DOI:
10.1039/B909754F
(Paper)
CrystEngComm, 2010,
12, 260-269
Hydrothermal syntheses, crystal structures and luminescent properties of zinc(II) coordination polymers constructed by bifunctional tetrazolate-5-carboxylate ligands†
Received
18th May 2009
, Accepted 24th August 2009
First published on
7th September 2009
Abstract
Two bifunctional 1H-tetrazolate-5-carboxylate ligands with different flexibilities, H2tza (1H-tetrazolate-5-acetic acid) and H2tzf (1H-tetrazolate-5-formic acid), were employed in the construction of zinc(II) complexes in the presence/absence of secondary ligands such as 2,2′-bipy and 4,4′-bipy. Three tza coordination polymers and two tzf dinuclear complexes, namely [Zn(tza)(H2O)]n (1), [Zn3(tza)2Cl2(2,2′-bipy)2(H2O)2]n (2), [Zn2(tza)2(4,4′-bipy)]n (3), [Zn(tzf)(H2O)3]2·2H2O (4) and [Zn(tzf)(2,2′-bipy)(H2O)]2·H2O (5), were hydrothermally synthesized and structurally characterized by single-crystal X-ray diffraction. Polymer 1 is a 3-D two-fold interpenetrating diamond-like network with tetranuclear [Zn(COO)]4 clusters as tertiary building units (TBUs) and µ3-κN3:κO2:κO1,N1 bridging tza as linkers. Polymer 2 presents a 2-D structure formed by the linkage of 1-D Zn-carboxylate [Zn(COO)]n helical chains and µ3-κN4:κO2:κO1,N1 bridging tza. In 3, 2-D (4,4) nets are built up with dinuclear [Zn(COO)]2 clusters and µ3-κN4:κO2:κO1,N1 bridging tza, which are pillared through 4,4′-bipy to gain a 3-D self-penetrating LB-1 (446108) topology. In dinuclear complexes 4 and 5, two Zn(II) atoms are bridged by a tzf ligand in a µ2-κN2:κO1,N1 fashion. The results denote that tetrazolate-5-carboxylate ligands can adopt variable coordination modes in the formation of the complexes, and different Zn-carboxylate aggregates can serve as tertiary building units (TBUs). The effects of the nature of tetrazolate-5-carboxylate ligands and secondary ligands, and hydrothermal reaction conditions on the structural topologies of the obtained complexes have been investigated. The photoluminescent properties and thermal stabilities of 1–5 have also been discussed.
Introduction
Exploration on the syntheses and properties of novel coordination polymers has been attracting more and more intense interest from chemists, not only for functional material purposes,1 but also for their fascinating structures.2 However, it would still be a challenge for a long time to design and construct metal–organic frameworks with the desired topologies and properties, because the molecular architectures of coordination polymers are affected by many factors, such as the ligand nature,3 the choice of secondary ligand, reaction conditions, especially reaction temperature and pH value.4 Among many influential factors, organic bridging ligands which contain adjustable flexibility and connectivity information, play a crucial role in the construction and structural tuning of coordination polymers.5 The introduction of secondary ligands can also result in structural and functional diversity.6 For example, secondary N,N′-ligands can manipulate the structural frameworks in either a terminal or bridging coordination mode.6c Among various organic ligands, polydentate ligands comprising multiple coordination sites, such as N-heterocyclic tetrazolate group7 and carboxylate group,8 have been extensively investigated and proved to be a rational choice to synthesize coordination polymers possessing intriguing structural varieties and potential applications. However, the investigation of bifunctional tetrazolate-carboxylate ligands with combined tetrazolate and carboxylate groups remains less developed.9–11 Especially, carboxylate-introduced 5-substituted tetrazolate (shortened as tetrazolate-5-carboxylate) ligands with four N and two O potential electron-donating centers can act as excellent ligands and exhibit diverse coordination modes.10,11 Notably, carboxylate groups on the ligands may be conductive to the formation of discrete multinuclear clusters and infinite building blocks by M–COO–M linkage, while tetrazolate groups may have the ability to link these clusters to an intricate extended network. Furthermore, the spacers between tetrazolate and carboxylate groups can be tunable in components, flexibilities and conjugated extent, which makes the structures and properties of the formed polymers more versatile. Finally, the introduction of secondary ligands into the reaction system may generate some interesting architectures. Recently, we employed the simplest tetrazolate-5-carboxylate, 1H-tetrazolate-5-formic acid (H2tzf), in assembly of coordination polymers, and reported the structure, nonlinear optical effect and luminescent properties of a 3-D diamond-like [Zn(Htzf)2]n.11a
In this context and as an extension of our research, we selected two structurally related tetrazolate-5-carboxylate ligands with different flexibilities, H2tza (1H-tetrazolate-5-acetic acid) and H2tzf obtained by in-situ hydrolysis of their respective ester (Scheme 1), as organic linkers to construct new metal–organic frameworks. With the introduction of bidentate chelating 2,2′-bipy and bridging 4,4′-bipy, three tza coordination polymers with infinite networks and two tzf dinuclear complexes, [Zn(tza)(H2O)]n (1), [Zn3(tza)2Cl2(2,2′-bipy)2(H2O)2]n (2), [Zn2(tza)2(4,4′-bipy)]n (3), [Zn(tzf)(H2O)3]2·2H2O (4) and [Zn(tzf)(2,2′-bipy)(H2O)]2·H2O (5), were synthesized by modifying hydrothermal reaction conditions including reactant ratio, reaction temperature, pH value and secondary ligand. Three coordination modes of tetrazolate-5-carboxylate are adopted (modes I–III Scheme 2), of which modes I and II are first observed. Interestingly, different discrete [Zn(COO)]n (n = 2, 4) cluster units and infinite [Zn(COO)]n chains can serve as tertiary building units (TBUs) in tza coordination polymers. As for tzf ligand containing N-donors in the β position, hydrothermal decarboxylation phenomena have been found in complexes formation. Luminescent properties and thermogravimetric analyses of 1–5 have also been investigated in this research.
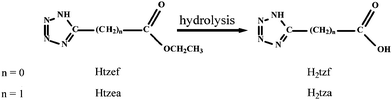 |
| Scheme 1 The formation of 1H-tetrazolate-5-carboxylic acid. | |
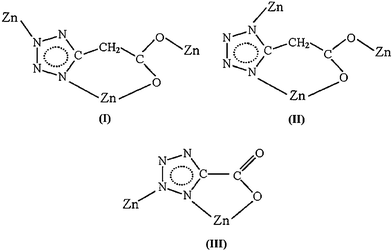 |
| Scheme 2 Three coordination modes of tetrazolate-5-carboxylate ligands observed in this study. | |
Experimental
Materials and Instruments
All chemicals were obtained from commercial sources and used without further purification. Na2tza and Na2tzf used in the analyses of photoluminescent spectra were prepared by the reaction of their respective ester, 1H-tetrazolate-5-ethyl acetate (Htzea) and 1H-tetrazolate-5-ethyl formate (Htzef), in a dilute aqueous solution of NaOH. The elemental analyses were measured on an Elementar Vario EL III microanalyzer. The FT-IR spectra were obtained on a Perkin-Elmer Spectrum using KBr disks in the range 4000–400 cm−1. Photoluminescent analyses were performed on an EI920 fluorescence spectrometer. Thermal analyses were made on a Netzsch STA 449 C Jupiter under an N2 atmosphere with the sample heated in an Al2O3 crucible at a heating rate of 10 K min−1. Powder X-ray diffraction data were collected using Rigaku Dmax2500PC powder diffractometer for 1–3 and X'Pert Pro powder diffractometer for 4 and 5 both with Cu Kα radiation and 5 ≤ 2θ ≤ 60°. Single crystal X-ray diffraction was carried out by a Rigaku Mercury CCD/AFC7R diffractometer.
Syntheses of 1–5
[Zn(tza)(H2O)]n (1).
A mixture of ZnCl2 (0.3 mmol), Htzea (0.3 mmol) and NaOH (0.4 mmol) in an aqueous solution (5 mL) was sealed into a 25 mL poly(tetrafluoroethylene)-lined stainless steel container under autogenous pressure and then heated at 160 °C for 3 d and cooled to 30 °C at a rate of 2.5 °C h−1. Colorless block crystals suitable for X-ray analyses were obtained, washed with distilled water and dried in air. Yield: 33% (based on Zn) for 1. Anal. Calcd for C3H4N4O3Zn: C, 17.20; H, 1.92; N, 26.75%. Found: C, 17.42; H, 1.82; N, 26.63%. IR (KBr pellet, cm−1): 3621s, 3496w, 3079w, 1581vs, 1500s, 1452vs, 1407s, 1376vs, 1284s, 1251s, 1141m, 941m, 810s, 740s, 567m.
[Zn3(tza)2Cl2(2,2′-bipy)2(H2O)2]n (2).
A mixture of ZnCl2 (0.3 mmol), Htzea (0.3 mmol), NaOH (0.4 mmol) and 2,2′-bipy (0.3mmol) in aqueous solution (5 mL) was sealed into a 25 mL poly(tetrafluoroethylene)-lined stainless steel container under autogenous pressure and then heated at 160 °C for 3 d and cooled to 30 °C at a rate of 2.5 °C h−1. Colorless prism crystals suitable for X-ray analyses were obtained, washed with distilled water and dried in air. Yield: 9% (based on Zn) for 2. Anal. Calcd for C26H24Cl2N12O6Zn3: C, 35.99; H, 2.79; N, 19.37%. Found: C, 35.67; H, 2.73; N, 19.12%. IR (KBr pellet, cm−1): 3394s, 3104m, 3083m, 1604vs, 1573s, 1457s, 1442vs, 1384s, 1315m, 1249m, 1081m, 1024m, 767s, 736s, 653m, 582m.
[Zn2(tza)2(4,4′-bipy)]n (3).
The procedure was the same as that for 2 except that 2,2′-bipy was replaced by 4,4′-bipy. Yield: 23% (based on Zn) for 3. Anal. Calcd for C16H12N10O4Zn2: C, 35.65; H, 2.24; N, 25.98%. Found: C, 35.83; H, 2.18; N, 25.69%. IR (KBr pellet, cm−1): 3423b, 3064w, 3000w, 1639vs, 1612s, 1454s, 1421s, 1265m, 1224m, 1076m, 808s, 723m, 636m, 599w.
[Zn(tzf)(H2O)3]2·2H2O (4).
The procedure was the same as that for 1 with Htzef in place of Htzea, and the reaction temperature was adjusted to 80 °C. Yield: 34% (based on Zn) for 4. Anal. Calcd for C4H16N8O12Zn2: C, 9.63; H, 3.23; N, 22.46%. Found: C, 9.77; H, 3.09; N, 22.22%. IR (KBr pellet, cm−1): 3357b, 1637vs, 1492vs, 1415s, 1338vs, 1230s, 1186m, 1089m, 1066m, 844s, 680b. If a smaller quantity of NaOH (0.2 mmol) was added, a 3D-diamond-like [Zn(Htzf)2]n11a with the carboxylate group uncoordinated was obtained. When the reaction temperature was raised above 120 °C and the NaOH amount was simultaneously changed from 0.2 to 0.4 mmol, the decarboxylated product [Zn(HCN4)2],12 was obtained.
[Zn(tzf)(2,2′-bipy)(H2O)]2·H2O (5).
The procedure was the same as that for 2 except that Htzea was substituted by Htzef, and the reaction temperature was adjusted to 80 °C. Yield: 11% (based on Zn) for 5. Anal. Calcd for C24H22N12O7Zn2: C, 39.97; H, 3.07; N, 23.30%. Found: C, 39.99; H, 3.04; N, 23.19%. IR (KBr pellet, cm−1): 3270b, 3114s, 1616vs, 1472vs, 1440s, 1402s, 1328vs, 1311s, 1025m, 765s, 734m, 651m, 410m. Notably, when the reaction temperature was increased above 120 °C, the decarboxylated product [Zn(HCN4)2],12 was obtained. And when 4,4′-bipy instead of 2,2′-bipy was added following the same procedure as 5, the 1-D polymer [ZnCl2(4,4′-bipy)]n13 was always obtained at different reaction temperatures (80–160 °C) and pH values (with the NaOH amount ranging from 0.2 to 0.4 mmol).
Single crystal structures determination
Single crystals of 1–5 suitable for X-ray analyses were mounted at the apex of a glass fiber for data collection. Data collections were performed at 293(2) K on a Rigaku AFC7R diffractometer for 1 and on a Rigaku Mercury CCD diffractometer for 2–5, both diffractometers being equipped with a graphite-monochromated Mo Kα radiation source (λ = 0.71073 Å). There was no evidence of crystal decay during data collection. The intensity data sets were collected with the ω scan technique and reduced by CrystalClear software.14 The structures were solved by the direct methods and refined by full-matrix least-squares techniques. Non-hydrogen atoms for 1–5 were located by difference Fourier maps and subjected to anisotropic refinement. Hydrogen atoms of lattice water molecules were located by difference Fourier maps and those of coordinated water molecules were calculated in idealized positions, and all hydrogen atoms of water molecules were refined with O–H distances restrained to a target value of 0.85 Å and Uiso (H) = 1.5Ueq (O). The remaining hydrogen atoms were calculated in idealized positions and allowed to ride on their parent atoms. Approximate anisotropic thermal refinement was applied to individual carbon atoms from 2,2′-bipy in 2 and 4,4′-bipy in 3. All calculations were performed by the Siemens SHELXTL version 5 package of crystallographic software.15 Pertinent crystal data and structural refinement results and selected bond distances and angles for 1–5 are listed in Table 1 and Table S1,† respectively.
|
1
|
2
|
3
|
4
|
5
|
R
1 = ∑(Fo - Fc)/∑ Fo.
wR
2 = [∑w(Fo2–Fc2)2/∑w(Fo2)2]1/2.
|
Formula |
C3H4N4O3Zn |
C26H24Cl2N12O6Zn3 |
C16H12N10O4Zn2 |
C4H16N8O12Zn2 |
C24H22N12O7Zn2 |
Formula mass |
209.47 |
867.58 |
539.10 |
499.03 |
721.28 |
Space group |
P4(2)/n |
Fdd2 |
P2(1)/c |
P-1 |
P-1 |
a/Å |
12.7076(15) |
22.118(3) |
13.515(3) |
7.153(4) |
9.396(3) |
b/Å |
12.7076(15) |
44.164(7) |
6.7465(13) |
7.752(4) |
11.094(3) |
c/Å |
7.7157(17) |
6.6646(7) |
10.667(2) |
8.775(4) |
13.567(4) |
α/° |
90 |
90 |
90 |
97.048(3) |
83.399(9) |
β/° |
90 |
90 |
91.33(3) |
112.728(4) |
81.828(7) |
γ/° |
90 |
90 |
90 |
108.627(4) |
74.782(9) |
V/Å3 |
1246.0(3) |
6510.1(15) |
972.4(3) |
408.1(4) |
1346.3(7) |
Z
|
8 |
8 |
2 |
1 |
2 |
D
c/g cm−3 |
2.233 |
1.770 |
1.841 |
2.031 |
1.779 |
µ/mm−1 |
3.899 |
2.418 |
2.517 |
3.020 |
1.853 |
Total reflns |
1313 |
11904 |
6394 |
2845 |
10119 |
Unique reflns |
1138 |
3596 |
1759 |
1487 |
4881 |
R
int
|
0.0543 |
0.0582 |
0.0403 |
0.0197 |
0.0402 |
GOF |
1.048 |
0.979 |
1.076 |
1.017 |
1.030 |
R
1
[I >2σ(I)] |
0.0327 |
0.0542 |
0.0435 |
0.0225 |
0.0379 |
wR
2
(all data) |
0.0917 |
0.1375 |
0.1068 |
0.0593 |
0.0886 |
Results and discussions
Syntheses
In order to investigate the influence of the ligand flexibility, secondary ligand and reaction conditions on the architecture of coordination polymers, we chose two tetrazolate-5-carboxylate ligands with different molecular backbones and flexibilities with the incorporation of different secondary ligands, and a modified reactant ratio, reaction temperature and pH value. The synthetic route is shown in Scheme 3. 1H-tetrazolate-5-carboxylate ligands were generated by in-situ hydrolysis of their respective ester and NaOH, which could simultaneously adjust the pH value of the reactant mixture. For the Htzea reaction system with the same ZnCl2, Htzea and NaOH molar ratio of 0.3
:
0.3
:
0.4 at the same reaction temperature (160 °C), polymers 1–3 with different topologies were obtained in the presence/absence of 2,2′-bipy and 4,4′-bipy. The results indicate that different secondary ligands have a big effect on the formation of tza coordination polymers. However, the effects on the final product of tzf coordination compounds are more complicated. The reaction mixture of ZnCl2, Htzef and NaOH in the reactant ratio of 0.3
:
0.3
:
0.4 with a higher pH value at 80 °C produced dinuclear 4, while the reactant ratio of 0.3
:
0.3
:
0.2 at a lower pH value gave protonated [Zn(Htzf)2]n, which was previously obtained from the reaction of H2tzf and ZnCl2 at room temperature.11a It is worthy of note that decarboxylation of tzf was observed at the higher reaction temperature. When the reaction temperature was raised above 120 °C in a limited extent of pH values with a reactant ratio of ZnCl2, Htzef and NaOH varying from 0.3
:
0.3
:
0.2 to 0.3
:
0.3
:
0.4, the decarboxylated product [Zn(HCN4)2] was formed, which was previously obtained by a hydrothermal reaction of Zn(OAc)2 and tetrazole.12 The hydrothermal decarboxylation of carboxylic acid containing N-donors in the β position has been well documented,16 which is first encountered for tzf ligand. Regretfully, the attempts to synthesize Zn(II) complex with mixed ligands of tzf and 4,4′-bipy failed at different reaction temperatures and reactant ratios, and 1-D polymer [ZnCl2(4,4′-bipy)]n was always produced, which was previously prepared by directly reacting 4,4′-bipy with ZnCl2.13 Based on the aforementioned facts, reaction conditions such as reaction temperature, pH value and secondary ligand exert a synergic effect on the formation of tzf coordination compounds.
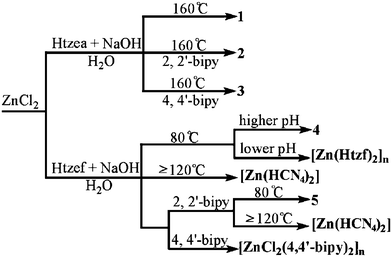 |
| Scheme 3 The synthetic route of tetrazolate-5-carboxylate complexes. | |
Structural descriptions and discussions
[Zn(tza)(H2O)]n (1).
The single-crystal X-ray structural analyses reveal that 1 features a 3-D net built up by the fusion of tetranuclear [Zn(COO)]4 cluster units and tetrazolate groups. Polymer 1 possesses an asymmetric unit consisting of one zinc atom, one tza ligand, and one coordinated water molecule (Fig. 1a). The coordination environment around Zn is a slightly distorted [ZnO3N2] trigonal bipyramid with the Zn–O distances being in the normal range of 1.991(2)–2.139(2) Å and Zn–N distance being 2.028(2) and 2.061(2) Å, where two nitrogen donor atoms [N1C, N3D] and two oxygen donor atoms [O1C, O2] belong to three different symmetry-related tza ligands. The remaining site is occupied by one coordinated water molecule. In 1, each tza ligand exhibits a µ3-κN3
:
κO2
:
κO1,N1 coordination mode (mode I in Scheme 2), and acts as a tetradentate linker to connect three Zn atoms, with a syn–anti configuration of the carboxylate group and a µ2-1,3N mode of the tetrazolate group, along with O1 and N1 atoms being in a chelated fashion. Although numerous coordination styles of tetrazolate-5-carboxylate ligands have been found,10,11 the unusual coordination mode of tza ligand in 1 is first observed, which favors the formation of a new structure with an interesting topology.
![Polymer 1: (a) The coordination environment around the Zn atom and coordination mode of tza ligand. Symmetry codes are defined in Table S1. (b) A 3-D network constructed by the linkage of [Zn(COO)]4 cluster units as TBUs and tetrazolate groups as “double-bridges”. The H atoms and coordinated water molecules are omitted for clarity. (c) Two-fold interpenetrated 3-D diamond-like topology. The black balls represent the TBUs of [Zn(COO)]4 clusters and the lines represent the “double-bridges”.](/image/article/2010/CE/b909754f/b909754f-f1.gif) |
| Fig. 1 Polymer 1: (a) The coordination environment around the Zn atom and coordination mode of tza ligand. Symmetry codes are defined in Table S1. (b) A 3-D network constructed by the linkage of [Zn(COO)]4 cluster units as TBUs and tetrazolate groups as “double-bridges”. The H atoms and coordinated water molecules are omitted for clarity. (c) Two-fold interpenetrated 3-D diamond-like topology. The black balls represent the TBUs of [Zn(COO)]4 clusters and the lines represent the “double-bridges”. | |
The most striking structural feature in 1 is that tetranuclear [Zn(COO)]4 cluster units are interlinked together via the µ2-1,3N tetrazolate groups to generate a novel 3-D net (Fig. 1b). Four Zn atoms are bridged alternately by four carboxylate groups to form a sixteen-membered cyclic [Zn(COO)]4 ring as a tertiary building unit (TBU). In the tetranuclear cluster unit, four Zn atoms are not in a plane, but arrange in a distorted tetrahedron. Notably, coordination polymers containing such a tetranuclear [Zn(COO)]4 TBU are scarce,17,18 in some of which four Zn atoms display a coplanar array.18d The tetranuclear cluster units are linked together by tetrazolate groups, each of which coordinates two Zn centers from different tetranuclear cluster units in a µ2-1,3N mode. As shown in Fig. 1b, each tetranuclear cluster unit is surrounded by eight tetrazolate groups, each pair of which connecting two tetranuclear cluster units to form “double-bridges”.19 As a result, each tetranuclear cluster unit is linked by four “double-bridges”, relating to four adjacent tetranuclear cluster units. Thus, if each “double-bridge” is considered as a linker,20 and each tetranuclear cluster unit is regarded as a four-connected node, with each node lying at the vertex of an approximate tetrahedron, 1 defines a two-fold interpenetrating diamond-like topology (Fig. 1c). The formation of such an interesting net for 1 constructed by tetranuclear [Zn(COO)]4 cluster units as TBUs and tetrazolate groups as “double-bridges” may be ascribed to the unusual coordination mode of the tza ligand.
[Zn3(tza)2Cl2(2,2′-bipy)2(H2O)2]n (2).
When secondary ligand 2,2′-bipy was introduced into the reaction system of ZnCl2, Htzea and NaOH, polymer 2 with a new 2-D framework was produced. As shown in Fig. 2a, there are two crystallographically independent Zn centers with four-coordinated Zn1 and six-coordinated Zn2 in 2. The Zn1 atom, lying on a twofold axis, is coordinated by two symmetry-related tetrazolate nitrogen atoms (N4, N4A) and two symmetry-related chlorine atoms (Cl1, Cl1A) to furnish a distorted tetrahedral coordination geometry with the angles varying from 102.13(10) to 112.33(4)°. The bond distance of Zn1–Cl1 is 2.273(1) Å, which is comparable with those of similar complexes with bond distances ranging from 2.196(2) to 2.2509(8) Å.21 The Zn2 atom adopts a distorted octahedral coordination geometry by two nitrogen atoms (N5, N6) of 2,2′-bipy ligand and two oxygen atoms (O1, O1W) in the almost equatorial plane, as well as O2B and N1 from two symmetry-related tza ligands at the axial sites with the O2B–Zn2–N1 angle of 168.22(6)°. The Zn–O distances are in the range of 2.107(2)–2.156(1) Å, as well as Zn–N distances 2.002(2)–2.145(2) Å. Each carboxylate group from tza ligand in 2 bridges two symmetry-related Zn2 centers with the same syn–anti mode as that in 1. However, unlike in 1, the tetrazolate group in 2 presents a µ2-1,4N mode rather than a µ2-1,3N mode, to bond Zn1 and Zn2 centers via N1 and N4 atoms [Zn1⋯Zn2 = 6.100(1) Å], along with O1 and N1 atoms chelating to the Zn2 center. So another unusual coordination mode µ3-κN4
:
κO2
:
κO1,N1 of tetrazolate-5-carboxylate ligands in 2 (mode II in Scheme 2) is found.
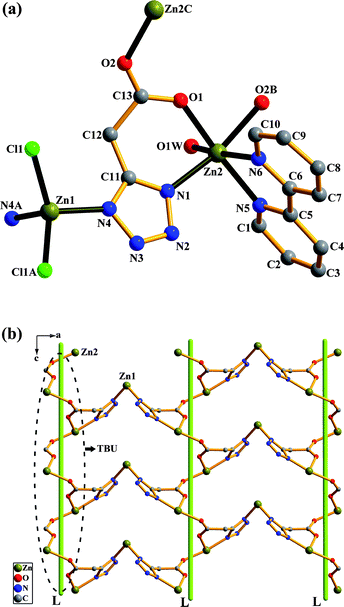 |
| Fig. 2 Polymer 2: (a) The coordination environment around the Zn atom and coordination mode of tza ligand. Symmetry codes are defined in Table S1. (b) View of the left-handed helical chains extending along the c axis and the 2-D sheet network. The Cl atoms, 2, 2′-bipy and coordinated water molecules are omitted for clarity. | |
Interestingly, syn–anti carboxylate groups from tza ligands link Zn2 atoms in an alternate manner to form left-handed helical [Zn(COO)]n infinite chains as TBUs, rather than a tetranuclear [Zn(COO)]4 cluster units in 1, extending along the c axis with the helical pitch being 6.6646(7) Å as shown in Fig. 2b. The similar [Zn(COO)]n infinite chains were widely investigated in numerous zinc(II) polymers with varied carboxylate-containing ligands.22 The helical chains arrange along the a direction to produce a chiral sheet, and the Zn2 centers between adjacent chains link to each other through V-shaped tetrazolate-Zn1-tetrazolate bridging units with an angle Zn2–Zn1–Zn2 of 101.059(4)°. The adjacent sheets, made up of right-handed helical chains of opposite chirality, are stacked parallel to each other in an –ABAB– sequence to give the whole achiral structure, as depicted in Fig. S1.† The generation of the 2-D structure in 2 may be attributed to the templating role of chelating 2,2′-bipy, as well as the versatile coordination modes of tza ligand.
[Zn2(tza)2(4,4′-bipy)]n (3).
When 4,4′-bipy was used instead of 2,2′-bipy, polymer 3 with an unusual 3-D self-penetrating structure differing from those of 1 and 2, was obtained. It is worthy of note that there is no coordinated or lattice water molecule in 3, although it was synthesized by a hydrothermal reaction. There are one zinc atom, one tza ligand and half a 4,4′-bipy molecule in an asymmetric unit of 3 (Fig. 3a). The whole 4,4′-bipy molecule was organized through an inversion center. The coordination geometry around the Zn atom can be described as a slightly distorted trigonal bipyramid. As illustrated in Fig. 3a, N1, N4C and O2A atoms from three different symmetry-related tza ligands constitute a basal plane, while the axial positions are occupied by O1 atom of the carboxylate group and N5 atom of 4,4′-bipy with the axial angle O1–Zn1–N5 of 172.37(7)°, deviating the ideal angle of 180°. The Zn–O distances are in the normal range of 1.977(2)–2.217(2) Å, as well as Zn–N distances 2.030(2)–2.143(2) Å. Each tza ligand connects to three symmetry-related Zn centers, adopting the same coordination mode µ3-κN4
:
κO2
:
κO1,N1 as in 2 (mode II in Scheme 2), with a µ2-1,4N mode of tetrazolate group and a syn–anti configuration of the carboxylate group, which functions one oxygen atom for chelating and the other for bridging.
![Polymer 3: (a) the coordination environment around the Zn atom and coordination mode of tza ligand. Symmetry codes are defined in Table S1. (b) View of the 2-D sheet network constructed by [Zn(COO)]2 TBUs and tetrazolate groups. (c) Schematic representation of a 3-D topology built by 2-D (4,4)-connected nets and 4,4′-bipy linkers. The black balls represent [Zn(COO)]2 TBUs. The pink and green lines represent tetrazolate groups and 4,4′-bipy molecules, respectively.](/image/article/2010/CE/b909754f/b909754f-f3.gif) |
| Fig. 3 Polymer 3: (a) the coordination environment around the Zn atom and coordination mode of tza ligand. Symmetry codes are defined in Table S1. (b) View of the 2-D sheet network constructed by [Zn(COO)]2 TBUs and tetrazolate groups. (c) Schematic representation of a 3-D topology built by 2-D (4,4)-connected nets and 4,4′-bipy linkers. The black balls represent [Zn(COO)]2 TBUs. The pink and green lines represent tetrazolate groups and 4,4′-bipy molecules, respectively. | |
To shed light on the network constructed by carboxylate-Zn clusters and tetrazolate group linkers, we first omit the 4,4′-bipy bridging secondary ligands. As shown in Fig. 3b, the carboxylate groups from tza ligands link Zn atoms in a syn–anti mode to give an eight-membered cyclic ring of a centrosymmetric dimeric unit [Zn(COO)]2 as TBU, differing from that in 1 and 2. And each unit, as a four-connecting node, relates to four equivalent ones through four µ2-1,4N tetrazolate groups as linkers. As a result, a 2-D layer network parallel to the bc plane is formed, displaying a (4,4)-connected topology. Then these layers, constructed by Zn atoms and tza ligands, are further pillared through 4,4′-bipy, resulting in an unusual 3-D six-connected self-penetrating LB-1 (446108) topology illustrated in Fig. 3c. In contrast to most six-connected nets based on the (4,4)-connected sheet: pcu (41263), roa (446108) and rob (48668), the existing examples for such self-penetrating LB-1 net are limited.23 The intricate 3-D infinite network is depicted in Fig. S2.† It is well known that 4,4′-bipy as an organic bridging linker in constructing new coordination polymers with specific structures and properties have been widely investigated.24 With this intention we successfully applied it into our case as a pillared connector to link adjacent 2-D layers into a final 3-D structure, which further confirms the key role of a rigid secondary ligand in mediating and constructing coordination polymers. More significantly, this offers a feasible way for us to rationally modify the structures and geometries of tetrazolate-5-carboxylate coordination polymers with an introduction of rigid secondary ligands.
[Zn(tzf)(H2O)3]2·2H2O (4).
Single-crystal X-ray diffraction analysis revealed that 4 is an isolated dinuclear structure with each asymmetric unit containing one Zn atom, one tzf ligand, three coordinated water molecules, and one lattice water molecule. The repetition of the Zn atoms through an inversion centre gives rise to a dinuclear Zn2 unit, as shown in Fig. 4a. In 4, the coordination geometry of the Zn atom can be described as a distorted octahedron [ZnN2O4] with an axial angle O2W–Zn1–O3W of 175.82(8)°. The tzf ligand acts as a tridentate linker to chelate one Zn atom and bridges the other Zn atom in a µ2-κN2
:
κO1,N1 coordination mode (mode III in Scheme 2). In contrast to modes I and II, the tetrazolate group in 4 presents a µ2-1,2N mode and one oxygen atom from the carboxylate group remains uncoordinated. This coordination mode is similar to that reported recently.10g In 4, two Zn atoms are bridged by tetrazolate groups from two symmetry-related tzf ligands to form one six-membered ring (Zn1–N1–N2–Zn1A–N1A–N2A). Similar six-membered rings were previously found in other tetrazolate-bridged Zn complexes.25 The formation of an isolated dinuclear unit for 4 could be ascribed to the nature of the rigid tzf ligand as well as the formation of a Zn2N4 six-membered ring. So far there have only been a few reports concerning dinuclear complexes bridged by tetrazolates.26
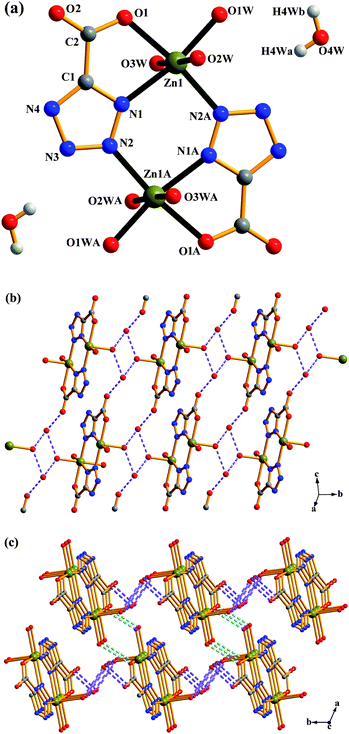 |
| Fig. 4 Complex 4: (a) molecular structure of 4. Symmetry codes are defined in Table S1. (b) A perspective view of a 2-D supramolecular architecture in 4via O4W⋯O1 and O4W⋯O2W hydrogen bonds. (c) Schematic representation of 2-D → 3-D supramolecular architecture via O1W⋯O1 hydrogen bonds in 4. | |
On the other hand, there are plenty of O–H⋯O/O–H⋯N hydrogen bonds as listed in Table S2,† resulting in an intricate supramolecular structure. For convenience, we first consider O–H⋯O hydrogen bonds involved with the lattice water molecule O4W, which leads to a 2-D layer (Fig. 4b); and then a self-assembly of 2-D layers by means of O1W⋯O1 hydrogen bonding interaction affords a 3-D supramolecular network (Fig. 4c). In fact, intermolecular O1W⋯O2, O4W⋯N3 and O3W⋯N4 hydrogen bonds, together with intramolecular O3W⋯O2, coexist in the final supramolecular 3-D network (Fig. S3).† No significant aromatic stacking is observed.
[Zn(tzf)(2,2′-bipy)(H2O)]2·H2O (5).
Another isolated neutral dinuclear complex 5 was obtained when 2,2′-bipy was introduced into the reaction mixture of ZnCl2, Htzef and NaOH. In 5, the asymmetric dinuclear unit is composed of two Zn atoms, two tzf ligands, two 2,2′-bipy and two coordinated water molecules (Fig. 5a). The configuration of the dinuclear core in 5 is similar to that in 4. The main difference is that one chelating 2,2′-bipy molecule in 5 replaces two coordinated water molecules to accomplish Zn coordination. The tzf ligand exhibits the same coordination mode as that in 4. There also exist extensive hydrogen bonds, which connect the dinuclear units to give a 2-D supramolecular network (Fig. 5b). Full details of the hydrogen-bonding geometries are given in Table S2.† No significant aromatic stacking is either observed.
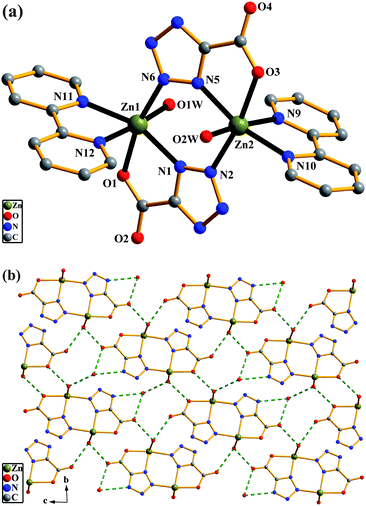 |
| Fig. 5 Complex 5: (a) molecular structure of 5 with lattice water molecules omitted for clarity. (b) A perspective view of a 2-D supramolecular network formed by hydrogen bonds in 5. | |
It's well known that the utilization of metal clusters or inorganic chains as building blocks in place of single metal ions has provided a promising pathway toward the generation of novel coordination polymers.27,28 In polymers 1–3, three different Zn-carboxylate moieties as TBUs are found in spite of the same syn–anti fashion of the carboxylate group: sixteen-membered cyclic tetranuclear clusters [Zn(COO)]4, infinite helix chains [Zn(COO)]n and eight-membered cyclic dinuclear clusters [Zn(COO)]2. This greatly enriches the architectures and properties of metal–organic frameworks. Owing to four N and two O binding centers, tetrazolate-5-carboxylate ligands display versatile coordination modes, which provides the possibility of constructing novel coordination polymers with desirable structures and properties. Two new coordination modes of tetrazolate-5-carboxylate ligands are first observed in this study (Modes I and II in Scheme 2). Obviously, mode I with the tetrazolate group being a µ2-1,3N mode can more effectively minimize the steric hindrance than mode II, in which the tetrazolate group adopts a µ2-1,4N mode. The tza ligand adopts mode I in 1 but presents mode II in 2 and 3. Thus, regardless of the secondary ligands 2,2′-bipy and 4,4′-bipy, 1 has higher dimensional topology (3-D), while 2 and 3 exhibit a lower dimensional one (2-D). On the other hand, two different kinds of rigid secondary ligands were used to enrich the diversities of polymeric architectures. A 2-D net is formed by the linkage of Zn and tza in 2, in which 2,2′-bipy merely exhibits a terminal chelation mode. In 3, the connection of Zn and tza constructs a 2-D net, which is further joined by linear bridging 4,4′-bipy to generate a self-penetrating 3-D framework. To the best of our knowledge, this is the first instance to consciously synthesize coordination polymers by incorporating different rigid secondary ligands into the flexible tza system with the aim to enrich their frameworks, although different rigid secondary ligands were introduced into the tzf case lately.10g It should be noted that the molecular backbone of tza ligand accompanying the centered sp3 carbon atom between tetrazolate and carboxylate groups, which endows tza ligand with the capability of properly distorting to meet the steric requirement upon metal coordination. Therefore, despite the same coordination fashion of the tza ligand, the TBUs and molecular frameworks of 2 and 3 are different from each other. Compared with the tza ligand, the tzf ligand exhibits a µ2-κN2
:
κO1,N1 coordination fashion (mode III in Scheme 2) in 4 and 5, which implies that the tza ligand possesses more flexibility than the tzf ligand in assembling coordination polymers. These observations suggest that the flexibilities of tetrazolate-5-carboxylate and the coordination tendencies of secondary ligands play important roles in tuning molecular frameworks.
Luminescence properties
The photoluminescence of d10 metal complexes has been attracting intensive research interest, owing to their potential applications in chemical sensors, photochemistry and electroluminescent (EL) displays.29 To examine the photoluminescent properties of tetrazolate-5-carboxylate coordination polymers/complexes, the excitation and emission spectra of 1–5 as well as Na2tza and Na2tzf in the solid-state were investigated at room temperature (Fig. S4† and Fig. 6), and the luminescent decay curves of 1–5 were further examined by nanosecond (ns) laser system at room temperature (Fig. S5).† Compounds 1–5 exhibit strong blue photoluminescence with emission maxima at 424 nm (τ = 4.75 ns), 404 nm (τ = 7.02 ns), 409 nm (τ = 7.20 ns), 428 nm (τ = 3.84 ns) and 401 nm (τ = 5.34 ns) upon excitation at 352, 322, 318, 338 and 324 nm, respectively. The sodium salts of tetrazolate-5-carboxylate, Na2tza and Na2tzf, display photoluminescent emission with maximum at 414 nm (λex = 338 nm) and 424 nm (λex = 356 nm), respectively. By comparing the locations and profiles of their emission peaks, the photoluminescent mechanism of 1–5 is tentatively attributed to intraligand transitions mainly through their tetrazolate rings.30 The results suggest that these complexes may be good blue light emitting candidate materials.
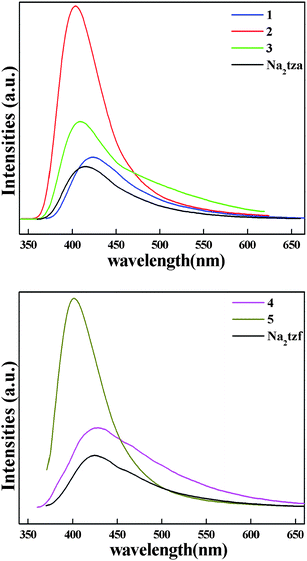 |
| Fig. 6 The emission spectra in the solid state: (a) 1–3 and Na2tza; (b) 4, 5 and Na2tzf. | |
Thermogravimetric analyses
There was no evidence of crystal decay during X-ray data collection for all compounds, which implies they are stable at ambient conditions. Thus, thermogravimetric analyses (TGA) experiments for 1–5 were performed to investigate their thermal stability, and the TGA curves were provided in Fig. 7. The results show that the weight loss of 8.55% in the temperature range of 166–316 °C and 4.32% in the temperature range of 166–316 °C for 1 and 2 (calculated: 8.6% and 4.15%) respectively, reveals evacuation of the coordinated water molecules. Then the decomposition of the tza ligand, with the framework collapse, occurred upon heating to 325 and 260 °C, for 1 and 2, respectively. As for 3, the TGA curve displays a weight loss of 46.52% from 320 to 500 °C, corresponding to the pyrolysis of one tza ligand (calculated: 46.77%), and then the framework continued to crash above 630 °C. It is notable that metal–organic coordination polymers with such high thermal stability as 3 are unusual,31,11b which may be the consequence of no coordinated aqua or lattice water molecule in 3. With regard to 4, the first weight loss of 29.09% from 70 to 220 °C corresponds to the release of six aqua and two lattice water molecules (calculated: 28.86%). With that, the remaining substance was decomposed starting at 260 °C. The TGA curve of 5 displays a similar character to that of 4. The first weight loss of 7.57% from 90 to 195 °C is consistent with the release of two aqua and one lattice water molecules (calculated: 7.49%), and then the rapid pyrolysis of the framework took place above 245 °C.
Conclusions
In summary, hydrothermal syntheses, crystal structures and luminescent properties of three tza coordination polymers with infinite networks and two tzf dinuclear complexes have been reported. In the tza polymers, three different Zn-carboxylate aggregates, [Zn(COO)]2 and [Zn(COO)]4 cluster units and [Zn(COO)]n chains, serve as TBUs and tza ligand exhibits two new coordination modes. The results show that the nature of the tza and tzf ligands as well as 2,2′-bipy and 4,4′-bipy secondary ligands, and the reaction conditions play an important role in governing the molecular frameworks of 1–5. Furthermore, emission spectra demonstrate that the tza/tzf complexes might be good candidates for efficient luminescent materials, mainly owing to intraligand n–π* or π–π* transitions of their tetrazolate rings.
Acknowledgements
This work was financially supported by 973 Program (2006CB932900 and 2007CB936703), the National Nature Science Foundation of China (20871115) and Fujian Province (A0420002).
references
-
(a) J. Kido and Y. Okamoto, Chem. Rev., 2002, 102, 2357 CrossRef CAS;
(b) N. L. Rosi, J. Eckert, M. Eddaoudi, D. T. Vodak, J. Kim, M. O'Keeffe and O. M. Yaghi, Science, 2003, 300, 1127 CrossRef CAS;
(c) C. Y. Niu, B. L. Wu, X. F. Zheng, H. Y. Zhang, H. W. Hou, Y. Y. Niu and Z. J. Li, Cryst. Growth Des., 2008, 8, 1566 CrossRef CAS.
-
(a) M. Eddaoudi, D. B. Moler, H. L. Li, B. L. Chen, T. M. Reineke, M. O'Keeffe and O. M. Yaghi, Acc. Chem. Res., 2001, 34, 319 CrossRef CAS;
(b) L. Brammer, Chem. Soc. Rev., 2004, 33, 476 RSC.
-
(a) M. J. Plater, M. R. S. J. Foreman, T. Gelbrich, S. J. Coles and M. B. Hursthouse, J. Chem. Soc., Dalton Trans., 2000, 3065 RSC;
(b) D. L. Reger, R. F. Semeniuc, L. Silaghi-Dumitrescu and M. D. Smith, Inorg. Chem., 2003, 42, 3751 CrossRef CAS;
(c) M. C. Fernández-Fernández, R. Bastida, A. Macias, P. Pérez-Lourido and L. Valencia, Inorg. Chem., 2006, 45, 2266 CrossRef CAS;
(d) A. Q. Wu, Y. Li, F. K. Zheng, G. C. Guo and J. S. Huang, Cryst. Growth Des., 2006, 6, 444 CrossRef CAS;
(e) J. Bourlier, M. W. Hosseini, J. M. Planeix and N. Kyritsakas, New J. Chem., 2007, 31, 25 RSC.
-
(a) J. F. Ma, J. F. Liu, X. Yan, H. Q. Jia and Y. H. Lin, J. Chem. Soc., Dalton Trans., 2000, 2403 RSC;
(b) M. C. Hong, Y. J. Shako, W. P. Su, F. Cao, M. Fujita, Z. Y. Zhou and A. S. C. Chan, Angew. Chem., Int. Ed., 2000, 39, 2468 CrossRef CAS;
(c) L. Pan, T. Frydel, M. B. Sander, X. Y. Huang and J. Li, Inorg. Chem., 2001, 40, 1271 CrossRef CAS;
(d) S. L. James, Chem. Soc. Rev., 2003, 32, 276 RSC;
(e) M. L. Tong, S. Kitagawa, H. C. Chang and M. Ohba, Chem. Commun., 2004, 418 RSC;
(f) P. M. Forster, A. R. Burbank, C. Livage, G. Férey and A. K. Cheetham, Chem. Commun., 2004, 368 RSC.
-
(a) X. H. Bu, W. Chen, S. L. Lu, R. H. Zhang, D. Z. Liao, W. M. Bu, M. Shionoya, F. Brisse and J. Ribas, Angew. Chem., Int. Ed., 2001, 40, 3201 CrossRef CAS;
(b) L. Carlucci, G. Ciani, D. M. Proserpio and S. Rizzato, CrystEngComm, 2002, 4, 413 RSC;
(c) X. L. Wang, C. Qin, E. B. Wang, L. Xu, Z. M. Su and C. W. Hu, Angew. Chem., Int. Ed., 2004, 43, 5036 CrossRef CAS.
-
(a) G. P. Yong, Z. Y. Wang and Y. Cui, Eur. J. Inorg. Chem., 2004, 4317 CrossRef CAS;
(b) Y. B. Dong, P. Wang, R. Q. Huang and M. D. Smith, Inorg. Chem., 2004, 43, 4727 CrossRef CAS;
(c) F. Li, Z. Ma, Y. L. Wang, R. Cao, W. H. Bi and X. Li, CrystEngComm, 2005, 7, 569 RSC;
(d) B. C. David and R. H. Lyall, Inorg. Chem., 2007, 46, 1634 CrossRef CAS.
-
(a) R. G. Xiong, X. Xue, H. Zhao, X. Z. You, B. F. Abrahams and Z. Xue, Angew. Chem., Int. Ed., 2002, 41, 3800 CrossRef CAS;
(b) M. Dinca, A. F. Yu and J. R. Long, J. Am. Chem. Soc., 2006, 128, 8904 CrossRef CAS;
(c) H. Zhao, Z. R. Qu, H. Y. Ye and R. G. Xiong, Chem. Soc. Rev., 2008, 37, 84 RSC.
-
(a) M. Eddaudi, J. Kim, J. B. Wachter, H. K. Chae, M. O'Keeffe and O. M. Yaghi, J. Am. Chem. Soc., 2001, 123, 4368 CrossRef CAS;
(b) L. Pan, M. B. Sander, X. Huang, J. Li, M. Smith, E. Bittner, B. Bockrath and J. K. Johnson, J. Am. Chem. Soc., 2004, 126, 1308 CrossRef CAS.
-
(a) F. He, M. L. Tong, X. L. Yu and X. M. Chen, Inorg. Chem., 2005, 44, 559 CrossRef CAS;
(b) Q. Yu, X. Q. Zhang, H. D. Bian, H. Liang, B. Zhao, S. P. Yan and D. Z. Liao, Cryst. Growth Des., 2008, 8, 1140 CrossRef CAS.
-
(a) Z. R. Qu, H. Zhao, X. S. Wang, Y. H. Li, Y. M. Song, Y. J. Liu, Q. Ye, R. G. Xiong, B. F. Abrahams, Z. L. Xue and X. Z. You, Inorg. Chem., 2003, 42, 7710 CrossRef CAS;
(b) X. S. Wang, X. F. Huang and R. G. Xiong, Chin. J. Inorg. Chem., 2005, 21, 1020 CAS;
(c) J. Tao, Y. F. Zhao and X. M. Zhang, Inorg. Chem. Commun., 2007, 10, 1194 CrossRef CAS;
(d) Z. P. Yu, Y. Xie, S. J. Wang, G. P. Yong and Z. Y. Wang, Inorg. Chem. Commun., 2008, 11, 372 CrossRef CAS;
(e) G. W. Yang, Q. Y. Li, Y. Zhou, P. Sha, Y. S. Ma and R. X. Yuan, Inorg. Chem. Commun., 2008, 11, 723 CrossRef CAS;
(f) Q. X. Jia, Y. Q. Wang, Q. Yue, Q. L. Wang and E. Q. Gao, Chem. Commun., 2008, 4894 RSC;
(g) Q. X. Jia, W. W. Sun, C. F. Yao, H. H. Wu, E. Q. Gao and C. M. Liu, Dalton Trans., 2009, 2721 RSC.
-
(a) Q. Y. Chen, Y. Li, F. K. Zheng, W. Q. Zou, M. F. Wu, G. C. Guo, A. Q. Wu and J. S. Huang, Inorg. Chem. Commun., 2008, 11, 969 CrossRef CAS;
(b) Y. Li, G. Xu, W. Q. Zou, M. S. Wang, F. K. Zheng, M. F. Wu, H. Y. Zeng, G. C. Guo and J. S. Huang, Inorg. Chem., 2008, 47, 7945 CrossRef CAS.
- X. S. Wang, Y. Z. Tang, X. F. Huang, Z. R. Qu, C. M. Che, P. W. H. Chan and R. G. Xiong, Inorg. Chem., 2005, 44, 5278 CrossRef CAS.
-
(a) Y. C. Liang, M. C. Hong, R. Cao, W. P. Su, Y. J. Zhao and J. B. Weng, Polyhedron, 2001, 20, 2477 CrossRef CAS;
(b) C. H. Hu and U. Englert, Angew. Chem., Int. Ed., 2005, 44, 2281 CrossRef CAS.
-
CrystalClear, version 1.35; Software User's Guide for the Rigaku R–Axis, and Mercury and Jupiter CCD Automated X-ray Imaging System; Rigaku Molecular Structure Corporation: Utah, 2002 Search PubMed.
-
SHELXTL Reference Manual, version 5; Siemens Energy & Automation Inc.: Madison, WI, 1994 Search PubMed.
-
(a) C. Y. Su, A. M. Goforth, M. D. Smith, P. J. Pellechia and H. C. Loye, J. Am. Chem. Soc., 2004, 126, 3576 CrossRef CAS;
(b) X. M. Zhang, Coord. Chem. Rev., 2005, 249, 1201 CrossRef CAS;
(c) Y. Q. Sun, J. Zhang and G. Y. Yang, Chem. Commun., 2006, 1947 RSC.
- Q. Chu, G. X. Liu, Y. Q. Huang, X. F. Wang and W. Y. Sun, Dalton Trans., 2007, 4302 RSC.
-
(a) I. Abrahams, M. A. Malik, M. Motevalli and P. O'Brien, J. Chem. Soc., Dalton Trans., 1995, 1043 RSC;
(b) Y. J. Tang, W. S. Kassel, L. N. Zakharov, A. L. Rheingold and R. A. Kemp, Inorg. Chem., 2005, 44, 359 CrossRef CAS;
(c) C. Redshaw and M. R. J. Elsegood, Angew. Chem., Int. Ed., 2007, 46, 7453 CrossRef CAS;
(d) D. C. Wen, S. X. Liu and J. Ribas, Polyhedron, 2007, 26, 3849 CrossRef CAS;
(e) M. Jarenmark, S. Kappen, M. Haukkab and E. Nordlander, Dalton Trans., 2008, 993 RSC.
-
(a) D. L. Long, A. J. Blake, N. R. Champness, C. Wilson and M. Schröder, Angew. Chem., Int. Ed., 2001, 40, 2443 CrossRef;
(b) W. Q. Zou, M. S. Wang, Y. Li, A. Q. Wu, F. K. Zheng, Q. Y. Chen, G. C. Guo and J. S. Huang, Inorg. Chem., 2007, 46, 6852 CrossRef CAS.
- D. T. Vodak, M. E. Braun, J. Kim, M. Eddaoudi and O. M. Yaghi, Chem. Commun., 2001, 2534 RSC.
-
(a) Y. Cui, D. Long, W. D. Chen and J. S. Huang, Acta Crystallogr., Sect. C: Cryst. Struct. Commun., 1998, 54, 1605 CrossRef;
(b) S. G. Roh, J. U. Yoon and J. H. Jeong, Polyhedron, 2004, 23, 2063 CrossRef CAS.
-
(a) N. L. Rosi, J. Kim, M. Eddaoudi, B. Chen, M. O'Keeffe and O. M. Yaghi, J. Am. Chem. Soc., 2005, 127, 1504 CrossRef CAS;
(b) J. Y. Zhang, Y. Q. Wang, H. Q. Peng, A. L. Cheng and E. Q. Gao, Struct. Chem., 2008, 19, 535 CrossRef CAS.
-
(a) J. Zhang, Y. G. Yao and X. H. Bu, Chem. Mater., 2007, 19, 5083 CrossRef CAS;
(b) J. Yang, J. F. Ma, Y. Y. Liu and S. R. Batten, CrystEngComm, 2009, 11, 151 RSC.
-
(a) S. Leininger, B. Olenyuk and P. J. Stang, Chem. Rev., 2000, 100, 853 CrossRef CAS;
(b) B. Moulton and M. J. Zaworotko, Chem. Rev., 2001, 101, 1629 CrossRef CAS;
(c) T. J. Prior, D. Bradshaw, S. J. Teat and M. J. Rosseinsky, Chem. Commun., 2003, 500 RSC.
-
(a) A. Rodríguez-Diéguez and E. Colacio, Chem. Commun., 2006, 4140 RSC;
(b) Y. B. Lu, M. S. Wang, W. W. Zhou, G. Xu, G. C. Guo and J. S. Huang, Inorg. Chem., 2008, 47, 8935 CrossRef CAS.
-
(a) S. F. Palopoli, S. J. Geib, A. L. Rheingold and T. B. Brill, Inorg. Chem., 1988, 27, 2963 CrossRef CAS;
(b) E. O. John, R. D. Willett, B. Scott, R. L. Kirchmeier and J. M. Shreeve, Inorg. Chem., 1989, 28, 893 CrossRef CAS;
(c) X. G. Zhou, Z. E. Huang, R. F. Cai, L. X. Zhang, X. F. Hou, X. J. Feng and X. Y. Huang, J. Organomet. Chem., 1998, 563, 101 CrossRef CAS;
(d) M. Kotera, Y. Sekioka and T. Suzuki, Inorg. Chem., 2008, 47, 3498 CrossRef CAS.
-
(a) D. Li, T. Wu, X. P. Zhou, R. Zhou and X. C. Huang, Angew. Chem., Int. Ed., 2005, 44, 4175 CrossRef CAS;
(b) X. M. Zhang, R. Q. Fang and H. S. Wu, J. Am. Chem. Soc., 2005, 127, 7670 CrossRef CAS;
(c) X. L. Wang, C. Qin, E. B. Wang, Z. M. Su, Y. G. Li and L. Xu, Angew. Chem., Int. Ed., 2006, 45, 7411 CrossRef CAS;
(d) J. H. Jia, X. Lin, C. Wilson, A. J. Blake, N. R. Champness, P. Hubberstey, G. Walker, E. J. Cussena and M. Schrőder, Chem. Commun., 2007, 840 RSC.
-
(a) D. M. Ciurtin, M. D. Smith and H. C. zur Loye, Chem. Commun., 2002, 74 RSC;
(b) N. L. Rosi, M. Eddaoudi, J. Kim, M. O'Keeffe and O. M. Yaghi, Angew. Chem., Int. Ed., 2002, 41, 284 CrossRef;
(c) Y. Li, J. P. Zou, W. Q. Zou, F. K. Zheng, G. C. Guo, C. Z. Lu and J. S. Huang, Inorg. Chem. Commun., 2007, 10, 1026 CrossRef CAS.
-
(a) J. J. Wang, C. S. Liu, T. L. Hu, Z. Chang, C. Y. Li, L. F. Yan, P. Q. Chen, X. H. Bu, Q. Wu, L. J. Zhao, Z. Wang and X. Z. Zhang, CrystEngComm, 2008, 10, 681 RSC;
(b) W. G. Lu, L. Jiang, X. L. Feng and T. B. Lu, Cryst. Growth Des., 2008, 8, 986 CrossRef CAS.
-
(a) X. Xue, X. S. Wang, L. Z. Wang, R. G. Xiong, B. F. Abrahams, X. Z. You, Z. L. Xue and C. M. Che, Inorg. Chem., 2002, 41, 6544 CrossRef CAS;
(b) Q. Ye, Y. H. Li, Y. M. Song, X. F. Huang, R. G. Xiong and Z. L. Xue, Inorg. Chem., 2005, 44, 3618.
-
(a) J. Perles, M. Iglesias, M. Á. Martín-Luengo, M. Á. Monge, C. Ruiz-Valero and N. Snejko, Chem. Mater., 2005, 17, 5837 CrossRef CAS;
(b) R. Q. Zou, R. Q. Zhong, M. Du, T. Kiyobayashia and Q. Xu, Chem. Commun., 2007, 2467 RSC.
|
This journal is © The Royal Society of Chemistry 2010 |