DOI:
10.1039/C0AY00575D
(Minireview)
Anal. Methods, 2010,
2, 1874-1879
Extraction of environmental pollutants using magnetic nanomaterials
Received
23rd September 2010
, Accepted 3rd November 2010
First published on
16th November 2010
Abstract
Magnetic nanomaterials are particularly useful for extracting and enriching a large volume of target analytes because they provide high surface-to-volume ratio, easy surface modification, and strong magnetism. This review summarizes the recent advance in synthesis, modification, and application of magnetic nanomaterials for the preconcentration of environmental pollutants. The extraction of environmental pollutants with magnetic nanomaterials is generally based on hydrophobic interaction, electrostatic attraction, and/or covalent bonding formation. Environmental pollutants adsorbed on the surface of nanomaterials are released by exchanging ligands, adjusting pH, or adding organic solvent. The liberated environmental pollutants are commonly detected by gas chromatography, high-performance liquid chromatography, and inductively coupled plasma-optical emission spectrometry. The basic principle of each nanomaterial and its advantages for the extraction of environmental pollutants are discussed in this review.
1. Introduction
Nanomaterials have novel optical, electrical, and magnetic properties that make them attractive in diverse fields, such as biosensors,1 separation science,2 nanomedicine,3 and cell imaging.4 Moreover, nanomaterials act as adsorbents for enriching target analytes from a complex matrix. Compared to liquid–liquid extraction5 and solid phase microextraction,6 nanomaterials not only exhibit high surface area-to-volume ratios, but also are easily chemically functionalized. Thus, recently, numerous nanomaterials modified different types of surface ligands, such as multi-walled carbon nanotubes,7–11 Au nanoparticles (NPs),12–14 TiO2 NPs,15,16 and Fe3O4 nanoparticles (NPs)17–42 have been developed for the extraction and enrichment of environmental pollutants. The binding types of nanomaterials with environmental pollutants include electrostatic interaction, covalent bonding formation, and hydrophobic adsorption. In contrast to other nanomaterials, Fe3O4 NPs possess strong magnetism and thereby are readily isolated from sample solutions by an external magnetic field without additional centrifugation or filtration.
This paper reviews the development of magnetic nanomaterials utilized for the extraction of environmental pollutants, including heavy metal ions, polycyclic aromatic hydrocarbons (PAH), phenolic compounds, pesticides, phthalates, drugs, and others. We also discuss the effects of surface ligand, nanomaterial concentration, eluent concentration, and eluent type on the extraction efficiency. Table 1 summarizes the extraction of environmental pollutants using different types of magnetic nanomaterials.
Table 1 Comparison of different types of magnetic nanomaterials
Materiala |
Additiveb |
Eluentc |
Analyte/sample volumed |
Detectione |
LOD |
Reference |
MPTMS, 3-mercaptopropyltrimethoxysilane; CTAB, cetyltrimethylammonium bromide; CPC, cetylpyridinium chloride; BA, barium alginate; β-CD, β-cyclodextrin, ; C16minBr, 1-hexadecyl-3-methylimidazolium bromide; OTMABr, octadecyltrimethylammonium bromide; SDS, sodium dodecyl sulfate.
PAN, 1-(2-pyridilazo)-2-naphthol.
THF, tetrahydrofuran; ACN, acetonitrile.
PFC, Perfluorinated compounds.
FL, fluorescence; CE-ECL, capillary electrophoresis–electrochemiluminescence.
|
MPTMS-capped Fe3O4@SiO2 NPs |
No |
HCl, thiourea |
Metal ions/50 mL |
ICP-MS |
24–107 pg/L |
17
|
MPTMS-capped Fe3O4 NPs |
No |
HCl, K2Cr2O7 |
Tl4+/160 mL |
ICP-MS |
0.079 ng L−1 |
18
|
Bismuthiol-capped Fe3O4@SiO2 NPs |
No |
HNO3 |
Metal ions/100 mL |
ICP-OES |
43–85 pg/mL |
19
|
Decanoic acid-capped Fe3O4 NPs |
PAN |
HCl |
Metal ions/50 mL |
ICP-OES |
0.2–0.8 μg L−1 |
20
|
CTAB-coated Fe3O4 NPs |
CTAB |
ACN |
Phenolic compounds/800 mL |
HPLC-FL |
12–34 ng L−1 |
21
|
CTAB-coated Fe3O4 NPs |
CTAB |
CH3OH |
Phenolic compounds/700 mL |
HPLC-UV |
0.11–0.15 μg L−1 |
22
|
CPC-coated Fe3O4 NPs |
CPC |
ACN |
Phenolic compounds/800 mL |
HPLC-FL |
7–20 ng L−1 |
23
|
β-CD-capped Fe3O4@SiO2 NPs |
No |
CH3OH |
Bisphenol A/250 mL |
HPLC-UV |
20 ng L−1 |
25
|
Magnetic MIP |
No |
CH3OH |
Bisphenol A/200 mL |
HPLC-UV |
14 ng L−1 |
26
|
C18-capped Fe3O4 microsphere |
No |
n-hexane |
PAH/20 mL |
GC-MS |
0.8–36 μg L−1 |
27
|
Tetradecanoate-coated Fe3O4 NPs |
No |
THF, ACN |
PAH/350 mL |
HPLC-FL |
0.1–0.25 ng L−1 |
28
|
Hydrophobic Fe3O4 NPs |
1-octanol |
ACN |
PAH/20 mL |
GC-MS |
11.7–61.4 ng L−1 |
29
|
BA caged Fe3O4@C18 NPs |
No |
ACN |
PAH/500 mL |
HPLC-FL |
3–5 ng L−1 |
30
|
C16minBr-coated Fe3O4 NPs |
C16minBr |
ACN |
PAH/300 mL |
HPLC-FL |
0.33–8.33 ng L−1 |
31
|
Decanoic acid-capped Fe3O4 NPs |
No |
HCl |
Pesticides/400 mL |
HPLC-MS |
0.01–0.03 μg L−1 |
32
|
Al2O3-coated Fe3O4 NPs |
No |
Na4P2O7 |
Pesticides/5 mL |
CE-ECL |
0.3, 30 ng mL−1 |
34
|
Chitosan-coated Fe3O4@C18 NPs |
No |
ACN |
Phthalate esters/500 mL |
HPLC-UV |
12.3–36.4 ng L−1 |
35
|
Alginate–polymer-caged Fe3O4-titanate@C18 nanotubes |
No |
ACN |
Phthalate esters/500 mL |
HPLC-FL |
11–46 ng L−1 |
36
|
OTMABr-coated Fe3O4 NPs |
OTMABr |
CH3OH |
Sulfonamides/500 mL |
HPLC-UV |
24–33 ng L−1 |
37
|
SDS-coated Fe3O4@ Al2O3 NPs |
SDS |
CH3OH |
Timethoprim/500 mL |
HPLC-UV |
0.09 μg L−1 |
38
|
Fe3O4@ Al2O3 NPs |
No |
CH3COOH |
Sulfonamides/2 g |
LC-MS/MS |
0.37–6.74 ng g−1 |
39
|
Magnetic MIP |
No |
CH3OH |
Tetracycline antibiotics/2 g |
LC-MS/MS |
0.2 ng g−1 |
40
|
Chitosan-coated Fe3O4@C18 NPs |
No |
CH3OH, NH3 |
PFC/500 mL |
LC-MS/MS |
0.075–0.24 ng L−1 |
41
|
Bare Fe3O4 NPs |
NH4SCN |
NaOH |
Fluoride/250 mL |
Absorption |
0.015 μg mL−1 |
42
|
2. Extraction of environmental pollutants
2.1 Heavy metal ions
Monitoring the level of heavy metal ions in an aquatic ecosystem has received significant attention over many years because they are severe environmental pollutants and have adverse effects on human health. For example, Pb2+ is a potential neurotoxin that can cause chronic inflammation of the kidney and heart, inhibit brain development, and decrease nerve conduction velocity. Moreover, highly toxic Hg2+ can damage the brain, heart, kidney, stomach, and intestines. The maximum levels of Hg, Cd, As, Pb, Cr, and Cu ions in drinking water permitted by the United States Environmental Protection Agency (U.S. EPA) are 2, 5, 10, 15, 100, and 1300 μg L−1, respectively. The current methods for the analysis of heavy metal ions are based on the use of sensitive element-specific detectors, such as flame atomic absorption spectrometry (AAS), inductively coupled plasma-mass spectrometry (ICP-MS), and ICP-optical emission spectrometry (ICP-OES). Huang and Hu synthesized silica-coated Fe3O4 NPs and modified them with 3-mercaptopropyltrimethoxysilane (MPTMS).17 These kind of NPs contain thiol groups so that selective extraction of Cd2+, Cu2+, Hg2+, and Pb2+ is achieved through the formation of the metal ion–thiol complex. The adsorbed metal ions on the NP surface were liberated upon the addition of a solution containing 1 M HCl and 2% thiourea. ICP-MS was used to analyze the extracted metal ions. Under the optimal extraction conditions (pH, sample volume, and eluent), the limits of detection (LODs) for Cd2+, Cu2+, Hg2+, and Pb2+ were as low as 24, 92, 107, and 56 pg/L. The same group reported that MPTMS-modified Fe3O4 NPs were utilized as effective sorbent materials for extracting Te4+ in a wide pH range, but not for Te6+.18 In other words, this kind of NP is capable of separating a mixture of Te4+ and Te6+. The adsorbed Te4+ on the NP surface is released by adding a mixture of 2 M HCl and 0.03 M K2Cr2O7. The LOD of Te4+ was determined at down to 0.079 ng L−1 by combining NP-based extraction and ICP-MS. Along a similar line, Suleiman et al. modified silica-coated Fe3O4 NPs with bismuthiol for extracting Cr3+, Cu2+, and Pb2+ from environmental samples and determined the extracted metal ions by ICP-OES.19 Bismuthiol containing thiol and amine groups possesses strong affinity with Cr3+, Cu2+, and Pb2+ in the pH range of 3–8. The extraction of 100 mL sample volume with bismuthiol-coated magnetic NPs resulted in approximately 100-fold improvement in sensitivity. Without the silica coating, decanoic acid-capped Fe3O4 NPs can be directly employed for solid phase extraction of Cd2+, Co2+, Cr3+, Ni2+, Pb2+, and Zn2+ from water samples after these metal ions formed stable complexes with amine and 1-(2-pyridilazo)-2-naphthol.20 The adsorbed metal ions were detached from the NP surface using a solution containing HCl and propanol. The released metal ions were determined using flow injection coupled to ICP-OES.
2.2 Phenolic compounds
Phenolic compounds are commonly used in several industrial processes to manufacture chemicals such as pesticides, explosives, drugs, and dyes. Chlorinated phenols originate from chlorine treatment of drinking water. Photochemical reaction induces the formation of nitrophenols in the presence of vehicle exhausts. Because phenolic compounds are potentially carcinogenic, their maximum permitted level in drinking water by the U.S. EPA is 0.5 μg L−1. HPLC combined with UV, fluorescence, electrochemical, or MS detection has been developed for the determination of phenolic compounds. A preconcentration step is prerequisite for the analysis of phenolic compounds because their concentration is quite low in environmental water samples. Zhao et al. prepared cetyltrimethylammonium bromide (CTAB)-coated Fe3O4 NPs for the preconcentration of bisphenol A, 4-tert-octylphenol, and 4-n-nonylphenol in the presence of CTAB.21 CTAB molecules were self-assembled on the surface of Fe3O4 NPs through electrostatic interaction between the head group of CTAB and the oppositely charged group on the Fe3O4 NPs. When more CTAB molecules were present in the solution, the hydrophobic interaction between the hydrophobic tails of CTAB led to the formation of admicelles. Under this condition, the mixed hemimicelles consisting of hydrophobic hemimicelles and ionic admicelles were formed on the surface of Fe3O4 NPs. Thus, the adsorption of analytes could be driven by both hydrophobic interaction and electrostatic attraction. In the mixed hemimicelle region, the extraction efficiency remarkably increased with an increase in CTAB concentration. Fig. 1 illustrates the whole procedure for the extraction of phenolic compounds with CTAB-coated Fe3O4 NPs. A 800-fold improvement in sensitivity was achieved by extracting 800 mL of water samples with CATB-coated Fe3O4 NPs. These kind of NPs were also applied to the extraction of 2-chlorophenol, 2,4-dichlorophenol, 2,4,6-trichorophenol, and pentachlorophenol in the presence of CTAB.22 The extraction of 700 mL of water sample with CTAB-coated Fe3O4 NPs resulted in 700-fold improvement in sensitivity. The LODs of four chlorophenols ranged from 0.11 and 0.15 μg L−1. Zhao et al. found that the replacement of CTAB by cetylpyridium chloride in Fe3O4 NPs resulted in lower elution volume and more effective adsorption, thereby providing better sensitivity improvement.23 Zhao et al. also reported the synthesis of 11-mercaptoundecanoic acid (MUA) and 11-dodecanethiol (DDT)-functionalized core/shell Fe3O4/Au NPs for effective adsorption of bisphenol A, 4-tert-octylphenol, and 4-n-nonylphenol.24 DDT-modified Fe3O4/Au NPs exhibited strong hydrophobic interaction with phenolic compounds and their extraction efficiency was insensitive to pH change. By contrast, the adsorption capacity of 11-MUA-modified Fe3O4/Au NPs remarkably decreased above pH 10.0 because of repulsive electrostatic interaction between the negatively charged 11-MUA (pKa = 4.8) and phenolic compounds (the pKa of three phenolic compounds were all below 10.0). Ji et al. synthesized β-cyclodextrin-modified core/shell Fe3O4/SiO2 NPs for selective and rapid enrichment of bisphenol A.25 The extracted bisphenol A was eluted with methanol and then determined by HPLC with UV detection. The LOD was down to 20 ng L−1 for bisphenol A after the extraction of 250 mL sample volume. The extraction procedure was completed within 25 min. Ji et al. prepared magnetic molecularly imprinted polymer (MIP) using bisphenol A as a template, vinylpyridine as a functional monomer, ethylene glycol dimethacrylate as a crosslinker, and methacryloxypropyltrimethoxysilane-modified Fe3O4 NP as a support.26 Based on molecular recognition, selective extraction of bisphenol in environmental water and milk samples was accomplished using magnetic MIP prior to the analysis by HPLC with UV detection. The LODs of bisphenol A were found to be 14 ng L−1 and 160 ng L−1 in water and milk, respectively.
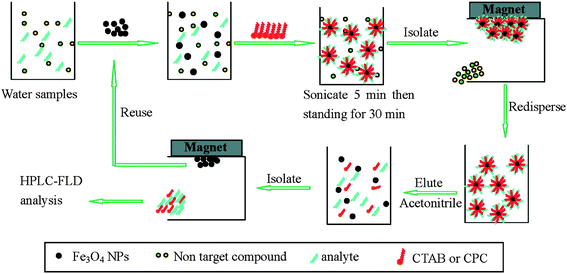 |
| Fig. 1 An illustration of the steps involved in extracting phenolic compounds from environmental water by using CTAB/nanomagnets Fe3O4 sorbents. (From ref. 21, with kind permission of American Chemical Society). | |
2.3 PAHs
PAH is a complex class of condensed multiring benzenoid compounds originating from countless natural process and human activities. The U.S. EPA lists the following 16 as “Consent Decree” priority pollutants: benz[a]anthracene, pyrene, benzo[b]fluoranthene, benzo[k]fluoranthene, naphthalene, fluorene, benzo[a]pyrene, dibenzo[a,h]anthracene, indeno[1,2,3-cd]pyrene, acenaphthylene, acenaphthene, phenanthrene, anthracene, fluoranthene, chrysene, and benzo[g,h,i]perylene. The methods currently available for the separation of PAH include gas chromatography (GC) and high performance liquid chromatography (HPLC). The extraction of PAH is an important step to reduce the matrix effect and enahnce their sensitivity. Liu et al. described the use of C18-functionalized Fe3O4 microspheres for extracting 16 PAH from tap water through hydrophobic interaction.27 The microspheres possess a high magnetic saturation and thus are easily manipulated by an external magnetic field. By the combination of Fe3O4 microsphere-based extraction and GC-MS, the LODs of 16 PAH were reached in the range from 0.8 to 36 μg L−1. Ballesteros–Gómez and Rubio compared different types of alkyl carboxylate-coated magnetic NPs to enrich PAH from surface water. These extracted PAHs were analyzed by HPLC with fluorescence detection.28 Tetradecanoate-coated magnetic NPs were selected because of their better extraction recovery and stability. The limits of quantification (LOQs) for 7 PAH ranged from 0.2 to 0.5 ng L−1, which are below the maximum permissible limits (3.8 ng L−1) of PAH in surface and ground water permitted by the U.S. EPA. Shi and Lee recently reported a two-step extraction approach based on the integration of dispersive liquid–liquid microextraction and microsolid-phase extraction (Fig. 2).29 1-Octanol, which is immiscible with water, was employed for the extraction and enrichment of PAH from an aqueous solution. After liquid–liquid extraction, magnetic NPs retrieved 1-octanol from an aqueous solution via hydrophobic interaction. A small amount of acetonitrile was introduced to release 1-octanol and PAH from the NP surface. The obtained supernatant was directly detected by the fast GC-MS. This method provided the LOQs ranging from 0.04 to 0.21 ng L−1. Zhang et al. modified octadecyl-functionalized Fe3O4 NPs with hydrophilic alginate polymers to improve their hydrophilicity and dispersibility in an aqueous solution.30 The formed NPs were applied to the extraction and enrichment of PAH from a large volume of environmental water samples prior to the analysis by HPLC with fluorescence detection. Selective extraction of PAH was successfully achieved using this kind of NPs because the alginate polymers can block the access of humic acid and colloid through electrostatic repulsion and size exclusion. Zhang et al. recently used ionic liquid-coated Fe3O4 NPs for the extraction and preconcentration of PAH in water and soil samples, followed by HPLC-fluorescence analysis.31 Compared to 1-decyl-3-methylimidazolium bromide and CTAB-coated Fe3O4 NPs, 1-hexadecyl-3-methylimidazolium bromide (C16minBr)-coated Fe3O4 NPs provided better extraction efficiency in the mixed hemimicelles region. When large volume (300 mL) of water sample was extracted with a small amount of C16minBr (50 mg) and Fe3O4 NPs (80 mg) at pH 10.0, the LODs for pyrene, benzo[a]anthracene, and benzo[a]pyrene (BaP) were found to be 8.33, 1.67, and 0.33 ng L−1, respectively.
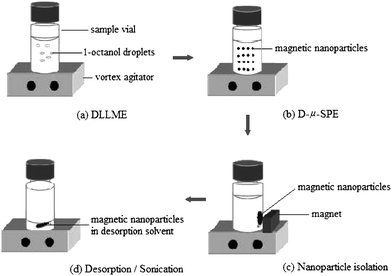 |
| Fig. 2 The combination of dispersive liquid–liquid microextraction (DLLME) and microsolid-phase extraction (D-μ-SPE) extraction for the enrichment of PAH. (From ref. 29, with kind permission of American Chemical Society). | |
Modern agricultural production in many countries relies heavily on the use of pesticides, and thus they are present in soil, ground and surface water, and food. Some pesticides can cause adverse effects to human health. According to the European Union Directive on Water Quality, the maximum permissible concentration of each and total pesticides in drinking water are 0.1 and 0.5 μg L−1, respectively. Song et al. prepared superparamagnetic Fe3O4 NPs for extracting triazine pesticides from lake water.32 When Fe3O4 NPs were added to 400 mL sample, the capture of triazine pesticides was completed within 10 min through electrostatic attraction. After extraction and collection, 10 M HCl was added to dissolve Fe3O4 NPs. The obtained solution was neutralized with NaOH and then detected by HPLC-MS. The combination of Fe3O4 NPs and HPLC-MS provided low LOD (0.01–0.03 ng mL−1) and large linear range (0.03–50 ng mL−1). Shen et al. described the use of C18-functionalized Fe3O4 NPs for cleanup and enrichment of 14 kinds of organophosphorous pesticides.33 The extracted organophosphorous pesticides were determined by GC with a nitrogen/phosphorus detector. Hsu and Wang have recently reported the synthesis alumina-coated Fe3O4 NPs for selectively capturing glyphosate and its major metabolite aminomethylphosphonic acid (AMPA).34 Because Al2O3-coated Fe3O4 NPs are capable of selectively binding phosphate-containing compounds, the extraction of 5 mL of glyphosate and AMPA was efficient (within 5 min) and selective. The adsorbed glyphosate and AMPA on the NP surface were liberated by ligand exchange between their phosphate groups and Na4P2O7. These released analytes were then determined by capillary electrophoresis with electrochemiluminescence detection. Improvements in sensitivity of 460- and 64-fold were reported for glyphosate and AMPA. The LODs of glyphosate and AMPA were 0.3 and 30 ng mL−1, respectively.
2.5 Phthalates
Phthalates are extensively used as polymer additives in many consumer products, such as plastics and cosmetics, resulting in the ubiquitous presence of these chemicals in the environment. They can migrate into food, water, soil, marine ecosystem, human urine, and human milk. Some phthalates can cause endocrine system disorders, have carcinogenic effects, and exhibit adverse reproductive effects. Current approaches to detecting phthalates include HPLC coupled with UV and fluorescence detection. Zhang et al. synthesized C18-functionalized Fe3O4 NPs, followed by coating a layer of chitosan–tripolyphosphate polymer on the surface.35 Hydrophobic C18 ligands enabled Fe3O4 NPs to interact with phthalate ester, while the porosity and basket-like structure of chitosan–tripolyphosphate polymer excluded macromolecules, such as humic acid. When 500 mL of water samples was concentrated to 0.5 mL, the LODs were found to be 12.3, 18.7, 36.4, and 15.6 ng L−1 for di-n-propyl phthalate, di-n-butyl phthalate, dicyclohexyl phthalate, and di-n-octylphthalate, respectively. By extracting the analytes from 500 mL of water samples, the LODs and recoveries for seven perfluorinated compounds ranged from 0.075 to 0.24 ng L−1 and from 63% to 109%, respectively. Niu et al. prepared the composites of C18-functionalized Fe3O4 NPs and titanate nanotubes, and coated them with alginate polymer.36 Because the function of the as-prepared composites was similar to that of chitosan–tripolyphosphate/C18 coated-capped Fe3O4 NPs, they are capable of preconcentrating phthalates from river water. When the incubation time, adsorbent amount, eluent volume, and sample volume were optimized to be 40 min, 80 mg, 6 mL, and 500 mL, the LODs of phthalates ranging from 11–46 ng L−1 was achieved.
Because antibiotics, such as sulfonamides, tetracyclines, quinolones, macrolides, and trimethoprim, are frequently used as feed additives to promote animal growth and as therapeutic agents to cure human/animal diseases, they are considered ubiquitous environmental contaminants. HPLC is one of the methods most used for the analysis of antibiotics when it is coupled with UV, fluorescence, and MS. Sun et al. showed that cationic octadecyltrimethylammonium bromide (OTMABr) formed mixed hemimicelles on the NP surface enabled Fe3O4 NPs to extract four sulfonamides.37 Through the hydrophobic interaction between mixed hemimicelles and analytes, the adsorption of sulfonamides onto OTMABr-coated Fe3O4 NPs gradually increased with increasing OTMABr concentration in the mixed hemimicelle region. When 10 mL of 10 mg mL−1 Fe3O4 NPs and 100 mg OTMABr were added to 500 mL of water samples at pH 9.0, the LODs of sulfonamides detected were at levels down to 0.024–0.033 μg L−1. Based on this concept, Sun et al. used sodium dodecyl sulfate (SDS)-coated core/shell Fe3O4/Al2O3 NPs for extracting trimethioprim through hydrophobic and electrostatic interaction prior to HPLC-UV detection.38 The coating of alumina was to avoid Fe3O4 NPs dissolving in acidic solution. The extraction efficiency was highly dependent on SDS concentration, solution pH, extraction volume, and desorption solvent. After 500 mL of trimethioprim was extracted with 0.1 g Fe3O4/Al2O3 NPs and 120 mg SDS (pH 2.0), the LOD and linear range for trimethioprim was found to be 0.09 μg L−1 and 0.24–30 μg mL−1. Additionally, in the absence of surfactant, core/shell Fe3O4/Al2O3 NPs provided higher adsorption capacity for sulfonamides when ultrasonic-assisted extraction was applied instead of magnetic stirring extraction.39 The adsorption of sulfonamides on the NP surface was driven by hydrophobic interaction. Sulfonamides desorbed from the NP surface were determined by HPLC-MS. When extraction time, adsorbent amount, and eluent volume were optimized to be 8 min, 100 mg, and 2 mL (0.5% acetic acid), the LODs of sulfonamides were as low as 0.37–6.74 ng g−1. The analysis of sulfonamides in different soil was accomplished using this kind of NP in combination with HPLC-MS. Chen et al. used MIP for selective preconcentration of oxytetracycline and its analogues from egg and tissue samples, followed by HPLC-MS/MS analysis.40 Magnetic MIP was synthesized using hydrophobic Fe3O4 NP as a magnetically susceptible component, oxytetracycline as a template molecule, methacrylic acid as a functional monomer, as well as styrene and divinylbenzene as polymer matrix components. Compared to other MIP-based methods for the extraction of tetracycline and oxytetracycline, magnetic MIP provides distinct advantages, including short extraction time, less solvent consumption, good reusability, and good extraction efficiency. The LODs of oxytetracycline and its analogues in egg and tissue were in the range of 0.06–0.16 ng g−1.
2.7 Other environmental pollutants
In addition to the above-mentioned environmental pollutants, perfluorinated compounds and fluoride ions are also hazardous to human health and environment. Zhang et al. prepared C18-functionalized Fe3O4 NPs and coated them with a layer of hydrophilic chitosan–tripolyphosphate polymer through ionotropic gelation. The formed chitosan-coated C18-functionalized Fe3O4 NPs were employed to concentrate anionic perfluorinated compounds through hydrophobic interaction with C18 ligands and electrostatic attraction with cationic chitosan.41 The coating of chitosan–tripolyphosphate polymer improved the dispersion of C18-functionalized Fe3O4 NPs and suppressed the adsorption of macromolecules on the NP surface by size exclusion or electrostatic interaction. Perfluorinated compounds were extracted from 500 mL of several environmental water samples using Fe3O4 NPs at pH 3.0. After being eluted from the adsorbent with 12 mL of methanol containing 0.28% ammonia, the analytes were further concentrated to 0.5 mL with a nitrogen flow. Finally, the concentrated analytes were analyzed by HPLC-ESI-MS/MS analysis. Under this extraction condition, the LODs of perfluorinated compounds were markedly improved, ranging between 0.075 and 0.24 ng L−1. Without chromatographic separation, Parham and Rahbar combined Fe3O4 NP-based extraction with visible spectrometry for the determination of fluoride ions in various water samples.42 Extraction of fluoride ions is based on the formation of FeF63− complex on the NP surface. The fluoride ions were released upon the addition of NaOH. The liberated fluoride ions were detected by the replacement reaction between colored Fe–SCN complex and fluoride ions.
Conclusions
The studies mentioned above demonstrate that Fe3O4 NPs are an appealing alternative for the extraction and enrichment of environmental pollutants because of their high surface-to-volume ratio, ease of modification, and strong magnetism. However, there are still numerous nanomaterials that are not utilized for the extraction of environmental pollutants. For example, analytes containing thiol (SH) or amino (NH2) groups are adsorbed spontaneously onto the surface of Au NPs.43 Thus, in the opinion of the authors, the extraction of thiol-containing degradation products of V-type nerve agents could be achieved using a composite of Au NPs and Fe3O4 NPs.44 Surface-to-volume ratio of nanomaterials increases with reducing particle size, therefore the extraction efficiency of an environmental pollutant may be remarkably improved using magnetic NPs of small size and narrow size distribution.
Acknowledgements
We would like to thank National Science Council (NSC 98-2113-M-110-009-MY3) and National Sun Yat-sen University-Kaohsiung Medical University Joint Research Center for the financial support of this study.
References
- R. Wilson, Chem. Soc. Rev., 2008, 37, 2028–2045 RSC.
- C. Nilsson, S. Birnbaum and S. Nilsson, J. Chromatogr., A, 2007, 1168, 212–224 CrossRef CAS.
- E. Boisselier and D. Astruc, Chem. Soc. Rev., 2009, 38, 1759–1782 RSC.
- A. Louie, Chem. Rev., 2010, 110, 3146–3195 CrossRef CAS.
- C. Mahugo Santana, Z. Sosa Ferrera, M. Esther Torres Padron and J. Juan Santana Rodriguez, Molecules, 2009, 14, 298–320 Search PubMed.
- H. Kataoka, Anal. Bioanal. Chem., 2010, 396, 339–364 CrossRef CAS.
- D. Du, M. Wang, J. Zhang, J. Cai, H. Tu and A. Zhang, Electrochem. Commun., 2008, 10, 85–89 CrossRef CAS.
- Y. Song, S. Zhao, P. Tchounwou and Y. M. Liu, J. Chromatogr., A, 2007, 1166, 79–84 CrossRef CAS.
- J. Muñoz, M. Gallego and M. Valcárcel, Anal. Chem., 2005, 77, 5389–5395 CrossRef CAS.
- C. Basheer, A. A. Alnedhary, B. S. Rao, S. Valliyaveettil and H. K. Lee, Anal. Chem., 2006, 78, 2853–2858 CrossRef CAS.
- S. Wang, P. Zhao, G. Min and G. Fang, J. Chromatogr., A, 2007, 1165, 166–171 CrossRef CAS.
- H. Wang and A. D. Campiglia, Anal. Chem., 2008, 80, 8202–8209 CrossRef CAS.
- H. Wang, S. Yu and A. D. Campiglia, Anal. Biochem., 2009, 385, 249–256 CrossRef CAS.
- K. Leopold, M. Foulkes and P. J. Worsfold, Anal. Chem., 2009, 81, 3421–3428 CrossRef CAS.
- E. Vssileva, K. Hadjiivanov, T. Stoychev and C. Daiev, Analyst, 2000, 125, 693–698 RSC.
- P. Liang, T. Shi, H. Lu, Z. Jiang and B. Hu, Spectrochim. Acta, Part B, 2003, 58, 1709–1714 CrossRef.
- C. Huang and B. Hu, Spectrochim. Acta, Part B, 2008, 63, 437–444 CrossRef.
- C. Huang and B. Hu, J. Sep. Sci., 2008, 31, 760–767 CrossRef CAS.
- J. S. Suleiman, B. Hu, H. Peng and C. Huang, Talanta, 2009, 77, 1579–1583 CrossRef CAS.
- M. Faraji, Y. Yamini, A. Saleh, M. Rezaee, M. Ghambarian and R. Hassani, Anal. Chim. Acta, 2010, 659, 172–177 CrossRef CAS.
- X. Zhao, Y. Shi, Y. Cai and S. Mou, Environ. Sci. Technol., 2008, 42, 1201–1206 CrossRef CAS.
- J. Li, X. Zhao, Y. Shi, Y. Cai, S. Mou and G. Jiang, J. Chromatogr., A, 2008, 1180, 24–31 CrossRef CAS.
- X. Zhao, Y. Shi, Y. Cai and G. Jiang, J. Chromatogr., A, 2008, 1188, 140–147 CrossRef CAS.
- X. Zhao, Y. Cai, T. Wang, Y. Shi and G. Jiang, Anal. Chem., 2008, 80, 9091–9096 CrossRef CAS.
- Y. Ji, X. Liu, M. Guan, C. Zhao, H. Huang, H. Zhang and C. Wang, J. Sep. Sci., 2009, 32, 2139–2145 CrossRef CAS.
- Y. Ji, J. Yin, Z. Xu, C. Zhao, H. Huang, H. Zhang and C. Wang, Anal. Bioanal. Chem., 2009, 395, 1125–1133 CrossRef CAS.
- Y. Liu, H. Li and J. M. Lin, Talanta, 2009, 77, 1037–1042 CrossRef CAS.
- A. Ballesteros-Gomez and S. Rubio, Anal. Chem., 2009, 81, 9012–9020 CrossRef CAS.
- Z. G. Shi and H. K. Lee, Anal. Chem., 2010, 82, 1540–1545 CrossRef CAS.
- S. Zhang, H. Niu, Y. Cai and Y. Shi, Anal. Chim. Acta, 2010, 665, 167–175 CrossRef CAS.
- Q. Zhang, F. Yang, F. Tang, K. Zeng, K. Wu, Q. Cai and S. Yao, Analyst, 2010, 135, 2426–2433 RSC.
- Y. Song, S. Zhao, P. Tchounwou and Y. M. Liu, J. Chromatogr., A, 2007, 1166, 79–84 CrossRef CAS.
- H.-Y. Shen, Y. Zhu, X.-E. Wen and Y.-M. Zhuang, Anal. Bioanal. Chem., 2007, 387, 2227–2237 CrossRef CAS.
- C. C. Hsu and C. W. Whang, J. Chromatogr., A, 2009, 1216, 8575–8580 CrossRef CAS.
- X. L. Zhang, H. Y. Niu, S. X. Zhang and Y. Q. Cai, Anal. Bioanal. Chem., 2010, 397, 791–798 CrossRef CAS.
- H. Niu, S. Zhang, X. Zhang and Y. Cai, ACS Appl. Mater. Interfaces, 2010, 2, 1157–1163 CrossRef CAS.
- L. Sun, L. Chen, X. Sun, X. Du, Y. Yue, D. He, H. Xu, Q. Zeng, H. Wang and L. Ding, Chemosphere, 2009, 77, 1306–1312 CrossRef CAS.
- L. Sun, C. Zhang, L. Chen, J. Liu, H. Jin, H. Xu and L. Ding, Anal. Chim. Acta, 2009, 638, 162–168 CrossRef CAS.
- L. Sun, X. Sun, X. Du, Y. Yue, L. Chen, H. Xu, Q. Zeng, H. Wang and L. Ding, Anal. Chim. Acta, 2010, 665, 185–192 CrossRef CAS.
- L. Chen, J. Liu, Q. Zeng, H. Wang, A. Yu, H. Zhang and L. Ding, J. Chromatogr., A, 2009, 1216, 3710–3719 CrossRef CAS.
- X. Zhang, H. Niu, Y. Pan, Y. Shi and Y. Cai, Anal. Chem., 2010, 82, 2363–2371 CrossRef CAS.
- H. Parham and N. Rahbar, Talanta, 2009, 80, 664–669 CrossRef CAS.
- C.-W. Chang and W.-L. Tseng, Anal. Chem., 2010, 82, 2696–2702 CrossRef CAS.
- K. A. Joshi, M. Prouza, M. Kum, J. Wang, J. Tang, R. Haddon, W. Chen and A. Mulchandani, Anal. Chem., 2006, 78, 331–336 CrossRef CAS.
|
This journal is © The Royal Society of Chemistry 2010 |
Click here to see how this site uses Cookies. View our privacy policy here.