DOI:
10.1039/B918763B
(Critical Review)
Chem. Soc. Rev., 2010,
39, 301-312
Green Chemistry: Principles and Practice
Received
9th September 2009
First published on
20th November 2009
Abstract
Green Chemistry is a relatively new emerging field that strives to work at the molecular level to achieve sustainability. The field has received widespread interest in the past decade due to its ability to harness chemical innovation to meet environmental and economic goals simultaneously. Green Chemistry has a framework of a cohesive set of Twelve Principles, which have been systematically surveyed in this critical review. This article covers the concepts of design and the scientific philosophy of Green Chemistry with a set of illustrative examples. Future trends in Green Chemistry are discussed with the challenge of using the Principles as a cohesive design system (93 references).
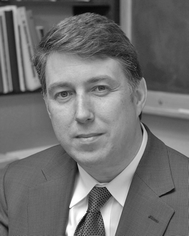
Paul Anastas
| Paul T. Anastas is the Teresa and H. John Heinz III Professor in the Practice of Chemistry for the Environment and the Director of the Center for Green Chemistry and Green Engineering at Yale University. Trained as a synthetic organic chemist, Dr Anastas received his PhD from Brandeis University and is credited with establishing the field of green chemistry in 1991. Dr Anastas has published ten books and numerous papers on sustainability through science. |
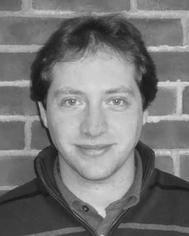
Nicolas Eghbali
| Nicolas Eghbali earned a BS in Molecular Biology and a MS in chemistry from Bordeaux I University (France). After completing his PhD dissertation in 2007 under the supervision of both Professors Chao-Jun Li and David Harpp at McGill University, he joined the Center for Green Chemistry and Green Engineering at Yale, where he worked as a post-doctoral research associate. His PhD work was centered on the utilization of carbon dioxide and his current research interests range from green organic synthesis to material science and biocatalysis. |
I Introduction
Green Chemistry is defined as the “design of chemical products and processes to reduce or eliminate the use and generation of hazardous substances.”1,2 This definition and the concept of Green Chemistry were first formulated at the beginning of the 1990s nearly 20 years ago.3 In the years since, there has been international adoption that resulted in the creation of literally hundreds of programs and governmental initiatives on Green Chemistry around the world with initial leading programs located in the U.S., United Kingdom, and Italy.4 These have played a significant role in informing sustainable design.5 Important early programs include the US Presidential Green Chemistry Challenge Awards established in 1995,6 the Green Chemistry Institute founded in 1997,7 and the publication of the first volume of the now well-established Green Chemistry journal of the Royal Society of Chemistry in 1999.8
The most important aspect of Green Chemistry is the concept of design. Design is a statement of human intention and one cannot do design by accident. It includes novelty, planning and systematic conception. The Twelve Principles of Green Chemistry are “design rules” to help chemists achieve the intentional goal of sustainability. Green Chemistry is characterized by careful planning of chemical synthesis and molecular design to reduce adverse consequences. Through proper design one can achieve synergies—not merely trade-offs.
The Green Chemistry approach strives to achieve sustainability at the molecular level. Because of this goal, it is not surprising it has been applied to all industry sectors. From aerospace, automobile, cosmetic, electronics, energy, household products, pharmaceutical, to agriculture, there are hundreds of examples of successful applications of award winning, economically competitive technologies.9
The concept of Green Chemistry has had this large impact due to the fact that it goes beyond the research laboratory in isolation and has touched industry, education, environment, and the general public. The field of Green Chemistry has demonstrated how chemists can design next generation products and processes so that they are profitable while being good for human health and the environment. Following the scientific enthusiasm of Green Chemistry, teaching initiatives, governmental funding, and the establishment of Green Chemistry Research Centers have multiplied in the past two decades. Many universities now offer classes on Green Chemistry and Green Engineering. Some institutions offer degrees in the field. Governmental funding has also increased in several countries around the world.10
II Framework of Green Chemistry
The three main points about the Green Chemistry framework can be summarized as:
1. Green Chemistry designs across all stages of the chemical life-cycle.
2. Green Chemistry seeks to design the inherent nature of the chemical products and processes to reduce their intrinsic hazard.
3. Green Chemistry works as a cohesive system of principles or design criteria.
The Twelve Principles of Green Chemistry are design criteria or guidelines that provide the framework for sustainable design. They constitute an overarching construct for the design of safer chemicals and chemical transformations. Chemistry has long been perceived as a dangerous science and often the public associates the word “chemical” with “toxic.” There are ways to reduce risk by using safety precautions such as protective gear. When safety protections fail, the risk, which is defined as a function of the hazard and exposure, increases (Fig. 1). If the hazard is high and exposure controls fail, the consequences can be catastrophic (injury or death). By minimizing the hazard portion of the equation instead of focusing only on exposure controls, the risk can be limited even in cases of undesirable circumstances (accident, spills, sabotage, etc…). Designing safer sustainable chemicals and processes requires striving to reduce the intrinsic hazards to a minimum and therefore limiting the risk of accident and damage.1
 |
| Fig. 1 Risk is a function of hazard and exposure. | |
The aim of Green Chemistry, to reduce hazards across all the life-cycle stages, has been shown to be economically profitable. Hazard is defined as the ability to cause adverse consequence to humans or the environment. Intrinsic hazard of a chemical substance or a chemical process can be designed to be minimized at every level of a process, whether it is toxicity, physical hazards (e.g., explosion, flammability) or global hazards such as stratospheric ozone depletion. Risks based on these hazards may rise from the nature of the feedstock and raw materials that are used in the chemical transformations as well as the final products that are made. Careful design will reduce or eliminate intrinsic hazards within chemicals and processes; a design based on the integration of the Twelve Principles as one cohesive set.
III The Twelve Principles
The Twelve Principles of Green Chemistry were introduced in 1998 by Paul Anastas and John Warner (Fig. 2). They are a guiding framework for the design of new chemical products and processes, applying to all aspects of the process life-cycle from the raw materials used to the efficiency and safety of the transformation, the toxicity and biodegradability of products and reagents used. They were summarized recently into the more convenient and memorable acronym, PRODUCTIVELY.11 The following sections are intended to provide a general knowledge about Green Chemistry. For the convenience of the reader, technical examples are cited for each principle.
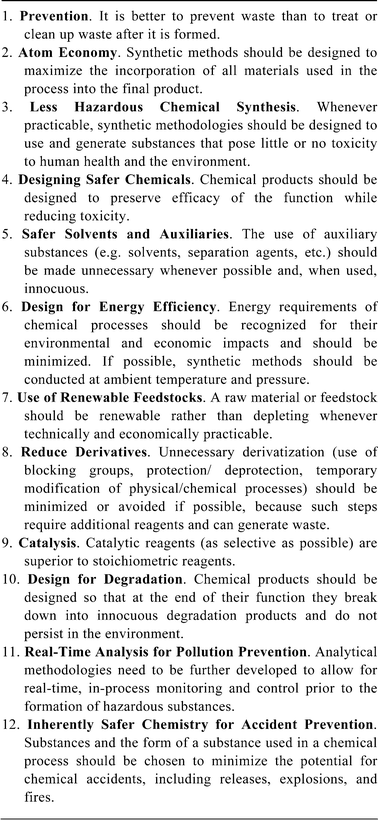 |
| Fig. 2 The Twelve Principles of Green Chemistry. | |
1 Waste
Waste prevention is the first of the Twelve Principles of Green Chemistry. It is better to prevent the formation of waste rather than to clean it up after the fact. The generation of any material that does not have realized value or the loss of unutilized energy can be considered a waste. As mentioned above, waste can take many forms and may impact the environment differently depending on its nature, its toxicity, its quantity, or the way it is released.12 When large portions of the initial raw materials used in a process are lost because of the original design of the process itself then it will inexorably generate waste which is by definition undesirable.
In 1992, the concept of what is now widely accepted as the E-Factor, or Environmental Impact Factor, was introduced by Roger Sheldon.13 This metric helps to quantify the amount of waste generated per kilogram of product. It is a means to assess the “environmental acceptability” of a manufacturing process.
The environmental factor which has been adopted by many in the chemical industry underscores how inefficient certain industrial processes have been and opened the door to creative solutions. One well-known example is the early synthesis of ethylene oxide which was prepared through a chlorohydrin intermediate (Fig. 3a). The E-Factor for the entire synthesis as stated above was equal to 5. For each kilogram of product, 5 Kg of waste were to be disposed. This does not take into consideration the waste water contaminated by chlorine by-products.12–14 When the synthesis was modified to use molecular oxygen, thus removing the need for chlorine, the E-Factor dropped to 0.3 Kg of waste. The new process was generating more than 16 times less waste than the original one, eliminating the formation of waste water as well.15
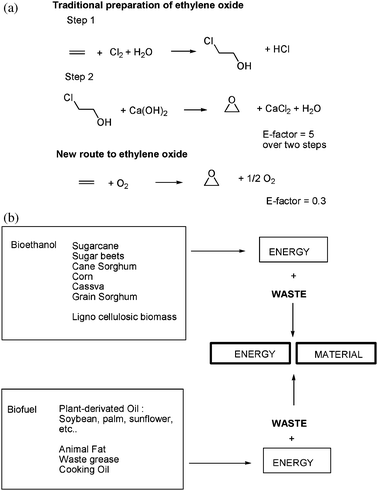 |
| Fig. 3 (a) Traditional preparation of ethylene oxide and the new route relying on molecular oxygen. (b) Revalorization of biofuel byproducts. | |
When byproducts cannot be avoided, other innovative solutions should be considered and a productive one is to seek an industrial ecology approach where waste can become a new raw material with significant value for another process as it re-enters the life-cycle. This approach is currently being applied to the production of biofuel (Fig. 3b).16
2 Atom economy
In 1990 Barry Trost introduced the concept of synthetic efficiency: Atom Economy (AE) also called Atom Efficiency.17 It refers to the concept of maximizing the use of raw materials so that the final product contains the maximum number of atoms from the reactants. The ideal reaction would incorporate all of the atoms of the reactants. The AE is measured as the ratio of the molecular weight of the desired product over the molecular weights of all reactants used in the reaction (Fig. 4). It is a theoretical value meant to quickly assess how efficient a reaction will be.
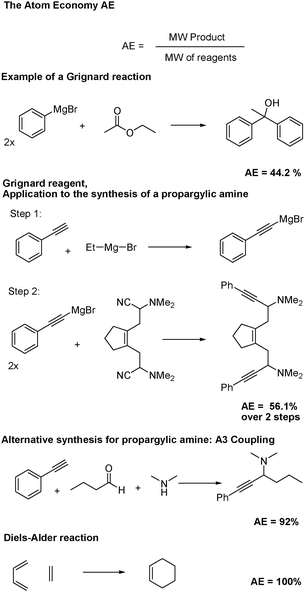 |
| Fig. 4 The Atom Economy AE and several illustrations. | |
To illustrate this concept, a few examples such as the Grignard reaction, A3 coupling and the Diels–Alder reaction are presented below. The Grignard reaction, which received the recognition of the scientific community for its importance in organic synthesis, is unfortunately a relatively poor atom-economical reaction due to the use of a stoichiometric amount of metal reactant and the necessity to prepare the Grignard reagent separately.18Fig. 4 presents a typical Grignard reaction19 and an application of the Grignard reagent to build a propargylic amine type structure.20 The values of the AE respectively are 44 and 56% which reflect a loss of half of the raw material. A solution in respect to the last example was proposed by C.-J. Li et al. in 2002 through the A3 coupling (Alkyne, Aldehyde and Amine).21 This one-step multicomponent coupling reaction is more efficient and conserves atoms since 92% of the original atoms used are found in the final product. The Diels–Alder reaction is also an excellent example of an atom-economical reaction17 (Fig. 4). Its AE is equal to 100% since all atoms from the reactants are incorporated into the final product. Diels–Alder type reactions belong to the category of cycloaddition which is among the greenest types of reactions in traditional chemistry.
3 Synthesis
As illustrated in Fig. 5, the synthetic toolbox of organic chemists has been improved by a significant amount of innovative work. Many of the new reactions that have been developed in the past decade add to the already existing green reactions that were discovered during the past century. Reactions based on cycloaddition,17,22 rearrangement,23 or multi-component coupling reactions24 were already known and constitute one category of efficient reactions. Cascade or tandem reactions,25 C–H activation,26 metathesis,27 and enzymatic reactions28,29 are rather new approaches and illustrate strong examples of cleaner, more efficient synthetic tools available to organic chemists. The Grubbs catalyst, for example, allows alkene metathesis through a mechanism similar to Wittig-type reactions such as the Horner–Wadsworth–Emmons reaction (formation of a four membered ring as reaction intermediate, Fig. 5). It is an essential tool for the construction of larger molecules. However, unlike the Wittig reaction, the metathesis reaction does not produce a large amount of waste. The formation of phosphonium salts in the case of the Wittig reaction is unfortunately unavoidable since it is part of the design of the reaction and is the main driving force.30
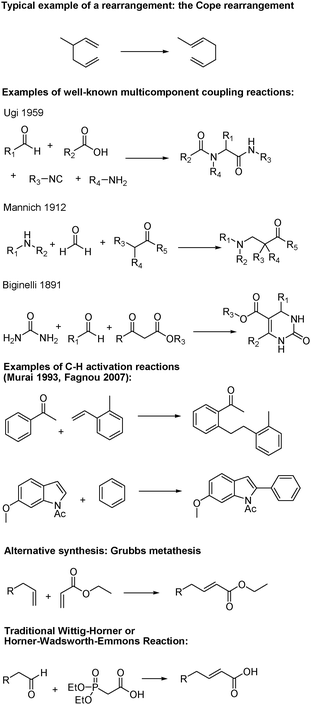 |
| Fig. 5 Examples of green reactions. | |
C–H activation is another relatively new area of chemistry which holds great promise for the future.26 In traditional coupling reactions, activated carbon–halogen bonds are usually used because of their high reactivity. Since halogenated molecules are rarely natural, it implies additional steps to produce the precursor. The replacement of traditional coupling reactions with C–H activation eliminates the need for halogenated precursors and therefore the halogenated waste byproduct generated.
Two famous examples of C–H activation were published in 1993 by Murai31 and in 2007 by Fagnou.32 In the first case, Murai et al. employed a ruthenium catalyst to couple the inactivated substrates acetophenone and 2-methylstyrene. This work was one of the first examples of C–H activation and represents a milestone in the field. In the second case, Fagnou and Stuart coupled two aromatic compounds selectively without the need for any activating or directing groups. Those examples demonstrate the power of C–H activation in advancing Green Chemistry.
4 Molecular design
While there has been significant focus on designing chemicals for various functions ranging from medicines to materials, there has been a surprising lack of interest in taking into consideration hazard in the design process. Understanding the properties of a molecule that have an impact on the environment and the transformations that take place in the biosphere is essential to sustainability. Through a mastery of this understanding, chemistry will be able to genuinely design molecules that are safer for humans and the environment. Work by Ariëns33 in 1984 and by Garrett and Devito in 1996 showed that designing safer chemicals is not only highly needed for the advancement of Green Chemistry, but is also possible.34
In recent decades, there has been a significant amount of work in the field of toxicology that has moved it from being a descriptive science to one that has a large mechanistic component,35 and even more recently progressively towards the incorporation of an in-silico component.36 Because of that transition, it has been possible to create correlations, equations, and models that relate structure, properties, and function. These approaches provide the basis for the work being pursued in the development of a comprehensive design strategy. For instance the existing understanding of medicinal chemistry can already help establish some ground rules for designing less toxic chemicals via incorporation of specific design features that block their access into humans and many animal organisms.37
Solvents are perhaps the most active area of Green Chemistry research.38 They represent an important challenge for Green Chemistry because they often account for the vast majority of mass wasted in syntheses and processes.39 Moreover, many conventional solvents are toxic, flammable, and/or corrosive. Their volatility and solubility have contributed to air, water and land pollution, have increased the risk of workers’ exposure, and have led to serious accidents. Recovery and reuse, when possible, is often associated with energy-intensive distillation and sometimes cross contamination. In an effort to address all those shortcomings, chemists started a search for safer solutions. Solventless systems,40 water,41 supercritical fluids42 (SCF) and more recently ionic liquids43 are some examples of those new “green” answers.
Where possible, the ideal situation would be to not use any solvent because the decision to include an auxiliary always implies efforts and energy to remove it from a designated system. Efforts have therefore been devoted to developing solventless systems.40 This idea was reinforced by the finding that solvents account for most of the industrial waste.39 Depending on the physical properties of the reagents used or the desired outcome of the transformation, the approach often requires a new or redesigned chemistry to allow the reaction to proceed without the original solvent.
Water is the most abundant molecule on the planet and is sometimes referred to as a benign “universal solvent.”41 Being able to run a reaction in or on water therefore has significant advantages. Water is safe and does not pose any hazards. It can be a useful solvent for large scale process chemistry. The properties of water have even led to improved reaction rates through the hydrophobic effect44 and easier separation since a lot of organic substances do not dissolve in water. The case of an improved Diels–Alder reaction in water is one of the useful examples illustrating the advantages of water as a solvent.17,41 One drawback which may slow down industrial applications and has yet to be addressed is the risk of water contamination that can be very energy intensive to clean.
SCF are another alternative to traditional organic solvents and have been extensively studied in the past decades.42 They are substances which have been simultaneously heated and compressed above their critical points (see Fig. 6).
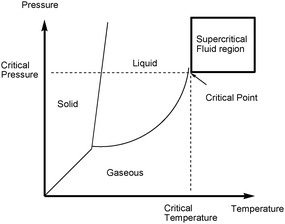 |
| Fig. 6 Pressure–temperature phase diagram showing the supercritical region. | |
Common SCF are generated from water, carbon dioxide, methane, methanol, ethanol or acetone. Carbon dioxide is one of the most widely used SCF. The resulting scCO2 has proven to be a versatile solvent, safe and easy to handle, as demonstrated by the work of Poliakoff, Leitner, Jessop, DeSimone, and others.45 What makes SCF so attractive in general and particularly scCO2 is the change of state that occurs when cooling down the vessel or reducing the pressure. Above critical points, CO2 will be a liquid in which reactions can be performed and below, it will be a gas. Degassing the system allows the complete removal of the solvent. ScCO2 has found a wide range of industrial applications with the most famous being the decaffeination of green coffee beans and the replacement of perchloroethylene in dry cleaning.46 Supercritical fluids have proven to be one valuable alternative to traditional solvents.
Another example of greener solvents would be ionic liquids pioneered in modern times by Seddon.43 As their name highlights, ionic liquids, or sometimes called room temperature ionic liquids, are liquid salts at room temperature. They have virtually no vapor pressure and very low flammability. What was discovered recently by Jessop et al. is a “switchable” ionic liquid.47 A “smart-obedient solvent” generated in situ just like the liquid scCO2. Addition of pressurized carbon dioxide into an organic mixture transforms it into an ionic liquid, generating a safer solvent in situ. Releasing the pressure reverses the phenomenon and the ionic liquid is retransformed into the original mixture, thus removing completely the solvent and eliminating tedious purification and extraction steps.
Another example based on the same concept is the development of fluorous biphasic catalysis advanced by Horvath.48 A fluorous phase or solvent containing a catalyst suited for the desired transformation is usually not miscible with organic reagents at ambient temperature. When heated, they form a single media, allowing the reaction to proceed. Upon cooling the organic phase and the fluorous solvent separate, simplifying the purification process. This is an attractive approach with the restriction that fluorous solvents are expensive.
These last examples are good illustrations of one of chemistry’s major challenges: separation. Apart from certain solventless systems, the new improved green solvents remain auxiliaries and therefore must be isolated from the desired product. If their use cannot be avoided then the issue of separation must be taken into consideration when choosing the appropriate solvent.
6 Energy
Rising concerns over the depletion of petroleum feedstocks and the increase in energy consumption have pushed the development of more energy efficient processes and for the search for renewable energies; non-depleting resources in a time frame relevant to human scale.49
As mentioned in the first section (first principle), unutilized energy may also be considered a waste. The design of chemical reactions or systems that do not require intensive energy use is highly desirable. Reducing the energy barrier of a chemical reaction or choosing appropriate reactants so that the transformation may proceed at room temperature is one example of what chemists can do to reduce energetic requirements, with all the direct and indirect benefits associated with it.1
Increasing the energy efficiency of a chemical system is merely one part of the solution. Alternative energies are also needed. Several of those renewable energies have been identified in biofuels production,49,50 solar power (thermal and photovoltaic),49,51–53 wind power, hydro power, geothermal energy,49 and hydrogen fuel cells.49,54 Once again, green chemists have an important role to play in this new challenge as they have the ability to design both energy efficient transformations and materials or chemical systems that can be used to harvest some of those renewable natural energies.
Solar energy, the primary sustainable energy source on earth, is one of those alternatives to petroleum. Considerable efforts have been dedicated to understand and design chemical systems that can convert solar radiations into voltaic energy.53,55 Organic, inorganic and hybrid solar cells have received interest although more focus has been placed on organic solar cells because of their higher efficiency. The principle of those cells relies on the ability of the material used to absorb photonic energy from solar radiations. The absorption leads to the formation of excited states that can be relayed and generate electronic current. Building materials and polymers that can efficiently transform light into current remain a challenge and are key to the success of this approach.
Proton Exchange Membrane (PEM) fuel cells using hydrogen and oxygen gases could also provide another solution to the upcoming increase in energy demand (Fig. 7).54,56 PEM fuel cells have generated research interest, especially in the past decade with the development of increasingly efficient catalysts such as nanoparticles or even hydrogenase enzymes.57 An important consideration in this approach is the hazard of handling hydrogen gas, which is highly flammable and explosive.
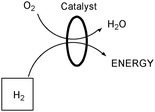 |
| Fig. 7 General concept of a hydrogen fuel cell. | |
7 Renewable materials
It has been estimated that the vast majority of our manufacturing products are derived from petroleum feedstock or natural gas.1,49,58 The depletion of those resources will touch many aspects of our consumer life and our economy. Turning towards renewable feedstocks both for material and fuel has now become more urgent. The major renewable feedstock on the planet both for material and energy is bio-mass,58 the material available from living organisms. This includes wood, crops, agricultural residues, food, etc.59
Examples of renewable material include cellulose, lignin, suberin and other wood compounds, polyhydroxyalkanoates, lactic acid, chitin, starch, glycerol and oil.60 Lignin, for instance, is a major waste of the pulp and paper industry. It has been burned on the production site to provide energy for many years. In recent years it has found new applications as, for example, dispersants, additives, and raw materials for the production of chemicals such as vanilin, DMSO or humic acid.61 Chitin is another abundant natural polymer that constitutes the exoskeleton of arthropods (e.g. crustaceans). It is a major byproduct of the seafood industry and can be transformed into chitosan by deacetylation.62 Numerous applications of chitosan have been described from water purification, biomedical applications and other industrial uses.62,63 Reusing this waste of the bio-industries should provide a large amount of raw materials to replace the current petroleum feedstocks.
8 Derivatives
Covalent derivatization is a ubiquitous technique in chemistry whether it is employed for organic synthesis or analytical chemistry.64 In the early 1990s, an innovative concept surfaced called non-covalent derivatization, a derivatization that does not rely on covalent bonding but rather on intermolecular interactions.65 The work by Warner was developed as a means to use little energy and less material to achieve chemical modifications from the original system. An early example of non-covalent derivatization is illustrated by the controlled diffusion and solubility of hydroquinones used in Polaroid films.66 Researchers at Polaroid sought to release hydroquinones at elevated pH. Instead of relying on base-labile covalent protecting groups which would be the traditional approach, they developed a non-covalent protecting group in the form of a co-crystal between hydroquinones and bis-(N,N-dialkyl)terephthalamides (Fig. 8). This approach was successful and viable for the industrial process. It solved the problem without modification of the original hydroquinone structures and minimized waste material and energy.
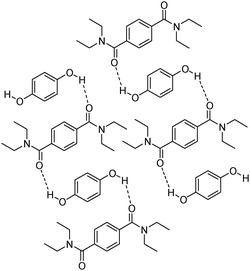 |
| Fig. 8 Hydroquinones protected by non-covalent interactions with bis-(N,N-dialkyl)terephthalamides. | |
9 Catalysis
In many cases, the formation of waste is linked to the traditional use of a stoichiometric amount of reagents.67 Switching from stoichiometric methodologies to catalytic processes is perceived as one major way to improve the efficiency of the synthetic toolbox. Catalysis can improve the efficiency of a reaction by lowering the energy input required, by avoiding the use of stoichiometric amount of reagents, and by greater product selectivity. This implies less energy, less feedstock and less waste.68 Moreover, it often opens the door to innovative chemical reactions and bring unconventional solutions to traditional chemical challenges.
Oxidation and reduction reactions illustrate this concept. Reduction employing DIBAL-H as the hydride donor is a well-established procedure used by organic chemists.69 It generates a significant amount of waste since a stoichiometric amount of reducing agent is needed to complete the reaction. Switching to catalytic hydrogenation like the Noyori hydrogenation70 eliminates the need for stoichiometric reagents and in consequence decreases the amount of feedstock needed and the amount of waste generated (Fig. 9).
Beyond efficiency, catalysis can allow for otherwise unfavorable reactions to be realized. This was the case for the metathesis reaction and the development of the Grubbs catalyst.27 As illustrated in Fig. 5, the development of a metathesis catalyst allowed a groundbreaking approach to the formation of unsaturated compounds. The environmental benefit was significant and the innovation was important.
Biocatalysis is yet another example of “green” chemistry as it is a biomimetic approach relying on natural or modified enzymes.28–29,71 It usually refers both to the direct use of purified enzymes and the transformations accomplished by engineered living organisms. Reaction conditions are relatively mild as the transformation can be performed in water at atmospheric pressure and ambient temperature. Moreover, enzymes have proven to be more chemo-, regio- and stereoselective.
10 Biodegradation
The problem of persistence has been known for a long time and became apparent in the early stages of industrial development.72,73 In the 1950s for instance, tetrapropylene alkylbenzene sulfonate (TPPS) was used as a surfactant for laundry detergents and accumulated into the water supply due to an incomplete degradation. The situation was so critical that there were examples where “water tended to foam when coming out of the tap.”73,74 The public outcry prompted the industry to seek an immediate solution and it was found that replacing the methyl branched chain of TPPS by a linear carbon chain reduces the biopersistence (Fig. 10). A common example is the replacement of TPPS by linear alkylbenzene sulfonate (LAS).
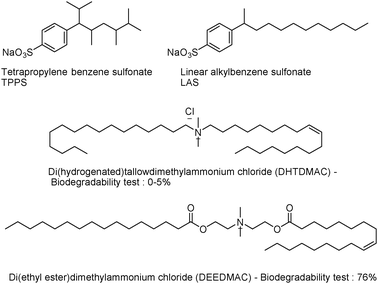 |
| Fig. 10 Integrating biodegradability in the design of surfactants. | |
Designing biodegradable materials and chemicals is not a simple task as illustrated by continuing problems of environmental pollution. Trends have emerged following decades of data collection. Certain chemical structures such as halogenated moieties, branched chains, quaternary carbons, tertiary amines, and certain heterocycles may possess enhanced persistence and are avoided. On the other hand, integrating functional groups such as esters or amides which are recognized by ubiquitous enzymes may help the design of environmental degradable products.73,75 This strategy was applied to surface-active quaternary ammonium compounds used as household fabric softeners.76 Until the 1990s, long chain ammonium salts such as di(hydrogenated)tallow dimethyl ammonium chloride (DHTDMAC) were released into the environment. It was then discovered that their rate of biodegradation in aqueous sediment was low and the intrinsic ecotoxicity, high. In response, hydrolyzable amide or ester linkages were introduced. The new ammonium salts have proven to be more biodegradable as illustrated in Fig. 10; DHTDMAC was replaced by di(ethyl-ester) dimethyl ammonium chloride (DEEDMAC) which was followed by a 70% increase in the biodegradability.
11 Analysis
It is the goal of green analytical chemistry to measure chemicals without generating waste.77–79 The environmental issues associated with analytical chemistry are usually linked to the analytical approach itself. Real time direct analysis is unfortunately not systematic as many methodologies still require a pre-treatment of the sample or rely on what one may call “ex situ analysis.” Process analytical chemistry79 is defined as the ability to monitor a transformation and act immediately upon it to prevent unwanted outcomes. It is not always possible, and therefore waste may be generated when the sample is analyzed. Green chemists must take into consideration the functional requirements of analytical methods since it will be counterproductive if the chosen methods contribute to further environmental problems. Green analytical chemistry can be defined as the use of analytical procedures that generate less waste and are safer to human health and the environment.79 This definition includes both aspects of “live” monitoring of a chemical transformation and the environmental shortcomings associated with traditional analysis. In situ monitoring of a reaction has significant advantages in terms of Green Chemistry. When action can be taken quickly, it may prevent accidents, save energy, and/or prevent the formation of significant amounts of by-products that would otherwise require additional purifications.
When it comes to the analytical methods themselves, most of them share two problems linked to the two major steps of a method: a pretreatment of the sample which includes extraction, separation or even sometimes chemical modification of the sample, and a signal acquisition step.79 Since the pretreatment step usually calls for large volumes of solvent, it has been the center of analytical chemists concerns. If the use of solvents can not be avoided for an extraction step, benign alternatives such as Accelerated Solvent Extraction (ASE) or SCF extraction should be considered.79,80
Material used in the manufacture of analytical apparatus should be taken into consideration. Both green chemists and green engineers building new sensors should be aware of the toxicity and any potential environmental problems related to the material they handle. Mercury electrodes, for example, are often used for electrochemistry. Replacing them with carbon-based electrodes such as nanotubes or nanofibers has proven to be an effective solution.81
12 Accident prevention
Dangerous substances and processes have multiplied in our working environment. According to the “Chemical accident prevention and the clean air act amendments of 1990,” preventing accidents starts by identifying and assessing the hazards.82 All types of hazards whether it is toxicity, physical hazards such as explosivity or flammability, and global hazards should be addressed in the design of chemicals and processes in order to prevent accidents such as Bhopal or the Love Canal incident.83
A recent and shocking illustration of these dangers and hazards can be found in the UCLA accident that occurred in January 2009.84 Handling of the very common and highly flammable butyllithium reagent resulted unfortunately in a terrible outcome with the death of the research assistant involved. This accident should be a strong reminder to the scientific community that many chemicals we still use present serious hazards and should be replaced by safer alternatives to prevent accidents wherever possible.
IV Accomplishments by industry
There are numerous examples of successful industrial changes using Green Chemistry.85 The following section is not intended to present an exhaustive list of the awards winners but rather introduce a few key examples to show how the industry has been adapting to the new challenges of Green Chemistry.
As a first example, a greener synthetic pathway which was attributed to Eastman for its enzymatic esterifications.85 This biocatalytic process runs under mild conditions, minimizes the formation of byproducts and saves energy, resulting in increased efficiency. Overall hundreds of litres of organic solvents were eliminated from the previous process.
In 2008, researchers at Dow AgroSciences were rewarded for the design of green pesticides. While trying to understand the structure–activity relationships of natural biopesticides in an effort to predict analogues that would be more active, they designed Spinetoram. The company expects that the production of this new pesticide will eliminate “about 1.8 million pounds of organophosphate insecticides during its first five years of use.”86
In 2006, Merck developed a greener synthetic pathway for Sitagliptin, a chiral β-amino acid derivative used for the treatment of type 2 diabetes.87 The approach was based on a novel asymmetric catalytic hydrogenation of unprotected enamines which avoided the need of excessive derivatization (Fig. 11).88 Merck presented a three-step synthesis, claiming an increase in the overall yield. Implementing the new route on a manufacturing scale showed a significant reduction in the amount of waste, making the new process a more cost-effective option.
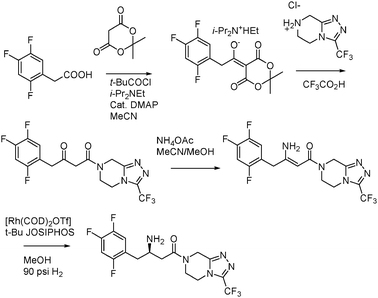 |
| Fig. 11 New synthesis of Sitagliptin. | |
In 2004, BMS developed a new approach to Paclitaxel, the active ingredient in the anticancer drug Taxol®.89 Paclitaxel is commercially produced from the naturally occurring precursor 10-deacetylbaccatin III (10-DAB) through an 11 step synthesis. This “semisynthetic route”, which was first developed as an economically viable approach to the molecule, was not without certain environmental concerns. A more sustainable process was therefore investigated by BMS using the latest biotechnological advances. Instead of synthesizing Paclitaxel from a precursor, the active compound was extracted directly from plant cell cultures. This method eliminated all organic solvents, hazardous reagents, and additional steps associated with the previous process. BMS is now manufacturing Paclitaxel using only plant cell cultures.
In 2002, Pfizer developed a new greener synthetic pathway for the redesigned synthesis of sertraline, an active ingredient used to treat depression (Fig. 12).90 The new process offered substantial environmental benefits while improving the overall efficiency and selectivity of the synthesis. Specifically, a three-step sequence in the original manufacturing process was streamlined to a single one.91 Raw material use was significantly cut and the process was optimized so that all steps could be performed in ethanol. This last change eliminated the need to use, distill, and recover four toxic solvents (methylene chloride, tetrahydrofuran, toluene, and hexane).
One final noteworthy example is the work accomplished in 1998 by Solutia, Inc. on the elimination of chlorine from the synthesis of 4-aminodiphenylamine (Fig. 13).92 Researchers at the company explored new routes to a variety of aromatic amines, including 4-aminodiphenylamine (4-ADPA), in order to eliminate the formation of aqueous waste streams containing high levels of inorganic salts. There were also concerns about the hazard associated with storage and handling of large quantities of chlorine gas. The solution came with a new synthesis to 4-ADPA that utilizes the base-promoted, direct coupling of aniline and nitrobenzene.93 The environmental benefits were significant and included a dramatic reduction in waste generated.
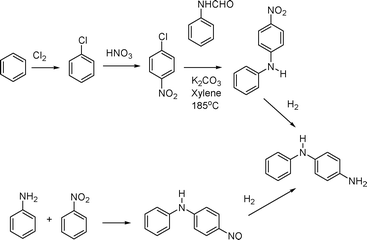 |
| Fig. 13 Differences between the chlorine-based and chlorine-free processes for the preparation of 4-ADPA. | |
V Future challenges
The accomplishments in the field of Green Chemistry thus far are impressive due to the scientists in academia, industry, and research institutes around the world. However, the accomplishments achieved thus far are a prelude to the grand challenges still to be addressed by the field. A few notable challenges are mentioned below.
Twelve Principles as a cohesive system
The design framework of the Twelve Principles of Green Chemistry has been a template for many advances in the field, however, the Twelve Principles were not meant to be twelve independent goals but rather an integrated cohesive system of design. Only through the applications of all principles can one hope to achieve a truly sustainable process. By seeking out the mutually reinforcing aspects of the principles, systemic sustainable design is possible and can facilitate transformative innovation rather than incremental improvement.
Catalysis has made significant progress during the past two decades. However, even today, most catalysts are designed to act on one transformation only and little is known about multi-functional catalysts, defined as the ability of one catalytic system to act on a series of transformations. If the same catalyst could be used for various independent reactions or achieve an entire synthesis in one pot, it will bring chemistry to a new level as more complex molecules could be made with higher material and energy efficiency.
Mastering weak forces for synthesis and properties
Non-covalent and weak-force interactions are likely to play an increasingly important role in the future of chemistry. Imparting properties through weak-forces and guiding synthetic pathways in the same manner while minimizing the amount of bond breaking and bond forming can result in significant advantages. These include reducing the amount of energy needed, suppressing the amount of waste, and an increase in efficiencies. Mastering the weak forces in the same way that the field of chemistry has mastered covalent forces holds great potential to help in reaching sustainability at the molecular level.
Integrative systems thinking
The traditional approach to scientific investigation has been largely based on the reductionist approach. This approach has brought about a depth of understanding and discovery that have made the things of modern life—from communication to transportation to medicine—possible. It has also resulted in tremendous unintended and unforeseen consequences that have had a damaging impact on humans and the environment. By thinking in terms of systems, Green Chemistry can pursue significant innovations while avoiding unintended results. Coupling reductive and integrated thinking can result in truly transformative innovations.
Conclusion
For generations, molecular scientists have invented the molecules, materials, and manufacturing processes that have allowed economic and societal development. Green Chemistry is ensuring that all of that creative ability that is the long tradition of the field of chemistry is practised in a way that builds in impact on people and the planet as a design criterion. In doing so, Green Chemistry has shown that through innovation companies can be economically more profitable and more environmental benign at the same time. Although an impressive amount of work has been done by practitioners of Green Chemistry around the world, the achievements of the past pale by comparison to the power and potential of the field.
Notes and references
-
P. T. Anastas and J. C. Warner, in Green Chemistry: Theory and Practice, Oxford University Press, New York, 1998 Search PubMed; I. Horvath and P. T. Anastas, Chem. Rev., 2007, 107, 2167 Search PubMed.
-
P. T. Anastas and T. C. Williamson, in Green Chemistry: Designing Chemistry for the Environment, American Chemical Series Books, Washington, DC, 1996, pp. 1–20 Search PubMed.
-
T. J. Collins, in Green Chemistry, Macmillan Encyclopedia of Chemistry, Simon and SchusterMacmillan, New York, 1997, vol. 2, pp. 691–697 Search PubMed.
- P. T. Anastas, Green Chem., 2003, 5, 29 Search PubMed.
- W. McDonough, M. Braungart, P. T. Anastas and J. B. Zimmerman, Environ. Sci. Technol., 2003, 37, 434A CAS.
- Office of Pollution Prevention and Toxics, The Presidential Green Chemistry Challenge Awards Program, Summary of 1996 Award Entries and Recipients, US Environmental Protection Agency, Washington, DC, EPA744K96001, 1996 Search PubMed.
- Forum, Green Chem., 1999, 1, G11 Search PubMed.
- J. Clark, Green Chem., 1999, 1, G1 RSC.
- Office of Pollution Prevention and Toxics, The Presidential Green Chemistry Challenge, Award Recipients, 1996–2009, US Environmental Protection Agency, Washington, DC, EPA 744K09002, 2009 Search PubMed.
- S2669, Green Chemistry Research and Development Act of 2008, 2008.
- S. L. Y. Tang, R. L. Smith and M. Poliakoff, Green Chem., 2005, 7, 761 RSC; S. Tang, R. Bourne, R. Smith and M. Poliakoff, Green Chem., 2008, 10, 268 RSC.
- R. A. Sheldon, Chem. Commun., 2008, 3352 RSC.
- R. A. Sheldon, Green Chem., 2007, 9, 1273 RSC.
- P. P. McClellan, Ind. Eng. Chem., 1950, 42, 2402 CrossRef CAS.
- P. A. Kilty and W. M. H. Sachtler, Catal. Rev., 1974, 10, 1 CrossRef CAS.
- S. K. Khanal, M. Rasmussen, P. Shrestha, H. V. Leeuwen, C. Visvanathan and H. Liu, Water Environ. Res., 2008, 80, 1625 CrossRef CAS;
G. Centi and R. A. Van Santen, in Catalysis for Renewables, From Feedstocks to Energy Production, Wiley-VCH Verlag GmbH & Co., KGaA, Weinheim, 2007 Search PubMed;
F. Cavani, G. Centi, S. Perathoner and F. Trifirò, in Sustainable Industrial Chemistry, Wiley-VCH Verlag GmbH & Co., KGaA, Weinheim, 2009 Search PubMed.
- B. M. Trost, Science, 1991, 254, 1471 CAS; B. M. Trost, Angew. Chem., Int. Ed. Engl., 1995, 34, 259 CrossRef CAS.
-
M. B. Smith and J. March, in March’s Advanced Organic Chemistry: Reactions Mechanisms and Structure, John Wiley & Sons Inc., New York, 5th edn, 2001, pp. 1205–1209 Search PubMed.
- X. Creary, J. Org. Chem., 1987, 52, 5026 CrossRef CAS.
- R. Epsztein and N. Le Goff, Tetrahedron, 1985, 41, 5347 CrossRef CAS.
- C. M. Wei and C.-J. Li, Green Chem., 2002, 4, 39 RSC; C. Wei, Z. Li and C.-J. Li, Synlett, 2004, 1472 CAS.
-
S. Kobayashi and K. A. Jorgensen, in Cycloaddition Reactions in Organic Synthesis, Wiley-VCH Verlag GmbH, Weinheim, 2002 Search PubMed;
N. Dennis, in Organic Reaction Mechanisms, Addition Reactions: Cycloaddition, John Wiley & Sons Ltd., West Sussex, 2008, ch. 12, p. 349 Search PubMed; U. Chiacchio, A. Padwa and G. Romeo, Curr. Org. Chem., 2009, 13, 422 Search PubMed.
-
M. B. Smith and J. March, in March’s Advanced Organic Chemistry: Reactions, Mechanisms, and Structure, John Wiley & Sons, Inc., New York, 5th edn, 2001, ch. 18, pp. 1377–1505 Search PubMed;
K. Banert and H. Hahn, in Organic Reaction Mechanisms, Molecular Rearrangement: Part 1, John Wiley & Sons Ltd, West Sussex, 2008, ch. 13, p. 451 Search PubMed;
A. Brandi and F. Pisaneschi, in Organic Reaction Mechanisms, Molecular Rearrangement: Part 2, John Wiley & Sons Ltd, West Sussex, 2008, ch. 14, p. 493 Search PubMed; L. R. Overman, Tetrahedron, 2009, 65, 6432 Search PubMed.
-
J. Zhu and H. Bienaymé, in Multicomponent Reactions, Wiley-VCH Verlag GmbH & Co. KGaA, Weinheim, 2005 Search PubMed; B. B. Touré and D. G. Hall, Chem. Rev., 2009, 109, 4439 Search PubMed; A. Dömling, Chem. Rev., 2006, 106, 17 CrossRef CAS; A. J. von Wangelin, H. Neumann, D. Gördes, S. Klaus, D. Strübing and M. Beller, Chem.–Eur. J., 2003, 9, 4286 CrossRef.
- K. C. Nicolaou, T. Montagnon and S. A. Snyder, Chem. Commun., 2003, 551 RSC ; For cascade reactions see: K. C. Nicolaou, D. J. Edmonds and P. G. Bulger, Angew. Chem., Int. Ed., 2006, 45, 7134 Search PubMed ; For tandem reactions, see: P. J. Parsons, C. S. Penkett and A. J. Shell, Chem. Rev., 1996, 96, 195 CrossRef CAS; A. Padwa, Pure Appl. Chem., 2004, 76, 1933 Search PubMed.
-
S. Murai, in Activation of Unreactive Bonds and Organic Synthesis, Topics in Organometallic Chemistry, Springer-Verlag, Berlin Heidelberg, 1999, vol. 3 Search PubMed;
K. Goldberg and A. S. Goldman, in Activation and Functionalization of C–H Bonds, ACS Symposium Series, Oxford University Press, 2004 Search PubMed; Y. Fujiwara and C. Jia, Pure Appl. Chem., 2001, 73, 319 Search PubMed; J. A. Labinger and J. E. Bercaw, Nature, 2002, 417, 507 Search PubMed; R. G. Bergman, Nature, 2007, 446, 391 CrossRef CAS; C. I. Herrerias, X. Yao, Z. Li and C.-J. Li, Chem. Rev., 2007, 107, 2546 CrossRef CAS.
- R. H. Grubbs, Tetrahedron, 2004, 60, 7117 CrossRef CAS.
-
R. B. Silverman, in The Organic Chemistry of Enzyme-Catalyzed Reactions, Academic Press, New York, 2002 Search PubMed;
A. S. Bommarius and B. R. Riebel, in Biocatalysis, Wiley-VCH Verlag GmbH & Co. KGaA, 2004 Search PubMed.
-
P. Anastas and R. Crabtree, in Handbook of Green Chemistry—Green Catalysis: Biocatalysis, Wiley-VCH Verlag GmbH, New York, 2009, vol. 3 Search PubMed.
-
M. B. Smith and J. March, in March’s Advanced Organic Chemistry: Reactions Mechanisms and Structure, John Wiley & Sons, Inc., New York, 5th edn, 2001, pp. 1231–1237 Search PubMed.
- S. Murai, F. Kakiuchi, S. Sekine, Y. Tanaka, A. Kamatani, M. Sonoda and N. Chatani, Nature, 1993, 366, 529 CrossRef CAS.
- D. R. Stuart and K. Fagnou, Science, 2007, 316, 1172 CrossRef CAS.
- E. J. Ariëns, Drug Metab. Rev., 1984, 15, 425 CrossRef CAS.
-
S. C. DeVito and R. L. Garrett, in Designing Safer Chemicals: Green Chemistry for Pollution Prevention, ACS Symposium Series, Washington, DC, 1996, vol. 640 Search PubMed.
-
C. Kent, in Basics of Toxicology, Preserving the Legacy, John Wiley & Sons Inc, New York, 1998 Search PubMed.
- J. C. Dearden, J. Comput. Aided Mol. Des., 2003, 17, 119 CrossRef CAS.
-
A. M. Voutchkova, L. A. Ferris, J. B. Zimmerman and P. T. Anastas, Toward Molecular Design for Hazard Reduction – Fundamental Relationships Between Chemical Properties and Toxicity, Tetrahedron, 2009, in press Search PubMed.
-
P. T. Anastas, in Clean Solvent Alternative Media for Chemical Reactions and Processing, ACS Symposium series 819, Washington, DC, 2002, ch. 1 Search PubMed; J. M. DeSimone, Science, 2002, 297, 799 Search PubMed; R. A. Sheldon, Green Chem., 2005, 7, 267 CrossRef CAS; C.-J. Li and B. Trost, Proc. Natl. Acad. Sci. U. S. A., 2008, 105, 13197 RSC.
- A. D. Curzons, D. J. C. Constable, D. N. Mortimer and V. L. Cunningham, Green Chem., 2001, 3, 1 RSC; D. J. C. Constable, A. D. Curzons and V. L. Cunningham, Green Chem., 2002, 4, 521 RSC.
-
F. M. Kerton, in Alternative Solvents for Green Chemistry, RSC Green Chemistry Book Series, Royal Society of Chemistry, 2009, ch. 2, p. 23 Search PubMed;
K. Tanaka, in Solvent-free Organic Synthesis, Wiley-VCH Verlag GmbH & Co KGaA, Weinheim, Germany, 2003 Search PubMed; G. W. V. Cave, C. L. Raston and J. L. Scott, Chem. Commun., 2001, 2159 Search PubMed;
R. S. Varma and Y. Ju, in Green Separation Processes, Wiley-VCH Verlag GmbH & Co KGaA, Weinheim, Germany, 2005, pp. 53–87 Search PubMed.
- R. Breslow, Green Chem., 1998, 225 Search PubMed;
C.-J. Li and T.-H. Chan, in Comprehensive Organic Reactions in Aqueous Media, ed. John Wiley & Sons, Inc., Hoboken, New Jersey, 2nd edn, 2007 Search PubMed;
F. M. Kerton, in Alternative Solvents for Green Chemistry, RSC Green Chemistry Book Series, Royal Society of Chemistry, 2009, ch. 3, p. 44 Search PubMed; C.-J. Li and L. Chen, Chem. Soc. Rev., 2006, 35, 68 Search PubMed; C.-J. Li, Chem. Rev., 2005, 105, 3095 Search PubMed.
-
F. M. Kerton, in Alternative Solvents for Green Chemistry, RSC Green Chemistry Book Series, Royal Society of Chemistry, 2009, ch. 8, p. 68 Search PubMed;
Y. Arai, T. Sako and Y. Takebayashi, in Supercritical Fluids, Springer series in materials processing, Springer, New York, 2002 Search PubMed;
C. M. Gordon and W. Leitner, in Catalyst Separation Recovery and Recycling, Springer, Netherlands, 2006, ch. 8, p. 215 Search PubMed; J. R. Hyde, P. Licence, D. Carter and M. Poliakoff, Appl. Catal., A, 2001, 222, 119 Search PubMed.
- T. Welton, Chem. Rev., 1999, 99, 2071 CrossRef CAS;
M. J. Earle and K. R. Seddon, in Clean Solvents: Alternative Media for Chemical Reactions and Processing—Ionic liquids: green solvents for the future, ACS Symposium Series, American Chemical Society, 2002, vol. 819, pp. 10–25 Search PubMed;
R. D. Rogers, K. R. Seddon and S. Volkov, in Green Industrial Applications of Ionic Liquids, Kluwer Academic Publishers, Dordrecht, 2002 Search PubMed;
R. D. Rogers and K. R. Seddon, in Ionic Liquids as Green Solvents, ACS Symposium Series, American Chemical Society, 2003, p. 856 Search PubMed;
P. Wasserscheid and T. Welton, in Ionic Liquids in Synthesis, Wiley-VCH Verlag GmbH & Co. KGaA, Weinheim, 2007 Search PubMed;
N. V. Plechkova and K. R. Seddon, in Methods and Reagents for Green Chemistry - Ionic liquids: “designer” solvents for green chemistry, John Wiley & Sons Inc, Hoboken, 2007, pp. 105–130 Search PubMed; K. R. Seddon, Nat. Mater., 2003, 2, 363 Search PubMed; M. J. Earle and K. R. Seddon, Pure Appl. Chem., 2000, 72, 1391 Search PubMed; A. E. Visser, R. P. Swatloski, W. M. Reichert, H. D. Willauer, J. G. Huddleston and R. D. Rogers, NATO Science Series: II, Mathematics Physics and Chemistry, 2003, 92, 137 Search PubMed; W. L. Hough and R. D. Rogers, Bull. Chem. Soc. Jpn., 2007, 80, 2262 Search PubMed.
- R. Breslow, Acc. Chem. Res., 1991, 24, 159 CrossRef CAS.
-
W. Leitner, in Modern Solvents in Organic Synthesis, Reactions in Supercritical Carbon Dioxide, Springer-Verlag, Berlin Heidelberg, 1999, vol. 206, p. 107 Search PubMed; W. Leitner, Acc. Chem. Res., 2002, 35, 746 Search PubMed; P. G. Jessop and B. Subramaniam, Chem. Rev., 2007, 107, 2666 CrossRef CAS; F. Franck, L. Peter, V. Howdle, M. Steven and P. Martyn, Actualite Chimique, 2003, 4–5, 62 CrossRef CAS; J. L. Young and J. M. DeSimone, Pure Appl. Chem., 2000, 72, 1357 Search PubMed;
C. D. Mistele and J. M. DeSimone, in Green Chemistry, Oxford University Press, Oxford, UK, 1998, pp. 286–311 CAS.
-
J. M. DeSimone and W. Tumas, in Green Chemistry Using Liquid and Supercritical Carbon Dioxide, Green Chemistry Series, Oxford University Press, Inc., 2003 Search PubMed.
- P. G. Jessop, D. J. Heldebrant, X. Li, C. A. Eckert and C. L. Liotta, Nature, 2005, 436, 1102 CrossRef CAS.
-
C. R. Mathison and D. J. Cole-Hamilton, in Catalyst Separation Recovery and Recycling, Springer, Netherlands, 2006, ch. 6, p. 145 Search PubMed;
J. A. Gladysz, D. P. Curran and I. T. Horvath, in Handbook of Fluorous Catalysis, Wiley-VCH Verlag GmbH & Co. KGaA, Weinheim, 2004 Search PubMed.
-
M. A. Laughton, in Renewable Energy Sources, Watt Committee report 22, Elsevier Applied Science, 1990 Search PubMed;
T. B. Johansson, H. Kelly, A. K. N. Reddy and R. H. Williams, in Renewable Energy, Sources for Fuels and Electricity, Island Press, 1993 Search PubMed;
W. C. Turner, in Energy Management Handbook, The Fairmont Press, Inc., Lilburn, USA, 5th edn, 2005 Search PubMed;
F. Kreith and D. Y. Goswami, in Handbook of Energy Efficiency and Renewable Energy, CRC Press, Taylor & Francis Group, LLC, Boca Raton, USA, 2007 Search PubMed.
-
L. Olssen, in Biofuels, Advances in Biochemical Engineering/Biotechnology series, Springer-Verlag, Berlin, Heidelberg, 2007, vol. 108 Search PubMed;
D. M. Mousdale, in Biofuels, Biotechnology Chemistry and Sustainable Development, CRC Press, Taylor & Francis Group, LLC, Boca Raton, 2008 Search PubMed;
W. Soetaert and E. J. Vandamme, in Biofuels, Wiley Series in Renewable Resources, John Wiley & Sons, Inc., United Kingdom, 2008 Search PubMed;
A. Pandey, in Handbook of Plant-Based Biofuels, CRC Press, Taylor & Francis Group, LLC, Boca Raton, 2008 Search PubMed; G. W. Huber, S. Iborra and A. Corma, Chem. Rev., 2006, 106, 4044 Search PubMed.
-
R. Foster, M. Ghassemi and A. Cota, in Solar Energy, Renewable Energy and the environment, CRC Press, Taylor & Francis Group, LLC, Boca Raton, 2009 Search PubMed; G. W. Crabtree and N. L. Lewis, Phys. Today, 2007, 60, 37 Search PubMed.
- For reviews on solar thermal energy: H. Yüncü, E. Paykoc and Y. Yener, in Solar Energy Utilization, Kluwer Academic Publishers, Dordrecht, 1987 Search PubMed;
T. A. Reddy, R. Battisti, H. Schweiger, W. Weiss, J. H. Morehouse, S. Vijayaraghavan and D. Y. Goswami, in Energy Conversion, Solar Thermal Energy Conversion, CRC Press, Taylor & Francis Group, Boca Raton, 2008, ch. 18, p. 18-1 Search PubMed.
- For reviews on photovoltaic energy, see:
A. Luque and S. Hegedus, in Handbook of photovoltaic science and engineering, John Wiley & Sons, Ltd, West Sussex, England, 2003 Search PubMed;
T. Markvart, in Solar Electricity, John Wiley & Sons, West Sussex, England, 2nd edn, 2000 Search PubMed; J. H. Fendler, J. Phys. Chem., 1985, 89, 2730 Search PubMed.
-
W. Vielstich, A. Lamm and H. A. Gasteiger, in Handbook of Fuel Cells: Fundamentals, Technology, Applications, John Wiley & Sons, 2003 Search PubMed;
B. Sørensen, in Hydrogen and Fuel Cells, Elsevier Academic Press, 2005 Search PubMed.
- S. Günes, H. Neugebauer and N. S. Sariciftci, Chem. Rev., 2007, 107, 1324 CrossRef; J.-L. Brédas, J. E. Norton, J. Cornil and V. Coropceanu, Acc. Chem. Res., 2009 DOI:10.1021/ar900099h , ASAP.
- H. A. Gasteiger and N. M. Markovic, Science, 2009, 324, 48 CrossRef CAS.
- J. W. Tye, M. B. Hall and M. Y. Darensbourg, Proc. Natl. Acad. Sci. U. S. A., 2005, 102, 16911 CrossRef CAS.
-
M. Graziani and P. Fornasiero, in Renewable Resources and Renewable Energy, A Global Challenge, CRC Press Taylor & Francis Group LLC, Boca Raton, 2007 Search PubMed;
J. J. Bozell and M. K. Patel, in Feedstocks for the Future, ACS Symposium Ser. 921, Oxford University Press, 2006 Search PubMed; P. Gallezot, Green Chem., 2007, 9, 295 Search PubMed.
-
B. Kamm, P. R. Gruber and M. Kamm, in Biorefineries—Industrial Processes and Products, Status Quo and Future Directions, Wiley-VCH Verlag GmbH, Weinheim, 2006, vol. 1 Search PubMed.
-
M. N. Belgacem and A. Gandini, in Monomers, Polymers and Composites from Renewable Resources, Elsevier Ltd., Oxford, 2008 Search PubMed; A. Gandini, Macromolecules, 2008, 41, 9491 Search PubMed; M. A. R. Meier, J. O. Metzger and U. S. Schubert, Chem. Soc. Rev., 2007, 36, 1788 CrossRef CAS; Y. Tokiwa and B. P. Calabia, Can. J. Chem., 2008, 86, 548 RSC.
-
T. Q. Hu, in Chemical Modifications, Properties, and Usage of Lignin, ed. Kluwer Academic/Plenum Publishers, 2002 Search PubMed;
M. Hofrichter and A. Steinbüchel, in Biopolymers, Lignin, Humic Substances and Coal, Wiley-VCH Verlag GmbH, Weinheim, 2001 Search PubMed.
-
T. Uragami and S. Tokura, in Material Science of Chitin and Chitosan, Kodansha Ltd, 2006 Search PubMed; C. K. S. Pillai, W. Paul and C. P. Sharma, Prog. Polym. Sci., 2009, 34, 641 Search PubMed.
- F. Renault, B. Sancey, P.-M. Badot and G. Crini, Eur. Polym. J., 2009, 45, 1337 CrossRef CAS; M. N. V. Ravi Kumar, R. A. A. Muzzarelli, C. Muzzarelli, H. Sashiwa and A. J. Domb, Chem. Rev., 2004, 104, 6017 CrossRef.
-
D. R. Knapp, in Handbook of Analytical Derivatization Reactions, John Wiley & Sons, Inc., 1979 Search PubMed.
-
L. D. Taylor and J. C. Warner, US Pat., 5 177 262, 1993 Search PubMed.
- A. S. Cannon and J. C. Warner, Cryst. Growth Des., 2002, 2, 255 CrossRef CAS; J. C. Warner, Pure Appl. Chem., 2006, 78, 2035 CrossRef CAS; S. Trakhtenberg and J. C. Warner, Chem. Rev., 2007, 107, 2174 CrossRef CAS.
- R. A. Sheldon, Pure Appl. Chem., 2000, 72, 1233 CAS; R. A. Sheldon, C. R. Acad. Sci. Paris, Serie IIc, 2000, 3, 541 Search PubMed;
R. A. Sheldon, I. Arends and U. Hanefeld, in Green Chemistry and Catalysis, Wiley-VCH Verlag GmbH & Co. KGaA, Weinheim, 2007 Search PubMed;
G. Rothenberg, in Catalysis, Concepts and Green Chemistry, Wiley-VCH Verlag GmbH, Weinheim, 2008 Search PubMed;
R. H. Crabtree, Handbook of Green Chemistry, Green Catalysis, Wiley-VCH Verlag GmbH, Weinheim, 2009 Search PubMed.
-
I. T. Horvath, in Encyclopedia of Catalysis, Wiley-VCH Verlag GmbH, Weinheim, 2003 Search PubMed;
B. Cornils, W. A. Hermann, M. Muhler and C.-H. Wong, in Catalysis from A to Z, Wiley-VCH Verlag GmbH, Weinheim, 2007 Search PubMed.
- N. M. Yoon and Y. S. Gyoung, J. Org. Chem., 1985, 50, 2443 CrossRef CAS.
- R. Noyori, T. Ohkuma, M. Kitamura, H. Takaya, N. Sayo, H. Kumobayashi and S. Akutagawa, J. Am. Chem. Soc., 1987, 109, 5856 CrossRef CAS.
-
A. S. Bommarius and B. R. Riebel-Bommarius, Biocatalysis, Fundamentals and Applications, Wiley-VCH Verlag GbmH, Weinheim, 1st edn, 2004 Search PubMed;
R. A. Sheldon, I. Arends and U. Hanefeld, in Green Chemistry and Catalysis, Wiley-VCH Verlag GmbH & Co. KGaA, Weinheim, 2007, ch. 1, p. 29 Search PubMed; F. van Rantwijk and R. A. Sheldon, Chem. Rev., 2007, 107, 2757 Search PubMed; H. R. Hobbs and N. R. Thomas, Chem. Rev., 2007, 107, 2786 Search PubMed.
-
R. L. Carson, in Silent Spring, Houghton Mifflin, Boston, 1962 Search PubMed; S. C. DeVito, Prog. Org. Coat., 1999, 35, 55 Search PubMed;
E. Homburg, A. S. Travis and H. G. Schröter, in The Chemical Industry in Europe, 1850–1914: Industrial Growth. Pollution, and Professionalization, Kluwer Academic
Publishers, Dordrecht, 1998 CrossRef CAS; B. A. Rattner, Ecotoxicology, 2009, 18, 773 CrossRef CAS; A. Wijbenga and O. Hutzinger, Naturwissenschaften, 1984, 71, 239 Search PubMed.
- R. S. Boethling, E. Sommer and D. DiFiore, Chem. Rev., 2007, 107, 2207 CrossRef CAS.
-
H. A. Painter, in The handbook of environmental chemistry, Springer-Verlag, Berlin, 1992, vol. 3F, p. 1 Search PubMed;
H. W. Stache, in Anionic Surfactants, Organic Chemistry, Surfactant Science Series, Marcel Dekker Inc, 1995, vol. 56 Search PubMed;
R. D. Swisher, in Surfactant Biodegradation, Surfactant Science Series, Marcel Dekker Inc, 2nd edn, 1987, vol. 18 Search PubMed.
-
M. Nendza, in Structure-Activity Relationships in Environmental Sciences, Chapman & Hall, London, 1998 Search PubMed;
M. H. van Agteren, S. Keuning and D. B. Jansee, in Handbook on Biodegradation and Biological Treatment of Hazardous Organic Compounds, Kluwer Academic Publishers, Dordrecht, 1998 Search PubMed.
-
R. S. Boethling, in Cationic Surfactants, Surfactant Science Series, Marcel Dekker, New York, 1994, vol. 53, p. 95 Search PubMed;
K. Holmberg, in Novel Surfactants, Preparation, Applications and Biodegradability, Surfactant Science Series, Marcel Dekker, Inc., 2003, vol. 114 Search PubMed;
H. Waldhoff and R. Spilker, in Handbook of Detergents, Part C: Analysis, Surfactant Science Series, Marcel Dekker, Inc., 2005, vol. 123 Search PubMed.
- P. T. Anastas, Crit. Rev. Anal. Chem., 1999, 29, 167 CrossRef CAS.
- F. R. P. Rocha, J. A. Nóbrega and O. F. Filho, Green Chem., 2001, 3, 216 RSC.
- L. H. Keith, L. U. Gron and J. L. Young, Chem. Rev., 2007, 107, 2695 CrossRef CAS.
-
C. A. M. Afonso and J. G. Crespo, in Green Separation Processes, Fundamentals and Applications, Wiley-VCH Verlag GmbH, Weinheim, 2005 Search PubMed.
- V. Vamvakaki, K. Tsagaraki and N. Chaniotakis, Anal. Chem., 2006, 78, 5538 CrossRef CAS.
- Solid Waste and Emergency response CEPPO, Chemical accident prevention and the clean air act amendments of 1990, US Environmental Protection Agency, Washington DC EPA 550K94001, 1994.
-
J. M. Stellman, in Encyclopaedia of Occupational Health and Safety, International Labour Office, Geneva, 1998, vol. 4 Search PubMed.
- J. N. Kemsley, Chem. Eng. News, 2009, 87, 29–31.
- Office of Pollution Prevention and Toxics, The Presidential Green Chemistry Challenge Awards Program, Summary of 2009 Award Entries and Recipients, US Environmental Protection Agency, Washington DC, 744K09001, 2009 Search PubMed.
- Office of Pollution Prevention and Toxics, The Presidential Green Chemistry Challenge Awards Program, Summary of 2008 Award Entries and Recipients, US Environmental Protection Agency, Washington DC, EPA 744R08002, 2008 Search PubMed.
- Office of Pollution Prevention and Toxics, The Presidential Green Chemistry Challenge Awards Program, Summary of 2006 Award Entries and Recipients, US Environmental Protection Agency, Washington DC, EPA 744R06003, 2006 Search PubMed.
- K. B. Hansen, Y. Hsiao, F. Xu, N. Rivera, A. Clausen, M. Kubryk, S. Krska, T. Rosner, B. Simmons, J. Balsells, N. Ikemoto, Y. Sun, F. Spindler, C. Malan, E. J. J. Grabowski and J. D. Armstrong III, J. Am. Chem. Soc., 2009, 131, 8798 CrossRef CAS.
- S. K. Ritter, Chem. Eng. News, 2004, 82, 25.
- S. K. Ritter, Chem. Eng. News, 2002, 80, 26.
- G. J. Quallich, Chirality, 2005, 17, S120 CrossRef CAS.
- Office of Pollution Prevention and Toxics, The Presidential Green Chemistry Challenge Awards Program, Summary of 1998 Award Entries and Recipients, US Environmental Protection Agency, Washington DC, EPA 744R98001, 1998 Search PubMed.
- M. K. Stern, B. K. Cheng, F. D. Hileman and J. M. Allman, J. Org. Chem., 1994, 59, 5627 CrossRef CAS; J. Bashkin, R. Rains and M. Stern, Green Chem., 1999, 1, G41 RSC.
|
This journal is © The Royal Society of Chemistry 2010 |
Click here to see how this site uses Cookies. View our privacy policy here.