DOI:
10.1039/B9AY00269C
(Paper)
Anal. Methods, 2010,
2, 728-733
Scintillating adsorptive membrane for preconcentration and determination of anionic radionuclides in aqueous samples
Received
23rd November 2009
, Accepted 24th March 2010
First published on
26th April 2010
Abstract
A composite polymer membrane containing liquid anion exchanger and scintillator molecules was developed for preconcentration and quantitative determination of anionic radionuclides like pertechnetate and iodide ions. The radiation emitted by the radionuclides sorbed in the membrane lead to scintillation signals. This scintillating adsorptive membrane was prepared by immobilizing trioctylmethyl ammonium chloride (liquid anion-exchanger) along with 2,5-diphenyl oxazole (primary scintillator), and 4-bis(methylstyryl)benzene (wavelength shifter) in the plasticized polystyrene matrix. The anion selectivity of the membrane follows Hofmeister series i.e. hydrophobic anions are preferentially sorbed in the membrane matrix. It was observed that TcO4− ions were transferred quantitatively to the membrane matrix from equilibrating aqueous solutions having pH 2–8 and multicomponent feed solution like seawater. The amount of TcO4− ions preconcentrated in the membrane was directly quantified by counting the scintillations produced by β-particles in the membrane matrix. The scintillating membrane developed in the present work was optimized in terms of its scintillation efficiency, β-scintillation pulse height, anion-exchange capacity and reusability.
Introduction
Ultra trace detection and quantification of α/β radioactivity in aqueous samples has important applications in process control and environmental monitoring. Conventional radiometric methods cannot be used without preconcentration of the radionuclides to produce signals above the background noise of the radiation detectors. In view of this, there is a need to develop analytical methods that have superior detection limits. One of the ways is to incorporate a preconcentration step in the methodology e.g. scintillating adsorptive membrane-like radionuclide sensor. A radionuclide sensor is a combination of a sensing element, that selectively interacts with a radioactive target, and a transducer that produces a signal generated due to the interaction between the target and the sensing element.1 The advantage of a radionuclide sensor over the conventional radiation detectors is that it can also provide an efficient preconcentration step integrated with the detection process. Generally, organic scintillator molecules are employed for α/β detection and measurement owing to their fast response time.2 Grate et al. have reviewed the radionuclide sensors developed for environmental monitoring.1
Extracting scintillator resins are being explored to develop the sensors for low level radionuclides in the environment. These are prepared by impregnating polymer beads with an organic phase containing both the extractant and the scintillator.1,3–5 The methodology involved in these radionuclide sensors is based on preconcentration of the target radionuclides from an aqueous sample into the scintillating organic phase immobilized in the resins followed by quantification of the radionuclides by the direct scintillation counting. The preconcentration of the analyte in a smaller volume can simplify the detection method and reduce the time of detection. The flow injection or sequential injection system has been used for developing the preconcentrating minicolumn radionuclide sensor.1 In another approach, the scintillating fibers were combined with a selective sorbent resin to create dual-functionality sensors for 137Cs in aqueous samples.6 Scintillating membranes have been reported for selective extraction and detection of trivalent elements like 147Pm, 154Eu, and 241Am.7,8 These scintillating membranes are prepared by using dioctyl phthalate (DOP) plasticized cellulose triacetate (CTA) membrane containing optimum amounts of extractant bis(2-ethylhexyl)phosphoric acid (HDEHP), scintillator 2,5-diphenyl oxazole (PPO) and wavelength shifter 1,4-bis(2-methylstyryl)benzene (MSB).
Technetium (99gTc) is an important radionuclide in the environmental monitoring as: (i) it is produced during thermal fission of 235U and 239Pu with a high fission yield (6%),9 (ii) it is a β-emitter and has a long half-life of 2.111 × 105 years,10 and (iii) it is highly mobile in the environment as pertechnetate anions (TcO4−).11 Therefore, a suitable analytical method is required to detect and quantify ultra trace concentration of 99gTc in the natural aqueous samples. Sensors for technetium were developed based on two extractants, viz. Aliquat-336 (trioctylmethylammonium chloride) and monomethylated-polyethylene glycol.3,4,12 The latter is known to bind pertechnetate ions from solutions having high ionic strength, which is useful for the analysis of nuclear waste and waste processing streams.13
In the present work, we have studied the possibility of using plasticized anion-exchange membranes for simultaneous preconcentration and detection of 99gTcO4− ions. The plasticized anion-exchange membranes are highly hydrophobic and have selectivity towards anions depending on their hydration and valence states.14–16 The scintillating membrane has been prepared by immobilizing liquid anion-exchanger Aliquat-336 along with the scintillators PPO and MSB in a tris-(2-ethylhexyl)phosphate (TEHP) plasticized polystyrene (PS) matrix. The choice of PS as a matrix is based on the fact that it gives a better pulse height as compared to other matrix forming polymers like cellulose triacetate (CTA). The uptake of TcO4− ions from aqueous samples having different pH and ionic strengths like tap water and seawater has been studied. The scintillating membrane developed in the present work has been characterized in terms of its ion-exchange capacity, TcO4− uptake efficiency, β-scintillation pulse height and regeneration. The membrane has also been characterized for another anionic β/γ-emitter radionuclide 131I−, which also sorbs in the membrane matrix. The scintillating membrane has been found to give a good linear response as a function of concentration of TcO4− ions transferred to the membrane matrix.
Experimental
Reagents and apparatus
Analytical reagent grade dichloromethane (DCM), de-ionized water (18 MΩ/cm) purified by model Quantum™ from Millipore (Mumbai, India), and suprapure grade nitric acid (Merck, Mumbai, India) were used throughout the work. Poly(styrene) (average Mw = 250000) (Acros Organics, New Jersey, USA), tris-(2-ethylhexyl)phosphate (TEHP) (Koch-Light Laboratories, Coinbrook Bucks, England), Aliquat-336 (Sigma-Aldrich, Steinheim, Germany), 2, 5-diphenyl oxazole (PPO) (Sigma-Aldrich, Steinheim, Germany), and 1,4-bis(methylstyryl)benzene (MSB) (Sigma-Aldrich, Steinheim, Germany) were used as obtained. A stock solution of 99gTcO4− in pH = 2 was obtained from Nuclear Chemistry Section, Radiochemistry Division, BARC and 131I radiotracer was obtained from Board of Radiation and Isotope Technology, Mumbai, India. Thickness of the membrane was measured by a digital micrometre (Mitutoyo, Japan) with a precision of ±0.001 mm. A microprocessor based pH meter model PHAN from Lab India (Mumbai, India) was used for pH measurements. UV- visible spectrophotometer model V 530 from JASCO (Tokyo, Japan) was used for recording the UV-Vis spectra. The β-radioactivity of 99gTc in equilibrating aqueous solutions was monitored by taking out 50 μL sample, and adding it to a vial containing 5 mL of scintillation cocktail-W. The composition of the cocktail-W was: 2,5-diphenyl oxazole = 10 g, 1,4-di-2-(5-phenyloxazolyl) benzene = 0.25 g, and naphthalene = 100 g in 1000 mL dioxane. The β-counting and pulse height measurement of the membrane samples were carried out using a home built liquid scintillation counter with EMI 9514 photomultiplier tube (13 stages) coupled with a PC-based multi-channel analyzer (MCA). The scintillation efficiency of β-particles of 99gTc was carried using a calibrated Instrument, 300SL TDCR Liquid Scintillation Counter (Hidex, Finland). The liquid scintillation cocktail used in these experiments was Ultima Gold AB (Perkin Elmer). The γ counting was carried out using a 3 cm × 3 cm well type NaI(Tl) detector as well as an HPGe detector coupled to a PC-based MCA. Fluorescence spectrometer (Hitachi F2000, Tokyo, Japan) was used to record the absorption and emission spectra of the scintillating membrane samples.
The adsorptive membranes were prepared by solution casting method. Appropriate amounts of PS, TEHP, Aliquat-336, and scintillators (PPO and MSB) were dissolved in dichloromethane (DCM). The solution was homogenized by ultrasonicating it for 5 min. This casting solution was spread over a flat-bottom glass Petri dish (diameter = 9 cm), kept on a levelled surface and DCM was allowed to evaporate slowly overnight. After complete evaporation of DCM, a homogeneous membrane was formed. This membrane was carefully peeled off from the Petri dish and laid over a linear graph paper to cut out strips of desired dimensions. The composition of the membrane studied in the present work was: PS = 79 ± 2%, Aliquat-336 = 8 ± 1%, TEHP = 4.0 ± 0.5%, PPO = 8 ± 1% and MSB = 1.0 ± 0.3%. The thickness of the membrane was varied by increasing the amount of components in a fixed proportion to keep the composition of the membrane same.
β-scintillation counting
The membrane samples were equilibrated with well stirred solutions containing 99gTcO4−/131I− ions. After equilibration, the samples were taken out of the solution, washed with distilled water and dried between the folds of tissue paper. These β-emitting radionuclide loaded membrane samples were mounted on to the inside wall of a quartz cell (1 cm × 1 cm × 5 cm) and was counted for 60–180 s in a scintillation counter. To get constant counting efficiency, a fixed geometry of the membrane samples was maintained during scintillation counting. For pulse height analysis, the signal from amplifier was fed to a PC-based multi-channel analyzer. For studying the time required to obtain optimum sorption or desorption of 99gTcO4− ions and reusability, the aqueous sample was taken out at regular intervals from equilibrating aqueous solution and subjected to scintillation counting. Sorption experiments were carried out by immersing the membrane sample (2 cm × 1 cm) in the 15 mL equilibrating solution containing 99gTcO4− ions at pH = 2. A 50 μL sample from equilibrating solution was taken out at different time intervals to monitor the sorption of 99gTcO4− ions in the membrane sample.
Uptake capacity and β-counting efficiency
Two different methods were used for measuring the TcO4− and I− uptake capacity (ion-exchange capacity) of the membrane. For the pure β-emitter 99gTcO4− ions, the membrane sample having known weight was equilibrated for 4 h with 15 mL of well stirred solution containing an excess of TcO4− ions. The moles of TcO4− ions sorbed in the membrane sample were obtained from the difference of total β-activities of solution before and after equilibration. Two different 50 μL samples of the equilibrating solution were taken; one before and another after equilibration with the membrane sample. These aqueous samples were added to 5 mL of Ultima Gold AB cocktail for the β-scintillation counting. The scintillation counting was carried out in a calibrated Liquid Scintillation Counter. The total β-activity of 99gTcO4− ions was converted to moles of TcO4− ions. Thus, the ion-exchange capacity of the membrane (mol g−1) was obtained.
As 131I is a γ-emitter, the amount of I− sorbed in the membrane sample was obtained by directly measuring the γ-activity of the membrane sample. For this experiment, the membrane samples (2 cm × 1 cm pieces) were equilibrated for 4 h with 25 mL of 0.1 mol L−1 KI stock solution containing known activity of 131I radiotracer. The concentration of I− ions in the equilibrating solution was kept much higher than the uptake capacity of the membrane sample to ensure saturation loading. After equilibration, the membrane samples were thoroughly washed with deionized water to remove equilibrating solution clinging to the surface of the samples. Filter paper standards (4 Nos.) were prepared by taking pieces of filter paper of the same dimensions (2 cm × 1 cm) as the membrane samples and spreading known volumes (50–200 μL) of the radiolabeled 0.1 mol L−1 KI stock solution. The amount of I− ions in these standards varied from 2.5 μmol to 10 μmol. The same KI stock solution was used to prepare the filter paper standards as well as equilibrating the membrane samples so as to keep 131I to 127I ratio fixed. Each standard was prepared in duplicate. The soaked filter papers were dried at room temperature and counted in a well type NaI(Tl) detector in a similar counting geometry as the membrane samples. The amount of I− ions in the membrane sample was obtained by comparing the γ-activity of 131I in the membrane samples with the filter paper standards. The loading capacity of the membrane sample was computed from the knowledge of amount of I− ions sorbed in the membrane sample and its weight.
The radionuclide 131I− was used for obtaining the scintillation counting efficiency as it emits γ-rays as well as β-particles where as 99gTc is a pure β-emitter. The γ-activity of 131I− (364 keV) was monitored by using an efficiency calibrated well-type NaI(Tl) detector connected to a multi-channel analyzer. The absolute activity was determined by using following equation.
|  | (1) |
where
εγ is the gamma detection efficiency of the NaI(Tl)
detector,
Iγ is the branching intensity or abundance of the 364 keV γ-ray and
Nγ is the measured count rate. The
membrane samples, loaded with the known radioactivity of
131I, were counted for β-scintillations as described above. Since the absolute activity of the
membrane sample was known, the β-counting efficiency (
εβ) was obtained from scintillation count rate (
Nβ) as:
|  | (2) |
For pure β-emitter 99gTc, the β-counting efficiency was also determined by indirect method using eqn (2). In this case, the value of Aabs was determined from the difference of total β-activity of solution before and after equilibration of the membrane sample.
Sorption and desorption of TcO4− ions
To study the effect of pH on TcO4− ion sorption, the uptake of TcO4− ions by the fixed size membrane samples (2 cm × 1 cm) was measured by immersing the samples in 15 mL aqueous solutions containing a total of ∼105 Bq radioactivity of 99gTc at different acidities adjusted with HNO3. The uptake of TcO4− ions in the membrane samples was calculated by radioactivity balance, which was obtained by measuring β-radioactivity of 99gTcO4− ions in the equilibrating solutions before and after the experiment. 99gTcO4− ions were desorbed from the membrane sample in 15 mL of 2 mol L−1 HNO3 solution. The effect of presence of other anions on the uptake of 99gTcO4− ions in the membrane were studied by equilibrating the membrane samples loaded with a representative Br−, I− or ClO4− anions with solution containing 99gTcO4− ions at different pH. Uptake behavior of the membrane towards TcO4− ions was also studied from real samples like tap water and seawater spiked with a known amount of 99gTc. The membrane samples were equilibrated overnight with 10 mL of each of the aqueous samples and then uptake was monitored by the β-scintillation counting.
Results and discussions
Mechanism of sorption and optical response
The anions are sorbed in the membrane matrix as an ion-pair with the quaternary ammonium molecules (Aliquat-336) immobilized in the membrane matrix. The matrix of the membrane is highly hydrophobic as its water content was found to be less than 1 wt. %. The hydrophobicity facilitates to stabilize the extractant and scintillator in the membrane matrix. The least hydrated oxyanions like TcO4−, HCrO4− and ClO4− are expected to sorb preferentially in the membrane matrix.11–13 The kinetic energy of the β-particles emitted by 99gTcO4− ions in the membrane is absorbed by the plasticized poly(styrene) matrix, which is subsequently transferred to the scintillator molecules. The composition of poly(styrene), TEHP, and Aliquat-336 was chosen in such a way to get a mechanically stable film with maximum sorption capacity of TcO4− ions. PPO is the primary scintillator and the role of MSB is to shift the emitted wavelength to the visible region in which PMT works efficiently. The amount of these scintillators in the membrane were based on our previous studies.8 In order to study the optical response, the absorption and emission spectra of the membrane samples were recorded. As can be seen from Fig. 1, the membrane absorbs in the UV-region and emits in the visible region (400–600 nm). This is suitable for the efficient conversion of kinetic energy of the β− particles deposited in the membrane matrix to scintillation signal in the visible region. In the absence of scintillators, there is no response in the visible region. Also, it is seen from Fig. 1 that the emission intensity is maximum when membrane is excited with 350 nm light, which is close to the absorption maximum of PPO (≈370 nm).
Sorption and desorption of TcO4− ions
The uptake of TcO4− ions by the scintillating membrane from aqueous solution at pH = 3, tap water, and seawater was studied by monitoring the β-radioactivity of the aqueous sample before and after equilibration with the membranes. It was observed that the sorption of TcO4− ions in the membrane was 95 ± 5%, obtained from 10 replicate experiments, from all the three aqueous samples. In order to study the effect of pH, the membrane samples were equilibrated with solutions having a known amount of TcO4− ions at different pH values. It is seen from Fig. 2 that the uptake of TcO4− ions increases with increasing pH of the solution, and attains an almost constant value (95 ± 5%) from pH 2 to 8. As the pH of the equilibrating solution was adjusted with HNO3, the decrease in the uptake of TcO4− ions by the membrane at higher acidity could be attributed to competition of NO3− ions with TcO4− ions for available ion exchange sites in the membrane samples. The effect of presence of other anions like Br−, I− and ClO4− on the uptake of TcO4− ions was also studied as a function of pH, and the results are shown in Fig. 2. It is seen from this figure that the uptake of TcO4− ions is not affected by the presence of the above anions in the membrane matrix. The membrane developed in the present work was also found to be efficient for preconcentration of TcO4− ions from large volume (100 mL) of aqueous samples. This was based on the fact that uptake values of TcO4− ions in the membrane samples equilibrated for overnight with 15 mL and 100 mL stirred aqueous solutions, spiked with the same amount of radioactivity of 99gTc, were found to agree within ±5% variation.
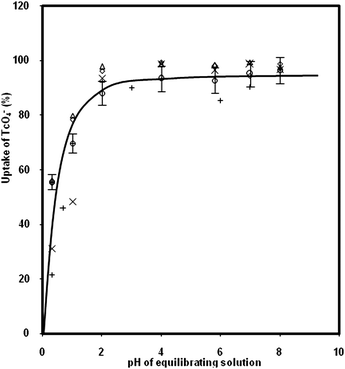 |
| Fig. 2 Uptake of 99gTcO4− ions by the scintillating membrane as a function of pH. The symbols +, ×, Δ, ○ and ◊ represent the Cl−, Br−, NO3−, ClO4−, and I− anionic forms of the membrane samples, respectively. | |
Desorption of TcO4− ions from the membrane samples were found to be 50–60% after overnight equilibration in 1 mol L−1 KI or NaNO3. This seems to suggest that TcO4− ions have strong electrostatic interactions with Aliquat-336 present in the scintillating membrane. Desorption of TcO4− ions from membrane sample could be improved to 70% on equilibration with 2 mol L−1 HNO3. However, the quantitative desorption (≈ 90%) of TcO4− ions from membrane was observed after second cycle of equilibration with 2 mol L−1 HNO3 for 4 h with constant stirring. The nearly complete desorption of TcO4− ions was observed after third time equilibration of the membrane sample with 2 mol L−1 HNO3. The sorption-desorption cycles were repeated 5 times, which indicated that TcO4− ions uptake remained same in the successive sorption cycles. Thus, the components (Aliquat-336, PPO, and MSB) physically immobilized in the plasticized poly(styrene) are quite stable.
β-Scintillation response
In order to optimize the response signal of the membrane, the scintillation pulse height analysis was carried out by coupling the amplifier output to a PC-based multi-channel analyzer. The spectra of β-scintillation pulses of 99gTc in liquid scintillation cocktail and membrane are shown in Fig. 3. It is evident from Fig. 3 that the 99gTcO4− ions loaded membrane produced a higher pulse height as compared to that obtained in liquid scintillation (LS) at a given setting of the amplifier gain. This may be due to better conversion efficiency of the kinetic energy of the β-particles to scintillation pulse in poly(styrene) matrix than in the liquid scintillation cocktail. The conjugated structure of poly(styrene) helps in the efficient transfer of energy.17 This scintillating membrane can also be termed as “optode” as it produces optical response in the form of scintillation pulses proportional to the concentration of TcO4− ions sorbed in the membrane.8
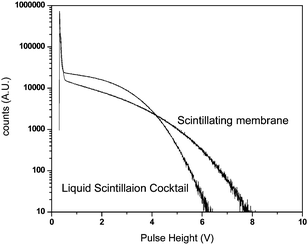 |
| Fig. 3 Comparison of scintillation pulse heights of β-particles emitted by 99gTcO4− in liquid scintillation cocktail and scintillating membrane. | |
In order to increase β-scintillation pulse height produced by 99gTc, the thickness of the membrane sample was increased from 270 ± 22 μm to 900 ± 72 μm. The thickness of the membrane sample was found to vary by 8%. However, different parts of the same membrane sheet were found to give almost same scintillation pulse when equilibrated with solutions having same concentration of TcO4− ions. This seems to suggest that the scintillating membrane prepared in the present work is fairly homogeneous.
It can be clearly seen from Fig. 4 that the pulse height increases with increase in thickness from 270 ± 22 μm to 520 ± 42 μm. This is expected as more energy will be deposited in a thicker membrane sample. But the pulse height was decreased for the membrane sample having 900 ± 72 μm thickness. A probable reason for this could be deterioration of optical transparency of the membrane sample on increasing the thickness to 900 ± 72 μm. This, in turn, would result in the loss of photons reaching the PMT.
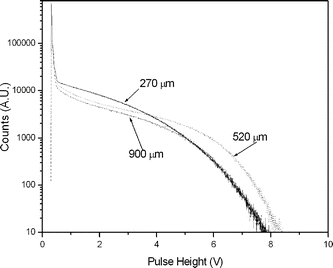 |
| Fig. 4 Pulse height spectra of β-scintillations of the TcO4− loaded samples having different thickness. | |
A similar experiment was carried out with 131I− ion loaded membrane samples having varying thickness. The pulse height spectra are plotted in Fig. 5. On comparing Figs. 4 and 5, it was observed that the scintillation pulses produced by β-particles (Eβmax = 606 keV) emitted by 131I were lower than those produced by β-particles (Eβmax = 294 keV) emitted by 99gTc. The pulse height increased significantly by increasing the thickness of the membrane sample from 270 ± 22 to 900 ± 72 μm. This seems to suggest that the higher thickness is required to increase the scintillation pulse height of higher energy β-particles.
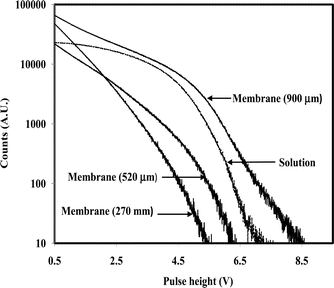 |
| Fig. 5 Pulse height spectra of β-scintillations in 131I−−loaded membrane samples having varying thickness. | |
Characterization of membrane for analytical applications
The average sorption capacities of the four membrane samples were found to be 19 ± 3 and 15 ± 2 μmoles per gram of the sample for TcO4− and I− ions, respectively. The experimental I− ions sorption capacity was 80% of that calculated from Aliquat-336 amount in the membrane sample. Sorption kinetics was studied by equilibrating the membrane sample (2 cm × 1 cm) in a well-stirred 15 mL solution (pH = 2) spiked with TcO4− ions. Fig. 6 shows the fractional attainment of sorption equilibrium of TcO4− ions in the membrane as a function of time. It is seen from Fig. 6 that the membrane attains equilibrium in about 100 min.
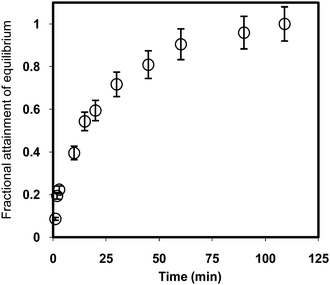 |
| Fig. 6 Variation of fractional attainment of equilibrium F(t) by TcO4− ions in scintillating membrane sample equilibrated with a well-stirred solution at pH = 2 as a function of equilibration time. | |
The absolute β-counting efficiency of the membrane was found to be 60 ± 2% for 131I (Eβmax = 606 keV) and 72 ± 4% for 99gTc (Eβmax = 294 keV). The lower β-counting efficiency for 131I indicates that the energy deposition in the membrane by higher energy β-particles is lower than that in the case of 99gTc. The similar trend was also observed in the pulse height spectra.
To study linearity in the variation of β-scintillation counts as a function of concentration of 99gTcO4− ions, the membrane samples having fixed dimensions were equilibrated with the well stirred solutions having pH = 2 and amount of 99gTcO4− ions varying from 0.5 nanomol to 5.5 nanomol. It is seen from Fig. 7 that the scintillation counts in the membrane samples varied linearly as a function of concentration of 99gTcO4− ions in the solution. Similar trend was observed when pH in the equilibrating solution was changed from 2 to 7. Thus, the scintillating membrane developed in the present work can be used for preconcentration and determination of TcO4− ions from wide range of aqueous samples.
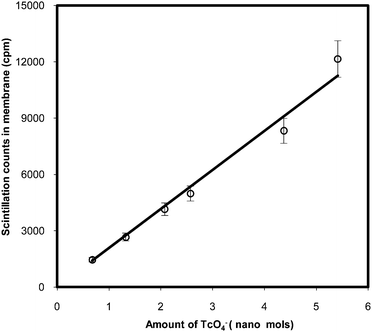 |
| Fig. 7 Variation of β-scintillation activity of 99gTcO4− in the membrane sample as a function of concentration of TcO4− ions in well-stirred 15 mL equilibrating solution at pH = 2. | |
Conclusions
A homogeneous scintillating membrane prepared by physically immobilizing scintillators (PPO and MSB) and an extractant (Aliquat-336) in a TEHP plasticized poly(styrene) matrix was found to be suitable for preconcentration of TcO4− ions. There is a possibility that other β-emitters like 131I− can be distinguished from 99gTcO4− by γ-rays. The membrane was characterized for its extraction efficiency, scintillation efficiency, pulse height and sorption kinetics. It was found that the membrane takes up TcO4− ions quantitatively (95 ± 5%) from a solution having pH ≥ 2, tap water and seawater. The pulse height of the membrane was slightly higher than that of the liquid scintillation cocktail. The β-counting efficiency of the membrane was found to be 72% for 99gTc. Thus, the scintillating membrane developed in the present work can be used for the preconcentration and determination of β-emitting anions like 99gTcO4− ions from the aqueous sample.
Acknowledgements
The part of the work was carried out as a research project for 51st Batch Chemistry Trainee, HRDD, BARC. Authors are thankful to Dr S.V. Godbole, Radiochemistry Division for his help during fluorescence measurements. Authors are also thankful to Dr V. K. Manchanda Head, Radiochemistry Division for his keen interest in this work.
Notes and references
- J. W. Grate, O. B. Egorov, M. J. O'Hara and T. A. DeVol, Chem. Rev., 2008, 108, 543–562 CrossRef CAS.
-
G. F. Knoll, Radiation Detection and Measurement, John Willey and Sons, New York, 1979, chapter 8 Search PubMed.
- T. A. DeVol, O. B. Egorov, J. E. Roane, A. Paulenova and J. W. Grate, J. Radioanal. Nucl. Chem., 2001, 249, 181–189 CrossRef CAS.
- O. B. Egorov, S. K. Fiskum, M. J. O'Hara and J. W. Grate, Anal. Chem., 1999, 71, 5420–5429 CrossRef CAS.
- J. E. Roane and T. A. DeVol, Anal. Chem., 2002, 74, 5629–5634 CrossRef.
- J. Headrick, M. Sepaniak, S. D. Alexandratos and P. Datskos, Anal. Chem., 2000, 72, 1994–2000 CrossRef CAS.
- S. Sodaye, R. Tripathi, A. K. Pandey and A. V. R. Reddy, Anal. Chim. Acta, 2004, 514, 159–165 CAS.
- S. Sodaye, Y. M. Scindia, A. K. Pandey and A. V. R. Reddy, Sens. Actuators, B, 2007, 123, 50–58 CrossRef.
- R. H. Iyer, H. Naik, A. K. Pandey, P. C. Kalsi, R. J. Singh, A. Ramaswami and A. G. C. Nair, Nuclear Science and Engineering, 2000, 135, 227–245 Search PubMed.
-
E. Browne,R. B. Firestone and V. S. Shirley, ed., Table of Radioactive Isotopes, 1986, John Wiley & Sons, New York Search PubMed.
- O. B. Egorov, M. J. O'Hara and J. W. Grate, Anal. Chem., 2006, 78, 5480–5490 CrossRef CAS.
- T. A. DeVol, J. E. Roane, J. M. Williamson, J. M. Duffey and J. T. Harvey, Radioact. Radiochem., 2000, 11, 34–46 Search PubMed.
- R. D. Rogers, A. H. Bond, S. T. Griffin and E. P. Horwitz, Solvent Extr. Ion Exch., 1996, 14, 919–946 CrossRef CAS.
- Y. M. Scindia, A. K. Pandey, A. V. R. Reddy and S. B. Manohar, Anal. Chem., 2002, 74, 4204–4212 CrossRef CAS.
- S. Sodaye, G. Suresh, A. K. Pandey and A. Goswami, Radiochim. Acta, 2006, 94, 347–350 CrossRef CAS.
- S. Sodaye, G. Suresh, A. K. Pandey and A. Goswami, J. Membr. Sci., 2007, 295, 108–113 CrossRef CAS.
- S. W. Moser, W. F. Harder, C. R. Hurlbut and M. R. Kusner, Radiat. Phys. Chem., 1993, 41, 31–36 CrossRef CAS.
|
This journal is © The Royal Society of Chemistry 2010 |