of the various regions within the GI tract.
2.1.1. Pre-ingestion.
The lipid droplets in foods and beverages have a variety of different compositions, physical states, and structural properties.27 The lipid phase may be either non-digestible (e.g., mineral oils) or digestible (e.g., triacylglycerol oils). It may vary in its physical state and polymorphic form, e.g., being liquid, solid, or partially solid at body temperature. Lipid droplets are usually surrounded by a coating consisting of emulsifier molecules and other adsorbed matter. The most common emulsifiers used in foods are proteins, polysaccharides, surfactants and phospholipids.28–30 Additional materials (such as minerals, solid particles, or biopolymers) may adsorb on top of these emulsifier layers. Consequently, lipid droplet coatings may vary in their electrical charge, thickness, permeability, environmental responsiveness, resistance to displacement, and susceptibility to enzymatic digestion.27 The lipid droplets themselves may vary in their physical dimensions (e.g., particle size distribution and shape) and in their aggregation state (e.g., isolated, flocculated or partially coalesced). There may also be considerable variations in the physicochemical and structural properties of the matrix that surrounds the lipid droplets. The droplets may be simply dispersed within a low viscosity aqueous liquid (as in soft drinks, nutritional beverages, or milk), they may be embedded within larger particles (as in filled hydrogel particles), or they may be distributed within macroscopic gel-like or solid matrices (as in jellies, meat products, cheese, ice cream, or hard candies). Many of these factors may impact the behavior, digestibility, and ultimate fate of lipid droplets within the human GI tract. Indeed, there have been concerted efforts recently to rationally design emulsion-based delivery systems to control lipid digestibility based on knowledge of these and other factors.4,5,14,21,22,31
2.1.2. Mouth.
The main function of the mouth is to ingest the foods, and to convert them into a form suitable for swallowing. The composition, structure, and properties of lipid droplets change appreciably during mastication due to the complex physiochemical and physiological processes that occur within the human mouth.32–35 An ingested food or beverage undergoes a number of processes: it is mixed with saliva; it may change its pH, ionic strength, and temperature; it may be acted upon by various digestive enzymes (e.g., lingual lipase, amylase, protease); it may interact with biopolymers in the saliva (e.g., mucin); it may interact with the surfaces of the tongue and mouth; it experiences a complex flow profile; and, it may be physically broken down into smaller pieces by chewing.36–43 Ideally, all of these factors should be simulated in an in vitro digestion model, although in practice many of them are often ignored because they are assumed not to have a major impact on lipid digestion.
One of the most important factors that influence the behavior of emulsified lipids in the mouth is their interaction with saliva. Human saliva is usually around pH 5.5 to 6.1 during fasting and around pH 7 to 8 after food ingestion.44 Saliva is typically secreted at a rate of about 0.2 to 4 ml per minute,35 with a total saliva output of 500 to 1500 mL per day.36 Saliva contains water (∼99%), minerals (<1%), and proteins (0.1–0.2%). The protein fraction is compositionally complex with many different kinds of molecules, including enzymes, immunoglobulins, antibacterial proteins, proline-rich proteins and glycosylated proteins (mucins).45–47 The mucins are capable of inducing coalescence and flocculation of ingested lipid droplets, which has been attributed to depletion and bridging mechanisms.36,41 In practice, it is difficult to accurately simulate the behavior of mucin using in vitro digestion models because there are large variations in the amount, composition, and properties of saliva for a given individual at different times, as well as between individuals.36
A food or beverage usually spends a relatively short time in the mouth before being swallowed,34 with the time depending on the nature of the ingested material, with liquids spending much less time in the mouth than solids that need masticating to break them into smaller pieces.35 Typically, swallowing only takes a few seconds to complete, and involves the integrated movements of parts of the tongue, pharynx, esophagus and stomach.48 The structural organization of the lipids within the mouth depends on their initial structural organization within the food, the duration and intensity of mastication, and the physiological characteristics of the individual consuming the food. A limited amount of lipid digestion may occur during mastication due to the presence of lingual lipases secreted by glands within the mouth. These lingual lipases are usually more important in human infants than in adults, and in rodents than in primates.49 Recently, it has been found that the mouth contains receptors capable of detecting fat and releasing signals that stimulate the body's ability to digest and absorb lipids.26 Consequently, it may be more important to monitor and control the behavior and digestion of the lipid phase within the mouth than previously believed.
The material that is swallowed after mastication of a food is referred to as the “bolus”.50
2.1.3. Stomach.
The stomach can be considered to be a bag-like structure where the food is processed and stored prior to being transferred to the small intestine.51 The rate at which the stomach is emptied can influence the subsequent rate and extent of nutrient digestion and absorption in the small intestine, as well as influencing the feeling of satiety.52,53 The stomach consists of three main regions with different physiological functions: the cardia (upper section), fundus (middle section) and antrum (lower section). The main function of the fundus is to secrete gastric juice containing acids and digestive enzymes, while the main function of the antrum is to generate mechanical forces that mix, disrupt and transport the stomach contents.52,53
After the bolus is swallowed it rapidly passes through the esophagus and into the stomach (Fig. 1), where it is mixed with the acidic digestive juices containing gastric enzymes, minerals, surface active materials, and various other biological components, and is also subjected to mechanical agitation due to stomach motility.54,55 The pH of the human stomach has been reported to be between 1 and 3 under fasting conditions,44,56,57 but to change considerably after the bolus enters the stomach.44 Usually, there is an appreciable increase in the pH of the stomach contents after food ingestion, followed by a gradual decrease over the next hour or so until a value around pH 2 is reached. The pH-time profile depends on the initial pH, buffering capacity, composition, and quantity of food ingested.44 The high acidity of the stomach plays a number of important physiological roles, including activating enzymes, hydrolysis of food components, and inactivation of microorganisms. In the fasted state, the ionic strength of the stomach contents are around 100 mM, with the major ionic species being: Na+ (70 ± 30 mM); K+ (13 ± 3 mM), Ca2+ (0.6 ± 0.2 mM) and Cl− (100 ± 30 mM).57 There is usually an appreciable increase in the ionic strength of the stomach contents after food ingestion due to the additional ions arising from the food.44
The stomach goes through a variety of contractive motions that mix the bolus with the digestive juices, breakdown any large fragments within the food, and transport the resulting material into the small intestine at controlled rate.54,55,58–60 These contractions vary in amplitude, frequency and duration depending on whether the stomach contains food or not. An ingested food component may remain in the stomach for a period ranging from a few minutes to a few hours depending on its quantity, physical state, dimensions, structure, and location. Typically, the amount of food remaining within the stomach after ingestion decreases by about 50% in 30 to 90 min, with fluid components being emptied more rapidly than solid components.54,55 The minimum size of any particles that can pass from the stomach into the small intestine via the pylorus is about 1 to 2 mm.51 Particles with larger dimensions will remain in the stomach until they have been broken down further. These particles may be fragments of a solid or gel-like food that was ingested, or they may be formed within the stomach itself e.g., due to the formation of a biopolymer gel under acidic conditions.61 Thus, the fracture properties of the food material consumed and the degree of mastication within the mouth may have a large impact on the subsequent digestion any encapsulated lipid droplets in the GI tract.
Appreciable digestion of emulsified lipids usually begins in the stomach due to the presence of gastric lipase. Human gastric lipase is a highly glycosylated globular protein with a molecular weight of about 50 kDa, that is stable in gastric acid juices over a wide range of pH values (2 to 7).62–64 Human gastric lipase has been reported to have an isoelectric point of 6.6 to 7.9 depending on the iso-form,65 consequently it should be positively charged in the highly acidic conditions in the human stomach. The concentration of gastric lipase in the stomach is typically between about 0.5 and 1 μM.
Gastric lipase binds to the surface of the lipid droplets, where it converts the encapsulated tricylglycerols (TAG) into diacylglycerols (DAG), monoacylglycerols (MAG), and free fatty acids (FFA).64,66,67 Gastric lipase has a preference for hydrolyzing fatty acids at the sn-3 position of the glycerol backbone of the TAG molecules.26 Lipid hydrolysis usually stops when the droplet surface becomes covered with free fatty acids, which occurs when about 4–40% of the fatty acids have been released from the TAGs, with the amount depending on droplet size.15,26 The extent of digestion is usually greater for smaller droplets (with a larger surface area), than for larger droplets (with a lower surface area), since the former have more surface area. The physicochemical mechanism for the inhibition of gastric lipase by fatty acids is believed to be the trapping of the lipase within a colloidal structure (200 nm) that is comprised of FFA, DAG, MAG and phospholipids.1 The trapped lipase is prevented from coming into close contact with the TAG at the oil–water interface and so its activity is decreased.
The FFA released in the stomach may play an important role in the subsequent digestion and absorption of foods: it may promote lipid digestion by enhancing droplet disruption; it may increase solubilization of digestion products in mixed micelles; it may stimulate hormone release, which stimulates the secretion of bile and pancreatic juices; it may increase the binding of co-lipase; it may increase the activity of pancreatic lipase in the small intestine.49,63 In particular, recent studies indicate that there are fat receptors in the stomach that may trigger the release of biological signals (such as CKK) that stimulate the release of pancreatic lipase and that slow down the emptying of the stomach.26
The partially digested and disrupted food that leaves the stomach and enters the small intestine is usually referred to as “chyme” (Fig. 1).
2.1.4. Small intestine.
The small intestine is the region in the GI tract where most of the lipid digestion and absorption processes normally occur. It can be considered to be a tube-like structure (about 2.5 to 3 cm in diameter) consisting of three major regions: duodenum (about 26 cm long); jejunum (about 2500 cm long); and, ileum (about 3500 cm long).51 The actual surface area of the small intestine is much greater than that calculated for a simple smooth tube because of the complex topology of the inner lining, e.g., villi and crypt structures.51
After entering the duodenum, the chyme is mixed with sodium bicarbonate, bile salts, phospholipids, and enzymes secreted by the liver, pancreas and gall bladder.50 The sodium bicarbonate secreted into the small intestine causes the pH to increase from highly acidic (pH 1 to 3) in the stomach to around neutral (pH 5.8–6.5) in the duodenum, where the pancreatic enzymes work most efficiently.63 Nevertheless, studies with human subjects have shown that there may be large variations in both stomach and duodenum pH.44 The bile salts and phospholipids originating from the liver (via the gall bladder) are surface-active and can facilitate emulsification of the lipids by adsorbing to the droplet surfaces.50 Lipid hydrolysis continues within the duodenum through the actions of lipases originating from the pancreas.62,64 Lipids and lipid digestion products (e.g., FFA, sn-2 MAG, cholesterol, phospholipids, and fat-soluble vitamins) are solubilized within mixed micelles and vesicles consisting of bile salts and phospholipids at the surface of the lipid droplets, and are then transported to the epithelium cells for absorption. The mixed micelles and vesicles must pass through the mucous layer that coats the epithelium walls before it can reach them.
In the fasted state, the ionic strength of the small intestine has been reported to be about 140 mM.57 After ingestion of food, there is usually an appreciable increase in ionic strength due to the additional ions arising from the food. The ionic strength is particularly important because it influences the magnitude and range of any electrostatic interactions in the system. The type of ions may also be important since it is known that multivalent cations (such as Ca2+ and Mg2+), may promote precipitation of bile salts and long chain saturated fatty acids in the small intestine, which has a major impact on lipid digestibility.68–70 In addition, multivalent ions may interact with certain kinds of biopolymers (such as alginate) to form gels that can inhibit lipid digestion.
Bile salts play an extremely important role in the lipid digestion and absorption processes. Bile salts are surface-active molecules that can adsorb to oil–water interfaces and can form micelles and other association colloids in water. They are therefore capable of facilitating lipid droplet deformation and break up during mechanical agitation, of stabilizing lipid droplets against aggregation, and forming (mixed) micelles that solubilize and transport hydrophobic molecules. In vivo they usually perform these roles in conjunction with other surface active substances, such as phospholipids, MAGs and FFAs. Bile salts are synthesized from cholesterol in the liver. They have structures that differ appreciably from other surfactants, because they do not consist of a hydrocarbon tail and a hydrophilic head group. Instead, they are fairly large rigid “plate-like” molecules that have a hydrophobic side and a hydrophilic side. The hydrophobic side can interact with substances that have a non-polar character (e.g., other bile salts or lipid droplet surfaces), whereas the hydrophilic side can interact with substances that have a polar character (e.g., water). The “backbone” of bile salt molecules is cholic acid, which may be conjugated with the amino acids taurine or glycine in the liver, thereby increasing its water-solubility.63 In the fasted state, the level of bile salts in the duodenum is around 4.3 to 6.4 mM, while after ingestion of a meal it increases to around 5 to 15 mM.44 Bile salts may adsorb to freshly formed oil–water interfaces, or they may displace other surface active substances already at oil–water interfaces.
As mentioned earlier, pancreatic lipase plays a critical role in the lipid digestion process because it is the digestive enzyme responsible for converting TAGs and DAGs into FFAs and MAGs (sn-2 position). Pancreatic lipase preferentially hydrolyses fatty acids in the sn-1 and sn-3 positions on the glycerol backbone, and has little specificity for fatty acid chain length.49 To catalyze this reaction the pancreatic lipase must adsorb to the oil–water interface so that it is in close proximity to the TAG and DAG molecules.71 Lipase usually does this as part of a complex with co-lipase and possibly bile salts.63 The extent of binding of pancreatic lipase to the droplet surfaces depends on the initial interfacial composition and properties, as well as the presence of any surface active substances in the continuous phase.63 Competitive adsorption processes occur at the oil droplet surfaces between the enzyme complex, bile salts, phospholipids, digestion products, and other surface-active substances, which could interfere with lipase adsorption to the droplet surfaces.71 Droplet surfaces may be coated with indigestible layers (such as dietary fibers72 or silica particles73) that inhibit the direct access of the lipase to the encapsulated lipids. The electrical charge on the interface may affect any electrostatic interactions between the enzyme complex and the droplet surfaces. The pH optimum of pancreatic lipase is around pH 8.5, but it also operates well around neutral pH.63 A co-enzyme known as “co-lipase” is required for the optimum activity of lipase. It has been proposed that this co-enzyme forms a stoichemetric complex with lipase that adsorbs to the oil–water interface and brings the lipase into close contact with the lipid substrate. Co-lipase is a polypeptide with a molecular weight of about 10 kDa, which has a hydrophobic side that is believed to bind to the oil–water interface, and a hydrophilic side that is believed to bind the lipase. Co-lipase is secreted from the pancreas as pro-co-lipase, and then is converted into its active form when trypsin cleaves off a peptide called enterostatin. This peptide is believed to be a hormone that regulates satiety, reduces fat intake, and inhibits pancreatic secretion.63
The presence of bile salts may either promote or inhibit the activity of pancreatic lipase depending on their concentration.63,74 At relatively low concentrations, they tend to promote lipase activity, which can mainly be attributed to their ability to solubilize lipid digestion products and remove them from the oil–water interface, e.g., sn-2 MAGs and FFAs. If these products are not removed from the droplet surfaces, then the fatty acids would accumulate at the oil–water interface thereby preventing the lipase from adsorbing and getting access to the TAGs and DAGs within the droplets. In addition, if the lipid digestion products were not removed from the site of enzyme activity, then the conversion of TAGs to MAGs and FFAs would be inhibited by the high local concentration of reaction products. On the other hand, relatively high bile salt concentrations may inhibit the ability of lipase to digest emulsified lipids. This effect can be attributed to the ability of the bile salts to compete for the oil–water interface with the lipase, thereby preventing it from coming into close proximity to the lipid substrate.75 One of the key roles of the bile salts is to form micelles that solubilize and transport the lipid digestion products from the lipid droplet surfaces to the intestinal membrane where they are absorbed. It is therefore important that the bile salt concentration exceeds the critical micelle concentration, which is usually around 1 to 2 mM.63 In practice, the bile salt concentrations in the small intestine are above the CMC: ∼6–15 mM in the duodenum (being higher after consumption of a meal); ∼10 mM in the jejunum; <4 mM in the ileum (where the bile salts are reabsorbed by the body).
Once the lipid digestion products have been transported across the mucous layer in micelles and vesicles they are absorbed by the intestinal enterocyte cells. The mucous layer has been reported to be a stagnant layer (i.e., transport is mainly diffusion controlled) with a thickness between 30 to 100 μm.76 The subsequent fate of the digestion products (FFA and MAG) depends largely on the molecular weight of their hydrocarbon chains. Long chain fatty acids (LCFA) tend to be reassembled into TAGS within the epithelium cell, packaged into colloidal particles (lipoproteins), and then transported to other tissues via the lymphatic system.15,63,77 On the other hand, short chain fatty acids (SCFA) and medium chain fatty acids (MCFA) tend to be absorbed directly into the portal vein and pass through the liver before entering the systemic circulation.3
2.1.5. Colon.
The material that is not absorbed within the small intestine passes from the ileum to the large intestine, which is a tube-like structure with a length of about 1500 mm.78 The large intestine can be divided into four major regions that differ in their physiological functions: caecum, colon, rectum, and anal canal. The colon itself can be divided into four regions, the ascending, transverse, descending, and sigmoid regions. The main physiological functions of the colon are the absorption of water and electrolytes, the fermentation of polysaccharides and proteins, the re-absorption of bile salts, and the formation, storage and elimination of fecal matter.51,78 The pH of the colon varies from region to region,78 with lower pH values occurring in regions where dietary fibers are fermented and short chain fatty acids (SCFA) are released.79 It has been reported that pH values around 5.5, 6.2 and 6.8 represent the proximal, transverse, and distal regions of the colon.80
Any material that is undigested in the upper GI tract will reach the colon. Normally, lipids would be fully digested in the stomach and small intestine, but some undigested lipid may pass through the upper GI tract, particularly in specially designed emulsion-based delivery systems. The droplets in oil-in-water emulsions prepared using a non-digestible lipid phase (such as a mineral oil or alkane) can reach the colon. If lipid droplets are surrounded by an indigestible coating or embedded within an indigestible matrix (e.g., dietary fiber), then they may not be fully digested in the small intestine. Under these circumstances it may be important to consider the processes that occur in the colon. The colon contains a large number of different kinds of anaerobic bacteria species, which are capable of fermenting food components that were not digested in the upper GI tract, e.g., dietary fibers, proteins and peptides.80 Thus, any lipids encapsulated within dietary fiber matrices may only be released after they reach the colon due to bacterial fermentation. It has been suggested that some undigested food ingredients may interfere with the normal metabolic activity of the colonic microbiota, which could alter the health status of the lower GI tract and even the whole body.81,82 Consequently it is important to consider this factor when designing any delivery system that might alter the normal digestibility of food components.
2.2. Key features of gastrointestinal fluids
A number of the most important processes that occur when emulsified lipids pass through the various regions of the human GI tract were highlighted in Section 2.1. Based on this information, we highlight some of the key parameters that need to be taken into account when developing in vitro models to simulate lipid digestion.
2.2.1. pH.
There are considerable variations in the pH of the aqueous medium surrounding the lipid droplets as they pass through the GI tract: mouth (pH ≈ 7); stomach (pH ≈ 1–3); small intestine (pH ≈ 6–7); large intestine (pH ≈ 5.5–7). These pH variations may cause considerably changes in the rate and extent of lipid digestion. Solution pH determines the physicochemical properties of many of the components within the GI tract, e.g., charge, solubility, aggregation state, physical interactions, and chemical reaction rates. The water-solubility of many minerals is highly dependent on pH, so that they may go from soluble to insoluble in different regions of the GI tract. The electrical charge of many emulsion droplets is pH-dependent, particularly those stabilized by amphiphilic proteins, polysaccharides, and ionic surfactants. The stability of many emulsions to droplet flocculation and coalescence depends on the magnitude and sign of their electrical charge.27 Protein-coated lipid droplets change from negative to positive when the pH is reduced from above to below their isoelectric point (pI). A positively charged droplet may interact with negatively charged components or surfaces within the GI tract, and vice versa. A protein-coated lipid droplet may aggregate near its isoelectric point since the electrostatic repulsion is no longer sufficient to prevent droplets from coming into close proximity. Some anionic polysaccharides, such as alginate and pectin, have pKa values around 3.5, and so they may have little or no negative charge in the highly acidic conditions within the stomach but have a high negative charge in the neutral conditions in the small intestine. The cationic polysaccharide chitosan, which is commonly used in fabricating emulsion-based delivery systems, has a pKa value around 6.5, and so it has a high positive charge at acidic pH but little or no positive charge at neutral pH. Consequently, any biopolymer matrices held together by electrostatic interactions may change their properties in different GI fluids (e.g., swell, shrink or disintegrate), which could impact the ability of lipase to interact with the lipid droplet surfaces.
2.2.2. Ionic composition.
There may be considerable variations in the type and concentration of ions surrounding the lipids droplets, which may impact the electrostatic interactions in the system through electrostatic screening or binding effects. For example, long chain fatty acids may precipitate in the presence of calcium ions, thereby removing them from the lipid droplet surface (which facilitates further digestion), but which may also reduce their subsequent absorption due to calcium soap formation.83 Sufficiently high concentrations of monovalent and multivalent counter-ions can promote extensive flocculation in emulsions containing electrically charged droplets, which may restrict the access of lipase to the oil–water interface and slow down digestion.84 Certain types of mineral ions are capable of promoting the gelation of biopolymers within the GI tract, which would affect the ability of digestive enzymes to reach any entrapped lipid droplets. For example, alginate or pectin form strong gels if there are sufficiently high levels of free calcium ions present in solution.85 If any calcium binding agents are present within a food product, such as EDTA or alginate, they may reduce the amount of free calcium present, which will then alter the ability of calcium ions to interact with other food components.84
2.2.3. Enzyme activity.
There are various kinds of enzymes in the mouth, stomach, small intestine and colon that can digest food components, such as lipids (lipases), proteins (proteases), starch (amylases) and dietary fibers (glycosidases).50 The ability of these enzymes to interact with their specific substrates within a food may impact lipid digestibility and the absorption of encapsulated lipophilic components. Enzyme accessibility to a substrate may be influenced by physical barriers between the encapsulated substrate and the surrounding aqueous phase where the digestive enzymes are normally located. Lipid digestion may not be initiated until the lipid droplets are released from their original emulsifier coatings or from any matrices that they are encapsulated in. The rate of lipid digestion may be decreased by coating lipid droplets with a dietary fiber layer86 or by embedding them within dietary fiber particles.87,88 Similarly, it may be necessary for protein or starch coatings or particles to be digested by proteases or amylases before the lipase can act on the lipids. Enzyme activity may also be influenced by any food components that can bind to them, either specifically or non-specifically. For example, some foods contain natural enzyme inhibitors, such as peptides from soybeans that inhibit proteases89 and polyphenols from fruits and vegetables that inhibit lipases.90
2.2.4. Surface active components.
There are a variety of endogeneous (e.g. proteins, peptides, phospholipids, and bile salts), exogeneous (e.g., surfactants, proteins), and internally generated (e.g., lipid and protein digestion products) surface active substances present within the aqueous phase surrounding the lipid droplets. These substances compete with the surface-active substances already present at the oil–water interface, potentially leading to changes in interfacial composition and properties.71,91 Some of these surface active components also play a crucial role in solubilizing lipid digestion products (MAG and FFA) and lipophilic components and carrying them to the epithelium cells for absorption. The mixed micelles and vesicles present responsible for solubilizing and transporting highly lipophilic components within the aqueous phase consist of bile salts and phospholipids secreted by the body, as well as MAG and FFA from the digested lipids.
2.2.5. Flow profiles and mechanical forces.
The encapsulated lipids may be exposed to various kinds of forces and flow profiles during their passage through the human body.51 These processes mix the various components together, breakdown structures (lipid droplets, protein particles, hydrogel matrices etc), and transport materials from one location to another. It is therefore important to simulate or model these flow profiles in in vitro digestion models.
3. Key physicochemical events occurring during lipid digestion
A number of the key physicochemical events that occur during the lipid digestion process are highlighted in this section (Fig. 2).
3.1. Matrix disruption
The lipid droplets in a food are surrounded by a matrix that may be liquid-like, gel-like, or solid-like. The disruption of this matrix within the GI tract may have an important impact on lipid digestion, since it determines how easily digestive enzymes and other components can access the emulsified lipids, as well as how easily any digestion products can leave the droplet surfaces. If the lipid droplets are dispersed within a simple low viscosity fluid (as in milk, nutritional beverages, or soft drinks), then they will be rapidly dispersed within the digestive juices. The surface of the lipid droplets will then be readily accessible to any surface active components and enzymes present in the surrounding aqueous media. On the other hand, if the lipid droplets are trapped within a gel-like or solid-like matrix, then it may be necessary for this matrix to be disrupted before the digestive components can access the lipid droplet surfaces. Alternatively, the digestive enzymes and other components may have to diffuse through this matrix before they can reach the lipid droplets. The rate at which these molecules diffuse depends on the pore size of the matrix, as well as any specific attractive interactions between the molecules and the matrix material. The initial size of the matrix particles, as well as their response to changes in environmental conditions as they pass through the GI tract, may therefore influence the rate and extent of lipid digestion. The matrix material surrounding a lipid droplet may respond to changing environmental conditions in a number of ways: (i) remain intact; (ii) swell or shrink; (iii) physically, chemically or enzymatically degrade; or (iv) physically fragment. The behavior of a particular matrix material will depend on the type and interactions of the molecules it contains. For example, some biopolymers are enzymatically digested in the stomach or small intestine (e.g., starches and proteins), whereas others are indigestible (e.g., dietary fibers and resistant starch). Electrostatic complexes formed between anionic polysaccharides and proteins at low pH values, may dissociate when the pH is raised above the isoelectric point of the protein because this weakens the electrostatic forces holding them together.92 This kind of information can be used to design matrices that control the digestion and release of lipids within the GI tract.14
3.2. Alterations in interfacial properties
The lipid droplets in foods are usually coated by an interfacial layer that consists of emulsifiers and any other substances that adsorb to the droplet surfaces, e.g., mineral ions, biopolymers or solid particles.27 After a food is consumed there may be appreciable changes in the properties of the interfacial coatings as the droplets pass through the GI tract. The original emulsifier molecules may be digested by enzymes, e.g., phospholipids by phospolipases, proteins by proteases, or non-ionic surfactants with ester bonds by esterases.93 The ability of the emulsifier molecules to stabilize the lipid droplets against aggregation may be changed considerably after they have been fully or partially hydrolyzed. The original emulsifier molecules (or their digestion products) may be displaced from the droplet surfaces by other surface-active components present in the system.94,95 These surface-active substances may come from the food itself (e.g., proteins, surfactants, phospholipids), or they may be generated as a result of the digestion process (e.g., FFA or MAG), or they may be secreted by the GI tract (e.g., bile salts, phospholipids, or proteins).63 Alternatively, components within the digestive juices may adsorb on top of the original layer of emulsifier molecules, e.g., anionic mucin molecules can form a coating around cationic protein-coated lipid droplets.96,97 All of these changes in interfacial composition may alter the subsequent susceptibility of the lipids to digestion.
3.3. Droplet fragmentation, aggregation, and dissolution
There may be considerable changes in the size and aggregation state of the lipid droplets in a food sample as it passes through the GI tract. Bulk fats or large fat droplets may be broken down into smaller droplets by the mechanical forces generated within various regions of the GI tract (such as mastication and swallowing in the mouth, churning in the stomach, passage through the pylorus, and peristaltic movements within the intestines). These processes may be facilitated by the presence of surface active components such as digestion products, phospholipids, bile salts, and proteins. If the interfacial layer surrounding the droplets is not strong enough, then the droplets may coalesce with each other when they collide within the GI tract, which leads to an increase in mean particle size.4,97 If the repulsive interactions between the lipid droplets are insufficiently strong, then the droplets may self-associate and form flocs.84 As the lipid phase within droplets is digested by lipase, there may be a decrease in droplet size due to movement of the digestion products from the droplet interior into the surrounding aqueous phase. Consequently, there may be appreciable changes in the particle size distribution and aggregation state of the lipid phase as it passes through the GI tract,15,63 which may influence the ability of enzymes to adsorb to the oil–water interface.
Microscopy studies have provided important insights into the structural changes that occur during lipid digestion.98,99 These studies have shown that digestion of emulsified lipids by pancreatic lipase in the small intestine takes place by a sequence of steps involving the formation of different phases. A liquid crystalline or crystalline phase is observed around the lipid droplet surfaces within the first few minutes of lipolysis, which gets thicker over time. Eventually, any undigested oil within the interior of the droplet may be expelled as a smaller oil droplet, leaving the liquid crystalline or crystalline phase behind. These latter phases are presumably formed due to the high local concentration of surface active lipids (FFA and MAG) at the droplet surfaces. The liquid crystalline phase tends to form at low calcium concentrations, whereas the crystalline phase forms at high calcium concentrations (due to fatty acid calcium soap formation). Nevertheless, in the presence of sufficiently high concentrations of bile salt micelles the lipid reaction products are solubilized and therefore the liquid crystalline phases may not be observed. The amount of bile salt micelles required to solubilize the reaction products depends on their solubilization capacity – once the available micelles have been saturated with digestion products, then liquid crystalline phases may be observed. A variety of different liquid crystalline phases may be formed depending on the nature of the original lipid, solution composition, and digestion time.98,99
3.5. Solubilization and mass transport processes
The efficiency of the digestion process depends on the mass transport of various reactants, catalysts, and products from one location to another. Digestive enzymes must come into close proximity to their substrates before they can carry out their biological actions. Thus, lipase must adsorb to the surfaces of lipid droplets before it can convert the encapsulated TAG molecules into MAG and FFA. These digestion products must then be incorporated within mixed micelles and vesicles, which must be transported across the mucous layer before they can be absorbed by the epithelium cells. Similarly, proteases have to adsorb to droplet surfaces if they are going to hydrolyze the adsorbed protein coatings.
Consequently, it is important to be aware of the various kinds of mass transport processes operating in the different regions of the GI tract, and to elucidate the major factors that impact them. Mass transport may occur predominantly by convective or by diffusive processes, depending on the nature of the food matrix and the specific region of the GI tract involved. The various mechanical forces generated by the GI tract tend to mix components together and move them from one location to another, e.g., the peristaltic actions of the intestine. Nevertheless, there are regions within the GI tract where mass transport is mainly diffusion-limited. The movement of digestion products solubilized within micelles and vesicles through the mucous layer is normally considered to be diffusion-limited. If lipid droplets are trapped within viscous, gel-like, or solid matrices, then any digestive enzymes may have to diffuse through these matrices before they can reach their substrates. In this case, the trapped droplets would be digested at a slower rate than free droplets because of the longer time taken for lipase to reach the droplet surfaces.
3.6. Binding interactions
Foods are highly complex systems that often contain a wide variety of different components, including sugars, salts, proteins, polysaccharides, lipids, vitamins, etc. Many of these components are capable of interacting with each other and forming complexes that could potentially alter the rate and extent of lipid digestion. In this section, we provide a few examples of some binding interactions that could occur, while acknowledging that there are many others possible. Indigestible polysaccharides (dietary fibers) may interact with other molecular species in the GI tract through electrostatic or hydrophobic interactions. For example, cationic chitosan can bind anionic bile salts and free fatty acids under simulated GI conditions, which may have a major impact on lipid digestion and absorption.100 Ionic dietary fibers may bind to the surfaces of oppositely charged lipid droplets where they form a protective coating that inhibits lipase adsorption and activity. Multivalent mineral ions can form electrostatic complexes with oppositely charged species, thereby altering their solubility, aggregation state, and physicochemical properties. For example, calcium ions can form electrostatic complexes with long chain fatty acids, which can promote lipid digestion by removing the FFAs from the lipid droplet surfaces, but which can also reduce the subsequent absorption of the FFAs by forming insoluble soaps. Some food components may be able to bind directly to digestive enzymes and thereby alter their activity or ability to bind to lipid droplet surfaces, e.g., polyphenols. At present there is a fairly poor understanding of how different components within complex foods alter the behavior and digestibility of lipids within the human GI tract. Clearly further research is required in this area using well characterized complex food products.
4. Lipid bioavailability
The term bioavailability has been defined as the fraction of an ingested component (or its products) that eventually ends up in the systemic circulation.101,102 For lipophilic components, the bioavailability (F) can be defined as:101Here, FB is defined as the bioaccessibility coefficient or fraction of the lipophilic components that is released from the food matrix into the juices of the gastrointestinal tract, FT is defined as the transport coefficient or the fraction of the released lipophilic components that are transported across the intestinal epithelium; and FM is the fraction of the lipophilic components that reaches the systemic circulation without being metabolized. The value of FM depends on the pathway that the lipophilic components follow to reach the systemic circulation, e.g., short chain fatty acids (SCFA) and medium chain fatty acids (MCFA) pass through the portal vein and liver (where they may be metabolized), while long chain fatty acids (LCFA) pass through the lymph system (thereby avoiding the first pass through the liver).3,50 LCFA are reassembled into TAGs in epithelium cells, packaged into colloidal structures, and then leave the epithelium cells, and enter the lymph system. Highly lipophilic bioactive components also tend to be transported via the lymph system, e.g., carotenoids. After the lipophilic components reach the systemic circulation they may be distributed between different tissues, where they may be stored, metabolized, or excreted.102,103 The relative rates of these various processes determine the time-dependence of the concentrations of the lipophilic component and its metabolites at specific locations within the body. The concentration-time profile of a specific lipid component at a particular site-of-action will determine its beneficial or adverse affects on human health and wellness. Consequently, it is usually important to measure the concentration of a lipid component at a particular location in order to establish its potential efficacy.103 When determining the bioavailability of bioactive lipophilic components it is important to account for the fact that they may be chemically modified during passage through the gastrointestinal tract prior to or after absorption, e.g., triglycerides are converted to monoglycerides and free fatty acids, whereas some lipophilic bioactives (such as polyphenolics) may be chemically derivitized.102,104
5. Overview of in vitro lipid digestion tests
5.1. Introduction
A number of in vitro approaches commonly used to study lipid digestion are highlighted in this section. Some of these approaches focus on one particular region of the gastrointestinal tract, whereas others utilize a number of sequential steps to more accurately mimic the entire digestion process.105In vitro digestion models can therefore be conveniently characterized as:
• Single Step Models: One particular region of the GI tract is simulated, e.g., the mouth, stomach, small intestine, or colon. The pH-stat method is an example of this type of model, which only simulates digestion in the small intestine (Section 6).
• Multiple Step Models: Two or more regions of the GI tract are simulated, e.g., mouth, stomach, small intestine, and colon. A multiple step in vitro digestion model designed to simulate the entire human GI tract is highlighted schematically in Fig. 3.
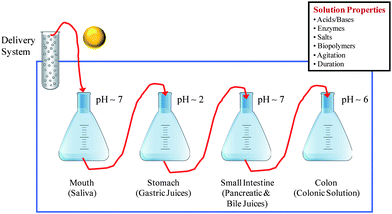 |
| Fig. 3 Schematic diagram of a multiple-step in vitro digestion model to simulate the whole of the GI tract. | |
Using either approach, a food sample is prepared and then subjected to one or more treatments designed to simulate specific regions of the human digestive tract, e.g., mouth, stomach, small intestine, and colon. These treatments usually involve mixing the food sample with a simulated digestive fluid of specific composition (e.g., pH, mineral composition, enzyme activity, etc) using controlled mixing conditions. All samples and solutions are normally maintained at 37 °C to mimic human body temperature. The level of sophistication of the simulated digestive fluids and mixing conditions used varies widely between different models. The composition, structure, and/or physicochemical properties of the sample being tested can be measured at specific points throughout this process (Section 5.3). Sophisticated analytical instruments specifically designed to simulate the full digestion process are now commercially available, e.g., the TIM™ lipid absorption system from TNO Quality of Life, The Netherlands.106,107 In this review, we mainly focus on those in vitro methods that use equipment available in most research laboratories, such as glassware, stirrers, and pH meters.
5.2. Simulation of different regions in GI tract
To accurately model the complex physiological and physicochemical events that occur within the human GI tract it is necessary to simulate the composition, structure, and dynamics of the various intestinal fluids that the lipid droplets encounter.107 In reality, this is often impractical because the in vitro digestion model would become too complicated, time consuming, and expensive to set up and operate. Consequently, researchers often only utilize the key components that are believed to impact the particular system that they are working with, and ignore the other minor components. For example, proteases and amylases may be omitted if the system studied does not contain proteins or starches. Similarly, the wide variety of different monovalent and multivalent ions present in the GI tract may be simulated by simply using NaCl and CaCl2, respectively. One limitation of this approach is that we currently do not have the detailed knowledge of how many of these components influence the behavior of lipid droplets in the GI tract. On the other hand, the simplicity of these models allows one to rapidly screen many samples, and provide some mechanistic understanding of the processes involved. Promising candidate formulations can then be tested using more sophisticated in vitro models, animal feeding studies, or human trials. In the following sections, we highlight some of the most important factors that need to be simulated in each region of the GI tract.
5.2.1. Mouth.
The major factors to consider when designing an in vitro digestion step that simulates the human mouth are the potential interactions of the lipid droplets with saliva, tongue, and oral cavity (Section 2.1.2). Most researchers ignore droplet interactions with the tongue and oral cavity because of the difficulty in accurately mimicking these events in the laboratory. Instead, the emulsion to be tested is mixed with a simulated saliva fluid (SSF) under specific conditions (shearing, time, temperature). The compositional complexity of the SSF used in mouth studies varies widely depending on the objectives of the researchers. Some researchers use a simple buffer solution (e.g., pH 7) without any additional components to simulate oral conditions. Other researchers use SSF that contains many of the components found in human saliva, such as acids, buffers, minerals, biopolymers, and enzymes.37,108 Recipes for preparing simulated saliva solutions have been published.43,108 Some researchers simply ignore the oral step altogether (Table 1), assuming that it does not have an impact on lipid digestion. This is likely to depend on the nature of the sample tested, and particularly on emulsifier type. For example, some emulsions undergo extensive flocculation and coalescence in the mouth due to their interactions with the mucin in saliva.36,43 These changes in droplet characteristics (size and interfacial properties) could alter the subsequent digestion of the lipids in the stomach and small intestine.
Table 1 Summary of previous studies using in vitro digestion models used to investigate the digestion and absorption of emulsified food lipids. Samples Tested Key: SEDS = Self-emulsifying delivery system; O = Oil; E = Emulsifier. Digestion Steps Key: M = mouth, S = stomach; SI = small intestine; C = colon. All were carried out at 37 °C unless stated. Emulsifier type: LF = lactoferrin; BLG = b-lactoglobulin; WPI = whey protein isolate; Cas = caseinate; T20 = Tween 20; Chit = Chitosan; Alg = Alginate; MG = monoglyceride; CHO = carbohydrate
Samples Tested |
Experimental Variables |
Parameters Measured |
Digestion Steps Modeled |
Comments |
References |
O/W Emulsions
|
[Bile] |
Optical microscopy |
• M: pH 7 (1 h) |
Digestion rate increased with [Bile] and dependent on PS |
202 |
O = Tuna |
[Lipase] |
ζ-potential |
• S: pH 2 (1 h) |
|
E = Lec, Lec/Chit |
Polysaccharides |
Particle size |
• SI: pH 5.3, pancreatic lipase, bile (2 h) |
|
Appearance |
• SI: pH 7.5 (2 h) |
|
FFA, Glucosamine |
|
|
O/W Emulsions
|
[Bile] |
Optical microscopy |
• SI: pH 7.5, CaCl2 (2 h) |
Protein charge effects displacement by bile salts |
132 |
O = soy oil |
Emulsifier type |
ζ-potential |
|
|
E = LF or BLG |
|
Particle size |
|
|
|
|
Protein Adsorbed |
|
|
O/W Emulsions
|
Emulsifier type |
Optical microscopy |
• SI: (Pancreatic lipase, bile salts) |
Digestion rate and extent depends on emulsifier type: Cas, WPI > Lec > T20 |
131 |
O = corn oil |
|
ζ-potential |
|
|
E = Cas, WPI, Lec, T20 |
|
Particle size |
|
|
|
|
FFA |
|
|
SEDS
|
Formulation |
Microscopical images, droplet size |
• SI: (Pancreatic lipase, bile salts) |
Digestion induces a change in lipid composition which affects the solubilization capacity of the lipid phase |
206 |
O = MCT |
|
|
|
|
E = Solutol® |
|
|
|
|
O/W Emulsions
|
Emulsifier type |
Optical microscopy |
• SI: (Pancreatic lipase, bile salts, pH 7) |
Protein interfacial cross-linking did not have a big impact on lipid digestion |
207 |
O = corn oil |
BLG cross-linking |
ζ-potential |
|
|
E = BLG or Lec |
|
Particle diameters |
|
|
|
|
Free fatty acid release |
|
|
O/W Emulsions
|
Emulsifier type |
Confocal microscopy |
• M: (α-amylase, Mucin, BSA) |
Emulsifier type effects aggregation behavior of lipid droplets throughout in vitro digestion model |
192 |
O = soybean oil |
|
ζ-potential |
• S: (Pepsin, Mucin) |
|
E = Cas, WPI, Lec, T20 |
|
Particle size |
• SI: (Pancreatin, Lipase, Bile salt) |
|
|
|
Creaming stability |
|
|
O/W Emulsions
|
Dietary fiber type |
Microscopy |
• M: pH 7 (1 h) |
Dietary fiber type effects aggregation behavior of lipid droplets throughout in vitro digestion, through bridging and/or depletion mechanisms. |
195 |
O = Corn oil |
|
Creaming stability |
• S: pH 2 (1 h) |
|
E = T20 |
|
ζ-potential |
• SI: pH 5.3, pancreatic lipase, bile (2 h) |
|
|
|
Particle size |
• SI: pH 7.5 (2 h) |
|
O/W Emulsions
|
Lipid physical state |
ζ-potential |
• SI: (Pancreatic lipase, bile salts, pH 7) |
Solid fat particles are digested more slowly than lipid fat droplets. |
155 |
O = Tripalmitin |
|
Particle size, DSC |
|
|
E = SDS |
|
FFA release |
|
|
O/W Emulsions
|
Emulsifier type |
ζ-potential |
• SI: (Pancreatic lipase, bile salts, pH 7) |
Lipid droplets with different initial protein compositions are all digested |
208 |
O = Corn oil |
Multilayer |
Particle size, DSC |
|
|
E = Cas, LF or Cas/LF |
|
FFA release |
|
|
O/W Emulsions
|
Oil type |
ζ-potential |
• SI: (Pancreatic lipase, bile salts, pH 7) |
Digestion rate increases with decreasing droplet size, decreasing oil molecular weight, but does not depend strongly on emulsifier type |
172 |
O = Corn oil, MCT |
Particle size |
Particle size |
|
|
E = BLG, T20, Lec |
Lipid content |
FFA release |
|
|
|
Emulsifier type |
Microscopy |
|
|
O/W Emulsions
|
Polysaccharide type |
ζ-potential |
• SI: (Pancreatic lipase, bile salts, pH 7) |
The rate and extent of lipid digestion was decreased when chitosan and chitosan/alginate coatings were present around droplets |
209 |
O = Fish oil |
Multilayer formation |
Creaming |
|
|
E = Citrem, Chit, Alg |
|
Microscopy |
|
|
|
|
Particle size |
|
|
|
|
FFA release |
|
|
O/W Emulsions
|
Polysaccharides |
Optical microscopy |
• M: pH 7 (1 h) |
Increasing amounts of chitosan reduced the amount of FFA produced. Chitosan was degraded by lipase |
210 |
O = Tuna oil |
|
ζ-potential |
• S: pH 2 (1 h) |
|
E = Lec, Lec/Chit |
|
Particle size |
• SI: pH 5.3, pancreatic lipase, bile (2 h) |
|
|
|
Appearance |
• SI: pH 7.5 (2 h) |
|
|
|
FFA, Glucosamine |
|
|
O/W Emulsions
|
Polysaccharide type |
ζ-potential |
• SI: (Pancreatic lipase, bile salts, pH 7) |
Coating droplets with non-cross linked or cross-linked chitosan decreased the digestion rate. |
72 |
O = Corn oil |
TPP concentration |
Particle size |
|
|
E =lyso-Lec, lyso-Lec/Chit |
|
FFA release |
|
|
O/W Emulsions
|
Interfacial composition |
Optical microscopy |
• SI: (Pancreatic lipase, bile salts, pH 7) |
Coating droplets with chitosan decreased lipid digestion, but having an additional pectin coating increased digestion again. |
86 |
O = Corn oil |
|
ζ-potential |
|
|
E = Lec, Lec/Chit, Lec/Chit/Pec |
|
Particle size |
|
|
|
|
FFA |
|
|
O/W Emulsions
|
Bile type |
ζ-potential |
• SI: (Pancreatic lipase, bile salts, pH 7.5) |
FFA released increased with increasing calcium concentration by amount depending on bile type |
221 |
O = olive oil |
[Bile] |
Particle size |
|
|
E = phosphatidylcholine |
[Calcium] |
FFA |
|
|
Oil
|
Triglycerides type |
Lipolysis products |
• SI: (Pancreatic lipase/colipase, bile salts/, pH 7.5) |
The rate and extent of lipid digestion was faster for medium chain triglycerides than long chain triglycerides |
124,212 |
O = MCT, soybean oil |
|
|
|
|
O/W Emulsions
|
Triglycerides type |
Lipolysis products |
• S: pH 5.5 (0.5 h) |
Formulation effects solubilization of encapsulated drugs in mixed micelles |
213 |
O = triacylglycerols |
Emulsifiers |
|
• SI: pH 6.25, pancreatic lipase, bile (1 h) |
|
E = PEG |
|
|
|
|
O/W Emulsions
|
Protein type |
SDS-PAGE |
• S: pH 2.5 (1 h) |
Protein hydrolysis by proteases is altered when adsorbed to lipid droplet surfaces |
178 |
O = Olive oil |
Protein adsorption |
Particle size |
• SI: pH 6.5, pancreatic lipase, bile (0.5 h) |
|
E = β-Cas/BLG |
|
ζ-potential |
|
|
|
|
Optical microscopy |
|
|
|
|
Interfacial tension |
|
|
Oil suspension
|
Oil amount |
Bioaccessibility |
• S: pH 2.0 (1 h) |
Increasing the oil content increases the bioaccessibility of lipophilic components |
214 |
|
|
|
• SI: pH 7.5, pancreatic lipase, bile (0.5 h) |
|
SEDDS
|
Emulsifier type |
FFA released |
• SI: pH 6.5, pancreatic lipase, bile (0.5 h) |
Digestion rate increases with calcium addition, and depends on surfactant type |
175 |
O = Soybean oil |
NaCl & CaCl2 |
|
|
|
E = Tweens, Spans |
|
|
|
|
O/W Emulsion
|
Emulsifier type |
Particle size |
• SI: pH 7.0, pancreatic lipase, bile (0.5 h) |
Digestion rate depends on surfactant type |
84 |
O = Olive oil |
|
ζ-potential |
|
|
E = Galactolipids |
|
FFA released |
|
|
|
|
Interfacial tension |
|
|
Oil bodies & O/W emulsions
|
Emulsifier type |
Particle size |
• SI: pH 7.0, pancreatic lipase, bile |
Digestion of oil bodies is slower than emulsion droplets |
215 |
O = Sunflower seed oil |
Microstructure |
FFA released |
|
|
E = Natural, WPI or T20 |
|
Protein analysis |
|
|
O/W Emulsion
|
Emulsifier type |
Particle size |
• S: pH 1.5, pepsin (1 h) |
Interfacial covalent (Maillard) caseinate-carbohydrate complexes protect droplets against coalescence |
216 |
O = Fish oil |
|
ζ-potential |
• SI: pH 6.8, pancreatin, bile (2 h) |
|
E = Cas, Cas-CHO complex |
|
Microstructure |
|
|
|
|
FFA released |
|
|
O/W Emulsion
|
Emulsifier type |
FFA released |
• TIM Model - Dynamic |
The digestion rate depends on initial emulsifier type, with MG inhibiting digestion |
200 |
O = Fish oil |
|
Interfacial tension |
• S: Acid pH, pepsin, lipase, minerals |
|
E = MG, BLG, Lyso-Lec |
|
|
• SI: ≈ Neutral pH, pancreatin, bile |
|
O/W Emulsion
|
Emulsifier type |
Particle size |
• SI: pH 6.8, pancreatin, bile (0.5 h) |
Interfacial covalent (Maillard) caseinate-carbohydrate complexes reduced lipid digestion. |
217 |
O = Fish oil |
|
Viscosity |
|
|
E = Cas, Cas-CHO complex |
|
Microstructure |
|
|
|
|
FFA released |
|
|
Oil
|
Oil type |
Microstructure |
• SI: pH 6.5, pancreatin (10 min) |
Initial digestion rate increased with [Lipase] and depends on oil type |
163 |
O = SCT, MCT, LCT |
[Lipase] |
FFA released |
|
|
E = MG |
|
|
|
|
SMEDDS
|
Oil type |
FFA released |
• SI: pH 7.5, pancreatin, bile (0.5 h) |
Lipid composition can influence drug solubilization behavior and formulation affects the lipid digestion rate |
218,219 |
O = SCT, MCT, LCT |
|
|
|
|
E = Surfactants |
|
|
|
|
Oil suspension
|
Oil type |
Drug solubilization |
• SI: pH 7.5, pancreatin, bile (0.5/1 h) |
TG-drug suspension has relatively high solubilizing capacity of colloidal phases produced on TG digestion |
220 |
O = MCT, LCT |
|
|
|
|
O/W Emulsion
|
Extract Effects |
Particle size |
• S: pH 5.4, gastric juice (0.5 h) |
A green tea extract inhibits lipid digestion |
221 |
O = SCT, LCT |
|
FFA released |
• SI: pH 7.5, pancreatin, bile (1 h) |
|
E = Lec |
|
|
|
|
O/W Emulsion
|
Polysaccharide type (guar gum, gum arabic, pectin) |
Particle size |
• S: human gastric juice (0.5 h) |
Dietary fibers can inhibit lipid digestion by an amount depending on their structure |
222 |
O = Triolein |
|
Viscosity |
|
|
E = Lec |
|
FFA released |
|
|
O/W Emulsion
|
Droplet size |
Particle size |
• S: pH 5.4, gastric juice (0–0.5 h) |
Gastric lipase has greater activity on the fine emulsion. Free fatty acid concentration plays a key role in the progressive inhibition of lipolysis |
198 |
O = LCT & MCT |
Triglycerides type |
Lipase activity |
• SI: pH 7.5, pancreatin, bile (1 h) |
|
E = Lec, Sugar esters |
Hydrolysis of gastric lipase |
|
|
|
O/W Emulsion
|
Droplet size |
Particle size |
• SI: pH 7.5, pancreatin, bile (0.5 h) |
MCT is hydrolyzed faster than LCT. Lipolysis rate is increased with decreasing particle size. Calcium is a key factor during digestion |
125 |
O = LCT & MCT |
Triacylglycerol composition |
Pancreatic lipase activity |
|
|
E = Lec, Sugar esters |
Calcium |
|
|
|
|
pH |
|
|
|
Recently, a number of researchers have developed analytical methods to better understand the interactions of lipid droplets with the tongue and oral cavity.109–111 A number of excellent reviews of the various in vitro/ex vivo/in vivo analytical methods developed have been published.96,97,112 These include methods such as imaging (X-ray, sonography, NMR, and endoscopy), microscopy (optical and confocal fluorescence microscopy), and rheology (shear rheology, large strain deformation, and tribology). These techniques can either be used in isolation or in combination with each other. A particularly innovative approach has been the use of combined tribology and microscopy methods to examine the flocculation, coalescence, and spreading of lipid droplets under simulated oral conditions by using a pig's tongue as one of the interacting surfaces.96,111
The test sample that results from mixing the food sample with the simulated saliva fluid can be referred to as the “bolus sample”.
5.2.2. Stomach.
The major factors to take into account when designing an in vitro digestion step to simulate the behavior of lipid droplets within the stomach are the high acidity, specific enzyme activity, mineral composition, and mechanical/flow profile (Section 2.1.3). Previous researchers have used simulated gastric fluids (SGF) with different compositional complexities in their in vitro digestion models (Table 1). The simplest digestion models involve adjusting the sample to a highly acidic pH (e.g., pH 1.2) for a fixed period (e.g., 2 h) with some form of mechanical agitation (e.g., stirring). More sophisticated digestion models include a range of different components in the SGF, including acids, buffers, salts, organic molecules, biopolymers, phospholipids, and digestive enzymes.44,57,108 In particular, it is important to note that the stomach contains gastric lipase, which may promote some initial lipid digestion within the stomach. Recipes for preparing simulated gastric juices of varying complexity have been published.2,108,113 A mechanical gut model has been developed to simulate the complex flow profiles, dynamic secretions, and mechanical forces that occur in the human stomach by researchers at the Institute of Food Research (Norwich, UK).114 Most researchers ignore droplet interactions with the surfaces of the stomach in their in vitro digestion models due to the inherent complexity of simulating the stomach's surface in the laboratory. Nevertheless, these interactions may be important in applications where the lipid droplets are designed to adhere to the stomach wall lining.
The test sample that results from mixing the food sample with the simulated gastric fluid can be referred to as the “chyme sample”.
5.2.3. Small intestine.
The major factors that need to be considered when designing an in vitro digestion step to simulate the behavior of lipid droplets within the small intestine are pH changes (from acid to neutral), enzyme activities (particularly lipase), biological surfactants (particularly bile and phospholipids), and mineral content (particularly calcium). Simulated small intestinal fluids (SSIF) of varying compositional complexity have previously been used within in vitro digestion models (Table 1). The simplest SSIF usually contain a mixture of lipase (or pancreatin) and bile salts (or bile extract) at a pH around neutral. More sophisticated models utilize SSIF that contain buffers, salts, small organic molecules, proteins, enzymes, co-enzymes, bile salts and phospholipids.2,44,108 SSIF composition has been shown to play a major role in determining the rate and extent of lipid digestion determined using in vitro models (see below). It is therefore important to establish an appropriate SSIF composition for a particular sample being tested, e.g., the type and concentration of the components within the SSIF that accurately reflect what happens in vivo. As in the stomach, most researchers ignore the interactions of the lipid droplets with the surfaces of the small intestine in their in vitro digestion models. Nevertheless, a number of researchers have recently examined the impact of the mucous layer on the behavior of lipid droplets and other particles under simulated small intestinal conditions.115 The lipid droplets may become trapped within the mucous layer depending on their size and charge, which is likely to impact how quickly they are digested and absorbed.
The test sample that results from mixing the food sample with the simulated small intestinal fluid can be referred to as the “digest sample”.
5.2.4. Colon.
The colon is one of the most difficult regions to simulate in the laboratory. In vitro testing methods designed to simulate the nutritional and environmental conditions in the human large intestine range from simple static batch microbial cultures to multiple stage continuous cultures.116–118 A food sample is typically incubated in one or more simulated colonic fluids (SCF) that contain populations of bacteria representative of those normally found in the human large intestine. These bacteria may be cultivated from animal caecal contents or human feces. One difficulty in accurately simulating the human colon is the considerable variations in bacterial populations that exist between individuals. Rather than using bacteria, it is possible to formulate SCF that contain a mixture of enzymes typically produced by colonic bacteria e.g., glycosidases to degrade dietary fibers and proteases to degrade proteins.80,119 Due to the difficulties in setting up and maintaining in vitro colonic models many researchers prefer to go directly to animal models.117 Alternatively, if there is strong evidence that the sample is fully digested and absorbed within the small intestine, then this step can be ignored.
5.3.
In vitro versus in vivo correlations
In vitro studies offer several advantages over in vivo studies, because they are usually faster, less expensive, more versatile, and provide more details about physicochemical mechanisms.2,105,120 Nevertheless, it is extremely difficult to accurately mimic the complex physicochemical and physiological processes that occur in the human digestive tract. For this reason, it is usually advisable to combine in vitro studies with in vivo studies using animals and humans (where possible). In addition, it is important to establish in vitro–in vivo correlations to ensure that any in vitro method being used to test a particular sample is reliable.2,121,122 In this case, the rate and/or extent of lipid digestion may be measured for similar test samples using an in vitro method (e.g., pH stat) and an in vivo method (e.g., human feeding study), and then the results correlated to one another. Eventually, one would like to obtain mathematical models that can predict the real-life performance of a sample from results obtained using an in vitro test model.
5.4. Physicochemical parameters measured in digestion studies
A variety of analytical techniques can be used to characterize the changes in the properties of emulsified lipids as they pass through simulated GI conditions. A number of the most important and commonly used are highlighted in this section:
5.4.1. Enzyme activity: Formation of digestion products.
A variety of digestive enzymes are active in different locations within the human GI tract, including lipases, phospholipases, proteases, amylases, and glycosidases.51,63,80 An accurate in vitro digestion model should therefore contain appropriate types and levels of digestive enzymes, which will depend on the nature of the sample being tested.
Lipases.
One of the most important parameters to measure in an in vitro digestion model is the rate and extent of lipid digestion due to the activity of gastric and/or pancreatic lipase, i.e., conversion of triacylglycerols (TAG) and diacylglycerols (DAG) into monoacylglycerols (MAG) and free fatty acids (FFA). The most widely used method of measuring lipid digestion is to determine the amount of free fatty acids produced by titration with an alkali solution.120 The automated “pH-stat method” based on this principle is discussed in a latter section (Section 6). Nevertheless, other methods can also be used. For example, the amounts of triacylglycerols, diacylglycerols monoacylglycerols, free fatty acids and phospholipids present in the system can be determined at specific digestion times using chromatography methods, such as thin layer chromatography or TLC.123,124 The concentration of specific fatty acids in the lipid and/or aqueous phases can be measured over time using gas chromatography.123 This information can be used to determine which chain lengths, degrees of saturation, and sn-positions of fatty acids on the triacylglycerols molecules are most susceptible to digestion. The human GI tract also contains phospholipases capable of removing free fatty acids from phospholipids and esterases capable of cleaving some non-ionic surfactants, and so it may be important to include these enzymes when testing systems containing these components.125
Proteases.
If a lipid droplet is initially coated with a protein-based emulsifier or if it is initially embedded within a protein-based matrix, then it may be important to monitor the rate and extent of protein digestion, since this may indirectly influence lipid digestion.93,126 For example, the lipase may be unable to access the lipid droplet surfaces until the protein has been removed by hydrolysis into amino acids. Hence, the lipid digestion rate could depend on the protein digestion rate. Protein digestion can also be measured using the pH-stat method,127 although most previous studies have utilized chromatography (particularly HPLC) or electrophoresis (particularly SDS-PAGE) to monitor the conversion of proteins into peptides and amino acids.93,113,128 The concentration and type of specific proteins present within a sample can be determined by combining electrophoresis and mass spectrometry methods.126
Glycosidases and amylases.
If a lipid droplet is trapped within a dietary fiber or starch matrix, then it may be important to monitor the digestion of these components by glycosidases or amylases, respectively.80,118 The rate and extent of polysaccharide digestibility is typically monitored by determining the amount of monosaccharides released over time using chemical, enzymatic, chromatography, electrophoresis or spectroscopic methods. As with proteins, the lipid digestion rate may depend on the polysaccharide digestion rate if the lipid droplets are encapsulated within impenetrable polysaccharide matrices.
5.4.2. Particle size distribution and microstructure.
The specific surface area of an emulsion is inversely related to the size of the droplets that it contains.129 Lipid digestion is an interfacial phenomenon that requires the lipase molecules to adsorb to oil droplet surfaces before hydrolysis can occur.15,63 Consequently, the rate of lipid digestion often depends on the size of the oil droplets in an emulsion.125,130 In addition, the size of the droplets may change as they pass through the different regions of the GI tract due to fragmentation, coalescence, flocculation, or digestion processes. Consequently, it is often important to have analytical tools to measure the particle size distribution of emulsions as they pass through simulated GI conditions. Optical microscopy techniques are suitable for studying emulsions that contain lipid droplets greater than about 1 μm in radius.98,99 Specific stains or dyes can be used to highlight particular components within an emulsion and determine its location, e.g., oil soluble dyes,113,131,132 protein stains,133 or polysaccharide stains.100 Thus, one can determine whether a component is present at the oil–water interface or dispersed within the surrounding aqueous phase, and whether its location changes during the digestion process. Electron microscopy (SEM or TEM) can also be used to measure the structural features and organization of the lipid droplets and other colloidal particles present in the digestive fluids.
A number of instrumental methods are also available for measuring the particle size distribution (PSD) of emulsions, including static light scattering (SLS), dynamic light scattering (DLS), particle counting, and ultrasonic spectrometry.27,134 Each of these methods has a range of particle diameters that it can reliably detect. For example, SLS instruments can typically detect particles from about 0.1 to 1000 μm, whereas DLS instruments can detect particles in the range 1 nm to 5 μm. Instrumental particle size analyzers are able to provide rapid analysis of the full PSD of emulsion samples in a few minutes, and are therefore extremely convenient for monitoring changes in particle dimensions during the lipid digestion process. They can be used to monitor the breakup, coalescence, flocculation, or degradation of lipid droplets, or to determine the dimensions of other colloidal species present within the digestion fluids such as micelles or vesicles. Nevertheless, these instrumental methods often have a number of limitations when applied to studying lipid digestion. There are often many components within the sample itself or from the simulated GI fluids that can scatter light and therefore contribute to the measured particle size distribution. For example, there may be insoluble matter in the bile extract or pancreatin used to prepare SSIF that obscure the light scattering signal from the lipid droplets. It may therefore be advisable to filter these SSIF solutions prior to utilization in the in vitro digestion model.
5.4.3. Interfacial composition and properties.
The composition and properties of the interfacial layer coating the lipid droplets usually changes as an emulsion passes through the GI tract. Consequently, it is useful to have analytical tools that can measure interfacial compositions or at least detect alterations in interfacial compositions. A number of direct and indirect methods have been used to obtain information about interfacial composition. Fluorescent probes can be used to tag surface-active species (such as proteins, phospholipids or polysaccharides) to determine changes in their location during the digestion process. The fluorescently-tagged molecules can either be observed directly under a fluorescent microscope or they can be analyzed by fluorescent spectroscopy. Front face fluorescence reflectance spectroscopy measurements can be carried out on emulsion samples without any sample preparation,135,136 whereas fluorescent transmission measurements can be carried out on transparent aqueous solutions after a suitable isolation step e.g., centrifugation, dialysis and/or filtration. Measurements of droplet charge (ζ-potential) can be used to provide information about changes in interfacial composition, e.g., due to the displacement of one surface-active substance by another.129,131 Knowledge of the droplet ζ-potential may also be important in its own right, since the charge on the droplets will determine how they interact with other components and surfaces within the GI tract. Cationic particles may become trapped within the anionic mucous layer (mucoadhesion), which will alter their ability to be digested and absorbed.137,138 Interfacial tension and rheology measurements are commonly used to detect changes in interfacial composition at planar air–water or oil–water interfaces, providing information that can be related to the behavior of lipid droplet surfaces under similar conditions.94,139 Chemical analysis methods can be used to measure changes in the aqueous phase composition during digestion, which can be used to infer changes in interfacial composition if the total amount of the component in the system is known: CAds = CAq − CTotal. A number of different microscopy methods have been used to examine the structural organization of adsorbed components at the oil–water interface, such as atomic force microscopy and confocal fluorescent microscopy.93,94
5.4.4. Formation of colloidal structures.
A number of analytical tools have been used to characterize the various kinds of colloidal structures generated during the lipid digestion process, such as liquid crystals, mixed micelles and vesicles. These methods include light scattering,140,141 X-ray scattering,142,143 neutron scattering,144 electron microscopy,145,146 electron spin resonance and NMR.147 In addition, some techniques can provide information about the distribution of lipid digestion products between the oil, water and colloidal phases, e.g., electron paramagnetic resonance.148 The solubilization of digestion products and lipophilic components within the colloidal structures can usually be determined using standard analytical methods, e.g., chemical, spectroscopic, chromatographic or electrophoresis methods.
5.4.5. Binding interactions and aggregation.
A number of different kinds of binding interactions may occur in the GI tract that could alter lipid digestibility: biopolymers may bind mineral ions, enzymes, lipid droplets, phospholipids, and surfactants; chelating agents may bind calcium; fatty acids and bile salts may bind calcium (Section 2.1.5). For example, cationic dietary fibers such as chitosan are known to strongly bind anionic bile salts, fatty acids, phospholipids, and lipid droplets, which may interfere with the normal digestion process.100 The method used to study the binding interactions will depend on the nature of the species involved. Some commonly used analytical methods include equilibrium dialysis, isothermal titration calorimetry, centrifugation, specific chemical reactions, chromatography, electrophoresis, and spectroscopy.
5.4.6. Flow profiles and rheology.
The rheological characteristics of the digestive fluids in the GI tract may play an important role in determining the rate and extent of lipid digestion.61,113 For instance, their rheology may influence the flow profile and mechanical forces generated within the GI tract, or the mass transport of components through them. The rheology of digestive juices may range from low viscosity liquids, to visco-elastic fluids, to gel-like materials depending on their composition and environmental conditions. Consequently, it is often important to be able to characterize the rheological characteristics of the system. The rheology may be measured using a number of different analytical instruments, such as viscometers, dynamic shear rheometers, uniaxial compression methods, and flow profiling techniques.27,149–151 The flow profiles in a reaction chamber can be monitored by imaging methods, such as those based on X-ray, NMR or acoustics. Alternatively, they can be simulated using appropriate mathematical models that take into account the geometry of the vessel and the nature of the applied mechanical forces.152
5.4.7. Physical state.
A number of studies show that the physical state (solid versus liquid) of lipid droplets influences the rate and extent of their digestion.153–155 Consequently, it may be useful to determine the physical state of lipid droplets during passage through a simulated GI tract if a high melting point fat phase is used. The physical state of lipid droplets can be determined using a number of analytical techniques, including DSC and NMR.27 Information about the packing of the crystals within the lipid droplets can be obtained by X-ray diffraction studies.156,157
5.4.8. Release of lipophilic components.
Researchers are often interested in monitoring the release of bioactive lipophilic components encapsulated within lipid droplets during the digestion process. One of the most commonly used methods is to centrifuge the digested sample (Fig. 4a), which typically separates into three different layers (Fig. 4b): a pellet at the bottom, an aqueous phase in the middle, and an oil phase at the top.158 The pellet typically contains dense insoluble matter, such as aggregates containing undigested proteins, dietary fibers, bile salts, free fatty acids, and minerals. For example, insoluble calcium soaps of free fatty acids are usually present in this layer. The aqueous phase typically contains mixed micelles and vesicles that contain bile salts, phospholipids, and lipid digestion products (MAG and FFA). In addition, any lipophilic agents that were originally encapsulated in the lipid droplets may be solubilized within the hydrophobic interiors of these micelles and vesicles, e.g., carotenoids. The cream layer contains any undigested lipid in the form of oil droplets or bulk oil. After centrifugation the different layers present can be collected separately and then analyzed to determine their composition, e.g., using chemical, spectroscopic, electrophoresis, or chromatographic methods. This approach can be used to determine the amount of lipophilic components released from the lipid droplets after digestion, which is important when determining the bioavailability of encapsulated substances.120,159
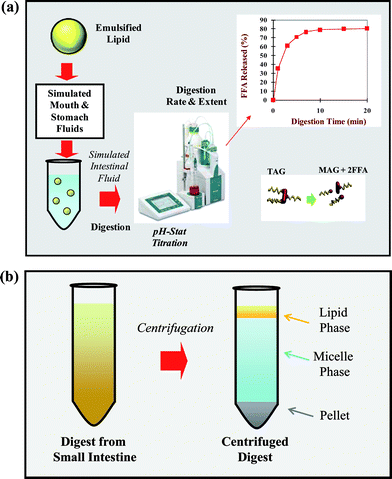 |
| Fig. 4
(a) Schematic of an in vitro digestion model used to determine the digestion and release of lipids encapsulated within nano-laminated lipid droplets. The picture of the pH-stat titrator is kindly donated by Metrohm® USA, Inc. A triacylglycerol (TAG) is converted into two free fatty acids (FFA) and one monoacylglycerol (MAG) by lipase. (b) Schematic diagram of the three phases typically formed after centrifugation of the material remaining after digestion in simulated small intestine conditions. | |
5.4.9. Absorption of lipophilic components.
The absorption of lipophilic components (digestion products or encapsulated agents) by epithelium cells can be simulated in vitro using various physical, biological, or cell-culture models:
Physical methods.
For some types of lipid it is assumed that the amount of digestion products released from the sample is representative of the amount of digestion products absorbed by the epithelium cells, i.e., the rate limiting step is lipid digestion, rather than absorption. The amount of lipid digestion products released can be determined by measuring their concentration in the aqueous phase, which can be measured directly or after they have been separated from the rest of the material by centrifugation, filtration, or dialysis.160,161 For example, the free fatty acids released from emulsified lipid droplets through the action of pancreatic lipase can simply be measured by acid titration or enzymatic techniques.86,162,163 The concentration of specific bioactive lipids solubilized by mixed micelles/vesicles can be determined using a suitable analytical method, usually after the middle (micelle) phase has been separated from the cream layer and pellet by centrifugation.164–166
Ex vivo permeation methods.
A section of the GI tract is cut from an animal after it has been sacrificed, and is then washed to remove any residual components. Part of the intestine is then clamped between two chambers: one of the chambers contains the sample to be analyzed (donor chamber), while the other chamber contains only buffer solution (receiver chamber).167 The transport of the samples across the chamber is then measured over time using suitable analytical methods. Alternatively, the sample to be tested can be placed inside an intact section of GI, which is then placed in an appropriate buffered solution. The amount of material that moves across the intestinal walls and into the surrounding buffer solution can then measured using an appropriate analytical method.
Cell culture methods.
Caco-2 cells are cell cultures that mimic the human intestinal epithelium.168–170 They can be used in two different ways to assess the absorption of lipid digestion products: (i) after the digested food material has been left in contact with a membrane coated with Caco-2 cells, the amount of lipid digestion products that pass through the coated membrane can be measured; (ii) the amount of lipid digestion products that are absorbed by the cells themselves can be determined. A suitable analytical technique can then be used to measure the location or quantify the amount of material absorbed, e.g., microscopy, chromatography, spectrometry or chemical methods.
6. pH-stat method
6.1. Principle of the pH-stat method
The pH-stat method is an analytical tool widely used in pharmaceutical and food research for the in vitro characterization of lipid digestion under simulated small intestinal conditions.120,122,125,171 It is based on measurements of the amount of free fatty acids released from lipids, usually triacylglcyerols, after lipase addition at pH values close to neutral. The sample to be analyzed is placed within a temperature-controlled reaction chamber that contains simulated small intestinal fluid (SSIF) (Fig. 4a). The SSIF should contain appropriate levels of the major digestive components known to influence lipid digestion, such as lipase, co-lipase, bile salts, phospholipids, and mineral ions (Table 2). It is usually assumed that the lipase in the SSIF catalyzes lipid digestion leading to the generation of two FFAs and one MAG per TAG molecule, although further digestion can occur in some situations. The concentration of alkali (NaOH) that must then be added to the digestion cell to neutralize the FFAs produced by lipid digestion, and thereby maintain the pH at the initial pre-set value (e.g., pH 7.0), is recorded versus time (Fig. 4a). The pH-stat method is relatively simple and rapid to carry out and enables comparison of different systems under similar experimental conditions. This technique can therefore be used to rapidly screen the impact of different physicochemical factors expected to affect lipid digestion.
Table 2 Summary of the simulated small intestinal fluid compositions used in previous pH-stat studies of the digestion of emulsified lipids. All experiments were carried out at 37 °C unless stated
Samples Tested |
Experimental Variables |
Lipid |
Lipase |
Bile |
Calcium |
Digestion times |
pH |
References |
O/W Emulsions
|
[CaCl2] |
0.4% |
1.6 mg/mL |
0–5 mg/mL |
0–20 mM |
20 min |
7.0 |
84 |
O = corn oil |
[EDTA] |
E = BLG, lecithin, or caseinate |
[Bile] |
|
Emulsifier type |
SEDDS
|
Ions |
0.5% |
2.5 mg/mL |
5 mM (1.25 mM lecithin) |
5–20 mM |
20 min |
6.5 |
175 |
|
pH |
|
Surfactant/Glycerides |
Oil suspension
|
Triglycerides |
50 mg/mL |
1000 IU/mL |
5 mM |
5 mM |
2–24 h |
6.8 |
167 |
O/W Emulsions
|
Emulsifier type |
0.4% |
2.9 mg/mL |
4.2 mg/mL |
|
5 min |
6.5–7.0 |
192 |
2 h |
2 h |
O/W Emulsions
|
Bile |
30.6 mM |
270–1340 units/mL |
4–16 mM |
4–20 mM |
40 min |
6.5 |
83 |
|
Ca2+ |
|
Lipase activity |
O/W Emulsions
|
Interfacial composition |
0.2% |
2 nM (10 nM colipase) |
9.7 mM |
10 mM |
0–2 h |
7.0 |
223 |
O/W Emulsions
|
Influence of dietary fiber |
0.2% |
0.4 mg/mL |
2.4 mg/mL |
|
2 h |
7.5 |
195,210 |
O/W Emulsions
|
Pancreatic lipase activity |
0.2% |
1 mg/mL (Lipase:colipase = 1 : 5) |
0–8 mM (0.74 mM phospholipid) |
0–30 mM |
0–3 h |
7.5 |
211 |
Oil suspension
|
pH |
0.039 mg/mL |
0–4.8 mg/mL |
0–20 mg/mL |
|
2 h |
6.5 |
166 |
|
Bile |
|
Pancreatic lipase |
Oil
|
Lipid type |
25 mg/mL |
1000 TBU/mL |
5/20 mM |
5 mM |
30 min |
7.5 |
124,212 |
SNEDDS
|
Lipolysis time |
0.3% |
800 TBU/mL |
5 mM (1 mM phosphatidylcholine) |
0.045 mM/min |
30 min |
6.5 |
141 |
O/W Emulsions
|
Lipase |
10% |
0–10 mg/mL |
0–25 mg/mL |
30 mM |
2 h |
7.5 |
224 |
|
Bile |
|
Lipolysis time |
O/W Emulsions
|
Lipid composition |
10.5 mg/mL |
200 tributyrin units/mL |
5 mM (1.25 mM phosphatidylcholine) |
5 mM |
35 min |
7.5 |
225 |
Recently, a simple mathematical model was developed to describe the FFA versus time profiles obtained by the pH stat method.172 The percentage of total free fatty acids released (Φ) as a function of time (t) measured by the pH-stat method is characterized by the following equation:
| 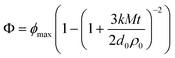 | (1) |
Here,
ϕmax provides a measure of the total
extent of
digestion (
i.e., the maximum percentage of the total FFA present that is released at the end of the reaction),
k provides a measure of the
rate of
digestion (
i.e., mmols of FFA released per unit droplet surface area per unit time),
d0 is the initial droplet diameter,
ρ0 is the oil droplet density, and
M is the molar mass of the oil. A pH-stat profile can then be characterized in terms of just two parameters:
ϕmax and
k, which can be determined by finding the values which give the best fit between the
experimental data and the mathematical model.
It should be noted that the pH stat method only simulates the small intestine, and it is usually necessary to simulate the other parts of the digestive tract to get more realistic results. For example, a sample could be passed through a simulated mouth and stomach model, and then analyzed using the pH stat method.
6.2. Influence of SSIF composition on the pH-stat method
One of the most important factors affecting the digestion rate determined using the pH-stat method is the composition of the simulated small intestinal fluid (SSIF) used.2 Previous researchers have used various SSIF compositions when carrying out in vitro lipid digestion studies using the pH stat method (Table 2). In addition, a number of workers have examined the influence of specific SSIF components on the rate and extent of lipid digestion. Ideally, the conditions used should closely simulate those found in the human GI tract (Section 2), and the digestion results obtained should correlate closely with those obtained using in vivo studies. The role of the major components within SSIF is highlighted below:
6.2.1. Lipase and other enzymes.
Pancreatic lipase is the key component in any in vitro model designed to simulate lipid digestion within the small intestine. Consequently, it is important to use an appropriate type and concentration of pancreatic lipase in the pH-stat method. Previous researchers have used various types and forms of lipase in SSIF, including pancreatin, pancreatic lipases, and non-pancreatic lipases. Pancreatin is a complex mixture of digestive enzymes (lipase, protease, amylase, etc.) and other biological components produced by the exocrine cells of the pancreas, which is typically obtained from animal sources, such as pigs or cows. The chemical composition and functional performance of pancreatin (and consequently its enzyme activity) varies depending on its biological origin, isolation, and purification procedures, and hence there are often considerable batch-to-batch and supplier-to-supplier variations.173 Purified pancreatic lipases are also available commercially that have been isolated from various animal and human sources, although these are more expensive that using pancreatin. In addition, it is important to use an appropriate amount of co-lipase with pancreatic lipase to ensure its optimum performance. The advantage of using pancreatic lipases is that they are chemically more well-defined, and there is less batch-to-batch variation. Some researchers have used non-pancreatic lipase sources for their in vitro lipid digestion studies, e.g., Candida rugosa, Candida cylindracea, Rhizopus niveus, and Mucor meihei.174 These lipases have the advantage of been highly pure and reproducible, and less expensive than pancreatic lipase. However, they may not accurately mimic the behavior of pancreatic lipase in the lipid digestion process.
The catalytic activity of lipase depends on its origin and history. For example, the catalytic activity of an enzyme ingredient may decrease if it is exposed to excessively high temperatures or if it is stored too long. Hence, researchers should be careful not to subject lipase ingredients to temperature abuse, and should prepare fresh lipase preparations for each experiment. The catalytic activity of lipase also depends on solution and environmental conditions, such as temperature, pH, ionic strength, and the presence of chemical denaturants.71,175–177 These conditions should therefore be standardized in any in vitro digestion test. It is therefore recommended that each batch of lipase should be standardized prior to utilization.120 A commonly used method for determining lipase activity is to measure the amount of free fatty acids released from a fixed amount of a standardized emulsified lipid (e.g., triolein or tributyrin) after a specific digestion time under standardized conditions (pH, ionic strength, temperature).120,167 Nevertheless, the rate of lipid digestion has previously been shown to depend on emulsifier type and droplet size distribution,130,131 and so these parameters should also be standardized. At present there is no consensus on the best values to use for these parameters. We propose using lyso-lecithin as a standard emulsifier since it can form stable emulsions and is readily available from chemical suppliers, and using lipid droplets with a standard mean diameter (d32) around 2 μm since these can be produced using simple high shear mixers and are fairly stable over the experimental time scales involved.
The concentration of pancreatic lipase in the human small intestine depends on many factors, including the individual, health status, age, time of day, and type and amount of food consumed.15,63 It is therefore difficult to recommend one definitive lipase level to use in an in vitro test method. Ideally, one should examine a range of lipase concentrations that encompasses those typically found in the human GI tract. However, it is usually assumed that humans have a great excess of lipase in the GI tract, and so it is advisable to use a relatively high level of lipase in in vitro studies, e.g., equivalent to 2.4 mg/mL pancreatin.
It should also be noted that there may be various other enzymes present in the small intestine that may directly or indirectly alter the rate of lipid digestion. If lipid droplets are coated by digestible emulsifiers (such as proteins, phospholipids, and some surfactants) or if they are embedded within gel-like or solid particles (such as proteins, or polysaccharides), then the digestion of these components may be important. For example, if lipid droplets are coated by a layer of adsorbed protein molecules, then this layer may have to be digested by proteases before the lipase can reach the triacylglycerols.93 Similarly, if lipid droplets are embedded within a protein hydrogel particle, then it may be necessary for the protein matrix to be fully or partially digested by proteases before the lipase can reach the triacylglycerols.13 Some of these other digestive enzymes are naturally present in pancreatin, e.g., proteases and amylases. On the other hand, it may be necessary to add some or all of these enzymes if pure pancreatic lipase or non-pancreatic lipase is used. For samples containing lipids encapsulated within other digestible components it would be informative to run experiments with and without non-lipase digestive enzymes to determine their influence on the overall lipid digestion rate.
An example of the influence of lipase concentration on the rate and extent of lipid digestion of β-lactoglobulin stabilized corn oil-in-water emulsions measured using the pH stat method is shown in Fig. 5a. The rate and extent of lipid digestion increased as the lipase concentration in the reaction vessel increased. At low lipase levels (≤0.2 mg/mL), FFAs were released slowly and most of the lipids within the droplets remained undigested after 30 min (<35% FFA released). At intermediate lipase levels (e.g., 0.4 and 0.8 mg/mL), there was an initial period from 0 to 10 min when FFAs were released slowly, followed by another period when the rate of FFA release increased appreciably. At high lipase levels (≥2.4 mg/mL), the amount of FFA released increased rapidly with time almost immediately after digestion started, and then leveled off at longer times because all the lipids within the droplets had been fully digested. One would expect the rate of lipid digestion to increase as the lipase concentration increased because there would be more total enzyme present in the system to catalyze the conversion of triacylglycerols to free fatty acids.71 In addition, the amount of lipase present at the oil–water interface, where the lipolysis reaction occurs, will increase as the lipase concentration increases.71 Lipase is a surface-active protein that can compete for the oil–water interface with other surface-active components,139 such as the β-lactoglobulin initially coating the lipid droplets or the bile salts added to the reaction vessel. At low lipase concentrations, there may be insufficient lipase present to displace β-lactoglobulin and/or bile from the oil–water interface, and so the enzyme cannot come into close contact with the lipid substrate within the droplets. The observation of an initial slow rate of FFA release at intermediate lipase concentrations may be explained by the finite time required for lipase to adsorb to the lipid droplet surfaces and displace the β-Lg and/or bile coating so as to get access to the triacylglyercols within the droplet core.178 Presumably at higher lipase concentrations the adsorption and displacement processes occur rapidly so that digestion could begin almost immediately after the digestive enzyme was added to the reaction vessel.
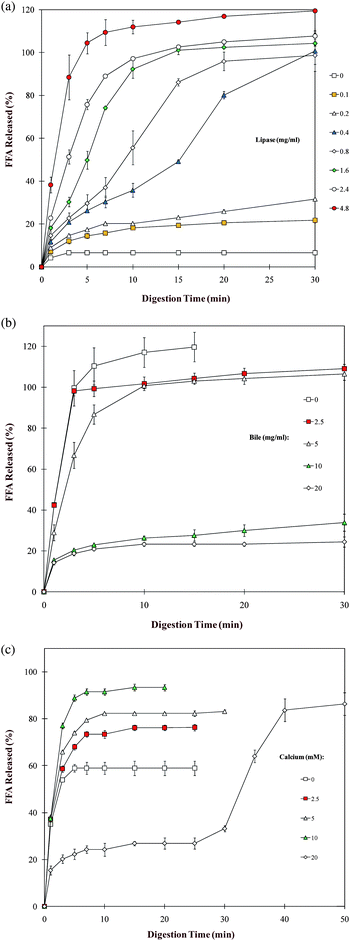 |
| Fig. 5
(a) Influence of lipase concentration in the pH-stat reaction vessel on the rate and extent of lipid digestion determined by monitoring the free fatty acids (FFA) released over time (adapted from Li et al. 2010). (b) Influence of bile salt concentration in the pH-stat reaction vessel on the rate and extent of lipid digestion determined by monitoring the free fatty acids (FFA) released over time (adapted from Li et al. 2010). (c) Influence of calcium concentration in the pH-stat reaction vessel on the rate and extent of lipid digestion determined by monitoring the free fatty acids (FFA) released over time (adapted from Li et al. 2010). | |
6.2.2. Bile.
Bile is another key component in any SSIF used to simulate lipid digestion in the small intestine.2 Typically, researchers use either bile extract or one or more individual bile acids in their simulated small intestinal fluids. Bile extract is a complex mixture of various kinds of molecules typically found in the GI tract (such as bile acids, phospholipids, and minerals), and may therefore more accurately reflect in vivo conditions than individual bile acids, however it tends to be more variable and inconsistent in composition and performance. In addition, bile extract often contains insoluble matter, which can interfere with the analysis of lipid droplets and other colloidal structures in digestion media, and therefore it should be filtered before use.
Individual bile acids can be purchased in pure form, which often facilitates the design and interpretation of experimental digestion measurements. On the other hand, using purified bile acids may be less representative of the complex composition of actual small intestinal fluids. For this reason, researchers often utilize a mixture of bile acids and other biological components (such as phospholipids) to reflect the bile composition in the human GI tract. Typically, bile contains four major types of bile acids (cholic, deoxycholic, chenodeoxycholic, and lithocholic acids), as well as their glycine and taurine derivatives.51 Some researchers have tried to simplify the complexity of bile composition by only using the major bile acid components. For example, in a recent study a mixture of sodium taurocholate (NaTC, 52.7%) and sodium glycodeoxycholate (NaGDC, 47.3%) was used to mimic human bile.95,179
An example of the influence of bile concentration on lipid digestion in β-lactoglobulin stabilized corn oil-in-water emulsions measured using the pH stat method is shown in Fig. 5b.180 The rate and extent of FFA release decreased as the concentration of bile extract in the reaction vessel increased from 0 to 20 mg/mL. The suppression of lipid digestion by bile salts is well established in the literature, where it has been attributed to the ability of surface-active bile salts to displace lipase from the oil–water interface thereby preventing it from coming into close contact with the lipid substrate.63,71,74,181 Addition of co-lipase has been shown to reverse this effect and increase lipase activity.63 The data shown in Fig. 5b was obtained using an isolated porcine lipase, rather than a crude pancreatin extract containing both lipase and co-lipase, which accounts for the observed decrease in lipase activity with increasing bile salt concentration. In the same study, the ability of bile salts to displace proteins from droplet surfaces was demonstrated using ζ-potential measurements.
6.2.3. Calcium and other minerals.
A number of in vitro digestion studies have highlighted the important role that calcium plays in determining the rate and extent of lipid digestion using the pH-stat method.83,84,182 Calcium ions may impact the lipid digestion process due to a number of different physicochemical mechanisms.
• Enzyme activity: Calcium is believed to be a necessary co-factor for the proper functioning of pancreatic lipase62,183–185
• Removal of FFA from droplet surfaces: Lipase digestion of emulsified lipids can be inhibited by accumulation of long-chain fatty acids (LCFA) at the droplet surfaces, since this restricts the access of the lipase to the triacylglycerols.15 Calcium is known to precipitate these LCFA, thereby removing them from the lipid droplet surface and allowing the lipase to access the emulsified lipids.98,99 Consequently, calcium ions are able to increase the rate and extent of lipolysis by this mechanism.83,125,182,186
• Reduced digestibility of flocculated droplets: Calcium ions are highly effective at causing droplet flocculation in emulsions containing lipid droplets coated with anionic emulsifiers. It is more difficult for the lipase molecules to reach the surfaces of the lipid droplets trapped in the center of a floc, which may slow down the lipid digestion rate in flocculated emulsions.84
• Reduced digestibility due to gel formation: Calcium ions may promote the gelation of certain types of biopolymers (such as alginate and pectin), which can lead to the formation of hydrogel matrices that trap lipid droplets and inhibit the diffusion of lipase to the droplet surfaces.
• Reduced bioavailability of precipitated FFA: Studies have shown that insoluble soaps are formed between calcium ions and LCFA, which can reduce the bioavailability of these fatty acid digestion products.70,187,188
It is therefore critical to use an appropriate level of calcium in the SSIF used in pH-stat studies. A certain level of calcium is naturally present in human digestive juices and additional amounts may also arise from ingested foods, particularly those having have levels of this mineral.83 Typically, the calcium levels in the human small intestine have been reported to be in the range 5 to 30 mM as reflected in the levels used in most pH-stat digestion models (Table 2).
The importance of calcium levels on lipid digestion in corn oil-in-water emulsions stabilized by β-lactoglobulin was recently studied in our laboratory.180 The lipid digestion process measured by the pH-stat clearly depended on the level of calcium ions present in the SSIF. The rate and extent of FFA production increased when the calcium level increased from 0 to 10 mM, but decreased when 20 mM calcium was added (Fig. 5c). Two different digestion regimes could be distinguished at 20 mM calcium: Regime I - an initial period from 0 to 30 min when digestion was relatively slow; Regime II – a later period when the digestion rate increased appreciably. The increase in digestion rate with increasing calcium in Regime I can be attributed to the ability of Ca2+ ions to precipitate LCFA digestion products that tend to accumulate at the oil–water interface and inhibit lipase activity.83,125 The dramatic suppression of FFA production observed in Regime II suggests that the ability of lipase to access the lipid droplet surfaces was restricted, presumably because the high calcium levels promoted extensive droplet flocculation.
The concentration of free calcium ions in the small intestine will depend on the presence of any other components that can bind calcium. These components may be naturally present within the human body such as mucins or specific proteins, or they may be present within ingested foods such as chelating agents (EDTA, phosphates) and biopolymers (proteins, peptides and polysaccharides).85,189–191 Recent studies have shown that food components that bind calcium strongly, such as EDTA and alginate, are able to greatly reduce the lipase activity in oil-in-water emulsions.84 This may be important when designing and interpreting pH-stat measurements of lipid digestion in complex food systems.
Other mineral ions, such as sodium, potassium, sulfates, phosphates and bicarbonates, may also be present in the digestive fluids in a human's small intestine.51 These minerals may play an important role in the digestion process since they can affect the magnitude and range of any electrostatic interactions in the system, which may alter the physicochemical properties, solubility, and aggregation state of various components within the system. It is therefore important to use mineral levels that reflect those found in vivo. Typically, a single monovalent salt (such as NaCl or KCl) is used to create an ionic strength that mimics physiological levels (≈ 150 mM),120 although some studies have used more complex mixtures.192
6.2.4. pH.
The pH of the small intestine depends on a number of factors and typically varies from one location to another.193 In the stomach, the droplets are surrounded by a highly acidic environment (pH 1 to 3), but when they enter the duodenum the pH is increased to around neutral (pH 5.8–6.5) due to secretion of sodium bicarbonate.63 Nevertheless, studies with human subjects have shown that there may be large variations in duodenum pH depending on the individual involved, and the type and amount of food consumed.44 In particular, the pH of the stomach contents may increase appreciably after ingestion of a food, before gradually returning to the fasting pH level. As the food passes along the small intestine the pH tends to increase to around pH 7 to 7.5. The pH used in an in vitro digestion model may influence the results for a number of different reasons:
• Enzyme activity: Pancreatic lipase has an optimum pH where it exhibits its maximum rate of lipid digestion.175 It has been reported that pancreatic lipase has a maximum activity around pH 8.5,63 but that it also has good activity at pH values around neutral.175
• FFA ionization: The ionization of free fatty acids depends on solution pH relative to their pKa value.174,194 In aqueous solution, the “true” pKa of FFAs has been reported to be around 4.7 to 4.9 depending on their chain length, and so they should be predominantly in their ionized anionic form around neutral pH.174 However, in the presence of lipid droplets the “apparent” pKa of free fatty acids may be considerably higher than their “true” values due to partitioning effects. The ionized form of FFA tends to partition between the oil and water phases, but the non-ionized form tends to accumulate predominantly in the lipid phase. Consequently, the tendency for any FFA formed at the droplet surfaces to move into the surrounding aqueous phase will depend on the pH relative to the pKa value. The apparent pKa of free fatty acids has been reported to increase with increasing hydrocarbon chain length and with increasing fat content, with values of pKa = 11.2 being reported for lauric acid in a 50% fat system.174 This effect may therefore be important in systems where long chain triacylglycerols are used as the lipid phase and the lipid content is relatively high.
• Ingredient properties: The electrical charge, interactions, aggregation, and solubility of many other ionic species may also depend on solution pH. For example, the stability of emulsified lipids to droplet aggregation often depends on pH.
Consequently, the rate and extent of lipid digestion will depend on the pH in the reaction vessel, which should be taken into account when comparing measurements on different systems.
6.2.5. Other factors.
Various other factors should also be considered when designing an appropriate simulated small intestinal fluid for pH-stat studies. Lipids are normally consumed by humans in a diet that contains a range of other components, including dietary fibers, proteins, carbohydrates, minerals. Many of these components may interfere with the digestion process, either decreasing or increasing the rate and extent of digestion. For example, dietary fibers may interact with the lipid digestion process through a variety of physicochemical mechanisms depending on their molecular characteristics.195 They may bind to components within the SSIF, such as calcium, bile salts or lipase, thereby altering their activity. They may form protective coatings around lipid droplets, or they may promote droplet flocculation, which alters the ability of the lipase molecules to access the lipids. Some food components (e.g., polyphenols) may be able to bind to digestive enzymes and reduce their activity.196
6.3. Proposed standardized pH-stat method for testing emulsified lipids
It would be useful to have standardized test conditions for in vitro lipid digestion models so that the results from one study can easily be compared to those of another within and between laboratories. Ideally, this model should give a measure of lipid digestibility that correlates well with that found for similar systems passing through the human digestive tract. In addition, it would be useful for the test procedure to be relatively inexpensive and easy to implement so that it can be adopted by many different laboratories. The pH-stat method is relatively straightforward to set up and carry out, only requiring an automatic titration unit capable of controlling pH.120 Based on the results of ours and others work we propose the experimental conditions outlined in Table 3 as a basis for a standardized pH-stat digestion model. This model is certainly too simplistic to accurately reflect the complex physiological and physicochemical processes that occur in the human gastrointestinal tract, but it does contain the major factors expected to impact lipid digestion and it is relatively simple to implement.
Table 3 Proposed standardized pH-stat method for testing emulsified lipids using the in vitro digestion model under fed state conditions
Experimental Parameter |
Proposed Value |
pH |
7 |
Reaction Cell Volume |
37.5 mL |
Temperature |
37 °C |
Stirring speed |
4 s−1 |
[NaOH] in titration unit |
0.1 mM |
Lipid content in reaction cell |
300 mg |
NaCl in reaction cell |
150 mM |
CaCl2 in reaction cell |
10 mM |
Bile Extract in reaction cell |
20 mg/mL |
Pancreatin (100–400 units/mg protein) in reaction cell |
2.4 mg/mL |
Ideally, the rate of FFA release should fall within a reasonably rapid timeframe (e.g., 30 min) so that multiple samples can be conveniently screened. The above conditions should lead to a release rate that falls within this timeframe. Nevertheless, there are often variations in the activity of the lipase within pancreatin from batch-to-batch or during storage, which means that it may be necessary to adjust its concentration so as to obtain a FFA release profile in the appropriate timeframe. The mean particle diameter and emulsifier used in an experiment should always be reported, since the rate of FFA release in the pH Stat method depends on both of these parameters. The effect of particle size may be taken into account by using the mathematical model described above to calculate the FFA release per unit time per unit surface area.
6.4. Application of pH-stat method
In this section, we demonstrate the usefulness of the pH-stat method using some recent data obtained from our laboratory.
6.4.1. Influence of lipid droplet composition.
The composition of the lipid phase present within food and beverage emulsions varies depending on the nature of the product.27 Studies in the pharmaceutical area indicate that lipid type can have a major impact on lipid digestion and absorption.3,121,197 Consequently, it is useful to study the impact of lipid type on the digestion of oil-in-water emulsions used in the food industry. We recently used the pH-stat method to study the influence of lipid type on the rate and extent of lipid digestion (Fig. 6a). Oil-in-water emulsions stabilized by β-Lg were prepared with similar droplet sizes and concentrations using either corn oil (long chain triglyceride, LCT) or medium chain triglyceride (MCT) as the lipid phase.172 The rate and extent of lipid digestion was clearly higher when MCT was used than when LCT was used. This effect can be attributed to the fact that the medium chain FFA digestion products arising from MCT have a higher dispersibility in aqueous media than the long chain FFA digestion products arising from LCT.3,121 The medium chain FFAs are able to migrate rapidly away from the droplet surfaces and into the surrounding aqueous phase, and so they do not inhibit the interfacial lipase reaction. On the other hand, the long chain FFAs tend to accumulate at the oil–water interface and inhibit lipase activity until they are removed by being solubilized in micelles or precipitated by calcium ions. Similar results have been reported by a number of other researchers using the pH stat method.160,167
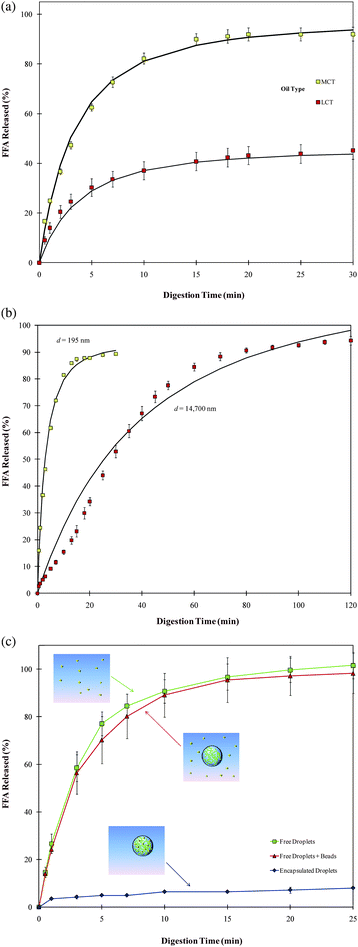 |
| Fig. 6
(a) Influence of lipid type (corn oil versus medium chain triglycerides) on the rate and extent of lipid digestion determined by monitoring the free fatty acids (FFA) released over time using the pH stat method (adapted from Li and McClements 2010). (b) Influence of initial mean droplet diameter on the rate and extent of lipid digestion determined by monitoring the free fatty acids (FFA) released over time using the pH stat method (Li et al. 2010). (c) Influence of initial mean droplet diameter on the rate and extent of lipid digestion determined by monitoring the free fatty acids (FFA) released over time using the pH stat method (Li et al. 2010). | |
6.4.2. Influence of lipid droplet size.
The initial size of the droplets in food and beverage emulsions can be controlled by controlling system composition and homogenization conditions.27 As the droplets pass through the GI tract their size may change due to fragmentation, coalescence, flocculation, or digestion processes (Section 3). The size of the oil droplets within the small intestine should impact their digestion rate since the surface area of lipid exposed to the surrounding aqueous environment is inversely related to the mean droplet diameter.4,27,63,198 Recently, it has been shown that lipid droplet size influences stomach motility and the release of gut hormones, which has important consequences for designing foods to combat obesity.22 It is therefore important to establish the influence of droplet size on the rate and extent of lipid digestion.
We recently used the pH stat method to monitor lipid digestion of MCT oil-in-water emulsions stabilized by β-Lg with different mean droplet diameters: d = 195 nm or 14,700 nm (Fig. 6b).172 The rate of FFA released per unit time (FFA% s−1) was appreciably higher for the emulsion with the smaller droplets, which should be expected because it has a bigger specific surface area for the lipase molecules to bind.27 This result is consistent with earlier in vitro digestion studies that also found the rate of lipid hydrolysis (expressed per unit time) increased with decreasing droplet size.125,198 On the other hand, when the digestion rate was normalized to the droplet surface area it actually increased with increasing droplet size: k = 0.65 and 3.93 μmol s−1 m−2 for the small and large droplets, respectively. The overall concentration of lipase within all of the emulsions was the same and so the increase in k with increasing d32 may have occurred because the amount of lipase available per unit droplet surface area increased as the droplet size decreased. Thus, there may have been more lipase molecules adsorbed per unit surface area in the emulsions containing the large oil droplets than those containing the smaller ones.71
6.4.4. Influence of lipid droplet encapsulation.
Encapsulating lipid droplets within hydrogel particles has been suggested as a means of controlling their digestibility.4,129 In these systems the rate of lipid digestion depends on how quickly the lipase in the aqueous phase can access the surfaces of the encapsulated lipids. The rate of lipid digestion can be controlled in a number of ways using this approach, e.g., controlling the permeability of the hydrogel matrix, controlling the dimensions of the hydrogel particles, or controlling the fragmentation or dissociation of the hydrogel particles in the digestive fluids.
The influence of encapsulating lipid droplets within hydrogel particles (calcium alginate beads) on the rate and extent of lipid digestion is shown in Fig. 6c. Three samples containing the sample total amount of lipid (MCT) but with different microstructures were prepared: (i) non-encapsulated lipid droplets; (ii) non-encapsulated lipid droplets mixed with unfilled calcium alginate beads; and, (iii) lipid droplets encapsulated inside calcium alginate beads. The non-encapsulated (free) lipid droplets were completely digested within the first 25 min of hydrolysis, with the amount of FFA released increasing steeply during the first 5 min and then leveling off at longer digestion times (Fig. 6c). On the other hand, the lipid droplets encapsulated within the calcium alginate beads were digested at a much slower rate, with <8% FFA being released within the first 25 min of digestion. The ability of the calcium alginate beads to retard digestion was attributed to a number of physicochemical phenomena: (i) lipase/co-lipase molecules have to diffuse through the hydrogel matrix before they can adsorb to the lipid droplet surfaces; (ii) FFA and MAG have to diffuse out of the hydrogel particles otherwise they will retard the hydrolysis reaction.15,63 Interestingly, when non-encapsulated (free) oil droplets were mixed with the same type and concentration of unfilled calcium alginate beads, there was no inhibition of lipolysis (Fig. 6c). This demonstrated that the beads themselves were not responsible for retarding the lipid digestion process (e.g., by trapping or binding the lipase or bile). Instead, the delayed digestion rate appears to be due to the fact that the droplets are trapped within the gel network.
6.4.4. Other factors.
A number of other factors have been studied recently using the pH stat method, which are summarized below:
Emulsifier type.
Some studies have shown that emulsifier type has a major impact on the rate of lipid digestion, whereas others have found little effect. Wickham and co-workers found that lipid droplets initially coated by phospholipids were digested more rapidly than those coated by proteins.199 Chu and co-workers found that the rate of lipolysis of lipid droplets could be inhibited by coating them with galactolipids with large hydrophilic head-groups that retard adsorption of bile salts and lipase through steric hindrance.179 Reis and co-workers found that the rate of lipid digestion was lower for droplets initially coated by monoglycerides than those coated by proteins or phospholipids.200 Our laboratory previously found that the resistance of lipid droplets stabilized by different emulsifiers to lipid digestion decreased in the following order: non-ionic surfactant (Tween 20) > phospholipids (lecithin) > protein (caseinate or WPI).131 Nevertheless, in a recent study we found that the rate of lipid digestion was fairly similar for MCT oil-in-water emulsions stabilized by four different types of emulsifier: β-Lg, Tween 20, lecithin and lyso-lecithin.172
Dietary fiber coatings.
It has been proposed that the rate of lipid digestion can be controlled by coating lipid droplets with one or more layers of dietary fiber.201 The rationale behind this proposal is the fact that dietary fibers should not be digested in the stomach or small intestine, and therefore they may prevent lipase from adsorbing to lipid droplet surfaces (provided the dietary fibers are not displaced from the droplet surfaces and that the layers formed are impermeable to lipase diffusion). The pH stat method has recently been used to study the impact of dietary fiber type, number of layers, sequence of layers, and layer cross-linking on the rate and extent of lipid digestion.72,86,201,202 These studies found that the lipid digestion rate could be decreased by depositing one or more dietary fiber layers around the lipid droplets, but that the droplets were still digested eventually. Dietary fiber coatings may therefore be a useful means of retarding lipid digestion in the human GI tract, which could be utilized for the development of functional foods to promote satiety.
Droplet physical state.
A number of in vitro studies have shown that the physical state of lipid droplets can influence their digestibility by pancreatic lipase.153,154,203,204 These studies found that solid lipid particles were digested by lipase, but at a slower rate than liquid lipid droplets. Recently, we carried out a study using two emulsions with the same lipid type (tripalmitin), but with one containing lipid droplets that were completely liquid and the other containing lipid particles that were completely solid.155 We found that the rate and extent of lipid digestion were greater in the emulsions containing liquid droplets, but that lipid digestion still occurred in the systems containing solid particles. Other researchers have shown that fat crystallization within lipid droplets may also indirectly influence the rate of lipid digestion.52 In this case, emulsions were designed so that they contained partly crystalline droplets that underwent partial coalescence in the small intestine.27,205 The partially coalesced emulsions exhibited slower digestion than stable emulsions, which was attributed to the fact that it was more difficult for the lipase molecules to reach the lipid droplet surfaces within the large clumps of fat droplets in the unstable system.
Ingredient interactions.
Foods and beverages generally have much more complicated compositions than the simple model systems used in in vitro digestion studies. A number of the components typically found in foods may impact lipid digestion. For example, we recently examined the influence of calcium binding agents (EDTA and alginate) on the rate and extent of lipid digestion.84 We found that both EDTA and alginate could greatly suppress the digestion of triacylglycerols containing long chain fatty acids, which was attributed to their ability to bind free calcium ions. Consequently, there would have been an accumulation of LCFA at the lipid droplet surfaces, which would have inhibited lipase activity.
7. Conclusions
There has been growing interest by food scientists in understanding and controlling the digestion of lipids within the human gastrointestinal tract. The main driving forces for this interest are the development of emulsion-based delivery systems to encapsulate, protect and release lipophilic bioactive components within the GI tract, and the possibility of modulating human hunger and appetite by controlling the location and rate of lipid digestion within the GI tract. This article provides an overview of the major physicochemical events that occur during lipid digestion, and reviews a number of in vitro testing methods that have been developed to monitor lipid digestion. In particular, we focused on the pH stat method for simulating lipid digestion in the small intestine, since this method is useful as a rapid screening tool for studying the influence of product composition and structure on lipid digestibility. Considerable advances have been made in this area throughout the past decade, which is providing the fundamental knowledge required to rationally develop new food-based strategies to tackle food related diseases, such as heart disease, diabetes, cancer, and hypertension.
Acknowledgements
This material is partly based upon work supported by United States Department of Agriculture, CREES, NRI Grants.
References
- Y. Pafumi,
et al., Mechanisms of inhibition of triacylglycerol hydrolysis by human gastric lipase, J. Biol. Chem., 2002, 277(31), 28070–28079 CrossRef CAS.
- C. J. H. Porter and W. N. Charman, In vitro assessment of oral lipid based formulations, Adv. Drug Delivery Rev., 2001, 50, S127–S147 CrossRef CAS.
- C. J. H. Porter, N. L. Trevaskis and W. N. Charman, Lipids and lipid-based formulations: optimizing the oral delivery of lipophilic drugs, Nat. Rev. Drug Discovery, 2007, 6(3), 231–248 CrossRef CAS.
- D. J. McClements, E. A. Decker and Y. Park, Controlling Lipid Bioavailability through Physicochemical and Structural Approaches, Crit. Rev. Food Sci. Nutr., 2009, 49(1), 48–67 CrossRef.
- H. Singh, A. Q. Ye and D. Horne, Structuring food emulsions in the gastrointestinal tract to modify lipid digestion, Prog. Lipid Res., 2009, 48(2), 92–100 CrossRef CAS.
- C. J. H. Porter and K. M. Wasan, Lipid-based systems for the enhanced delivery of poorly water soluble drugs, Adv. Drug Delivery Rev., 2008, 60(6), 615–616 CrossRef CAS.
- C. W. Pouton, Formulation of poorly water-soluble drugs for oral administration: Physicochemical and physiological issues and the lipid formulation classification system, Eur. J. Pharm. Sci., 2006, 29(3–4), 278–287 CrossRef CAS.
-
D. J. McClements, et al., Designing food structure to control stability, digestion, release and absorption of lipophilic food components. in 2nd International Symposium on Delivery of Functionality in Complex Food Systems. 2007. Amherst, MA Search PubMed.
- G. S. Patten,
et al., Site Specific Delivery of Microencapsulated Fish Oil to the Gastrointestinal Tract of the Rat, Dig. Dis. Sci., 2009, 54(3), 511–521 CrossRef CAS.
- A. Shefer and S. Shefer, Novel encapsulation system provides controlled release of ingredients, Food Technology, 2003, 57, 40–43 CAS.
- J. Ubbink, Flavor delivery systems: Trends, technologies and applications, Abstracts of Papers of the American Chemical Society, 2002, 223, U34–U34.
- J. Ubbink and J. Kruger, Physical approaches for the delivery of active ingredients in foods, Trends Food Sci. Technol., 2006, 17(5), 244–254 CrossRef CAS.
- L. Y. Chen, G. E. Remondetto and M. Subirade, Food protein-based materials as nutraceutical delivery systems, Trends Food Sci. Technol., 2006, 17(5), 272–283 CrossRef CAS.
- D. J. McClements,
et al., Structural Design Principles for Delivery of Bioactive Components in Nutraceuticals and Functional Foods, Crit. Rev. Food Sci. Nutr., 2009, 49(6), 577–606 CrossRef CAS.
- G. Fave, T. C. Coste and M. Armand, Physicochemical properties of lipids: New strategies to manage fatty acid bioavailability, Cellular and Molecular Biology, 2004, 50(7), 815–831 Search PubMed.
- C. Beglinger and L. Degen, Fat in the intestine as a regulator of appetite - role of CCK, Physiol. Behav., 2004, 83(4), 617–621 CrossRef CAS.
- D. E. Cummings and J. Overduin, Gastrointestinal regulation of food intake, J. Clin. Invest., 2007, 117(1), 13–23 CrossRef CAS.
- E. Karra and R. L. Batterham, The role of gut hormones in the regulation of body weight and energy homeostasis, Mol. Cell. Endocrinol., 2010, 316(2), 120–128 CrossRef CAS.
-
W. Langhans and N. Geary, Overview of the Physiological Control of Eating, in Frontiers in Eating and Weight Regulation, 2010. p. 9–53 Search PubMed.
- A. D. Strader and S. C. Woods, Gastrointestinal hormones and food intake, Gastroenterology, 2005, 128(1), 175–191 CrossRef CAS.
- M. Golding and T. J. Wooster, The influence of emulsion structure and stability on lipid digestion, Curr. Opin. Colloid Interface Sci., 2010, 15(1–2), 90–101 CrossRef CAS.
- R. V. Seimon,
et al., The droplet size of intraduodenal fat emulsions influences antropyloroduodenal motility, hormone release, and appetite in healthy males, Am. J. Clin. Nutr., 2009, 89(6), 1729–1736 CrossRef CAS.
- Y. Li,
et al., Emulsion-Based Delivery Systems for Tributyrin, a Potential Colon Cancer Preventative Agent, J. Agric. Food Chem., 2009, 57(19), 9243–9249 CrossRef CAS.
- D. J. McClements, E. A. Decker and J. Weiss, Emulsion-based delivery systems for lipophilic bioactive components, J. Food Sci., 2007, 72(8), R109–R124 CrossRef CAS.
-
D. J. McClements, E. A. Decker, and Y. Park, Physicochemical and structural aspects of lipid digestion, in Understanding and Controlling the Microstructure of Complex Foods, D. J. McClements, Editor 2007, CRC Press: Boca Raton, FL. p. 483–503 Search PubMed.
- M. C. Michalski, Specific molecular and colloidal structures of milk fat affecting lipolysis, absorption and postprandial lipemia, Eur. J. Lipid Sci. Technol., 2009, 111(5), 413–431 CrossRef CAS.
-
D. J. McClements, Food Emulsions: Principles, Practice, and Techniques. 2nd ed. CRC series in contemporary food science. 2005, Boca Raton: CRC Press Search PubMed.
-
S. Stauffer, Emulsifiers, 1999, St Paul, MN: Eagen Press Search PubMed.
-
R. J. Whitehurst, Emulsifiers in Food Technology, 2006, Oxford, UK: Blackwell Publishing Search PubMed.
- I. Kralova and J. Sjoblom, Surfactants Used in Food Industry: A Review, J. Dispersion Sci. Technol., 2009, 30(9), 1363–1383 CrossRef CAS.
- R. V. Seimon,
et al., The Particle Size of Intraduodenal (ID) Fat Emulsions Modifies Antropyloroduodenal (APD) Motility, Hormone Release and Appetite in Healthy Males, Gastroenterology, 2009, 136(5), A25–A25.
- T. Sanz and H. Luyten, In vitro evaluation of genistein bioaccessibility from enriched custards, Food Hydrocolloids, 2007, 21(2), 203–211 CrossRef CAS.
- T. Sanz and H. Luyten, Release, partitioning and stability of isoflavones from enriched custards during mouth, stomach and intestine in vitro simulations, Food Hydrocolloids, 2006, 20(6), 892–900 CrossRef CAS.
- T. Sanz and H. Luyten, Effect of thickening agent in the in vitro mouth, stomach and intestine release of tyrosol from enriched custards, Food Hydrocolloids, 2006, 20(5), 703–711 CrossRef CAS.
-
A. van der Bilt, Oral physiology, mastication and food perception, in Designing Functional Foods: Measuring and Controlling Food Structure Breakdown and Nutrient Absorption, D. J. McClements and E. A. Decker, Editors. 2009, CRC Press: Boca Raton, FL. p. 3–37 Search PubMed.
- M. H. Vingerhoeds,
et al., Emulsion flocculation induced by saliva and mucin, Food Hydrocolloids, 2005, 19(5), 915–922 CrossRef CAS.
- M. E. Malone, I. A. M. Appelqvist and I. T. Norton, Oral behaviour of food hydrocolloids and emulsions. Part 1. Lubrication and deposition considerations, Food Hydrocolloids, 2003, 17(6), 763–773 CrossRef CAS.
- M. E. Malone, I. A. M. Appelqvist and I. T. Norton, Oral behaviour of food hydrocolloids and emulsions. Part 2. Taste and aroma release, Food Hydrocolloids, 2003, 17(6), 775–784 CrossRef CAS.
- T. B. J. Blijdenstein,
et al., Scaling behavior of delayed demixing, rheology, and microstructure of emulsions flocculated by depletion and bridging, Langmuir, 2004, 20(26), 11321–11328 CrossRef CAS.
- E. H. A. de Hoog,
et al., Lubrication of oral surfaces by food emulsions: the importance of surface characteristics, J. Food Sci., 2006, 71(7), E337–E341 CrossRef CAS.
- E. Silletti,
et al., The role of electrostatics in saliva-induced emulsion flocculation, Food Hydrocolloids, 2007, 21(4), 596–606 CrossRef CAS.
- E. A. Gwartney, D. K. Larick and E. A. Foegeding, Sensory texture and mechanical properties of stranded and particulate whey protein emulsion gels, J. Food Sci., 2004, 69(9), S333–S339 CAS.
- A. Sarkar, K. K. T. Goh and H. Singh, Colloidal stability and interactions of milk-protein-stabilized emulsions in an artificial saliva, Food Hydrocolloids, 2009, 23(5), 1270–1278 CrossRef CAS.
- L. Kalantzi,
et al., Characterization of the human upper gastrointestinal contents under conditions simulating bioavailability/bioequivalence studies, Pharm. Res., 2006, 23(1), 165–176 CrossRef CAS.
- F. M. L. Amado,
et al., Analysis of the human saliva proteome, Expert Rev. Proteomics, 2005, 2(4), 521–539 Search PubMed.
- R. Vitorino,
et al., Analysis of salivary peptides using HPLC-electrospray mass spectrometry, Biomed. Chromatogr., 2004, 18(8), 570–575 CrossRef CAS.
- R. Vitorino,
et al., Identification of human whole saliva protein components using proteomics, Proteomics, 2004, 4(4), 1109–1115 CrossRef CAS.
-
N. W. Weisbrodt, Swallowing, in Gastrointestinal Physiology, 6th Edition, L. R. Johnson, Editor 2001, Mosby: St. Louis, MI. p. 27–35 Search PubMed.
- H. L. Mu and C. E. Hoy, The digestion of dietary triacylglycerols, Prog. Lipid Res., 2004, 43(2), 105–133 CrossRef CAS.
-
P. Tso, Overview of digestion and absorption, in Biochemical and Physiological Aspects of Human Nutrition, K. D. Crissinger and M. H. Stipanuk, Editors. 2000, W.B. Saunders Company: Philadelphia, Pennsylvania, p. 75–90 Search PubMed.
-
K. E. Barrett, Gastrointestinal Physiology, 2006, New York, NY: McGraw-Hill Search PubMed.
- L. Lundin, M. Golding and T. J. Wooster, Understanding food structure and function in developing food for appetite control, Nutr. Diet., 2008, 65, S79–S85 Search PubMed.
- L. Marciani,
et al., Delaying gastric emptying and enhancing cholecystokinin release and satiety by using acid stable fat emulsions, Gastroenterology, 2006, 130(4), A227–A227.
-
N. W. Weisbrodt, Gastric emptying, in Gastrointestinal Physiology, 6th Edition, L. R. Johnson, Editor 2001, Mosby: St. Louis, MI.p. 37–45 Search PubMed.
-
N. W. Weisbrodt, Motility of the small intestine, in Gastrointestinal Physiology, 6th Edition, L. R. Johnson, Editor 2001, Mosby: St. Louis, MI. p. 47–56 Search PubMed.
- C. Ekmekcioglu, A physiological approach for preparing and conducting intestinal bioavailability studies using experimental systems, Food Chem., 2002, 76(2), 225–230 CrossRef CAS.
- A. Lindahl,
et al., Characterization of fluids from the stomach and proximal jejunum in men and women, Pharm. Res., 1997, 14(4), 497–502 CrossRef CAS.
- K. Schulze, Imaging and modelling of digestion in the stomach and the duodenum, Neurogastroenterol. Motil., 2006, 18(3), 172–183 CrossRef CAS.
- W. Schwizer, M. Fox and A. Steingotter, Non-invasive investigation of gastrointestinal functions with magnetic resonance imaging: towards an “ideal” investigation of gastrointestinal function, Gut, 2003, 52, 34–39 CrossRef.
- A. Shafik,
et al., Mechanism of gastric emptying through the pyloric sphincter: A human study, Medical Science Monitor, 2007, 13(1), CR24–CR29 Search PubMed.
- I. T. Norton, W. J. Frith and S. Ablett, Fluid gels, mixed fluid gels and satiety, Food Hydrocolloids, 2006, 20(2–3), 229–239 CrossRef CAS.
- M. Mukherjee, Human digestive and metabolic lipases - a brief review, J. Mol. Catal. B: Enzym., 2003, 22(5–6), 369–376 CrossRef CAS.
- E. Bauer, S. Jakob and R. Mosenthin, Principles of physiology of lipid digestion, Asian-Australasian Journal of Animal Sciences, 2005, 18(2), 282–295 Search PubMed.
- M. Armand, Lipases and lipolysis in the human digestive tract: where do we stand?, Curr. Opin. Clin. Nutr. Metab. Care, 2007, 10(2), 156–164 CrossRef CAS.
- N. Miled,
et al., Discrimination between closed and open forms of lipases using electrophoretic techniques, Anal. Biochem., 2005, 338(2), 171–178 CrossRef CAS.
- D. C. Whitcomb and M. E. Lowe, Human pancreatic digestive enzymes, Dig. Dis. Sci., 2007, 52(1), 1–17 CrossRef CAS.
- E. Jurado,
et al., Kinetic model for the enzymatic hydrolysis of tributyrin in O/W emulsions, Chem. Eng. Sci., 2006, 61(15), 5010–5020 CrossRef CAS.
- I. Read, Effects of calcium supplementation on circulating lipids - Potential pharmacoeconomic implications, Drugs Aging, 2004, 21(1), 7–17 CrossRef.
- T. Vaskonen, Dietary minerals and modification of cardiovascular risk factors, J. Nutr. Biochem., 2003, 14(9), 492–506 CrossRef CAS.
- T. Karupaiah and K. Sundram, Effects of stereospecific positioning of fatty acids in triacylglycerol structures in native and randomized fats: a review of their nutritional implications, Nutrition and Metabolism, 2007, 4(16), 1–17 Search PubMed.
- P. Reis,
et al., Lipases at interfaces: A review, Adv. Colloid Interface Sci., 2009, 147–148, 237–250 CrossRef CAS.
- M. Hu,
et al., Influence of Tripolyphosphate Cross-Linking on the Physical Stability and Lipase Digestibility of Chitosan-Coated Lipid Droplets, J. Agric. Food Chem., 2010, 58(2), 1283–1289 CrossRef CAS.
- A. Tan,
et al., Silica Nanoparticles To Control the Lipase-Mediated Digestion of Lipid-Based Oral Delivery Systems, Mol. Pharmaceutics, 2010, 7(2), 522–532 CrossRef CAS.
- M. Lowe, The triglyceride lipases of the pancreas, J. Lipid Res., 2002, 43(12), 2007–2016 CAS.
- Y. Gargouri,
et al., Studies on the Detergent Inhibition of Pancreatic Lipase Activity, Journal of Lipid Research, 1983, 24(10), 1336–1342 Search PubMed.
- E. Roger,
et al., Biopharmaceutical parameters to consider in order to alter the fate of nanocarriers after oral delivery, Nanomedicine, 2010, 5(2), 287–306 CrossRef CAS.
- B. Bermudez,
et al., Digestion and absorption of olive oil, Grasas Y Aceites, 2004, 55(1), 1–10 Search PubMed.
- A. W. Basit, Advances in colonic drug delivery, Drugs, 2005, 65(14), 1991–2007 CrossRef CAS.
-
H. J. Flint, S. H. Duncan, and P. Louis, Gut microbial ecology, in Designing Functional Foods: Measuring and Controlling Food Structure Breakdown and Nutrient Absorption, D. J. McClements and E. A. Decker, Editors. 2009, CRC Press: Boca Raton, FL. p. 38–65 Search PubMed.
- L. Yang, Biorelevant dissolution testing of colon-specific delivery systems activated by colonic microflora, J. Controlled Release, 2008, 125(2), 77–86 CrossRef CAS.
- K. M. Tuohy,
et al., Metabolism of Maillard reaction products by the human gut microbiota - implications for health, Mol. Nutr. Food Res., 2006, 50(9), 847–857 CrossRef CAS.
- D. M. Jacobs,
et al., Non-Digestible Food Ingredients, Colonic Microbiota and the Impact on Gut Health and Immunity: A Role for Metabolomics, Curr. Drug Metab., 2009, 10(1), 41–54 Search PubMed.
- N. H. Zangenberg,
et al., A dynamic in vitro lipolysis model I. Controlling the rate of lipolysis by continuous addition of calcium, Eur. J. Pharm. Sci., 2001, 14(2), 115–122 CrossRef CAS.
- M. Hu,
et al., Role of calcium and calcium-binding agents on the lipase digestibility of emulsified lipids using an in vitro digestion model, Food Hydrocolloids, 2010, 24(8), 719–725 CrossRef CAS.
- I. Braccini and S. Perez, Molecular basis of Ca2+-induced gelation in alginates and pectins: The egg-box model revisited, Biomacromolecules, 2001, 2(4), 1089–1096 CrossRef CAS.
- S. Mun,
et al., Influence of interfacial composition on in vitro digestibility of emulsified lipids: Potential mechanism for chitosan's ability to inhibit fat digestion, Food Biophys., 2006, 1(1), 21–29 CrossRef.
- P. Rayment,
et al., Investigation of alginate beads for gastro-intestinal functionality, Part 1: In vitro characterisation, Food Hydrocolloids, 2009, 23(3), 816–822 CrossRef CAS.
- C. Hoad,
et al., Investigation of alginate beads for gastro-intestinal functionality, Part 2: In vivo characterisation, Food Hydrocolloids, 2009, 23(3), 833–839 CrossRef CAS.
- J. N. Losso, The biochemical and functional food properties of the Bowman-Birk inhibitor, Crit. Rev. Food Sci. Nutr., 2008, 48(1), 94–118 CrossRef CAS.
- M. P. Raghavendra, P. R. Kumar and V. Prakash, Mechanism of inhibition of rice bran lipase by polyphenols - A case study with chlorogenic acid and caffeic acid, J. Food Sci., 2007, 72(8), E412–E419 CrossRef CAS.
- P. Reis,
et al., Lipases at interfaces: Unique interfacial properties as globular proteins, Langmuir, 2008, 24(13), 6812–6819 CrossRef CAS.
- C. L. Cooper,
et al., Polyelectrolyte-protein complexes, Curr. Opin. Colloid Interface Sci., 2005, 10(1–2), 52–78 CrossRef CAS.
- A. Mackie and A. Macierzanka, Colloidal aspects of protein digestion, Curr. Opin. Colloid Interface Sci., 2010, 15(1–2), 102–108 CrossRef CAS.
- J. Maldonado-Valderrama,
et al., The effect of physiological conditions on the surface structure of proteins: Setting the scene for human digestion of emulsions, Eur. Phys. J. E, 2009, 30(2), 165–174 CrossRef CAS.
- J. Maldonado-Valderrama,
et al., Interfacial characterization of beta-lactoglobulin networks: Displacement by bile salts, Langmuir, 2008, 24(13), 6759–6767 CrossRef CAS.
-
G. A. van Aken and E. H. A. de Hoog, Oral processing and perception of food emulsions: relevence for fat reduction in foods, in Designing Functional Foods: Measuring and Controlling Food Structure Breakdown and Nutrient Absorption, D. J. McClements and E. A. Decker, Editors. 2009, CRC Press: Boca Raton, FL. p. 265–294 Search PubMed.
-
G. A. van Aken, M. H. Vingerhoeds, and E. H. A. de Hoog, Colloidal behavior of food emulsions under oral conditions, in Food Hydrocolloids, Interactions, Microstructure and Processing, E. Dickinson, Editor 2005, The Royal Society of Chemistry: Cambridge, UK. p. 356–366 Search PubMed.
- J. S. Patton and M. C. Carey, Watching Fat Digestion, Science, 1979, 204(4389), 145–148 CrossRef CAS.
- J. S. Patton,
et al., The Light-Microscopy of Triglyceride Digestion, Food Microstructure, 1985, 4(1), 29–41 Search PubMed.
- T. Helgason,
et al., Influence of molecular character of chitosan on the adsorption of chitosan to oil droplet interfaces in an in vitro digestion model, Food Hydrocolloids, 2009, 23(8), 2243–2253 CrossRef CAS.
-
C. H. M. Versantvoort, E. Van de Kamp, and C. J. M. Rompelberg, Development and applicability of an in vitro digestion model in assessing the bioaccessibility of contaminants from food, in Report Number 3201020022004, National Institute of Public Health and the Environment: Bilthoven, The Netherlands. p. 1–87 Search PubMed.
- B. Holst and G. Williamson, Nutrients and phytochemicals: from bioavailability to bioefficacy beyond antioxidants, Curr. Opin. Biotechnol., 2008, 19(2), 73–82 CrossRef CAS.
- H. Verhagen,
et al., Assessment of the efficacy of functional food ingredients-introducing the concept “kinetics of biomarkers”, Mutat. Res., Fundam. Mol. Mech. Mutagen., 2004, 551(1–2), 65–78 CrossRef CAS.
- S. Scholz and G. Williamson, Interactions affecting the Bioavailability of dietary polyphenols in vivo, Int. J. Vitam. Nutr. Res., 2007, 77(3), 224–235 CrossRef CAS.
- J. Y. Yoo and X. D. Chen, GIT Physicochemical Modeling - A Critical Review, International Journal of Food Engineering, 2006, 2(4) Search PubMed.
- M. Minekus,
et al., A computer-controlled system to simulate conditions of the large intestine with peristaltic mixing, water absorption and absorption of fermentation products, Appl. Microbiol. Biotechnol., 1999, 53(1), 108–114 CrossRef CAS.
-
K. Venema, R. Havenaar, and M. Minekus, Improving In vitro Simulation of the Stomach and Intestines, in Designing Functional Foods: Measuring and Controlling Food Structure Breakdown and Nutrient Absorption, D. J. McClements and E. A. Decker, Editors. 2009, CRC Press: Boca Raton, FL. p. 314–339 Search PubMed.
- C. H. M. Versantvoort,
et al., Applicability of an in vitro digestion model in assessing the bioaccessibility of mycotoxins from food, Food Chem. Toxicol., 2005, 43(1), 31–40 CrossRef CAS.
- D. M. Dresselhuis,
et al., Fat retention at the tongue and the role of saliva: Adhesion and spreading of ‘protein-poor’ versus ‘protein-rich’ emulsions, J. Colloid Interface Sci., 2008, 321(1), 21–29 CrossRef CAS.
- D. M. Dresselhuis,
et al., Tribology of o/w emulsions under mouth-like conditions: Determinants of friction, Food Biophys., 2007, 2(4), 158–171 CrossRef.
- G. A. van Aken, M. H. Vingerhoeds and E. H. A. de Hoog, Food colloids under oral conditions, Curr. Opin. Colloid Interface Sci., 2007, 12(4–5), 251–262 CrossRef CAS.
-
I. A. M. Appelqvist, Measuring the Oral Behavior of Foods, in Designing Functional Foods: Measuring and Controlling Food Structure Breakdown and Nutrient Absorption, D. J. McClements and E. A. Decker, Editors. 2009, CRC Press: Boca Raton, FL. p. 265–294 Search PubMed.
- A. Sarkar,
et al., Behaviour of an oil-in-water emulsion stabilized by beta-lactoglobulin in an in vitro gastric model, Food Hydrocolloids, 2009, 23(6), 1563–1569 CrossRef CAS.
- A. Mercuri,
et al., Dynamic gastric model (DGM): a novel in vitro apparatus to assess the impact of gastric digestion on the droplet size of self-emulsifying drug-delivery systems, J. Pharm. Pharmacol., 2008, 60, 4 CrossRef.
- A. des Rieux,
et al., Nanoparticles as potential oral delivery systems of proteins and vaccines: A mechanistic approach, J. Controlled Release, 2006, 116(1), 1–27 CrossRef CAS.
- C. J. Rummey and I. R. Rowland, In vivo and in vitro models of the human colonic flora, Crit. Rev. Food Sci. Nutr., 1992, 31, 299–331 CrossRef.
- G. T. Macfarlane and S. Macfarlane, Models for intestinal fermentation: association between food components, delivery systems, bioavailability and functional interactions in the gut, Curr. Opin. Biotechnol., 2007, 18(2), 156–162 CrossRef CAS.
-
A. C. Ouwehand, et al., Non-starch polysaccharides in the gastrointestinal tract, in Designing Functional Foods: Measuring and Controlling Food Structure Breakdown and Nutrient Absorption, D. J. McClements and E. A. Decker, Editors. 2009, CRC Press: Boca Raton, FL. p. 126–147 Search PubMed.
- J. F. A. Souto-Maior,
et al., Phosphated crosslinked pectin as a potential excipient for specific drug delivery: preparation and physicochemical characterization, Polym. Int., 2009, 59(1), 127–135.
- A. Dahan and A. Hoffman, Use of a dynamic in vitro lipolysis model to rationalize oral formulation development for poor water soluble drugs: Correlation with in vivo data and the relationship to intra-enterocyte processes in rats, Pharm. Res., 2006, 23(9), 2165–2174 CrossRef CAS.
-
C. W. Pouton and C. J. H. Porter. Formulation of lipid-based delivery systems for oral administration: Materials, methods and strategies. in Annual Meeting of the American-Association-of-Pharmaceutical-Scientists. 2006. San Antonio, TX Search PubMed.
- A. Dahan and A. Hoffman, Rationalizing the selection of oral lipid based drug delivery systems by an in vitro dynamic lipolysis model for improved oral bioavailability of poorly water soluble drugs, J. Controlled Release, 2008, 129(1), 1–10 CrossRef CAS.
- S. Amara,
et al., Lipolysis of natural long chain and synthetic medium chain galactolipids by pancreatic lipase-related protein 2, Biochim. Biophys. Acta, Mol. Cell Biol. Lipids, 2010, 1801(4), 508–516 CrossRef CAS.
- L. Sek, C. J. H. Porter and W. N. Charman, Characterisation and quantification of medium chain and long chain triglycerides and their in vitro digestion products, by HPTLC coupled with in situ densitometric analysis, J. Pharm. Biomed. Anal., 2001, 25(3–4), 651–661 CrossRef CAS.
- M. Armand,
et al., Effects of Droplet Size, Triacylglycerol Composition, and Calcium on the Hydrolysis of Complex Emulsions by Pancreatic Lipase - an Invitro Study, J. Nutr. Biochem., 1992, 3(7), 333–341 CrossRef CAS.
-
P. J. Moughan, Digestion and Absorption of Proteins and Peptides, in Designing Functional Foods: Measuring and Controlling Food Structure Breakdown and Nutrient Absorption, D. J. McClements and E. A. Decker, Editors. 2009, CRC Press: Boca Raton, FL. p. 148–170 Search PubMed.
- B. Pedersen and B. O. Eggum, Prediction of Protein Digestibility by invitro Procedures Based on 2 Multi-Enzyme Systems, Zeitschrift Fur Tierphysiologie Tierernahrung Und Futtermittelkunde-Journal of Animal Physiology and Animal Nutrition, 1981, 45(4), 190–200 Search PubMed.
- A. Sarkar, K. K. T. Goh and H. Singh, Properties of oil-in-water emulsions stabilized by beta-lactoglobulin in simulated gastric fluid as influenced by ionic strength and presence of mucin, Food Hydrocolloids, 2010, 24(5), 534–541 CrossRef CAS.
-
D. J. McClements, Emulsion Design to Improve the Delivery of Functional Lipophilic Components, in Annual Review of Food Science and Technology, Vol. 1, 2010. p. 241–269 Search PubMed.
- L. Lundin and M. Golding, Structure design for healthy food, Australian Journal of Dairy Technology, 2009, 64(1), 68–74 Search PubMed.
- S. Mun, E. A. Decker and D. J. McClements, Influence of emulsifier type on in vitro digestibility of lipid droplets by pancreatic lipase, Food Res. Int., 2007, 40(6), 770–781 CrossRef CAS.
- A. Sarkar, D. S. Horne and H. Singh, Interactions of milk protein-stabilized oil-in-water emulsions with bile salts in a simulated upper intestinal model, Food Hydrocolloids, 2010, 24(2–3), 142–151 CrossRef CAS.
- R. Lutz,
et al., On the confocal images and the rheology of whey protein isolated and modified pectins associated complex, Colloids Surf., B, 2009, 69(1), 43–50 CrossRef CAS.
- D. J. McClements, Critical review of techniques and methodologies for characterization of emulsion stability, Crit. Rev. Food Sci. Nutr., 2007, 47(7), 611–649 CrossRef CAS.
- V. Rampon,
et al., Evidence that homogenization of BSA-stabilized hexadecane-in-water emulsions induces structure modification of the nonadsorbed protein, J. Agric. Food Chem., 2003, 51(20), 5900–5905 CrossRef CAS.
- V. Rampon,
et al., Front-face fluorescence spectroscopy study of globular proteins in emulsions: Displacement of BSA by a nonionic surfactant, J. Agric. Food Chem., 2003, 51(9), 2482–2489 CrossRef CAS.
- H. Bouwmeester,
et al., Review of health safety aspects of nanotechnologies in food production, Regul. Toxicol. Pharmacol., 2009, 53(1), 52–62 CrossRef CAS.
- W. I. Hagens,
et al., What do we (need to) know about the kinetic properties of nanoparticles in the body?, Regul. Toxicol. Pharmacol., 2007, 49(3), 217–229 CrossRef CAS.
- P. Reis,
et al., Competition between lipases and monoglycerides at interfaces, Langmuir, 2008, 24(14), 7400–7407 CrossRef CAS.
- C. Leggio,
et al., Small-angle X-ray scattering and light scattering
on lysozyme and sodium glycocholate micelles, J. Phys. Chem. B, 2005, 109(50), 23857–23869 CrossRef CAS.
- D. G. Fatouros, B. Bergenstahl and A. Mullertz, Morphological observations on a lipid-based drug delivery system during in vitro digestion, Eur. J. Pharm. Sci., 2007, 31(2), 85–94 CrossRef CAS.
- Y. Nonomura,
et al., Phase behavior of bile acid/lipid/water systems containing model dietary lipids, J. Colloid Interface Sci., 2009, 339(1), 222–229 CrossRef CAS.
- D. G. Fatouros,
et al., Structural development of self nano emulsifying drug delivery systems (SNEDDS) during In vitro lipid digestion monitored by small-angle X-ray scattering, Pharm. Res., 2007, 24(10), 1844–1853 CrossRef CAS.
- R. P. Hjelm,
et al., Structure of conjugated bile salt-fatty acid-monoglyceride mixed colloids: Studies by small-angle neutron scattering, J. Phys. Chem. B, 2000, 104(2), 197–211 CrossRef CAS.
- D. G. Fatouros,
et al., Physicochemical Characterization of Simulated Intestinal Fed-State Fluids Containing Lyso-Phosphatidylcholine and Cholesterol, Dissolution Technologies, 2009, 16(3), 47–50 Search PubMed.
- D. G. Fatouros,
et al., Colloidal Structures in Media Simulating Intestinal Fed State Conditions with and Without Lipolysis Products, Pharm. Res., 2009, 26(2), 361–374 CrossRef CAS.
- J. Singh,
et al., Aggregate properties of sodium deoxycholate and dimyristoylphosphatidylcholine mixed micelles, J. Phys. Chem. B, 2008, 112(13), 3997–4008 CrossRef CAS.
- A. Rube, S. Klein and K. Mader, Monitoring of in vitro fat digestion by electron paramagnetic resonance spectroscopy, Pharm. Res., 2006, 23(9), 2024–2029 CrossRef.
-
M. A. Rao, Rheology of Fluids and Semisolid Foods: Principles and Applications, 1999, New York: Springer. 433 Search PubMed.
- T. F. Tadros, Fundamental Principles of Emulsion Rheology and Their Applications, Colloids Surf., A, 1994, 91, 39–55 CrossRef CAS.
-
C. W. Macosko, Rheology: Principles, Measurements and Applications, 1994, New York, NY: VCH Publishers Search PubMed.
- D. H. Liao, J. B. Zhao and H. Gregersen, Gastrointestinal tract modelling in health and disease, World J. Gastroenterol., 2009, 15(2), 169–176 Search PubMed.
- C. Olbrich, O. Kayser and R. H. Muller, Lipase degradation of Dynasan 114 and 116 solid lipid nanoparticles (SLN) - effect of surfactants, storage time and crystallinity, Int. J. Pharm., 2002, 237(1–2), 119–128 CrossRef CAS.
- R. H. Muller, D. Ruhl and S. A. Runge, Biodegradation of solid lipid nanoparticles as a function of lipase incubation time, Int. J. Pharm., 1996, 144(1), 115–121 CrossRef.
- L. Bonnaire,
et al., Influence of lipid physical state on the in vitro digestibility of emulsified lipids, J. Agric. Food Chem., 2008, 56(10), 3791–3797 CrossRef CAS.
- S. Ueno, Y. Hamada and K. Sato, Controlling polymorphic crystallization of n-alkane crystals in emulsion droplets through interfacial heterogeneous nucleation, Cryst. Growth Des., 2003, 3(6), 935–939 CrossRef CAS.
- M. Higami,
et al., Simultaneous synchrotron radiation X-ray diffraction - DSC analysis of melting and crystallization behavior of trilauroylglycerol in nanoparticles of oil-in-water emulsion, J. Am. Oil Chem. Soc., 2003, 80(8), 731–739 CrossRef CAS.
- D. G. Fatouros and A. Mullertz, In vitro lipid digestion models in design of drug delivery systems for enhancing oral bioavailability, Expert Opin. Drug Metab. Toxicol., 2008, 4(1), 65–76 Search PubMed.
-
M. L. Failla, T. Huo, and S. K. Thakkar. In vitro screening of relative bioaccessibility of carotenoids from foods. in 10th Asian Congress of Nutrition. 2007. Taipei, TAIWAN: H E C Press, Healthy Eating Club Pty Ltd Search PubMed.
- J. O. Christensen,
et al., Solubilisation of poorly water-soluble drugs during in vitro lipolysis of medium- and long-chain triacylglycerols, Eur. J. Pharm. Sci., 2004, 23(3), 287–296 CrossRef CAS.
- T. Do Thi,
et al., Formulate-ability of ten compounds with different physicochemical profiles in SMEDDS, Eur. J. Pharm. Sci., 2009, 38(5), 479–488 CrossRef.
- S. Mun, E. A. Decker and D. J. McClements, Influence of droplet characteristics on the formation of oil-in-water emulsions stabilized by surfactant-chitosan layers, Langmuir, 2005, 21(14), 6228–6234 CrossRef CAS.
- M. Brogard,
et al., A
new standardized lipolysis approach for characterization of emulsions and dispersions, J. Colloid Interface Sci., 2007, 308(2), 500–507 CrossRef CAS.
- V. Tyssandier,
et al., Processing of vegetable-borne carotenoids in the human stomach and duodenum, American Journal of Physiology-Gastrointestinal and Liver Physiology, 2003, 284(6), G913–G923 Search PubMed.
- V. Tyssandier, B. Lyan and P. Borel, Main factors governing the transfer of carotenoids from emulsion lipid droplets to micelles, Biochim. Biophys. Acta, Mol. Cell Biol. Lipids, 2001, 1533(3), 285–292 CrossRef CAS.
- A. J. Wright, C. Pietrangelo and A. MacNaughton, Influence of simulated upper intestinal parameters on the efficiency of beta carotene micellarisation using an in vitro model of digestion, Food Chemistry, 2008, 107(3), 1253–1260 CAS.
- A. Dahan and A. Hoffman, The effect of different lipid based formulations on the oral absorption of lipophilic drugs: The ability of in vitro lipolysis and consecutive ex vivo intestinal permeability data to predict in vivo bioavailability in rats, Eur. J. Pharm. Biopharm., 2007, 67(1), 96–105 CrossRef CAS.
- C. H. M. Versantvoort,
et al., Monolayers of IEC-18 cells as an in vitro model for screening the passive transcellular and paracellular transport across the intestinal barrier: comparison of active and passive transport with the human colon carcinoma Caco-2 cell line (vol 11, pg 335, 2002), Environ. Toxicol. Pharmacol., 2003, 13(1), 55–55 CrossRef CAS.
- E. Reboul,
et al., Bioaccessibility of carotenoids and vitamin E from their main dietary sources, J. Agric. Food Chem., 2006, 54(23), 8749–8755 CrossRef CAS.
- C. Dhuique-Mayer,
et al., beta-Cryptoxanthin from Citrus juices: assessment of bioaccessibility using an in vitro digestion/Caco-2 cell culture model, Br. J. Nutr., 2007, 97(5), 883–890 CrossRef CAS.
- N. O. Nilsson and P. Belfrage, Continuous Monitoring of Free Fatty-Acid Release from Adipocytes by Ph-Stat Titration, Journal of Lipid Research, 1979, 20(4), 557–560 Search PubMed.
- Y. Li and D. J. McClements, New Mathematical Model for Interpreting pH-Stat Digestion Profiles: Impact of Lipid Droplet Characteristics on in Vitro Digestibility, J. Agric. Food Chem., 2010, 58(13), 8085–8092 CrossRef CAS.
- J. M. Lohr,
et al., Properties of different pancreatin preparations used in pancreatic exocrine insufficiency, Eur. J. Gastroenterol. Hepatol., 2009, 21(9), 1024–1031 CrossRef.
- P. D. de Maria,
et al., Role of apparent pK(a) of carboxylic acids in lipase-catalyzed esterifications in biphasic systems, J. Mol. Catal. B: Enzym., 2009, 59(1–3), 220–224 CrossRef.
- H. Ali, A. Siddiqui and S. Nazzal, The Effect of Media Composition, pH, and Formulation Excipients on the In Vitro Lipolysis of Self-Emulsifying Drug Delivery Systems (SEDDS), J. Dispersion Sci. Technol., 2010, 31(2), 226–232 CrossRef CAS.
- D. Y. Colin,
et al., Exploring the active site cavity of human pancreatic lipase, Biochem. Biophys. Res. Commun., 2008, 370(3), 394–398 CrossRef CAS.
- P. Reis,
et al., Lipase reaction at interfaces as self-limiting processes, C. R. Chim., 2009, 12(1–2), 163–170 CrossRef CAS.
- A. Macierzanka,
et al., Emulsification alters simulated gastrointestinal proteolysis of beta-casein and beta-lactoglobulin, Soft Matter, 2009, 5(3), 538–550 RSC.
- B. S. Chu,
et al., Modulating Pancreatic Lipase Activity with Galactolipids: Effects of Emulsion Interfacial Composition, Langmuir, 2009, 25(16), 9352–9360 CrossRef CAS.
- Y. Li, M. Hu and D. J. McClements, Factors Affecting Lipase Digestibility of Emulsified Lipids using an in vitro Digestion Model: Proposal for a Standardized pH-Stat Method, Food Chemistry, 2010 Search PubMed Submitted.
- J. S. Patton and M. C. Carey, Inhibition of Human Pancreatic Lipase-Colipase Activity by Mixed Bile Salt-Phospholipid Micelles, American Journal of Physiology, 1981, 241(4), G328–G336 CAS.
- N. H. Zangenberg,
et al., A dynamic in vitro lipolysis model II: Evaluation of the model, Eur. J. Pharm. Sci., 2001, 14(3), 237–244 CrossRef CAS.
- T. F. Whayne and J. M. Felts, Activation of Lipoprotein Lipase - an Evaluation of Calcium as a Cofactor, Journal of the American Oil Chemists Society, 1971, 48(2), A101 Search PubMed.
- T. F. Whayne and J. M. Felts, Activation of Lipoprotein Lipase - Evaluation of Calcium, Magnesium, and Ammonium as Cofactors, Circulation Research, 1971, 28(6), 649 CAS.
- H. Kimura,
et al., Activation of Human Pancreatic Lipase Activity by Calcium and Bile-Salts, Journal of Biochemistry, 1982, 92(1), 243–251 Search PubMed.
- S. Hwang,
et al., Highly efficient production of monoglycerides by the continuous removal of fatty acids from lipase-catalyzed oil hydrolysis, Biocatal. Biotransform., 2009, 27(5–6), 290–295 CrossRef CAS.
- J. K. Lorenzen,
et al., Effect of dairy calcium or supplementary calcium intake on postprandial fat metabolism, appetite, and subsequent energy intake, American Journal of Clinical Nutrition, 2007, 85(3), 678–687 Search PubMed.
- K. E. Scholz-Ahrens and J. Schrezenmeir, Milk minerals and the metabolic syndrome, Int. Dairy J., 2006, 16(11), 1399–1407 CrossRef CAS.
- X. U. Rui, Calcium binding of peptides derived from enzymatic hydrolysates of whey protein concentrate, Int. J. Dairy Technol., 2009, 62(2), 170–173 CrossRef CAS.
- S. B. Kim and J. W. Lim, Calcium-binding peptides derived from tryptic hydrolysates of cheese whey protein, Asian-Australasian Journal of Animal Sciences, 2004, 17(10), 1459–1464 Search PubMed.
- T. D. Perry, R. T. Cygan and R. Mitchell, Molecular models of alginic acid: Interactions with calcium ions and calcite surfaces, Geochim. Cosmochim. Acta, 2006, 70(14), 3508–3532 CrossRef CAS.
- S. J. Hur, E. A. Decker and D. J. McClements, Influence of initial emulsifier type on microstructural changes occurring in emulsified lipids during in vitro digestion, Food Chem., 2009, 114(1), 253–262 CrossRef CAS.
- J. Fallingborg, Intraluminal pH of the human gastrointestinal tract, Danish Medical Bulletin, 1999, 46(3), 183–196 Search PubMed.
- T. Snabe, M. T. Neves-Petersen and S. B. Petersen, Enzymatic lipid removal from surfaces-lipid desorption by a pH-induced “electrostatic explosion”, Chem. Phys. Lipids, 2005, 133(1), 37–49 CrossRef CAS.
- M. Beysseriat, E. A. Decker and D. J. McClements, Preliminary study of the influence of dietary fiber on the properties of oil-in-water emulsions passing through an in vitro human digestion model, Food Hydrocolloids, 2006, 20(6), 800–809 CrossRef CAS.
- K. Nakahara,
et al., Inhibition of Postprandial Hyperglycemia by Oolong Tea Extract (OTE), Phytother. Res., 1994, 8(7), 433–435 CrossRef.
-
C. J. H. Porter, et al. Enhancing intestinal drug solubilisation using lipid-based delivery systems. in Annual Meeting of the American-Association-of-Pharmaceutical-Scientists. 2006. San Antonio, TX Search PubMed.
- P. Borel,
et al., Hydrolysis of Emulsions with Different Triglycerides and Droplet Sizes by Gastric Lipase in vitro - Effect on Pancreatic Lipase Activity, J. Nutr. Biochem., 1994, 5(3), 124–133 CrossRef CAS.
- M. Wickham,
et al., Modification of a phospholipid stabilized emulsion interface by bile salt: effect on pancreatic lipase activity, Journal of Lipid Research, 1998, 39(3), 623–632 Search PubMed.
- P. M. Reis,
et al., Influence of Surfactants on Lipase Fat Digestion in a Model Gastro-intestinal System, Food Biophys., 2008, 3(4), 370–381 CrossRef.
- D. J. McClements, Design of Nano-Laminated Coatings to Control Bioavailability of Lipophilic Food Components, J. Food Sci., 2010, 75(1), R30–R42 CrossRef CAS.
- U. Klinkesorn and D. J. McClements, Impact of Lipase, Bile Salts, and Polysaccharides on Properties and Digestibility of Tuna Oil Multilayer Emulsions Stabilized by Lecithin-Chitosan, Food Biophys., 2010, 5(2), 73–81 CrossRef.
- C. Olbrich, O. Kayser and R. H. Muller, Enzymatic degradation of Dynasan 114 SLN - effect of surfactants and particle size, J. Nanopart. Res., 2002, 4(1/2), 121–129 CrossRef CAS.
- C. Olbrich and R. H. Muller, Enzymatic degradation of SLN - effect of surfactant and surfactant mixtures, Int. J. Pharm., 1999, 180(1), 31–39 CrossRef CAS.
-
P. Walstra, Physical Chemistry of Foods, 2003, New York, NY: Marcel Decker Search PubMed.
- A. Abdalla, S. Klein and K. Mäder, A new self-emulsifying drug delivery system (SEDDS) for poorly soluble drugs: Characterization, dissolution, in vitro digestion and incorporation into solid pellets, Eur. J. Pharm. Sci., 2008, 35(5), 457–464 CrossRef CAS.
- S. Sandra, E. A. Decker and D. J. McClements, Effect of interfacial protein cross-linking on the in vitro digestibility of emulsified corn oil by pancreatic lipase, J. Agric. Food Chem., 2008, 56(16), 7488–7494 CrossRef CAS.
- U. Lesmes, P. Baudot and D. J. McClements, Impact of Interfacial Composition on Physical Stability and In Vitro Lipase Digestibility of Triacylglycerol Oil Droplets Coated with Lactoferrin and/or Caseinate, J. Agric. Food Chem., 2010, 58(13), 7962–7969 CrossRef CAS.
- V. Gudipati, S. Sandra, D. J. McClements and E. A. Decker, Oxidative Stability and in Vitro Digestibility of Fish Oil-in-Water Emulsions Containing Multilayered Membranes, J. Agric. Food Chem., 2010, 58(13), 8093–8099 CrossRef CAS.
- U. Klinkesorn and D. J. McClements, Influence of chitosan on stability and lipase digestibility of lecithin-stabilized tuna oil-in-water emulsions, Food Chem., 2009, 114(4), 1308–1315 CrossRef CAS.
- M. Wickham, M. Garrood, J. Leney, P. D. G. Wilson and A. Fillery-Travis, Modification of a phospholipid stabilized emulsion interface by bile salt: effect on pancreatic lipase activity, J. Lipid Res., 1998, 39(3), 623–632 CAS.
- L. Sek, C. J. H. Porter, A. M. Kaukonen and W. N. Charman, Evaluation of the in vitro digestion profiles of long and medium chain glycerides and the phase behaviour of their lipolytic products, J. Pharm. Pharmacol., 2002, 54(1), 29–41 CrossRef CAS.
- S. Fernandez, S. Chevrier, N. Ritter, B. Mahler, F. Demarne, F. Carriere and V. Jannin, In Vitro Gastrointestinal Lipolysis of Four Formulations of Piroxicam and Cinnarizine with the Self Emulsifying Excipients Labrasol (R) and Gelucire (R) 44/14, Pharm. Res., 2009, 26(8), 1901–1910 CrossRef CAS.
- E. Hedren, V. Diaz and U. Svanberg, Estimation of carotenoid accessibility from carrots determined by an in vitro digestion method, Eur. J. Clin. Nutr., 2002, 56(5), 425–430 CrossRef CAS.
- D. A. White, I. D. Fisk, S. Makkhun and D. A. Gray, In Vitro Assessment of the Bioaccessibility of Tocopherol and Fatty Acids from Sunflower Seed Oil Bodies, J. Agric. Food Chem., 2009, 57(13), 5720–5726 CrossRef CAS.
- C. M. Oliver, M. A. Augustin and L. Sanguansri, Maillard-based casein-carbohydrate microcapsules for the delivery of fish oil: emulsion stability during in vitro digestion, Australian Journal of Dairy Technology, 2009, 64(1), 80–83 Search PubMed.
- C. Chung, L. Sanguansri and M. A. Augustin, Effects of modification of encapsulant materials on the susceptibility of fish oil microcapsules to lipolysis, Food Biophys., 2008, 3(2), 140–145 CrossRef.
- C. J. H. Porter, A. M. Kaukonen, B. J. Boyd, G. A. Edwards and W. N. Charman, Susceptibility to lipase-mediated digestion reduces the oral bioavailability of danazol after administration as a medium-chain lipid-based microemulsion formulation, Pharm. Res., 2004, 21(8), 1405–1412 CrossRef CAS.
- C. J. H. Porter, A. M. Kaukonen, A. Taillardat-Bertschinger, B. J. Boyd, J. M. O'Connor, G. A. Edwards and W. N. Charman, Use of in vitro lipid digestion data to explain the in vivo performance of triglyceride-based oral lipid formulations of poorly water-soluble drugs: Studies with halofantrine, J. Pharm. Sci., 2004, 93(5), 1110–1121 CrossRef CAS.
- A. M. Kaukonen, B. J. Boyd, W. N. Charman and C. J. H. Porter, Drug solubilization behavior during in vitro digestion of suspension formulations of poorly water-soluble drugs in triglyceride lipids, Pharm. Res., 2004, 21(2), 254–260 CrossRef CAS.
- C. Juhel, M. Armand, Y. Pafumi, C. Rosier, J. Vandermander and D. Lairon, Green tea extract (AR25 (R)) inhibits lipolysis of triglycerides in gastric and duodenal medium in vitro, J. Nutr. Biochem., 2000, 11(1), 45–51 CrossRef CAS.
- B. Pasquier, M. Armand, C. Castelain, F. Guillon, P. Borel, H. Lafont and D. Lairon, Emulsification and lipolysis of triacylglycerols are altered by viscous soluble dietary fibres in acidic gastric medium in vitro, Biochemical Journal, 1996, 314, 269–275 CAS.
- B.-S. Chu, G. T. Rich, M. J. Ridout, R. M. Faulks, M. S. J. Wickham and P. J. Wilde, Modulating Pancreatic Lipase Activity with Galactolipids: Effects of Emulsion Interfacial Composition, Langmuir, 2009, 25(16), 9352–9360 CrossRef CAS.
- A. Sarkar, D. S. Horne and H. Singh, Pancreatin-induced coalescence of oil-in-water emulsions in an in vitro duodenal model, Int. Dairy J., 2010, 20(9), 589–597 CrossRef CAS.
- S. F. Han, T. T. Yao, X. X. Zhang, L. Gan, C. L. Zhu, H. Z. Yua and Y. Gan, Lipid-based formulations to enhance oral bioavailability of the poorly water-soluble drug anethol trithione: Effects of lipid composition and formulation, Int. J. Pharm., 2009, 379(1), 18–24 CrossRef CAS.
|
This journal is © The Royal Society of Chemistry 2010 |