DOI:
10.1039/C0AY00418A
(Paper)
Anal. Methods, 2010,
2, 1803-1809
A comparative study of selected sorbents for sampling of aromatic VOCs from indoor air
Received
30th June 2010
, Accepted 24th August 2010
First published on
24th September 2010
Abstract
Indoor air can become polluted with VOCs, and understanding the factors which affect adsorption of VOCs from indoor air is important for: (i) the accurate measurement of VOCs, and (ii) to apply mitigation strategies when high analyte concentrations are measured. In this study four VOCs (toluene, ethylbenzene, cumene and dichlorobenzene) were generated as a constant and controlled polluted air stream of VOCs from a dynamic atmospheric chamber. The effects of relative humidity, and sampling flow rate, on adsorption onto Tenax TA and the relatively new silica adsorbents SBA-15 or MCM-41 were studied. Air samples were collected and analyzed by thermal desorption followed by GC/MS. All sorbents were shown to be affected by changing the RH conditions from 25 to 80% RH, and sampling flow rates from 25 to 200 cm3 min−1, even when pollutant concentrations and sampled air volumes remained consistent. Although further work is required to examine the effect of the full RH range on scavenging potential, in this study Tenax TA was shown to provide best performance in high RH conditions whereas silica sorbents were more effective at low RH. Moreover it was shown that to provide accurate measurements in the field (e.g., when humidity conditions are fixed) it is suggested that Tenax TA is the preferred sorbent of choice as the masses of VOCs collected were less affected by changing the sampling flow rates.
1 Introduction
Volatile organic compounds (VOCs) are an important class of indoor air pollutants due to the potentially serious health effects from long-term exposure to low pollutant concentrations.1 The sources of VOCs commonly include building materials and products used in the household.2 Since the 1980s, several studies focused on measuring VOC concentrations in various types of buildings.3–8 Several hundred VOCs have been detected in indoor air and Salthammer9 gave a complete description for those VOCs, and their concentrations, at different places. Indeed monoaromatic hydrocarbons (MAHCs), including benzene, toluene, ethylbenzene, xylene, cumene and dichlorobenzene also form an important group of air pollutants in urban areas.10
The nature of the material used to adsorb pollutants from indoor air depends on the VOC mix; a list of sorbents commonly used in active indoor air sampling campaigns is given in Table 1. In all reported literature Tenax TA (poly-2,6-diphenylphenylene oxide), with high thermal stability (up to 350 °C), is the most often used, and best evaluated sorbent, for VOCs sampling,14 thus it tends to be the default choice for most research groups monitoring indoor air.
Table 1 Sorbents used to collect VOCs11–13
Sorbent |
Compounds sampled |
Main advantages and disadvantages |
Can be used (mainly low recoveries).
|
Tenax TA |
–Most non-polar VOCs |
–Low background |
–Terpenes |
–Well investigated |
–Slightly polar VOCs |
–Some decomposition |
–Aldehydes > C5 |
products (benzaldehyde, |
–Acids > C3a |
acetophenone) |
![[thin space (1/6-em)]](https://www.rsc.org/images/entities/char_2009.gif) |
Carbotrap |
–Most non-polar VOCs |
–Low background |
–Slightly polar VOCs |
–Reactions of some |
|
compounds (i.e. |
|
aldehydes, terpenes) |
![[thin space (1/6-em)]](https://www.rsc.org/images/entities/char_2009.gif) |
Activated |
–Most non-polar VOCs |
–High capacity |
carbon |
–Slightly polar VOCs |
–Reactions of some |
|
|
compounds |
![[thin space (1/6-em)]](https://www.rsc.org/images/entities/char_2009.gif) |
Porapak Q |
–Most non-polar VOCs |
–High background |
–Slightly polar VOCs |
–Low thermal stability |
![[thin space (1/6-em)]](https://www.rsc.org/images/entities/char_2009.gif) |
Organic |
–Polar and non-polar |
–Water adsorption |
molecular sieves |
VOCs |
|
(e.g. Carboxen |
|
|
563, 564, |
|
|
Carbosieve- |
|
|
S 111) |
|
|
Recently mesoporous silicas have been introduced as an alternative sorbent for the removal of VOCs due to their uniform pore size, open pore structure, and in particular, reliable desorption performance.15 Wu et al.16 compared the efficiency of sorption of VOCs from the environment using mesoporous silica and compared the results with other widely used commercial carbon-based molecular sieves of micro-porosity. The results indicated that carbon sorbents quantitatively trapped a wide range of VOCs from C3 to C12 at room temperature whereas mesoporous silica trapped considerably larger molecules from C8 to C12 with the potential to go beyond C12.
However, it is not often recognized that the adsorption of VOCs depends on numerous factors, in addition to VOC type, such as: temperature,17 relative humidity (RH),18 air velocity,19 and VOC concentration.20 Thus representative air samples might not always be collected. The influence of adsorption of VOCs in the presence of different RH values is a complicated phenomenon. In one study21 changing relative humidity values did not influence VOC (cyclohexane, toluene, ethyl acetate, isopropyl alcohol) adsorption onto a ceiling tile. A second study22 also found no impact of changing RH conditions on the sorption of seven VOCs (methyl tert-butyl ether, cyclohexane, toluene, tetrachloroethene, ethylbenzene, o-dichlorobenzene, and 1,2,4-trichlorobenzene) on various materials (carpet, vinyl and wood flooring, upholstery and fibreglass shower stall). In contrast, however, Kirchner et al.23 found that the sorption strength of 2-butoxyethanol on gypsum board was weaker under higher humidity levels and Zhang et al.24 confirmed a decrease in adsorption of dodecane and an increase of benzaldehyde when RH values altered from 50 to 80%. The interaction between VOCs and adsorbent will depend primarily on the polarity of both the sorbent and the compound which is absorbed. Normally non-polar VOCs on non-polar sorbents will be least affected by changing the humidity.
In this study, the adsorption performance of Tenax TA was compared to mesoporous silica trapping media MCM-41 or SBA-15 for 4 commonly found MAHCs (C6–C9). The sorbents' adsorption capacities and breakthrough conditions were examined at low and high RH conditions. Moreover, the effect of altering sampling flow rates at a constant RH value was examined. Finally the applicability of direct injection as a calibration method was assessed for the 3 adsorbents used in this study.
2 Experimental
2.1 Sampling chamber set up
To provide a contaminated air stream containing known concentrations of VOCs, an atmospheric chamber was built using an air compressor (Jun Air 12-50) and Kin-Tek permeation system ‘the temperature controlled oven’ (Eco-Scientific, Model 491 M-B), see Fig. 1. Air passed through a humidification system (controlled by a mass flow controller at 0.2 dm3 min−1) to provide the sample chamber with a diluent air flow that either contained additional moisture or was relatively dry (see Flow 1 in Fig. 1). The high-relative humidity (H-RH) or low-relative humidity (L-RH) conditions, at approximately 80% RH or 25% RH, were achieved by passing the air through a dreschel bottle containing distilled water or air, respectively. Approximate RH values were measured inside the permeation chamber using a room dial hydrometer which indicates the level of humidity in 5% divisions over the range of 0 to 100% RH (ETI Ltd.). Air passed into the Kin Tek system, containing the permeation vials held in the oven, at an accurately measured flow rate of 0.2 dm3 min−1 (Flow 2 in Fig. 1). The mass flow controller used was housed inside the Kin Tek system and ensured a constant accurate flow rate of air passing over the heated VOC sources. To provide controlled VOC emission into the flowing stream, 4 gas chromatography (GC) autosampler vials (with silicon-seal tops) were used to store 1 cm3 each of toluene (Bamford Laboratories, 99%), ethylbenzene (Sigma Aldrich, 99%), cumene (Fisons Scientific Apparatus, 99.5%) or dichlorobenzene (Aldrich Chemical, 99%). Prior to insertion into the permeation oven each vial was pierced with an 18 gauge GC syringe needle. The VOC emission rates were controlled by the oven temperature and the rate of Flow 2. At 25 °C and 0.2 dm3 min−1, average (n = 6) emission rates of 13
643, 7221, 4939 and 3130 ng min−1 were calculated for toluene, ethylbenzene, cumene and dichlorobenzene, with RSD% values in the range of 2 to 5. The chambers were set up and left for at least 48 hours prior to use to ensure equilibration of VOC concentrations and RH conditions. To ensure that air was not diluted during sampling, a range of sampling tubes were used to test the system at different flow rates, using replicate sampling tubes. The mass of VOCs trapped by sampling tubes increased linearly with flow rate up to 0.25 dm−3 min−1 suggesting no dilution of sampled air.
 |
| Fig. 1 Atmospheric chamber set-up. | |
2.2 SBA-15 preparation
SBA-15 was prepared by modifying the original method reported by Zhao et al.25 A surfactant tri-block copolymer solution of 4 g Pluronic P123, PEO20PPO70PEO20 (BASF Corporation, 3000 Continental Drive) was dissolved in 120 cm3 of 2 M hydrochloric acid (HCl, 37%) and 60 cm3 of distilled water in a sealed glass bottle at room temperature, and the mixture was magnetically stirred at 330 rpm. The surfactant solution was then heated to 40 °C and 11.3 g of tetraethyl orthosilicate (TEOS) were added and left for 24 h at 40 °C. The mixture was then placed in an oven for 5 days at 60 °C. The material was filtered and washed with water and dried overnight at 60 °C before calcination at 550 °C for 24 h. The resulting mixture of SiO2 : P123 : HCl : H2O had a molar ratio of 1 : 0.0032 : 4.4 : 144.
2.3 MCM-41 preparation
MCM-41 was synthesised using a method reported by Xia and Mokaya,26 where 4.4 g of cetyl trimethyl ammonium bromide (CTAB) were added to 32 g of distilled water and 3.5 g of tetramethyl ammonium hydroxide (TMAOH). The solution was heated to 35 °C with constant stirring for 30 min before the drop wise addition of 10 g of TEOS. The solution was stirred for a further hour at 38 °C then stirred for a further two hours without heat. The solution was transferred without filtration or washing to a steel bomb for autoclaving at 100 °C for 24 h. Surfactant removal was achieved by acidified solvent extraction; 1 g of silicate material was added to 25 cm3 HCl and 300 cm3 ethanol (Sigma Aldrich). The mixture was then refluxed for 24 h. Once the solution was cool the silicate material was collected by gravity filtration. The expected molar ratio of SiO2 : CTAB : TMAOH : H2O was 1 : 0.25 : 0.10 : 39.
2.4 Sampling tube preparation, VOC trapping and analysis
Commercially available sampling tubes containing 100 mg of Tenax TA held in stainless steel tubes, (Supelco, Philidelphia, USA), were used. Sampling tubes were also packed with 100 mg of SBA-15 or MCM-41 after the powder was pressed, crushed and sieved (40–60 mesh). To aid air flow through these sampling tubes, the materials were interdispersed with 1.5 g of glass beads (750–1000 µm) prior to packing. Air samples were collected from an outlet sampling port in the sampling chamber using an air sampling pump (SKC sidekick pump). Air flow rates passing through sampling tubes were individually measured using a bubble flow meter. VOCs were desorbed from adsorbents using a thermal desorption unit (TDU, TurboMatrix, Perkin-Elmer). Tubes were heated to 300 °C for 5 min and desorbed VOCs were focused in an internal trap at −30 °C before they were heated to 300 °C. The eluate was diverted to a TurboMatrix gas chromatograph mass spectrometer (Perkin-Elmer, Connecticut, USA) using an inlet line temperature of 180 °C. A Perkin-Elmer, SMS Elite (dimethylpolysiloxane, 5% diphenyl) 30 m 0.25 mm i.d. column was used and the oven was programmed with an initial temperature of 65 °C for 5 min before heating to 90 °C for 8 min. All sorbent tubes were pre-conditioned prior to use.
When assessing the influence of RH and sampling flow rates on VOC adsorption, the volume of air passing through each sampling tube was controlled to 100 cm3; achieved using sampling flow rates of 25, 50, 100, 150 or 200 cm3 min−1 with sampling times of 4, 2, 1, 0.667 or 0.5 min, respectively.
In breakthrough experiments, atmospheres containing 26.8, 14.2, 9.8 or 6.2 ng cm−3 of toluene, ethylbenzene, cumene or dichlorobenzene, respectively, were measured using a sampling flow rate of 100 cm3 min−1 for 1 min. The masses of VOCs trapped by sorbents were measured for the 100 cm3 aliquot of air. To introduce higher masses of analyte to each sorbent, sampling times were increased to 2, 3, 5, 10, 15, 20, 30, 40, 50 or 60 min and Table 2 shows masses (M1) of each pollutant passing through the sorbent for each sampling period. To determine analyte breakthrough volume, the masses (M2) of VOCs trapped by a second Tenax tube (placed in-line directly after tube 1) were measured and the percentages of VOCs trapped were calculated using:
Table 2 Total pollutant mass (ng), M1, passing through each sorbent for different sampling periods
VOCs |
Sampling time/min |
1 |
2 |
3 |
5 |
10 |
15 |
20 |
30 |
40 |
50 |
60 |
T |
2681 |
5362 |
8043 |
13 405 |
26 810 |
40 215 |
53 620 |
80 430 |
107 240 |
134 050 |
160 860 |
EB |
1419 |
2838 |
4257 |
7095 |
14 190 |
21 285 |
28 380 |
42 570 |
56 760 |
70 950 |
85 140 |
C |
975 |
1950 |
2925 |
4875 |
9750 |
14 625 |
19 500 |
29 250 |
39 000 |
48 750 |
58 500 |
DCB |
615 |
1230 |
1845 |
3075 |
6150 |
9225 |
12 300 |
18 450 |
24 600 |
30 750 |
36 900 |
3 Results and discussion
3.1 Material characterisation
The nitrogen adsorption/desorption isotherms of the calcined SBA-15 and solvent extracted MCM-41 samples were measured at liquid nitrogen temperature using a micrometric Gemini BET analyzer. The nitrogen adsorption isotherms were type IV isotherm for both SBA-15 and MCM-41, with H1 hysteresis loops, according to the IUPAC nomenclature, typical of mesoporous materials with 1D cylindrical channel27 (Fig. 2). SBA-15 had a BET surface area of 849.5 m2 g−1, a BJH pore volume of 1.01 cm3 g−1 and a micropore volume of 0.1346 cm3 g−1. The diffraction pattern produced for SBA-15 (Fig. 3) has the (1 0 0) diffraction peak intensity of approximately 150
000 a.u. at an angle 2θ of 0.871. The pore wall thickness was determined as 47 Å with a repeating unit of 116 Å. The BET surface area of the MCM-41 was 644.6 m2 g−1, with a BJH pore volume of 0.670 cm3 g−1. The diffraction pattern for MCM-41 (see Fig. 3) gave sharp diffraction peak (1 0 0), with an intensity of approximately 119
000 a.u. at an angle 2θ of 2.007 which corresponded to a d spacing of 44 Å, and repeating unit (ao) of 50 Å. The repeating unit includes the pore diameter and pore wall distance therefore given the BJH pore diameter the pore wall was determined as 18 Å.
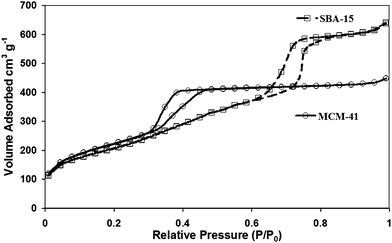 |
| Fig. 2 Nitrogen adsorption isotherms for SBA-15 and MCM-41. | |
 |
| Fig. 3 PXRD of SBA-15 and MCM-41. | |
3.2 Use of adsorbents to scavenge VOCs from indoor air
When the RH of the polluted stream was set to 80%, the VOC masses trapped onto Tenax TA increased when air sampling flow rates were increased from 25 cm3 min−1 to 200 cm3 min−1, see Fig. 4 (note: sampling times were reduced accordingly to ensure constant air volumes were sampled). This effect was not observed for ethylbenzene, cumene or dichlorobenzene when the air sampling flow rates were increased similarly at L-RH conditions as measured masses were relatively stable when sampling flow rates were altered. However, at L-RH the mass of toluene adsorbed by Tenax TA appeared to have been significantly increased when the air flow rate was reduced below 100 cm3 min−1. In general higher masses of VOCs were retained by Tenax TA when the humidity of the polluted air stream was altered from 25% to 80% RH and sampling flow rates greater than 50 cm3 min−1 were used. A different picture was observed for the silica based adsorbents where, in general, higher VOC masses were obtained at L-RH conditions particularly when sampling flow rates above 50 cm3 min−1 were used (Fig. 5 and 6). The effect of changing RH conditions on VOC masses adsorbed was most pronounced for SBA-15 compared to MCM-41 which favoured lower RH conditions for best adsorption performance. Perhaps SBA-15 has a higher adsorption capacity than MCM-41 because it contains a bimodal pore system and it has a higher surface area (850 m2 g−1 compared to 645 m2 g−1, respectively). These results suggest that, as expected, the presence of water molecules had a large influence on the performance of the silica-based adsorbents when used to trap VOCs from polluted air, apparently competing with the VOCs for adsorption sites leading to lower measured VOC masses. These results confirm those reported by Serna-Guerrero and Sayari,28 who concluded that the adsorption of aromatic compounds on mesoporous silicate in dry conditions is higher than humidified conditions; presumably as a result of monolayer water coverage on the surface of the silica.
 |
| Fig. 4 Mass of VOCs adsorbed on Tenax TA. | |
 |
| Fig. 5 Mass of VOCs adsorbed on SBA-15. | |
 |
| Fig. 6 Mass of VOCs adsorbed on MCM-41. | |
3.3 Sorbent breakthrough and dynamic capacity
By comparing the VOC masses trapped by two sorbent tubes in series, the breakthrough and dynamic capacity of each material were examined at H-RH or L-RH conditions. When increased VOC masses were introduced to the first sorbent tube (see total pollutant masses introduced to the tube in Table 2), toluene was the first analyte to be measured by the second sampling tube. The percentage breakthrough volumes for toluene, given in Fig. 7A, indicated that a larger volume of air passed through the Tenax TA sorbent tube before breakthrough was measured (5% m/m) compared to silica adsorbents. When L-RH conditions were used, Tenax TA sampled 6 dm3 of polluted air prior to breakthrough; this volume decreased to 3.9 dm3 at H-RH conditions. Using the chosen experimental sampling conditions (a 100 cm3 min−1 sampling flow rate), Tenax TA was shown to sample approximately 4 times the volume of contaminated air, in both L-RH or H-RH conditions, compared to SBA-15 or MCM-41 before breakthrough of toluene occurred. Moreover for every sorbent studied, higher air volumes were sampled before toluene breakthrough at L-RH conditions suggesting that water vapour may have been involved in chemical displacement as VOC masses increased.
 |
| Fig. 7 Calculated breakthrough volumes for analytes on each sorbent. | |
At the point of toluene breakthrough the masses of ethylbenzene, cumene and dichorobenzene trapped by each sorbent were also calculated and the percentage breakthrough of each analyte calculated. The curves (Fig. 7B–D, respectively) demonstrated a rapid increase in breakthrough of ethylbenzene and cumene for all three materials, but not for dichlorbenzene whose breakthrough percentage was approximately 0.5% or lower.
The calibrated VOC masses retained by each sorbent at the point of toluene breakthrough are given in Table 3 (i.e., sorbent capacity). Tenax-TA had similar capacity for the analytes sampled here whether L-RH or H-RH conditions were chosen. When SBA-15 was used in sampling tubes it had the ability to trap higher masses of VOCs at L-RH, however, its capacity decreased dramatically at H-RH. A similar observation can be shown for MCM-41 with much lower analyte masses at L-RH conditions. The results indicated that the bimodal sorbent, SBA-15, can only compete effectively with Tenax-TA at the extreme L-RH conditions examined here. The dynamic adsorption capacity of each material was also calculated (Table 4) as the mass of analyte trapped per unit volume of air sampled at breakthrough. Although MCM-41 had low air breakthrough volumes for toluene this sorbent had the highest dynamic capacity for toluene (i.e. it trapped the highest mass of toluene per unit volume of air) at both L-RH or H-RH.
Table 3 Total analyte mass trapped (ng) at the point of toluene breakthrougha
VOCs |
Tenax TA |
SBA-15 |
MCM-41 |
25% RH |
80% RH |
25% RH |
80% RH |
25% RH |
80% RH |
Reported values are based on an average result of three experiments; analytical precision (RSD) was typically 1–10%.
|
Toluene |
16 709 |
17 140 |
17 624 |
3568 |
10 004 |
2448 |
Ethylbenzene |
8304 |
8228 |
12 063 |
2471 |
6342 |
1633 |
Cumene |
6450 |
5876 |
10 326 |
2123 |
4926 |
1289 |
Dichlorobenzene |
11 742 |
9012 |
14 184 |
2346 |
3365 |
773 |
TVOC |
43 205 |
40 256 |
54 197 |
10 508 |
24 637 |
6143 |
Table 4 Sorbent adsorption capacities for toluene
Adsorbents |
Relative humidity (%RH) |
Air volume at breakthrough/dm3 |
Dynamic adsorption capacity/ng dm−3 |
Tenax TA |
25 |
6.0 |
2785 |
Tenax TA |
80 |
4.0 |
4285 |
SBA-15 |
25 |
3.0 |
5875 |
SBA-15 |
80 |
1.3 |
2745 |
MCM-41 |
25 |
1.3 |
7695 |
MCM-41 |
80 |
0.3 |
8160 |
3.4 Calibration curves for VOCs trapped onto sorbent tubes
Finally, to quantify VOC masses trapped in air samples an internal standard method of calibration was assessed using a 60 ng µL−1 methanolic stock solution containing toluene, ethylbenzene, cumene and dichlorobenzene. Sampling tubes containing 100 mg of Tenax TA, SBA-15 or MCM-41 were spiked with 2, 4, 6, 8 or 10 µL of the stock solution together with 5 µL of o-xylene (at 60 ng µL−1). Despite using identical TDU-GC-MS conditions, and internal standard method of calibration, different regression lines were observed for each adsorbent material (see Table 5). Less sensitive calibrations were measured for toluene, ethylbenzene and cumene for the silica adsorbents, compared to Tenax TA. The recovery of analytes from each tube was calculated and it was shown that Tenax TA has almost 100% recovery for all analytes of interest whereas the silica based adsorbents retained <50% of toluene, ethylbenzene and cumene. This lower analyte recovery was only observed when tubes were spiked with methanolic VOC solutions as a calibration technique and indicates that the methanol competes with the VOCs for the surface of the silica. Calibration by spiked solutions therefore can only be accurately performed using Tenax TA and not with silica adsorbents.
Table 5 Statistical evaluation for the calibration curves of the VOCs with different sorbents
VOCs |
Slope |
Intercept |
Correlation coefficient |
Error on slope |
Error on intercept |
Tenax TA |
Toluene |
0.0042 |
0.4567 |
0.9932 |
0.00020 |
0.0802 |
Ethylbenzene |
0.0065 |
−0.0890 |
0.9951 |
0.00026 |
0.1054 |
Cumene |
0.0059 |
−0.0365 |
0.9983 |
0.00014 |
0.0552 |
Dichlorobenzene |
0.0021 |
−0.1048 |
0.9942 |
0.00009 |
0.0373 |
SBA-15 |
Toluene |
0.0021 |
−0.0975 |
0.9951 |
0.00009 |
0.0341 |
Ethylbenzene |
0.0039 |
−0.1708 |
0.9962 |
0.00014 |
0.0559 |
Cumene |
0.0034 |
0.0187 |
0.9940 |
0.00015 |
0.0615 |
Dichlorobenzene |
0.0022 |
−0.0393 |
0.9944 |
0.00010 |
0.0383 |
MCM-41 |
Toluene |
0.0013 |
0.3202 |
0.9968 |
0.00004 |
0.0165 |
Ethylbenzene |
0.0016 |
0.6539 |
0.9927 |
0.00008 |
0.0313 |
Cumene |
0.0024 |
0.5019 |
0.9958 |
0.00009 |
0.0366 |
Dichlorobenzene |
0.0022 |
−0.0276 |
0.9949 |
0.00009 |
0.0365 |
4 Conclusions
The results of this small study indicate that VOC sampling using adsorbent tubes is complicated and care needs to be taken to ensure accurate results are obtained. There are perhaps 3 questions that can be addressed from this study:
• What sorbent type can be used for quantitative air sampling campaigns?
• How are sampling tubes calibrated?
• Which adsorbent would be a useful scavenger to reduce the concentration of indoor air pollutants?
If accurate results are to be achieved from indoor air sampling campaigns and the humidity of the sampling environment is unknown, sampling tubes should be filled with Tenax TA as this material was affected less by changing the humidity of the sampling environment from 25% to 80% RH or by altering the flow rates from 25 to 200 cm3 min−1. The silica based sorbents were strongly influenced by the presence of water vapour leading to decreasing amounts of VOCs adsorbed onto MCM-41 or SBA-15 at H-RH. Moreover, it was shown that a simple calibration method of spiking sampling tubes with methanolic solutions of VOCs can be achieved with Tenax TA. To calibrate silica tubes it would be necessary to provide a range of atmospheres of known VOC concentration at the same humidity as the sampling site. In general the masses of VOCs trapped by Tenax TA sampling tubes were also affected less when sampling flow rates were altered, compared to silica, although it was interesting to note that different masses were measured for the same volume of air passing over the Tenax TA sorbent bed especially at 80% RH. Thus the interaction between RH and sampling flow rate on accuracy of the VOC masses collected by Tenax TA needs further study. Nonetheless this material was more reliable than MCM-41 or SBA-15 when quantification was required.
When the combined VOC masses were calculated it was shown that the mass uptake of VOCs was greater for MCM-41 than for SBA-15 or Tenax TA at L-RH conditions regardless of sampling rate. At H-RH conditions the difference in the combined VOC masses was less pronounced although the highest amounts were still removed by MCM-41. Overall, the results of this study demonstrated that, for VOC scavenging, silica adsorbents performed better than Tenax TA. Moreover when the air sampling rate was increased from 25 cm3 min−1 to 200 cm3 min−1, there was a general trend towards higher scavenging efficiencies, even when the same volume of air passed over the sorbent bed. This would suggest that should an adsorbent tube be used to extract VOCs from air (as opposed to being used to measure the exact concentration of VOCs in air) then the silica-based sorbent MCM-41 would be the ideal sorbent of choice, followed by SBA-15 at L-RH conditions then Tenax TA at H-RH conditions using a sampling rate of at least 200 cm3 min−1.
References
-
Department of Health, Guidance on the Effects on Health of Indoor Air Pollutants, COMEAP, London, 2004 Search PubMed.
-
W. Gene Tucker, Air Pollution and Control, Indoor, in Kirk-Othmer Encyclopedia of Chemical Technology, ed. A. Seidel, John Wiley & Sons, Inc, Hoboken, New Jersey, 5th edn, 2004, vol. 1, pp. 816–839 Search PubMed.
- S. Brown, M. Sim and M. Abramson, Indoor Air, 1994, 4, 123 CrossRef CAS.
- R. Edwards, J. Jurvelin, K. Saarela and M. Jantunen, Atmos. Environ., 2001, 35, 4531 CrossRef CAS.
- R. Michael, V. Winkle and P. Scheff, Indoor Air, 2001, 11, 49 CrossRef CAS.
- S. K. Brown, Indoor Air, 2002, 12, 55 CrossRef CAS.
- C. Chao and G. Chan, Atmos. Environ., 2001, 35, 5895 CrossRef CAS.
- Y. Kim, S. Harrad and R. Harrison, Indoor Built Environ., 2001, 10, 147 Search PubMed.
-
T. Salthammer, Organic Indoor Air Pollutants, Occurrence, Measurement, Evaluation, Wiley-VCH Verlag GmbH, Weinheim, Germany, 1999 Search PubMed.
- S. Li, S. Chen, L. Zhu, X. Chen, C. Yao and X. Shen, Sci. Total Environ., 2009, 407, 2004 CrossRef CAS.
-
European Collaborative Action on Indoor Air Quality and its Impact on Man, Sampling Organic Strategies for Volatile Compounds (VOCs) in Indoor Air Report No 14, Eur 16051en, Luxembourg Office for Official Publications of the European Community, 1994 Search PubMed.
- B. Seifert and D. Ullrich, Atmos. Environ., 1987, 21, 395 CrossRef CAS.
- A. Kumar and I. viden, Environ. Monit. Assess., 2007, 131, 301 CrossRef CAS.
- L. Mølhave, G. Clausen, B. Berglund, J. De Ceaurriz, A. Kettrup, T. Lindvall, M. Maroni, A. C. Pickering, U. Risse, H. Rothweiler, B. Seifert and M. Younes, Indoor Air, 1997, 7, 225 CrossRef CAS.
- K. Kosuge, S. Kubo, N. Kikukawa and M. Takemori, Langmuir, 2007, 23, 3095 CrossRef CAS.
- T.-M. Wu, G. Wu, H. Kao and J. Wang, J. Chromatogr., A, 2006, 1105, 168 CrossRef CAS.
- B. A. Tichenor, Z. Guo and J. Dunn, Indoor Air, 1991, 1, 23 CrossRef.
- R. Meininghaus, S. Kirchner, F. Maupetit, H. Sallée and D. Quenard, Indoor Built Environ., 2000, 9, 277 Search PubMed.
- C. S. Lee, F. Haghighat and W. S. Ghaly, Indoor Air, 2005, 15, 183 CrossRef CAS.
- S. Kephalopoulos, H. Knoeppel and M. De Bortoli, Proceedings of Indoor Air, 1996, 12, 61 Search PubMed.
- H. Huang, F. Haghighat and P. Blondeau, Indoor Air, 2006, 16, 236 CrossRef CAS.
- R. Corsi, D. Won and M. Rynes, Proceedings of Indoor Air, 1999, 1, 390 Search PubMed.
-
S. Kirchner, P. Karpe and P. Rouxel, Adsorption/desorption processes of organic pollutants on indoor material areas, CSTB Report EAE/SB-96059, 1996.
- J. Zhang, J. S. Zhang and Q. Chen, ASHRAE Trans., 2002, 108, 273 Search PubMed.
- D. Zhao, Q. Huo, J. Feng, B. F. Chmelka and G. D. Stucky, J. Am. Chem. Soc., 1998, 120, 6024 CrossRef CAS.
- Y. D. Xia and R. Mokaya, J. Mater. Chem., 2004, 14, 2507 RSC.
- A. A. Campos, L. Dimitrov, C. R. Da Silva, M. Wallau and E. A. Urquieta-Gonzales, Microporous Mesoporous Mater., 2006, 61, 92 CrossRef.
- R. Serna-Guerrero and A. Sayari, Environ. Sci. Technol., 2007, 41, 4761 CrossRef CAS.
|
This journal is © The Royal Society of Chemistry 2010 |
Click here to see how this site uses Cookies. View our privacy policy here.