DOI:
10.1039/B9AY00194H
(Review Article)
Anal. Methods, 2010,
2, 110-122
Recent advances in on-line multidimensional liquid chromatography
Received
2nd October 2009
, Accepted 2nd December 2009
First published on
23rd December 2009
Abstract
Multidimensional liquid chromatography (MD LC) has, due to enhanced peak capacity compared to one-dimensional LC, become an important analytical tool for separating components in complex samples, e.g. in proteomics. MD LC can be performed both on-line and off-line. Because of the advantages like possible automation and minimal sample loss, on-line MD LC has appeared to be very attractive also for high throughput analysis. This review includes only on-line coupled MD LC. Different aspects related to the compatibility of separation principles and column dimensions are highlighted and recent applications using on-line mainly two-dimensional (2D) LC have been included.
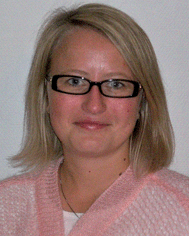 Helle Malerod | Helle Malerod received her cand.scient degree in analytical chemistry at the Department of Chemistry, University of Oslo (UiO) in 2005. She started her PhD degree at UiO under supervision of Prof. Elsa Lundanes and Prof. Tyge Greibrokk in 2006. In her PhD, she is developing multidimensional liquid chromatographic methods coupled with mass spectrometry for identification of peptides and proteins in various biological samples. She is now in her last year as a PhD student. |
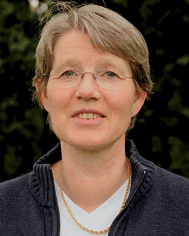 Elsa Lundanes | Elsa Lundanes is Professor of Analytical Chemistry at the Department of Chemistry, University of Oslo, Norway. Her research interests are development of analytical methods for organic compounds based on miniaturized liquid chromatography and mass spectrometry. Together with Prof. Tyge Greibrokk, Lundanes is a core member of Bioanalytics@UiO, the center of analytical chemistry and separation science in Norway. |
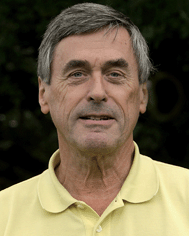 Tyge Greibrokk | Tyge Greibrokk is Professor of Analytical Chemistry at the Department of Chemistry, University of Oslo, Norway. During the last 35 years he has worked on the development of new methods and techniques in chromatography, mainly HPLC but formerly also supercritical fluid chromatography, electrochromatography as well as microplasma GC-MS. He is one of the editors of the Journal of Separation Science and has served on the editorial boards of half a dozen other journals. Currently his main research is connected to miniaturizing HPLC columns with particular emphasis on 2D systems. Greibrokk is also a core member of Bioanalytics@UiO. |
1. Introduction
Multidimensional liquid chromatography (MD LC) methods, with enhanced peak capacity compared to one-dimensional (1D) LC have in recent years become more frequently used for routine analyses, and in more explorative applications e.g. proteomics. In MD LC, components are subjected to separation by two or more separation mechanisms before detection. An alternative is a combination of a chromatographic separation and a subsequent detection by mass spectrometry (MS) or photodiode array detection (PDA) serving as the second dimension. MS provides more information than PDA and is often the detector of choice in MD LC. Furthermore, due to the requirement of increased peak capacity, MS or PDA is more commonly applied as the third dimension. The total theoretical peak capacity of the method is the product of the peak capacity of each dimension; Evans et al. reported a peak capacity of 3200 for a two-dimensional (2D) 15-fraction multidimensional protein identification technology (MudPIT) analysis, but when incorporating MS as a third dimension, the total peak capacity increased to 23 000.1
The term MD LC has been used for combinations of different LC separation principles as well as methods including electrophoresis.2,3 Several reviews on different aspects of MD LC have been published the last years.4–14 In this review, we will only focus on on-line MD LC applying LC in two or more dimensions. Compared to off-line MD LC, on-line MD LC methods are more complicated, require more technical skills and demand a higher compatibility between the applied separation systems. The advantages are minimal sample loss and contamination, high repeatability and possibility of automation, which make the on-line methods very attractive, also for high throughput analysis. In this review, the term on-line MD LC is used for instrument systems where the eluent from the first dimension is automatically transferred to the second dimension via tubing and/or a valve with connecting storage loops or trap columns.15 Both heart-cutting (LC - LC) and comprehensive fractionation (LC × LC) can be performed by on-line MD LC. In the former approach, only a part of the first dimension eluent is transferred to the second dimension for subsequent separation.16–18 This technique is mostly used for determination of one or few target analytes. In comprehensive MD LC, the entire sample, divided in several fractions is transferred from the first to the second dimension for further LC analysis.18 Most on-line MD LC systems reported apply 2D LC, we will therefore restrict our description of MD LC instrumentation to on-line 2D LC.
2. Multidimensional liquid chromatographic (MD LC) methodology
2.1 Peak capacity in MD LC
2.1.1 Theoretical peak capacity.
Peak capacity, nc, can be defined as the maximum number of peaks that can theoretically be resolved in a separation window between the first and the last eluting peak.16,19 When two separation mechanisms are completely orthogonal, the theoretical peak capacity (nMD) is the product of the peak capacity of each dimension, the so-called peak capacity product rule.4,6,20,21n1 and n2 are the peak capacities contributed from the first and second LC dimension. Other definitions of peak capacity have also been published, for instance as the maximum number of separated components on a column within a gradient time.22 However, when using that definition, the theoretical peak capacity is generally higher compared to the former one, because of possible elution windows at the beginning and at the end of the gradient where no components are eluting.
2.1.2 Orthogonality.
When considering the peak capacity in MD LC, the orthogonality of the dimensions also needs to be discussed.16,17,19 Two dimensions are orthogonal when the separation mechanisms provide different selectivity and show distinctly different retention profiles.19 If the peak distribution is statistically independent, the LC separation mechanisms are considered to be orthogonal.17 However, completely different separation mechanisms do not guarantee orthogonality, and the theoretical peak capacity is usually greater than the practical peak capacity.10,19,22 For example, electrostatic interaction is the main cause of retention in strong cation exchange chromatography (SCX), but secondary hydrophobic interactions occur too.10,19 Consequently, the combination with reversed phase (SCX-RP) is not completely orthogonal.10,19 Therefore, the theoretical peak capacity usually overestimates the actual peak capacity.4,19,22–24
2.1.3 First dimension undersampling.
If the sample amount is unlimited, high peak capacity can be obtained by reducing the sampling time per fraction in the first dimension, in the following called sampling time. In order to maintain the first dimension separation peak capacity, each peak should be divided in several fractions.19,25–29 This is referred to as oversampling. Horvath et al. showed that when each peak was divided in two fractions, the relative peak capacity was approximately 80% of the theoretical peak capacity.30 The relative peak capacity increased with decreasing sampling time and when one peak was collected in ten fractions, the relative peak capacity was 97%.30 As a consequence of short sampling time, the second dimension separation needs to be fast, unless special storage devices are used.
However, if the number of collected fractions is low, separable peaks will be collected in the same fractions (undersampling) and the resolution is reduced.19,25 Undersampling occurs very often in separation of complex samples as in proteomics and peptidomics, when the sample amount is limited and sensitivity is often more crucial than increased resolution and peak capacity. Due to first dimension undersampling, the actual peak capacity in the first dimension cannot be higher than the number of fractions collected.28 Even though the peak capacity is increased with increasing fractionation frequency, peak overlap makes the identification of compounds more difficult, especially in shot gun analysis. Thus, the peak width and complexity of the sample should decide the number of fractions to be collected.2 Hence, when the peak width varies during the first dimension separation, the fractionation frequency should vary as a function of the peak width. Additionally, with complex samples, it may be impossible to divide each peak in three fractions and hence a compromise has to be made. The effect of undersampling on first dimension peak capacity as described by Li et al. should also be considered.23
| 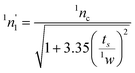 | (2) |
1n1′ represents the corrected peak capacity while
1nc is the first dimension peak capacity.
23 The terms
ts and
1w are the sampling time and the first dimension peak width.
23 Horváth
et al. claimed that the maximum peak capacity that is possible to obtain with on-line MD
LC is 10 000, and if higher capacity is needed an off-line approach has to be used instead.
26
2.1.4 Column dimensions.
With unlimited sample amount, long conventional sized columns (3–5 mm ID)31 are generally used in the first dimension to increase the total peak capacity. To avoid first dimension undersampling the number of fractions collected in the first dimension must be large and a fast second dimension separation is required. Thus, short columns with a large inner diameter in combination with high flow rates are applied in the second dimension and the fraction volume transferred from the first dimension can be directly injected on the second dimension column without giving rise to extra band broadening. Furthermore, solvent incompatibility is more easily handled that way. However, if the amount of sample is limited as in proteomics, high sensitivity is more crucial than increased peak capacity and capillary (0.1–0.5 mm ID)31 columns are commonly used in the first dimension. In some cases incorporation of a column with larger inner diameter is necessary to have sufficient sample capacity. In order to avoid dilution and splitting the flow prior to ESI-MS detection, a narrow inner diameter second dimension column is chosen. Extended length is used to increase the peak capacity. By narrow inner diameters, the volume of each fraction will be smaller and hence the sensitivity will be maintained. This will be further discussed below.
2.1.5 Isocratic and gradient elution.
Due to smaller bandwidths with gradient elution, higher peak capacity is generally obtained with gradient elution compared to isocratic elution within a certain time frame.19,24,26 With gradient elution, all components are eluted within a reasonable analysis time. But how long should a first dimension gradient be? This depends of course on the complexity of the sample and the analytes of interest. In a heart-cut analysis, relative fast gradients can be applied as long as the analytes are separated from the matrix components, while in comprehensive MD LC analysis the gradient slope should be decreased allowing improved separation. The first dimension gradient is often longer than the second gradient, and gradients between 100 and 200 min are common.32,33 Additionally, when fast secondary gradients are used, rapid column re-equilibration is necessary and must be included in the second dimension time window.34
2.1.6 Mobile phase compatibility.
Mobile phase incompatibility between dimensions can influence the actual peak capacity. This is especially important in comprehensive MD LC where the whole first dimension eluent is transferred in fractions to the second dimension.16 If the eluent introduced from the first dimension onto the second dimension column has high elution strength, band broadening will occur and the resolution and hence the peak capacity will be affected. Thus, the mobile phase compatibility has high impact on the total peak capacity in on-line MD LC and should be kept in mind when developing a new on-line MD LC method.
2.1.7 Actual peak capacity.
Since many parameters influence the peak capacity in MD LC analysis, the theoretical peak capacity provides only limited information about the separation potential. Peak capacity is only rarely reported in the literature and if it is, most often the theoretical peak capacity is given. This is perhaps because it is easier to calculate, and constitutes a higher number than the actual peak capacity. More effort should be used to calculate the actual peak capacity; otherwise the peak capacity can only be used as a measure of the instrumental potential and not the separation power of the method.35
2.2 2D LC method development
When developing an on-line 2D LC method for comprehensive purposes, substantial knowledge as well as technical skills are needed due to the requirement of compatibility of LC dimensions. The analytes and sample type decide which separation mechanisms to apply and in order to achieve as much sample information as possible, an 2D LC system with high peak capacity is sought. In heart-cut analysis, it is more important to be able to separate the target compounds from all the matrix compounds, than to obtain high peak capacity.
In both 2D LC techniques, column type and dimension, particle size, mobile phase composition and flow rate applied in both dimensions have to be compatible and optimized in order to maximize the peak capacity. In addition the dimensions of the tubing and valves/connectors used in the 2D LC system need to be compatible with the column dimensions and the flow rates in order to avoid flow inconsistency and extended dilution. Flow inconsistency can especially become a problem in column-switching 2D LC where two or more valves are included and can cause significantly changes in system-back pressure and consequently deviations in mobile phase flow rate and reduction in column lifetime.36 It is also important that the experimental conditions are optimized for minimum dilution in order to have maximum detector response.37 The dilution factor in a 2D LC system is the product of the dilution factor in each dimension and has been reported to be between 200 and 3000.37 However, when trap columns are incorporated for fraction collection, the analytes will be reconcentrated and the dilution factor will therefore be significantly decreased.
In an on-line 2D LC the analytes can be focused on the second dimension column by having a suitable mobile phase composition, volume of the fraction and column dimension. If the mobile phases differ in viscosity, like mixing ACN–water and MeOH–water mobile phases, considerable band broadening may occur and mobile phases with different viscosity should be avoided.16 The second (or last) dimension usually requires compatibility with MS, which is most often used for detection in 2D LC, and with electrospray ionization (ESI) the problem of ion suppression must be handled. When developing an on-line 2D LC method, it can therefore be useful to optimize the last dimension before designing the first dimension instrumental set up by choosing the correct flow rate, mobile phase composition, column dimension and particle size.38
2.3 On-line 2D LC instrumentation
On-line 2D LC can be performed by directly coupled columns or by column switching 2D LC. In column-switching 2D LC, a valve is used to connect the dimensions. With a valve interface, greater flexibility and repeatability is gained and it is easier to achieve compatibility with different separation mechanisms than directly coupled columns.1
2.3.1 Directly coupled 2D LC systems.
Directly coupled 2D LC utilizes simpler instrumentation, having either two columns directly connected or one column packed with segments of different stationary phases. Directly coupled 2D LC unfortunately suffers from limited flexibility, selectivity and compatibility and is therefore not that frequently applied in on-line 2D LC.1 Columns packed with several stationary phases in segments are highly sensitive for dead volumes and are generally more difficult to reproduce.39 Systems with directly coupled MD LC columns with two or three stationary phase segments are called bi- and triphasic and have previously been referred to as semi-comprehensive.2,24 Taylor et al. applied a 2D LC column packed with segments of SCX followed by RP stationary phase for separation of tryptic digested proteins in a MudPIT technology as shown in Fig. 1.40 This column is only utilized in proteomics using nano 2D LC. Peptide elution was carried out with a salt-step gradient combined with a RP-gradient.40 The gradient elution can be very time demanding, gradients up to 600 min have been reported with complex samples.41 The outlet of the column is connected to the nanospray interface for direct MS detection and minimizing the peak dispersion.1 Since the peak capacity is proportional with the plate numbers, the peak capacity will increase with increasing column length or increasing number of directly connected columns, but the peak capacity will level off when more additional columns are connected.39 Fairchild et al. showed that when increasing the column length five times, the obtained peak capacity was approximately the same as when the length was increased ten times.39 In addition to increasing the column length, the gradient time has to be extended as well in order to increase the peak capacity.
 |
| Fig. 1 A biphasic column containing segments of SCX and RP for use in an on-line 2D LC system. | |
2.3.2 Column-switching 2D LC systems.
Column-switching 2D LC is performed by fractionating the first dimension eluent using either storage loops, trap columns or the stop-flow approach, before transferring the fraction to the second dimension for subsequent separation.8 However, it is important that each fraction is completely eluted off the second dimension before transferring the next fraction.16Fig. 2 shows the instrumental set-up of column-switching 2D LC systems with storage loops and trap columns (Fig. 2a and b), parallel second dimension analytical columns (Fig. 2c) and when using the stop-flow approach (Fig. 2d). The on-line 2D LC instruments shown in Fig. 2 can be applied for both comprehensive and heart-cut analyses. The stop-flow mode, is however not relevant for heart-cut target determinations. In comprehensive proteomics and peptidomics where the concentration of the analytes is limited, trap columns and parallel second dimension analytical columns are preferred in order to reconcentrate the analytes prior to the second dimension separation.
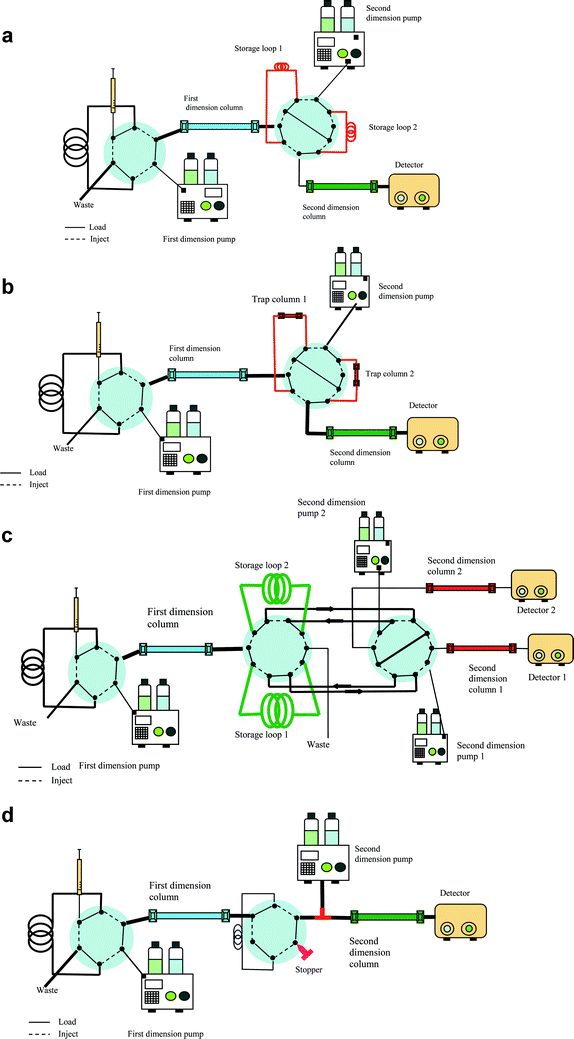 |
| Fig. 2 Column-switching 2D LC instrumentation using a) storage loops b) trap columns c) parallel analytical columns in the second dimension or the d) stop-flow approach for collecting first dimension fractions on-line. | |
Storage loops.
When using multiple storage loops, the loop volumes should be identical.8,16 The storage volume should at least be equal to the volume of each fraction, but due to the band broadening in the connecting tubing, a higher volume is preferred.19 In a 2D LC system with two storage loops only, the content of one loop is transferred for second dimension separation, while the other loop is filled with first dimension eluent.8 With this approach multiple fractionations can be performed. However, the fractionation frequency governs the second dimension separation time; with frequent (less than 1–2 min) fractionation, the second dimension separation including the re-equilibration of the column needs to be very fast.8 Fast second dimension separation can be accomplished by having a short analytical column packed with small particles, elevated temperature or monolithic columns.24,42,43When using higher temperature, the peak shape can be improved and the backpressure of the column will additionally be reduced, allowing faster separation. Fast gradients can be applied on monolithic columns without affecting the resolution, and the columns require short conditioning times.44
Trap columns and parallel analytical columns.
The second dimension peak capacity has a large impact on the total peak capacity and if improved separation is required using longer separation time, storage loops can be replaced by trap columns enabling reconcentration of the components prior to the subsequent separation.8,45 When trap columns are applied for fractionation, the analytes can be focused on the trap column prior to the second dimension separation allowing narrow sample bands to be introduced to the second dimension column. The number of trap columns depends on the number of first dimension fractions and the second dimension separation time, but two trap columns are often applied, as shown in Fig. 2b. In a column-switching 2D LC system with only two trap columns, the analytes retained on the first trap column is transferred (by backflushing) to the second dimension for subsequent analysis, while the next fraction is diverted on the second trap column. When a longer second dimension separation is required two trap columns might, however, not be sufficient and the number has to be increased. Wilson et al. demonstrated the use of a column-switching MD LC system with 18 trap columns.46,47 With this on-line 2D LC instrumentation, several first dimension fractions can be collected on individual trap columns during the separation. The second dimension separation can therefore be longer. However, the total analysis time will be increased. Alternatively, the trap columns can be replaced by two analytical columns that are connected in parallel in the second dimension with individual sample loops (Fig. 2c). With such an on-line 2D LC system, one column is being loaded with a fraction collected from the first dimension, while the components retained on the second column are separated.2
Stop-flow.
A longer second dimension separation can also be accomplished by the stop-flow technique with step gradients.48 In the stop-flow technique, two analytical columns are connected via a valve without using storage loops or trap columns (Fig. 2d).16,19,48,49 By switching the valve, the desired fraction is transferred to the second dimension, the primary flow is stopped and hence the first dimension separation, in order to carry out separation on the second column.16,19,48 After the second dimension separation, the primary flow is restarted to provide another fraction. When using this approach, the second dimension column needs to be longer to obtain a higher plate number compared to on-line 2D LC with continuous flow.16 This column-switching 2D LC approach can be very time demanding compared to the other 2D LC techniques and only few first dimension fractions are therefore collected, but the stop-flow technique with refocusing does not result in additional band broadening.49
2.4 Column dimensions
The column dimensions depend on the application, but also on the mobile phase composition, flow rate and MS compatibility. LC columns with capillary/microflow and nanoflow inner diameters are dominating today due to the high MS compatibility and many life science applications.
2.4.1 Size of the first dimension column.
The actual MD LC peak capacity is dependent on the column size. On-line MD LC is usually applied for analytes present at very low concentrations in complex samples. Thus, a first dimension column with sufficient sample capacity is required for accommodating sufficient injection volumes for trace component determination. This can be accomplished by using conventional sized (2–4 mm ID) columns or alternatively a capillary/microflow or nanoflow column with large-volume injection.46,47 For industrial applications, where the amount of sample rarely is limited, conventional-sized columns are most often used and the injection volume is typically 10 to 1000 μL. When capillary/microflow and nanoflow columns are applied in the first dimension, the transferred fractions are less diluted and more compatible with the second dimension due to the smaller volumetric flow rates, and hence the observed peak capacity is increased.8,10,42 With large-volume injection systems the analytes must be preconcentrated prior to the first dimension separation. Furthermore, when combining narrow inner diameters with longer columns at low flow rates, small volume fractions are provided.8,16
2.4.2 Size of the second dimension column.
While the choice of first dimension column depends on the requirement for sample capacity, the dimension of the second column is dependent on the type of MD LC interface and the detection mode. As a rule of thumb, the second dimension columns should allow fast separation in order to have optimal fractionation rate in the first dimension.34 If storage loops are used as MD LC interface, fast second dimension separation is needed and the column has to be short and effective.8 Conventional sized columns have limited compatibility with MS requiring flow splitting. To prevent compromising MS detection, the inner diameter of the second column should be compatible with MS.2 In some areas like in proteomics, high MS sensitivity is a necessity, and nanoflow columns and even PLOT columns with inner diameters at 10 μm are normally applied.19,50,51 Narrow second dimension columns are also frequently used when focusing trap columns are applied as the interface in MD LC. With trap columns, a fast second dimension separation is not that crucial, and the column can be longer, increasing the peak capacity.26,45 The dimension of the trap columns can typically be 1 × 5 mm or smaller.46,47
As previously mentioned, the mobile phases applied in the different dimensions of on-line MD LC need to be sufficient compatible. The first dimension eluent is required to have a weak elution strength in the second LC dimension to avoid band broadening and to preserve the first dimension separation on the second LC dimension.10,52 Some mobile phase incompatibility can to a certain degree be compensated for by choosing the appropriate column dimensions. For instance, when a nanoflow column is used in the first dimension and a conventional sized column in the second dimension, only a small volume of the first dimension mobile phase is introduced on the second column and with no extra band broadening even when introducing a solvent with high elution strength. Finding optimal combinations of mobile phases in the different dimensions is often the most challenging part in developing MD LC methods.
2.5 Combination of different separation mechanisms in 2D LC
Columns with different separation mechanisms should be selected to achieve the needed separation orthogonality in 2D LC. Mechanisms could include reversed phase (RP), ion-exchange chromatography (IEC) including cation exchange chromatography (CX) and anion exchange chromatography (AX), size exclusion chromatography (SEC) and normal phase chromatography (NP) depending on the analytes and type of sample. All combinations provide high selectivity as well as peak capacity compared to 1D LC. Due to high compatibility with ESI-MS, RP is usually used in the second dimension.
2.5.1 Ion-exchange chromatography-reversed phase chromatography (IEC-RP).
IEC has become very important for separation of both smaller ions and macromolecules, especially in bioanalysis. Whereas CX is mostly used for peptides, AX is preferred for proteins. IEC has compared to RP-LC limited peak capacity, but is well suited for protein analysis due to its nearly non-denaturing elution conditions.53,54
In IEC-RP, components are mainly separated by electrostatic interactions in the first dimension and hydrophobic interactions in the second RP dimension. Ions are eluted from the IEC column by a salt and/or pH gradient.6,55–58 When IEC is used in the first dimension in combination with a salt gradient, the RP column or a trap column will also function as a desalting column prior to MS detection.6,55 With a pH gradient, proteins are eluted according to their isoelectric point (pI).56–58 During pH gradient elution, the components can be focused in narrow bands providing better peak shape than with the salt gradient. Negatively charged components are retained on an AX column when the pH is higher than their pI and will elute when the pH is lower or the same as their pI.53,54,59 The scarcity of MS-compatible buffers represents a problem when IEC is used in the second dimension.53,54,60
SCX-RP is used in on-line 2D LC combinations for many different applications, for instance in peptidomics/proteomics using MudPIT,61–72 for alkaloids,73 traditional Chinese medicine74 and B-vitamins in food analysis.75 The orthogonality between SCX and RP is high, but in addition to electrostatic interactions in the SCX, hydrophobic interactions may take place due to the polymeric backbones of the stationary phase and the orthogonality is slightly reduced.4 Thus for SCX elution, addition of an organic modifier to the mobile phase will decrease the hydrophobic interactions.4 Dependent on the application, the amount of organic modifier may however be restricted. In order to maintain weak elution strength into the second RP dimension, only 5–10% acetonitrile (ACN) or methanol (MeOH) can normally be included in the mobile phase for peptides at a low pH.4
An AX pH gradient usually contains a low concentration of salts and organic modifier and is therefore highly compatible with on-line RP LC. AX-RP is used for separation of macromolecules like proteins,56–58 but also for smaller anions like benzoic acids.76 SAX-RP has also been applied for protein fractionation with on-line transfer to an immobilized trypsin reactor for on-line trypsination and the resulting peptides were subsequently separated on an RP analytical column.58 Ren et al. recently also demonstrated the use of SAX-RP MS for determination of tryptic digested E. coli expressed human IgG Fc fusion protein.77
2.5.2 Size exclusion chromatography-reversed phase chromatography (SEC-RP).
Due to the separation by size in the first dimension, SEC-RP is mostly applied for macromolecules like proteins and polymers, but Hu et al. reported the use of on-line SCX/SEC-RP-MS also for determination of peptides in human serum. Polymers have also been characterized using IEC-SEC in addition to SEC-RP.79,80 The compatibility of SEC-RP mobile phases is relative high, but depending on the analytes. The mobile phase used in SEC can be aqueous and therefore a weak eluent in the second RP dimension.49 In some cases, secondary interactions may occur in aqueous SEC, and MeOH is required in the mobile phase, making the on-line coupling less compatible.10,81 The total peak capacity is limited due to the narrow separation window of SEC. Gilar et al. examined the peak capacity of different 2D LC combinations and reported the peak capacity of a SEC-RP system to be 708 compared to 2430 of SCX-RP.22 SEC with larger inner diameters columns (4.6–7.8 mm)82–85 or several SEC columns connected in series22 can be used to increase the peak capacity. With RP in the first dimension and SEC in the second dimension, in an RP-SEC system, the efficiency and hence the total peak capacity can increase. With RP-SEC the components will be focused in the first dimension and not in the second dimension as in SEC-RP. However, due to the relatively limited peak capacity, few reports on the use of SEC in 2D LC have been published.
2.5.3 Normal phase chromatography-reversed phase chromatography (NP-RP).
NP is highly orthogonal with RP and the combined peak capacity is expected to be high. However, NP mobile phases are often immiscible with the RP mobile phase, and have strong elution strength in RP.10 Due to the requirement of mobile phase compatibility in on-line 2D LC, special interfaces are required, and the NP-RP combination is consequently rarely reported. 2D LC analyses with NR-RP have been performed using RP mobile phases in both dimensions in order to avoid incompatible mobile phases, but the orthogonality was reported to be limited.86 Tian et al. used a loop interface with incorporated vacuum evaporation for analysis of traditional Chinese medicine.87,88 A ten port valve with two storage loops was used as the interface between the first NP dimension and the second RP dimension.87,88 Eluent from the first dimension filled one of the loops and was subsequently evaporated at 25 °C under vacuum in line. During evaporation, the analytes were adsorbed to the inner side of the loop.87,88 Subsequently, the second dimension mobile phase desorbed and transferred the analytes for second dimension separation. The instrumentation in this approach is more complicated than other on-line instrumentations, the loops need to be washed carefully between analyses and must be made in materials that are compatible with both the first and second dimension mobile phase. This approach can only be applied for volatile solvents and the analytes can be lost due to poor solubility in the second dimension mobile phase. The alternative to a loop evaporation interface can be a low flow rate (8 μL/min) in the first dimension and a high flow rate (4 mL/min) in the second dimension.89 As a result, only a small aliquot of the non-polar NP mobile phase is introduced on the RP-column and the analytes are focused on the column keeping the band broadening at a minimum.89
A variant of NP chromatography, hydrophilic interaction liquid chromatography (HILIC) appears to be more compatible with RP than the ordinary NP. HILIC can be characterized as NP, with hydrophilic functional groups as the stationary phase, applying aqueous mobile phases, most often with ACN as the organic component.90 Because of different separation mechanisms, the orthogonality of a HILIC-RP 2D LC system is high resulting in high theoretical peak capacity. Gilar et al. determined the peak capacity of a HILIC-RP system to be 4616, approximately twice as high as SCX-RP.22 Improved resolution and peak capacity compared to SCX-RP were also reported by Mihailova et al. for separation of neuropeptides in rat brain tissue.46 The HILIC mobile phase contains a high percentage of organic modifier, and analyte focusing on the second dimension might be difficult in an on-line coupling. However, by applying a rather narrow first dimension column and low flow rate, while introducing a larger inner diameter column in the second dimension and higher flow rate, the eluent is diluted and only a small percentage of organic solvent is introduced into the second dimension. Alternatively, a diluting pump can be placed between the two dimensions in order to decrease the percentage of organic modifier before transfer to the RP-column with the same dimensions as the HILIC column, as shown in Fig. 3.46,47,91 When applying an instrumental set-up like this, the volumetric flow rate of the diluting pump must be considered and must not be too high in order to avoid breakthrough on the trap columns serving as the interface in column-switching 2D LC.
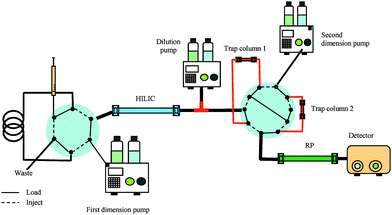 |
| Fig. 3 An on-line HILIC-RP column-switching system with two trap columns, using a diluter pump between the first and second dimension in order to dilute the HILIC eluate containing a high percentage with ACN. | |
To obtain phase focusing on a HILIC column in order to increase sample load, the analytes have to be dissolved in a high percentage of organic modifier, like acetonitrile. Nevertheless, polar compounds like peptides have limited solubility in acetonitrile and prefer more aqueous solvents. This can be arranged by connecting a small RP SPE column connected to the 2D LC system prior to the HILIC column for injecting aqueous samples.46,47 On-line HILIC-RP have recently been applied for separation of samples containing peptides46,47,55 and drugs.91,92 Due to the compatibility with MS, HILIC can also be applied in the second dimension for determination of e.g. peptides.93,94
2.5.4 Reversed phase chromatography-reversed phase chromatography (RP-RP).
Even though it is more common to apply different separation mechanisms in 2D LC, RP LC can be utilized in both dimensions by using different RP columns or mobile phase pH. The separation will then be based on hydrophobic interactions in both dimensions, but with different selectivity. When applying different mobile phase pH or organic modifier, the RP selectivity difference is due to pH dependent solubility or other interactions.22,95–97 In contrast, when using two different RP columns, the provided selectivity difference and hence orthogonality, is limited.22 Higher peak capacity is obtained using different mobile phases.22 Gilar et al. reported the peak capacity of an RP-RP 2D LC system with two different mobile phase pHs to be higher, compared to using two different types of RP stationary phase analytical columns with the same mobile phase pH and other on-line 2D LC systems including SCX-RP, HILIC-RP, SCX-HILIC and SEC-RP.19,22 The higher peak capacity of RP-RP is due to increased separation power in the first dimension.19 On the other hand, mobile phase incompatibility may also be a problem in RP-RP 2D LC.2,16 Since similar mobile phases are applied in RP-RP, it is especially crucial that the first dimension eluent has weak elution strength in the second dimension. This can be arranged by isocratic elution at a low percentage of organic modifier in the first dimension, combined with a higher percentage in the second dimension.43,98 However, higher peak capacity is obtained with gradient elution and is most often required for complex samples.42 A gradient with a rather low percentage of organic modifier can be employed in the first dimension and a gradient increasing to a higher percentage is used in the second dimension. Alternatively, a diluter pump can be incorporated between the dimensions to dilute the organic modifier prior to the second dimension. A third option is to incorporate an analytical column with smaller inner diameter in the first dimension and a column with larger inner diameter in the second dimension.43 Thus, for some applications it can be more practical to perform this 2D LC approach off-line rather than on-line. RP-RP LC has been applied for different samples containing natural antioxidants and drugs,18,44,99–103 peptides,97,104 flavonoids and phenolic acids,105 prostaglandins106 as well as plant extracts.98,107–109
Even though 2D LC provides higher peak capacity than 1D LC, the resolution might still be too limited for highly complex samples. Thus, a third dimension can be incorporated to increase the peak capacity even more.
The 3D LC separation requires the choice of three independent and mutually orthogonal separation dimensions. Unfortunately, the selection of three types of LC modes with sufficient orthogonal selectivity and mobile phase compatibility is a difficult task.110 It will also be more complicated to obtain compatibility between column dimensions, mobile phase compositions and flow rates. Additionally, studies have shown that when incorporating a third LC dimension, the peak capacity does not necessarily improve significantly compared to 2D LC. This may be the case when storage loops are used to collect fractions from the first dimension applying fast second dimension separations. Then, the third separation has to be carried out even faster than the second dimension separation and consequently the peak capacity for each dimension will decrease.110 The amount of data that will be generated with 3D LC will be extensive and problematic to handle even though new and improved software for data acquisition and processing likely will be developed in the future.110 Only a few studies have been reported to be on-line 3D LC.111,112 In two studies the reported 3D LC separation was performed using an triphasic column with segments of RP, SCX and RP.111,112 With this on-line 3D LC system, no additional valves and pumps are incorporated making the system simpler and easier to handle.
4. Recent applications
As mentioned above, on-line 2D LC is widely used for separation in life science applications like peptidomics/proteomics as well as for food and industrial applications. Some of the recently published applications (from 2007 to September 2009) are included in this review.
Two-dimensional gel electrophoresis is widely used for separating proteins but this method is not always suited e.g. not for membrane proteins. LC has therefore become an alternative method to 2D gel electrophoresis in proteomics. However, proteins require special conditions for solubilization to prevent denaturation. Hence in most cases, proteins are digested prior to on-line MD LC analysis, since peptides are easier to handle, in a bottom-up approach. Alternatively, the proteins can be separated in the first dimension before on-line digestion and subsequent second dimension peptide separation, as reported by Tran et al.58,113 Far more studies using MD LC for separation of digested proteins (peptides) compared to separation of intact proteins have been published.
The traditional MudPIT strategy with SCX-RP for peptide separation can be used for protein separation as well.72,114–116 Gao et al. used this approach to deplete high-abundance proteins in human liver tissue.72 After protein depletion of the 53 most abundant proteins, the remaining proteins were digested before subsequent peptide separation.72 As previously mentioned, AX is often preferred for protein separation due to high capacity and almost non-denaturating elution conditions.53,54 AX has been combined with RP for identification and quantification of degradation products in E. coli and determination of apolipoproteins in human plasma.113,57,56,77 Tran et al. demonstrated the use of an SAX-RP system with on-line trypsination.113 Native proteins were separated on a SAX column using a pH gradient, before fractionation on C4 trap columns with on-column reduction and alkylation. Nine fractions were collected in total. The reduced and alkylated proteins were subsequently separated on a RP column followed by on-line digestion and ESI-MS detection.113 The reduction, alkylation and tryptic digestion step were carried out using the stop-flow approach. Even though this on-line instrumentation is fairly complicated, both protein separation and digestion can be performed on-line minimizing the sample handling. The total time for each analysis was reported to be 320 min for one fraction and with additional 60 min for each fraction. Although, this is a quite time consuming MD LC method, the total time is still reduced compared to the traditional off-line methods including protein digestion procedure which is commonly done overnight.
AX has also been combined with HILIC for separating derivatives of N-glycans.117 The AX and HILIC columns were directly coupled and a mobile phase containing water, ACN and ammonium acetate was used.117 With a step-gradient, four fractions in total were transferred to the HILIC column.117
Depletion of high abundant proteins has also been carried out using RP in combination with columns packed with restricted access material (RAM) or single or tandem immunoaffinity columns.118–120 Different immunoaffinity columns can be combined depending on which proteins are most abundant. The depletion is never complete, but is still reported to be very high (99.7%).120
Since the concentration of most proteins is usually low and necessitates a compatible MS detection, capillary/microflow and nanoflow columns have become a requirement in proteomics. However, peptides are easier to determine and proteins can therefore be digested after the on-line 2D LC separation with an additional LC separation and MS detection. Because of high complexity it is also important to use an MD LC method with high peak capacity. Trap columns are often used to collect fractions in order to have an additional preconcentration prior to the second dimension separation.
Peptides, either as digested proteins in proteomics or endogenous as in peptidomics, are frequently separated by on-line MD LC applying different combinations of separation mechanisms. However, the method of choice is often the MudPIT strategy using SCX in the first dimension and RP in the second dimension.40,51,61–72,78,121–128
As an alternative to MudPIT, Motoyama et al. demonstrated the use of a mixed-bed ion exchange column with a combination of AX and CX to improve peptide recovery and detection compared to only SCX-RP.4 The reported increased recovery is due to the added retention of acidic peptides in addition to the basic and neutral peptides, but the retention of basic and neutral peptides was slightly reduced.4 Even though AX is more used for protein analysis, a fully automated AX-RP system was used to separate and identify phosphopeptides in HeLa cells using a pH gradient in the first dimension.129 More than 1800 phosphopeptides were identified and when comparing the AX-RP method with another using combined cation exchange and titanium dioxide, the AX-RP system identified more acidic and multiphosphorylated peptides.129 As mentioned earlier, RP in combination with HILIC has demonstrated increased peak capacity compared to SCX-RP and has therefore also been applied for separation of peptides.46,47,93
Francois et al. applied an RP-RP LC system with different mobile phase pH using the same RP analytical columns for separation of tryptic digested BSA and human blood serum.97 The pH in the first dimension was 1.8, while the pH in the second dimension was 10.97 Acidic peptides were retained more on the first RP dimension at lower pH, while the basic peptides have stronger retention on the second RP dimension at higher mobile phase pH.97 The selectivity of neutral peptides also altered with changing pH.97 The authors reported this 2D LC system to be robust and could also be applied with high pH in the first RP dimension mobile phase and low pH in the second dimension.97 The peak capacity of peptides from digested BSA was determined to be 4677.97 Peptide analysis can also be performed using RP-RP LC with different analytical columns, like a combination of a C5 column and a C18 column.104 This RP-RP 2D LC combination was applied for determination of C-peptide, a biomarker for insulin secretion and can be sufficient for target analysis, but the peak capacity will not be sufficient for comprehensive analysis.104 Thus even though the MudPIT strategy is most often used for peptides, alternative combinations of separation mechanisms have been introduced showing similar as well as improved peptide separation and identification as compared to SCX-RP.
Capillary/microflow and nanoflow columns are most often used for peptides, this is because the concentration of the peptides is often low and the flow rate has to be compatible with MS which has become a necessity for peptide identification. Both storage loops and trap columns are used for collecting first dimension fractions prior to the second dimension separation. The number of collected fractions differs and depends on the complexity of the sample.
Food constitutes a complex matrix and contains many different components like lipids, proteins, vitamins, antioxidants, water and minerals.7 Due to the content and direct human impact, food analyses are important and most often comprehensive approaches are applied rather than heart-cut, particularly in the area of lipids.7
Saturated, unsaturated and trans fatty acids have a large impact on blood serum and cholesterol levels. Thus, it has become a requirement that the trans fat content must be listed on nutrition fact labels for packaged food.130 One such type of trans fat is triacylglycerols (TAGs).130 Recently, TAGs separation in different plant oils have been carried out using a silver-ion coated CX column as the primary column and a RP column in the second dimension.131 This method has become one of the most common MD LC techniques for quantification of TAGs.131,130 A MeOH–ACN containing gradient was used to elute the TAGs from the first dimension column.131 The second dimension mobile phase contained MeOH and tert-butyl methyl ether (70/30, v/v) obtaining high compatibility with the first dimension mobile phase. The authors determined more than 40 TAGs in corn oil.131
Carotenoids found in plant-derived food and products have been described to have beneficial health properties like antioxidant activity and prevention of cancer and have therefore attracted increased attention recently.32,33 Dugo et al. determined the carotenoids composition in red orange and mandarin oil using on-line NP-RP comprehensive LC.32,33 In the first dimension, the carotenoids were separated by NP LC using either a cyano based column or a silica column. When using the cyano column, the mobile phase contained n-hexane, butylacetate and acetone, while a mixture of n-hexane and ethyl alcohol was used in combination with the silica column. A two-minute second dimension gradient was accomplished using a monolithic RP column.32,33 The authors detected more than 20 and 40 carotenoids in mandarin and red orange oil, respectively.32,33 A similar comprehensive NP-RP LC system was used to analyze lemon oil extracts.48 The first dimension separation was carried out with a gradient of n-hexane and ethyl acetate using a diol-based NP column, while two C18-analytical columns using a mobile phase containing ACN/water were used for the second dimension separation.48
Polyphenols present in skins and seeds in grapes and berries, have also shown antioxidant effects.18,99,102 Recently, samples containing phenolic compounds have been analyzed using RP-RP LC with different RP stationary phases and either UV or MS for detection.18,102 Column-switching MD LC with storage loops, trap columns and the stop-flow approach has been applied to determine polyphenols in juice and wines.99,102,18,132 When using the stop-flow technique, after separation on the first dimension C18 column (250 × 4.6 mm ID), ten fractions were subsequently transferred to the second dimension carbon based column.18 The first dimension mobile phase contained MeOH and 10 mM ammonium acetate, while MeOH and phosphate buffer was used in the second dimension.18 As demonstrated above, different combinations of separation mechanisms are used in food analysis depending on the properties of the analytes. In most cases, mobile phase compatibility is obtained having a larger second dimension column compared to the first dimension analytical column. Storage loops are often used to connect the two dimensions and the number of collected fractions varies from 30 to 120.
4.4 Plant extracts analysis
Plant extracts contain hundreds of different components at various concentration levels with different pharmaceutical and toxic properties. Different approaches have been applied, but due to the improved peak capacity of MD LC, this is frequently used. Depending on the properties of the analytes, different MD LC combinations have been reported e.g. NP-RP,87,88,133 RP-RP48,134,135 and RP in combination with IEC.74,136,137 The main problem with NP-RP MD LC is the mobile phase compatibility between dimensions. However, Wei et al. added 1,4-dioxane, which is a water soluble and non-polar solvent, to n-hexane (99.5/0.5, v/v) in the first dimension mobile phase to overcome the compatibility problem with the RP mobile phase for analysis of plant extracts using a stop-flow NP-RP LC system.133 A silica-based analytical column was applied for the first dimension separation. In this MD LC system, the inner diameter of the analytical columns were the same in both dimensions, but two RP columns, 25 cm each, were connected in series to improve the peak capacity. A linear gradient of isopropanol/water and MeOH was applied in the second separation.133 Thirty fractions were collected in storage loops before the subsequent second dimension separation.
Kivilompolo et al. explored different stationary phases for analyzing the components in a herb matrix.135 The most promising comprehensive MD LC combination appeared to be RP-RP interfaced to MS applying different RP stationary phases, C18 and CN in the first and second dimension, respectively.135 Chen et al. applied a comprehensive SAX-RP UV-MS method for identification of compounds in a Chinese medicine.137 Fifty-eight components were identified using a gradient of MeOH and phosphate buffer in the first dimension and a fast gradient with ACN and 0.1% acetic acid in the second.137
Typically, when analyzing plant extracts the number of collected fractions varies from 10 to 60, depending on the complexity of the sample. If the separation requires an extended second dimension separation, storage loops can be replaced with trap columns or the stop-flow approach. In most applications RP is used in one or both LC dimensions.
4.5 Polymers and industrial products
Polymers, like various acrylates and methacrylates, are frequently used in cosmetics such as mascara, nail enamels and hair dyes.138 Such polymers have large distributions in molecular mass and chemical composition.14,138 The increased selectivity obtained with MD LC is therefore advantageous when analyzing polymers and other complex industrial products, and SEC is often used in combination with NP or RP.16 In heart-cut MD LC, SEC is mostly applied in the first dimension whereas NP or RP is applied in the second dimension.16 When SEC is used in the first dimension, the eluent which often contains tetrahydrofuran (THF), has high elution strength and may affect the second separation.14 On the other hand, in comprehensive MD LC, SEC can be advantageously used in the second dimension, and NP or RP in the first dimension to improve the separation according to the composition of the polymers.16 With such an instrument set-up, higher sample capacity of the first dimension is obtained.14
Raust et al. used a fully automated on-line RP-SEC 2D LC system with storage loops when analyzing a mixture of acrylate and methacrylate esters.138 The first dimension separation was carried out using a RP column (150 × 4.6 mm ID) with an ACN–THF gradient.138 The eluate was collected in two storage loops, and in total 109 fractions were transferred to the SEC column (150 × 7.5 mm ID) for subsequent separation. An on-line SEC-NP-UV system with storage loops was used for quantitative characterization of solid epoxy resins.83 The mobile phase in both dimensions contained MeOH and dichloromethane.83
When analyzing polymer samples using on-line MD LC, SEC is generally the separation mechanism of choice in combination with either RP or NP. Usually, large amounts of sample are available and the inner diameter of the columns is therefore often large (4.6–7.5 mm). Mobile phase compatibility is accomplished using columns with different inner diameters. Storage loops are most often used for on-line fractionation and combining the LC dimensions.
4.6 Other applications
Prostaglandins and metabolites in human plasma have been separated using an MD LC system with two different RP columns with MS detection.106 In addition to different stationary phases, increased selectivity and resolution were obtained using MeOH in the first dimension and ACN for the second dimension separation.106 One RP trap column was used to preconcentrate the analytes prior to the second dimension. A 2D LC system with two different RP columns was also used to analyze a mixture of phenolic compounds and flavones.42 The analytical column in the first dimension was 2.1 × 150 mm, while the second dimension analytical column was 4 × 30 mm.42 The flow rates were 50 μL/min and 2 mL/min, respectively. Matching parallel gradients were used in both dimensions and hence no second dimension column re-equilibration was necessary. A ten-port valve with two storage loops was used to combine the two dimensions and 70 fractions were collected in total.42
On-line MD LC can also be used for determination of drugs. The activity of a drug component can be dependent of the component configuration, ketorolac is a non-steroidal anti-inflammatory drug where the (−)S enantiomer is responsible for the anti-inflammatory and analgesic activity, while the (+)R enantiomer has little or no activity.101,128 Thus, in order to determine the different isomers of ketorolac, Ing-Lorenzini et al. used a heart-cut MD LC-MS method with a RP-C18 column in the first dimension and a chiral column in the second dimension.101 The whole analysis was carried out in less than 20 min, using a fast first dimension gradient and isocratic elution in the second dimension. A similar MD LC approach, only comprehensive, was applied to determine carvedilol, a non-selective beta blocker, in plasma samples.139 Alternatively, drug samples can be analyzed using RP-RP with two different stationary phases.100
5. Future aspects of on-line MD LC
On-line MD LC will most probably become more frequently utilized in the future, due to the increasing need for automatic, high throughput comprehensive and target analysis of complex samples. At present, miniaturized on-line MD LC systems are becoming more common with capillary/microflow and nanoflow columns due to the increasing need of detecting different components at very low concentration levels and such systems will be more frequently applied also in routine analyses. Miniaturization is especially important in proteomics, peptidomics and possibly metabonomics where the available sample amount is limited, and the target analytes are present in low concentration. On the other hand, for food and polymer analyses where there are no sample limitations, second dimension columns with narrow inner diameter are not that crucial. Hence, in these fields standard size column will continue to be used unless environmental requirements force laboratories to minimize the columns (and use split-free pumping systems to spend less solvent).
Many on-line MD LC methods are reported using fast second dimension separation reducing the total analysis time. Thus, monolithic columns or UPLC systems will be more frequently incorporated in the second dimension. Even though the availability of monolithic columns so far has been limited, these columns have gained much attention due to the potential higher performance compared to particle-packed columns. Thus, we believe that the quest for monolithic columns will increase, leading to more commercial products. UPLC, on the other hand suffers from lack of suitable hardware like low dead volume high pressure valves, but when such valves become more easily available there is no reason why UPLC could not be included more frequently in on-line MD LC. However, depending on the sample and the goal, it is not possible to substantially decrease the time of analysis and still obtain high total peak capacity. The total run time will always be dependent on the first and second dimension elution as well as the fractionation frequency. Thus, a compromise has to be made between the peak capacity and time.
Even though some have started to use 3D LC, due to the limitations with orthogonality, compatibility between dimensions, data processing and instrumental complexity compared to 2D LC, 3D LC is presently not widely used. 2D LC also still suffers from limitations which have to be overcome. One such problem is data processing and presentation. At present, software with statistical and data-visualizing tools is often incorporated with the data acquisition using MS for detection but not that often for other detection methods like UV. Statistical calculations have become a necessity in comparison-studies when e.g. searching for potential biomarkers for cancer or other diseases in a large number of samples, but the user friendliness of such software should in some cases be improved.
When analyzing a large number of samples, it is advantageous to use a fully automated on-line MD LC system with limited manual sample handling. Hence, in the future we will see that many off-line MD LC methods will be replaced with on-line MD LC methods, also in order to save time. Presently, only a few commercial MD LC instrumentations are reported to be fully automated. However, we believe that more fully automated on-line 2D LC methods will be reported in the near future and more effort will be made to overcome the present limitations with on-line 2D LC in order to perform high throughput analyses.
6. References
- C. R. Evans and J. W. Jorgenson, Anal. Bioanal. Chem., 2004, 378, 1952–1961 CrossRef CAS.
- K. Sandra, M. Moshir, F. D'Hondt, R. Tuytten, K. Verleysen, K. Kas, I. Francois and P. Sandra, J. Chromatogr., B: Anal. Technol. Biomed. Life Sci., 2009, 877, 1019–1039 CrossRef CAS.
- L. N. Waller, K. Shores and D. R. Knapp, J. Proteome Res., 2008, 7, 4577–4584 CrossRef CAS.
- A. Motoyama and J. R. Yates, Anal. Chem., 2008, 80, 7187–7193 CrossRef CAS.
- R. E. Majors, LCGC North Am., 2008, 70–73 CAS.
- R. Tomas, K. Kleparnik and F. Foret, J. Sep. Sci., 2008, 31, 1964–1979 CrossRef CAS.
- P. Dugo, T. Kumm, F. Cacciola, G. Dugo and L. Mondello, J. Liq. Chromatogr. Relat. Technol., 2008, 31, 1758–1807 CrossRef CAS.
- P. Jandera, J. Sep. Sci., 2008, 31, 1421–1437 CrossRef CAS.
- J. Tang, M. Gao, C. Deng and X. Zhang, J. Chromatogr., B: Anal. Technol. Biomed. Life Sci., 2008, 866, 123–132 CrossRef CAS.
- P. Dugo, F. Cacciola, T. Kumm, G. Dugo and L. Mondello, J. Chromatogr., A, 2008, 1184, 353–368 CrossRef CAS.
- M. L. Fournier, J. M. Gilmore, S. A. Martin-Brown and M. P. Washburn, Chem. Rev., 2007, 107, 3654–3686 CrossRef CAS.
- F. David, G. Vanhoenacker, B. Tienpont, I. Francois and P. Sandra, LC-GC Eur., 2007, 20, 154–158 CAS 160–162.
-
C. R. Evans and J. W. Jorgenson, in Multidimensional liquid chromatography theory and applications in industrial chemistry and the life sciences, ed. S. A. Cohen and M. R. Schure, John Wiley & Sons, Inc., Hoboken, Editon edn, 2008, pp. 177–204 Search PubMed.
-
F. Rittig and H. Pasch, in Multidimensional liquid chromatography theory and applications in industrial chemistry and the life sciences, ed. S. A. Cohen and M. R. Schure, John Wiley & Sons, Inc., Hoboken, Editon edn, 2008, pp. 387, 394, 387–423 Search PubMed.
- T. Stroink, M. C. Ortiz, A. Bult, H. Lingeman, G. J. de Jong and W. J. M. Underberg, J. Chromatogr., B: Anal. Technol. Biomed. Life Sci., 2005, 817, 49–66 CrossRef CAS.
- P. Jandera, J. Sep. Sci., 2006, 29, 1763–1783 CrossRef CAS.
- L. Blumberg and M. S. Klee, J. Chromatogr., A, 2010, 1217, 99–103 CrossRef CAS.
- F. Cacciola, P. Jandera and L. Mondello, Chromatographia, 2007, 66, 661–667 CrossRef CAS.
- I. Francois, K. Sandra and P. Sandra, Anal. Chim. Acta, 2009, 641, 14–31 CrossRef CAS.
- J. M. Davis, D. R. Stoll and P. W. Carr, Anal. Chem., 2008, 80, 8122–8134 CrossRef CAS.
- G. Guiochon, L. A. Beaver, M. F. Gonnord, A. M. Siouffi and M. Zakaria, J. Chromatogr., A, 1983, 255, 415–437 CrossRef CAS.
- M. Gilar, P. Olivova, A. E. Daly and J. C. Gebler, Anal. Chem., 2005, 77, 6426–6434 CrossRef CAS.
- X. Li, D. R. Stoll and P. W. Carr, Anal. Chem., 2009, 81, 845–850 CrossRef CAS.
- R. A. Shellie and P. R. Haddad, Anal. Bioanal. Chem., 2006, 386, 405–415 CrossRef CAS.
- R. E. Murphy, M. R. Schure and J. P. Foley, Anal. Chem., 1998, 70, 1585–1594 CrossRef CAS.
- K. Horvath, J. N. Fairchild and G. Guiochon, Anal. Chem., 2009, 81, 3879–3888 CrossRef CAS.
- D. R. Stoll, J. D. Cohen and P. W. Carr, J. Chromatogr., A, 2006, 1122, 123–137 CrossRef CAS.
- S. Eeltink, S. Dolman, R. Swart, M. Ursem and P. J. Schoenmakers, J. Chromatogr., A, 2009, 1216, 7368–7374 CrossRef CAS.
- J. M. Davis, D. R. Stoll and P. W. Carr, Anal. Chem., 2008, 80, 461–473 CrossRef CAS.
- K. Horvath, J. Fairchild and G. Guiochon, J. Chromatogr., A, 2009, 1216, 2511–2518 CrossRef CAS.
- Y. Saito, K. Jinno and T. Greibrokk, J. Sep. Sci., 2004, 27, 1379–1390 CrossRef CAS.
- P. Dugo, M. Herrero, D. Giuffrida, T. Kumm, G. Dugo and L. Mondello, J. Agric. Food Chem., 2008, 56, 3478–3485 CrossRef CAS.
- P. Dugo, M. Herrero, T. Kumm, D. Giuffrida, G. Dugo and L. Mondello, J. Chromatogr., A, 2008, 1189, 196–206 CrossRef CAS.
-
R. E. Murphy and M. R. Schure, in Multidimensional liquid chromatography theory and applications in industrial chemistry and the life sciences ed. S. A. Cohen and M. R. Schure, John Wiley & Sons, Inc., Hoboken, Editon edn, 2008, p. 137 Search PubMed.
-
J. M. Davis, in Multidimensional liquid chromatography theory and applications in industrial chemistry and the life sciences, ed. S. A. Cohen and M. R. Schure, John Wiley & Sons, Inc., Hoboken, Editon edn, 2008, pp. 49–50 Search PubMed.
- E. Rogatsky, K. Braaten, G. Cruikshank, H. Jayatillake, B. Zheng and D. Stein, J. Chromatogr., A, 2009, 1216, 7721–7727 CrossRef CAS.
- K. Horvath, J. N. Fairchild and G. Guiochon, J. Chromatogr., A, 2009, 1216, 7785–7792 CrossRef CAS.
-
R. E. Murphy and M. R. Schure, in Multidimensional liquid chromatography theory and applications in industrial chemistry and the life sciences, ed. S. A. Cohen and M. R. Schure, John Wiley & Sons, Inc., Hoboken, Editon edn, 2008, p. 132 Search PubMed.
- J. N. Fairchild, K. Horvath and G. Guiochon, J. Chromatogr., A, 2009, 1216, 6210–6217 CrossRef CAS.
- P. Taylor, P. A. Nielsen, M. B. Trelle, O. B. Horning, M. B. Andersen, O. Vorm, M. F. Moran and T. Kislinger, J. Proteome Res., 2009, 8, 1610–1616 CrossRef CAS.
- M. P. Washburn, D. Wolters and J. R. Yates, 3rd, Nat. Biotechnol., 2001, 19, 242–247 CrossRef CAS.
- P. Jandera, P. Cesla, T. Hajek, G. Vohralik, K. Vynuchalova and J. Fischer, J. Chromatogr., A, 2008, 1189, 207–220 CrossRef CAS.
-
T. Ikegami, H. Aoki, H. Kimura, K. Hosoya and N. Tanaka, in Multidimensional liquid chromatography theory and applications in industrial chemistry and the life sciences, ed. S. A. Cohen and M. R. Schure, John Wiley & Sons, Inc., Hoboken, Editon edn, 2008, pp. 147, 160, 163 Search PubMed.
- F. Cacciola, P. Jandera, Z. Hajdu, P. Cesla and L. Mondello, J. Chromatogr., A, 2007, 1149, 73–87 CrossRef CAS.
- K. Sandra, M. Moshir, F. D'Hondt, K. Verleysen, K. Kas and P. Sandra, J. Chromatogr., B: Anal. Technol. Biomed. Life Sci., 2008, 866, 48–63 CrossRef CAS.
- A. Mihailova, H. Malerod, R. Wilson Steven, B. Karaszewski, R. Hauser, E. Lundanes and T. Greibrokk, J. Sep. Sci., 2008, 31, 459–467 CrossRef CAS.
- S. R. Wilson, M. Jankowski, M. Pepaj, A. Mihailova, F. Boix, G. V. Truyols, E. Lundanes and T. Greibrokk, Chromatographia, 2007, 66, 469–474 CrossRef CAS.
- I. Francois, A. de Villiers, B. Tienpont, F. David and P. Sandra, J. Chromatogr., A, 2008, 1178, 33–42 CrossRef CAS.
- F. Bedani, W. T. Kok and H.-G. Janssen, J. Chromatogr., A, 2006, 1133, 126–134 CrossRef CAS.
- S. Mirabaud, C. Rolando and M. Regert, Anal. Chem., 2007, 79, 6182–6192 CrossRef CAS.
- Q. Luo, Y. Gu, S.-L. Wu, T. Rejtar and B. L. Karger, Electrophoresis, 2008, 29, 1604–1611 CrossRef CAS.
- E. Hogendoorn, P. Van Zoonen and F. Hernandez, LC-GC Eur., 2003, 16, 44–51 CAS.
- M. Pepaj, S. R. Wilson, K. Novotna, E. Lundanes and T. Greibrokk, J. Chromatogr., A, 2006, 1120, 132–141 CrossRef CAS.
- M. Pepaj, A. Holm, B. Fleckenstein, E. Lundanes and T. Grelbrokk, J. Sep. Sci., 2006, 29, 519–528 CrossRef CAS.
- P. J. Boersema, N. Divecha, A. J. R. Heck and S. Mohammed, J. Proteome Res., 2007, 6, 937–946 CrossRef CAS.
- M. Pepaj, E. Lundanes and T. Greibrokk, J. Liq. Chromatogr. Relat. Technol., 2007, 30, 1879–1894 CrossRef CAS.
- B. Q. Tran, M. Pepaj, E. Lundanes and T. Greibrokk, Chromatographia, 2007, 66, 709–715 CrossRef CAS.
- B. Q. Tran, M. Pepaj, E. Lundanes and T. Greibrokk, J. Liq. Chromatogr. Relat. Technol., 2008, 31, 1387–1411 CrossRef CAS.
- T. Andersen, M. Pepaj, R. Trones, E. Lundanes and T. Greibrokk, J. Chromatogr., A, 2004, 1025, 217–226 CrossRef CAS.
- L. Shan and D. J. Anderson, Anal. Chem., 2002, 74, 5641–5649 CrossRef CAS.
- C. I. Balog, P. J. Hensbergen, R. Derks, J. J. Verweij, G. J. van Dam, B. J. Vennervald, A. M. Deelder and O. A. Mayboroda, Clin. Chem., 2009, 55, 117–125 CAS.
- R. C. Dwivedi, V. Spicer, M. Harder, M. Antonovici, W. Ens, K. G. Standing, J. A. Wilkins and O. V. Krokhin, Anal. Chem., 2008, 80, 7036–7042 CrossRef CAS.
- F. F. Evans, M. J. Raftery, S. Egan and S. Kjelleberg, J. Proteome Res., 2007, 6, 967–975 CrossRef CAS.
- M. Gilar, Y.-Q. Yu, J. Ahn, J. Fournier and J. C. Gebler, J. Chromatogr., A, 2008, 1191, 162–170 CrossRef CAS.
- J. A. Dowell, D. C. Frost, J. Zhang and L. Li, Anal. Chem., 2008, 80, 6715–6723 CrossRef CAS.
- P. A. Kirkland, M. A. Humbard, C. J. Daniels and J. A. Maupin-Furlow, J. Proteome Res., 2008, 7, 5033–5039 CrossRef CAS.
- J. H. Prieto, S. Koncarevic, S. K. Park, J. Yates, III and K. Becker, PLoS One, 2008, 3 Search PubMed No pp given.
- E. P. Romijn and J. R. Yates, III, Methods Mol. Biol., 2008, 432, 1–16 CAS.
- H. Yuan, L. Zhang, W. Zhang, Z. Liang and Y. Zhang, Fenxi Ceshi Xuebao, 2008, 27, 227–230 Search PubMed.
- T. Umemura and H. Kobayshi, Chromatography, 2009, 30, 43–44 CAS.
- J. F. Kelly and W. Ding, Methods Mol. Biol., 2008, 439, 257–267 CAS.
- M. Gao, C. Deng, W. Yu, Y. Zhang, P. Yang and X. Zhang, Proteomics, 2008, 8, 939–947 CrossRef CAS.
- C. V. Hoffmann, M. Laemmerhofer and W. Lindner, Anal. Bioanal. Chem., 2009, 393, 1257–1265 CrossRef CAS.
- T. Tang, W.-B. Zhang, T. Li and F.-Y. Wang, Fenxi Huaxue, 2007, 35, 1767–1771 CAS.
- R. J. Goldschmidt and W. R. Wolf, J. AOAC Int., 2007, 90, 1084–1089 CAS.
- T. Leitner and C. W. Klampfl, J. Liq. Chromatogr. Relat. Technol., 2008, 31, 169–178 CrossRef CAS.
- D. Ren, G. Ratnaswamy, J. Beierle, M. J. Treuheit, D. N. Brems and P. V. Bondarenko, Int. J. Biol. Macromol., 2009, 44, 81–85 CrossRef CAS.
- L. Hu, K.-S. Boos, M. Ye, R. a. Wu and H. Zou, J. Chromatogr., A, 2009, 1216, 5377–5384 CrossRef CAS.
- K. Im, H.-w. Park, S. Lee and T. Chang, J. Chromatogr., A, 2009, 1216, 4606–4610 CrossRef CAS.
- V. Mass, V. Bellas and H. Pasch, Macromol. Chem. Phys., 2008, 209, 2026–2039 CrossRef CAS.
- B. Winther, J. Leon and E. Reubsaet, J. Sep. Sci., 2005, 28, 477–482 CrossRef CAS.
- L. M. de Souza, T. R. Cipriani, C. F. Sant'Ana, M. Iacomini, P. A. J. Gorin and G. L. Sassaki, J. Chromatogr., A, 2009, 1216, 99–105 CrossRef CAS.
- S. Julka, H. Cortes, R. Harfmann, B. Bell, A. Schweizer-Theobaldt, M. Pursch, L. Mondello, S. Maynard and D. West, Anal. Chem., 2009, 81, 4271–4279 CrossRef CAS.
- D. Boschmann, R. Edam, P. J. Schoenmakers and P. Vana, Macromol. Symp., 2009, 275–276, 184–196 CrossRef CAS.
- R. Edam, D. M. Meunier, E. P. C. Mes, F. A. Van Damme and P. J. Schoenmakers, J. Chromatogr., A, 2008, 1201, 208–214 CrossRef CAS.
- R. E. Murphy, M. R. Schure and J. P. Foley, Anal. Chem., 1998, 70, 4353–4360 CrossRef CAS.
- H.-z. Tian, J. Xu and Y.-f. Guan, Fenxi Huaxue, 2008, 36, 860–864 CAS.
- H.-Z. Tian, J. Xu and Y. Guan, J. Sep. Sci., 2008, 31, 1677–1685 CrossRef CAS.
- H.-Z. Tian, J. Xu and Y.-F. Guan, Gaodeng Xuexiao Huaxue Xuebao, 2007, 28, 630–634 CAS.
- P. Hemstroem and K. Irgum, J. Sep. Sci., 2006, 29, 1784–1821 CrossRef.
- C. Apostolou, C. Kousoulos, Y. Dotsikas and L. Loukas Yannis, Biomed. Chromatogr., 2008, 22, 1393–1402 CrossRef CAS.
- S. Louw, A. S. Pereira, F. Lynen, M. Hanna-Brown and P. Sandra, J. Chromatogr., A, 2008, 1208, 90–94 CrossRef CAS.
- A. Liu, J. Tweed and C. E. Wujcik, J. Chromatogr., B: Anal. Technol. Biomed. Life Sci., 2009, 877, 1873–1881 CrossRef CAS.
- C. J. Platerink, H.-G. M. Janssen and J. Haverkamp, Anal. Bioanal. Chem., 2008, 391, 299–307 CrossRef CAS.
- T. Ikegami, T. Hara, H. Kimura, H. Kobayashi, K. Hosoya, K. Cabrera and N. Tanaka, J. Chromatogr., A, 2006, 1106, 112–117 CrossRef CAS.
- C. J. Venkatramani and A. Patel, J. Sep. Sci., 2006, 29, 510–518 CrossRef CAS.
- I. Francois, D. Cabooter, K. Sandra, F. Lynen, G. Desmet and P. Sandra, J. Sep. Sci., 2009, 32, 1137–1144 CrossRef CAS.
- J. Cong and B. Lin, J. Liq. Chromatogr. Relat. Technol., 2008, 31, 891–911 CrossRef CAS.
- P. Cesla, T. Hajek and P. Jandera, J. Chromatogr., A, 2009, 1216, 3443–3457 CrossRef CAS.
- A. J. Alexander and L. Ma, J. Chromatogr., A, 2009, 1216, 1338–1345 CrossRef CAS.
- K. R. Ing-Lorenzini, A. Desmeules Jules, M. Besson, J.-L. Veuthey, P. Dayer and Y. Daali, J. Chromatogr., A, 2009, 1216, 3851–3856 CrossRef CAS.
- M. Kivilompolo and T. Hyotylainen, J. Sep. Sci., 2008, 31, 3466–3472 CrossRef CAS.
- T. Hajek, V. Skerikova, P. Cesla, K. Vynuchalova and P. Jandera, J. Sep. Sci., 2008, 31, 3309–3328 CrossRef CAS.
- E. Rogatsky, V. Tomuta, H. Jayatillake, G. Cruikshank, L. Vele and D. T. Stein, J. Sep. Sci., 2007, 30, 226–233 CrossRef CAS.
- P. Jandera, K. Vynuchalova, T. Hajek, P. Cesla and G. Vohralik, J. Chemom., 2008, 22, 203–217 CrossRef CAS.
- J. Komaba, D. Matsuda, K. Shibakawa, S. Nakade, Y. Hashimoto, Y. Miyata and M. Ogawa, Biomed. Chromatogr., 2009, 23, 315–323 CrossRef CAS.
- J. Pol, B. Hohnova and T. Hyoetylaeinen, J. Chromatogr., A, 2007, 1150, 85–92 CrossRef CAS.
- A. Ghassempour, H. Rezadoost, M. Ahmadi and H. Y. Aboul-Enein, J. Liq. Chromatogr. Relat. Technol., 2009, 32, 1434–1447 CrossRef CAS.
- Y. Zhou, Y. Wang, R. Wang, F. Guo and C. Yan, J. Sep. Sci., 2008, 31, 2388–2394 CrossRef CAS.
- G. Guiochon, N. Marchetti, K. Mriziq and R. A. Shalliker, J. Chromatogr., A, 2008, 1189, 109–168 CrossRef CAS.
- J. Wei, J. Sun, W. Yu, A. Jones, P. Oeller, M. Keller, G. Woodnutt and M. Short Jay, J. Proteome Res., 2005, 4, 801–808 CrossRef CAS.
- W. H. McDonald, R. Ohi, D. T. Miyamoto, T. J. Mitchison and J. R. Yates, Int. J. Mass Spectrom., 2002, 219, 245–251 Search PubMed.
- B. Q. Tran, H. Loftheim, L. Reubsaet, E. Lundanes and T. Greibrokk, J. Sep. Sci., 2008, 31, 2913–2923 CrossRef CAS.
- J. A. Karty, W. E. Running and J. P. Reilly, J. Chromatogr., B: Anal. Technol. Biomed. Life Sci., 2007, 847, 103–113 CrossRef CAS.
- J.-L. Zhou, J.-J. An, P. Li, H.-J. Li, Y. Jiang and J.-F. Cheng, J. Chromatogr., A, 2009, 1216, 2394–2403 CrossRef CAS.
- Z.-B. Ning, Q.-R. Li, J. Dai, R.-X. Li, C.-H. Shieh and R. Zeng, J. Proteome Res., 2008, 7, 4525–4537 CrossRef CAS.
- K. Deguchi, T. Keira, K. Yamada, H. Ito, Y. Takegawa, H. Nakagawa and S.-I. Nishimura, J. Chromatogr., A, 2008, 1189, 169–174 CrossRef CAS.
- Y. Jmeian and Z. El Rassi, Electrophoresis, 2008, 29, 2801–2811 CAS.
- N. A. Cellar, A. S. Karnoup, D. R. Albers, M. L. Langhorst and S. A. Young, J. Chromatogr., B: Anal. Technol. Biomed. Life Sci., 2009, 877, 79–85 CrossRef CAS.
- L. Rieux, R. Bischoff, E. Verpoorte and H. A. G. Niederlaender, J. Chromatogr., A, 2007, 1149, 169–177 CrossRef CAS.
- A. Mihailova, B. Karaszewski, E. M. Faergestad, R. Hauser, W. M. Nyka, E. Lundanes and T. Greibrokk, J. Sep. Sci., 2008, 31, 468–479 CrossRef CAS.
- A. Mihailova, B. Karaszewski, R. Hauser, E. Lundanes and T. Greibrokk, J. Sep. Sci., 2007, 30, 249–256 CrossRef CAS.
- C. Liu and X. Zhang, J. Chromatogr., A, 2007, 1139, 191–198 CrossRef CAS.
- F. Wang, J. Dong, X. Jiang, M. Ye and H. Zou, Anal. Chem., 2007, 79, 6599–6606 CrossRef CAS.
- F. Wang, J. Dong, M. Ye, X. Jiang, R. a. Wu and H. Zou, J. Proteome Res., 2008, 7, 306–310 CrossRef CAS.
- T. Kajdan, H. Cortes, K. Kuppannan and S. A. Young, J. Chromatogr., A, 2008, 1189, 183–195 CrossRef CAS.
- Q. Luo, G. Yue, G. A. Valaskovic, Y. Gu, S.-L. Wu and B. L. Karger, Anal. Chem., 2007, 79, 6174–6181 CrossRef.
- A.-M. Hesse, P. Marcelo, J. Rossier and J. Vinh, J. Chromatogr., A, 2008, 1189, 175–182 CrossRef CAS.
- J. Dai, L.-S. Wang, Y.-B. Wu, Q.-H. Sheng, J.-R. Wu, C.-H. Shieh and R. Zeng, J. Proteome Res., 2009, 8, 133–141 CrossRef CAS.
- R. G. Harfmann, S. Julka and H. J. Cortes, J. Sep. Sci., 2008, 31, 915–920 CrossRef CAS.
- E. J. C. van der Klift, G. Vivo-Truyols, F. W. Claassen, F. L. van Holthoon and T. A. van Beek, J. Chromatogr., A, 2008, 1178, 43–55 CrossRef CAS.
- F. Cacciola, P. Jandera and L. Mondello, J. Sep. Sci., 2007, 30, 462–474 CrossRef CAS.
- Y. Wei, T. Lan, T. Tang, L. Zhang, F. Wang, T. Li, Y. Du and W. Zhang, J. Chromatogr., A, 2009, 1216, 7466–7471 CrossRef CAS.
- C. Champmartin, P. Simon, P. Delsaut, M. Dorotte and B. Bianchi, J. Chromatogr., A, 2007, 1142, 164–171 CrossRef CAS.
- M. Kivilompolo and T. Hyoetylaeinen, J. Chromatogr., A, 2007, 1145, 155–164 CrossRef CAS.
- M. Eggink, M. Wijtmans, R. Ekkebus, H. Lingeman, I. J. P. de Esch, J. Kool, W. M. A. Niessen and H. Irth, Anal. Chem., 2008, 80, 9042–9051 CrossRef CAS.
- X. Chen, Z. Jiang, Y. Zhu and J. Tan, Chromatographia, 2007, 65, 141–147 CrossRef CAS.
- J.-A. Raust, A. Bruell, C. Moire, C. Farcet and H. Pasch, J. Chromatogr., A, 2008, 1203, 207–216 CrossRef CAS.
- A. Medvedovici, F. Albu, C. Georgita, D. I. Sora, T. Galaon, S. Udrescu and V. David, J. Chromatogr., B: Anal. Technol. Biomed. Life Sci., 2007, 850, 327–335 CrossRef CAS.
|
This journal is © The Royal Society of Chemistry 2010 |
Click here to see how this site uses Cookies. View our privacy policy here.