DOI:
10.1039/B816209C
(Paper)
J. Mater. Chem., 2009,
19, 2310-2315
Received
16th September 2008
, Accepted 12th January 2009
First published on 27th February 2009
Abstract
We report on the therapeutic ability of a novel cyclodextrin-covered gold nanoparticle (AuNP) carrier for noncovalent encapsulation of an anti-cancer drug. The surface of the AuNPs was functionalized with cyclodextrin as a drug pocket, anti-epidermal growth factor receptor (anti-EGFR) antibody as a targeting moiety, and poly(ethyleneglycol) (PEG) as an anti-fouling shell. β-Lapachone, an anti-cancer drug, was efficiently encapsulated into the hydrophobic cavity of cyclodextrin on the surface of the AuNP carriers (AuNP-1). The glutathione-mediated release of β-lapachone from the surface of AuNP-1 was demonstrated by an experiment with MCF-7 (low glutathione concentration) and A549 cells (high glutathione concentration). We also show that the introduction of an anti-EGFR antibody onto the AuNP carriers (AuNP-2) increased the intracellular uptake of AuNP carriers as compared with AuNP-1, which does not contain a targeting ligand. In the in vitro cytotoxicity study, AuNP-2 with β-lapachone exhibited a higher apoptosis effect than that caused by AuNP-1 with β-lapachone. This work suggests that AuNPs covered with cyclodextrin and tumor-targeting ligands may find useful applications for the development of nanoparticles with therapeutic and diagnostic modalities.
1. Introduction
There has been considerable interest in nanomaterials for medical applications, and various systems have been developed for delivery vectors and diagnostic markers.1–4 Recently, the combination of organic building blocks with anti-cancer drugs and inorganic nanocrystals has been considered as a novel approach in cancer therapy and diagnosis.4a–c Following the first report of the unique ability of various inorganic nanomaterials for diagnosis, the potential capability of gold nanoparticles (AuNPs) for computed tomography imaging was recently revealed.4d–f Furthermore, gold nanoparticles (AuNPs) have recently been a focus of increased interest for their therapeutic potential as drug-delivery carriers due to their attractive characteristics such as size, robust stability, and biocompatibility.5 Recent work from several laboratories have shown that AuNPs functionalized with drug molecules have a substantially expanded performance as efficient therapeutic vehicles.6–10 In these systems, drug molecules can be released from the surface of AuNP by desorption through ligand-exchange processes or the protonation of thiol groups at low pH.6–10 However, since most methods for the integration of drug molecules with inorganic nanoparticles reported to date require chemical modification of drugs to anchor them to the surface, inorganic nanoparticles have a limitation as therapeutic vehicles. Therefore, the use of inorganic nanoparticles such as AuNPs as therapeutic vehicles requires the development of a reliable approach that enables noncovalent encapsulation of drug molecules without sacrificing the unique advantages of inorganic nanoparticles.
Here we report the therapeutic ability of a novel AuNP carrier system composed of a poly(ethylene glycol) (PEG) shell, per-6-thio-β-cyclodextrin (SH-CD) as a drug pocket, and an anti-epidermal growth factor receptor antibody (anti-EGFR antibody) as a targeting ligand. PEG derivatives are biocompatible polymers and can protect nanocarrier and payload from enzymatic degradation.11 Cyclodextrins (CDs) have been used as drug-delivery agents because of their ability to protect drugs from physical, chemical, and enzymatic degradation and also to solubilize hydrophobic drugs.12 Therefore, SH-CDs on the surface of AuNPs can provide sites to accommodate hydrophobic molecules. β-Lapachone, which can be encapsulated into the cavity of β-CD (association constant: Kc = (1.23 ± 0.01) × 103 M−1),13 was adopted as a chemotherapeutic drug, because it exhibits superior activity against various cancer cells in breast, lung, and prostate tissue.14
2. Results and discussion
2.1 Synthesis and characterization of AuNP carriers
For the preparation of AuNP carriers functionalised with CD and PEG (AuNP-1), an aqueous solution of AuNPs (average diameter ∼27 nm) stabilized with citrate (AuNP-0) was stirred for 12 h with SH-CD and α-methoxy-ω-mercapto-poly(ethylene glycol) (mPEG-SH, MW 2000 Da) (Scheme 1).15,16†AuNP-1.5, which contains succinimidyl-functionalized PEG and CD, was prepared by the reaction of AuNP-0 with SH-CD, mPEG-SH, and α-succinimidyl propionate-ω-lipoic acid-poly(ethylene glycol) (NHS-PEG-SH).† Both AuNP-1 and AuNP-1.5 were purified by ultracentrifugation and washing with water.
The FT-IR spectra of AuNP-1 and AuNP-1.5 showed absorption bands at 3430 cm−1 (OH stretching H-bonded), 2920–2855 cm−1 (CH2 stretching), 1632 cm−1 (OH bending), and 1041 cm−1 (COC stretching), indicating that the surface of AuNP-0 was functionalized with SH-CDs and PEG ligands17 (Fig. S1†). The thermogravimetric analysis (TGA) showed that the organic content in AuNP-1 and AuNP-1.5 was 4.47 wt% and 4.49 wt%, respectively (Fig. S2). Based on the results from the TGA and 1H NMR studies, it was estimated that one AuNP-1 carrier was covered with ∼800 SH-CDs and ∼2200 mPEG-SHs.
In the case of AuNP-1.5, the approximate number of SH-CDs, mPEG-SH, and NHS-PEG-SH per particle was 800, 1800, and 180, respectively.†
For the introduction of a ligand able to target cancer cells that overexpress EGFR (such as colon, lung, prostate, and breast cancer cells), AuNP-1.5 were incubated with the anti-EGFR solution (HEPES pH 7.4) for 12 h, and then the mixture (containing AuNP-2) was purified by ultracentrifugation and washing with water. The conjugation of anti-EGFR with the AuNP-1.5 was detected using the Dot blot method (Fig. S3†). The FT-IR spectrum of AuNP-2 also confirmed the presence of anti-EGFR, which exhibited absorption bands in the amide I (1651 cm−1) and amide II (1552 cm−1) regions (Fig. S1). The amount of anti-EGFR conjugated to the AuNP carriers, quantified using a Micro BCA assay, was 15 µg mL−1 of AuNP-2 (∼2.72 anti-EGFRs per one AuNP-2). The aqueous solution of AuNP-0 exhibited a surface plasmon resonance at 527 nm (ε527nm = 2.4 × 109 M−1 cm−1). The absorption maxima of AuNP-1 and AuNP-1.5 were shifted to 541 nm. The absorption maximum of the AuNP-2 solution was further red-shifted to 544 nm (Fig. 1a). AuNP-1.5 and AuNP-2 exhibited excellent colloidal stability in water and various pH conditions (Fig. S4). Au carriers loaded with β-lapachone (AuNP-1/lap and AuNP-2/lap) respectively were prepared as shown in Scheme 1. To obtain Au nanocarriers with β-lapachone, an excess amount of β-lapachone to SH-CDs on Au nanocarriers was added to Au nanocarrier solutions and sonicated for 3 min. AuNP-1/lap and AuNP-2/lap were purified with filtration and ultracentrifugation respectively. The relative absorbances of AuNP-1/lap and AuNP-2/lap over 24 h were constant, which indicated that no precipitation or flocculation of the nanocarriers occurred in aqueous solution (Fig. 1b). The average hydrodynamic diameters of AuNP-0, AuNP-1, AuNP-2, AuNP-1/lap, and AuNP-2/lap measured by dynamic light scattering (DLS) were 28.9 nm, 40.4 nm, 46.7 nm, 42.6 nm, and 47.0 nm, respectively. The increase of hydrodynamic diameters in AuNP-1 and AuNP-2 revealed the formation of a monolayer of PEG ligands and SH-CDs on the surface of AuNP-0. The CONTIN analysis of the autocorrelation functions for AuNP-0, AuNP-1/lap and AuNP-2/lap showed a monomodal distribution, which indicates that no aggregation occurred between Au nanocarriers after functionalization or encapsulation of β-lapachone (Fig. 1c). The slope of the angular dependence of the apparent diffusion coefficient (Dapp) was zero, and the size of the aggregates was constant for the investigated range of angle, which confirmed the spherical shape of the carriers (Fig. 1d and S5).18 The transmission electron microscopy (TEM) analysis revealed that the average diameters of the Au core in AuNP-0, AuNP-1, AuNP-1.5, AuNP-2, AuNP-1/lap, and AuNP-2/lap were 27.2 ± 3 nm, 27.1 ± 3 nm, 27.1 ± 3 nm, 27.4 ± 2 nm, 27.0 ± 3 nm, and 27.6 ± 3 nm, respectively (Fig. 2 and S6). These results confirmed that there is no significant transformation or deformation of AuNPs after the surface functionalization.
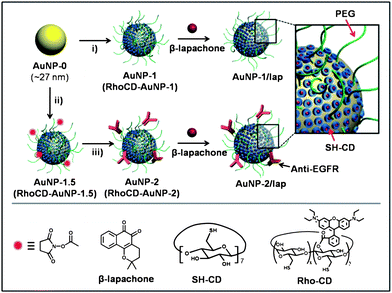 |
| Scheme 1 Schematic illustration of the functionalization of AuNP carriers with β-lapachone, using: i) SH-CD and mPEG-SH for AuNP-1 (RhoCD and mPEG-SH for RhoCD-AuNP-1); ii) SH-CD, mPEG-SH, and NHS-PEG-SH for AuNP-1.5 (RhoCD, mPEG-SH, NHS-PEG-SH for RhoCD-AuNP-1.5) and iii) anti-EGFR. | |
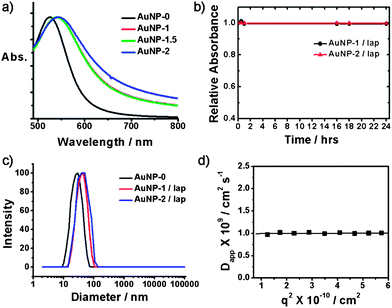 |
| Fig. 1 (a) Absorption spectra of AuNP carriers. (b) Time dependence of relative absorbance at 541 nm for AuNP-1/lap and AuNP-2/lap. (c) DLS size distribution of AuNPs at a scattering angle of 90°. (d) Angular dependence of apparent diffusion coefficient (Dapp) of AuNP-2/lap (T = 25 °C). | |
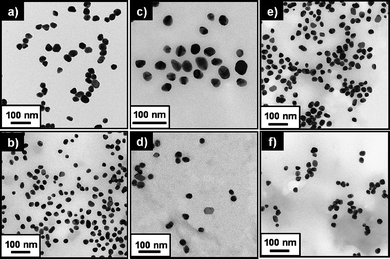 |
| Fig. 2
TEM images of (a) AuNP-0, (b) AuNP-1, (c) AuNP-1.5, (d) AuNP-2, (e) AuNP-1/lap, and (f) AuNP-2/lap. | |
The potential usefulness of AuNPs as a therapeutic carrier of anti-cancer drugs was investigated as follows. First, the release characteristics of functional molecules from the AuNP surface in the intracellular environment were investigated. The release of SH-CD from Au surfaces can be induced by ligand exchange with glutathione in the intracellular matrix of cancer cells.7,8 To trace the release of SH-CD from AuNP, rhodamine B was conjugated with SH-CD (RhoCD)† and then introduced on the surface of AuNP-1 to prepare RhoCD-AuNP-1. The fluorescence of rhodamine B is quenched on the surface of Au nanoparticles (Fig. S7). However, the fluorescence of rhodamine B can be detected when RhoCD is removed from the Au surface. Therefore, we can monitor the release behavior of SH-CD from the Au surface during the intracellular migration of AuNP carriers.†
2.2
Glutathione-mediated release charateristics
The fluorescence intensity of the RhoCD moiety increased when RhoCD-AuNP-1 was incubated in HEPES buffer at pH 7.4 containing 10 mM glutathione, compared with RhoCD-AuNP-2 maintained in the buffer without glutathione. This result indicates that the release of RhoCD from the surface of RhoCD-AuNP-2 was promoted by the presence of glutathione (Fig. S7†). The importance of the role of glutathione in the intracellular release of RhoCD from RhoCD-AuNP1 was further demonstrated in the following experiment. We determined the intracellular release of RhoCD from RhoCD-AuNP-1 in the MCF-7 cells containing a low concentration of glutathione and A549 cells containing a high concentration of glutathione (Fig. S8). Based on the changes in the fluorescence intensity of the RhoCD moiety from the confocal laser scanning microscopy (CLSM) image, it could be concluded that RhoCD release from RhoCD-AuNP-1 was faster in the A549 cells compared to the MCF-7 cells (Fig. 3). We then investigated how the presence of anti-EGFR antibody on AuNP surface influences the intracellularRhoCD content using RhoCD-AuNP-2. Note that the EGFR expression on the surface of A549 cells has been reported to be high.19 The results shown in Fig. 3 clearly indicate that the concentration of RhoCD released from RhoCD-AuNP-2 was greater, and increased more rapidly, in A549 cells than in MCF-7 cells. Taken together, it is concluded that, in A549 cells, the relatively high level of EGFR expression on the cell surface augmented the internalization of RhoCD-AuNP-2, and the high intracellular concentration of glutathione increased the RhoCD release from the internalized RhoCD-AuNP-2.
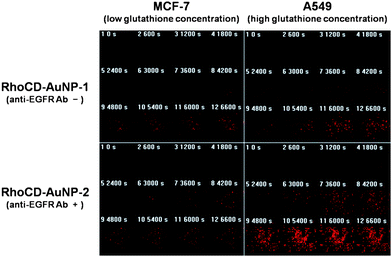 |
| Fig. 3 The effect of anti-EGFR antibody and glutathione on the cellular uptake and intracellular release in different cell lines. Time courses of RhoCD fluorescence intensity from CLSM images in MCF-7 cell (low glutathione) and A549 cell (high glutathione) incubated with RhoCD-AuNP-1 or RhoCD-AuNP-2. | |
2.3
Intracellular uptake of AuNP
Fig. 4a shows the CLSM images of A549 cells incubated with RhoCD-AuNP-1 or RhoCD-AuNP-2 for 1 h. The stronger RhoCD fluorescence in the cells incubated with RhoCD-AuNP-2, as compared with the cells incubated with RhoCD-AuNP-1, demonstrated that anti-EGFR antibody effectively augmented the internalization of AuNPs. The transmission electron microscopy study (Fig. 4b) also demonstrated that the intracellular uptake of AuNPs was greater in the A549 cells incubated with AuNP-2 compared with the cells incubated with AuNP-1. It appeared that the increase in the internalization of AuNP-2 mediated by anti-EGFR antibody increased the intracellular uptake of AuNPs.
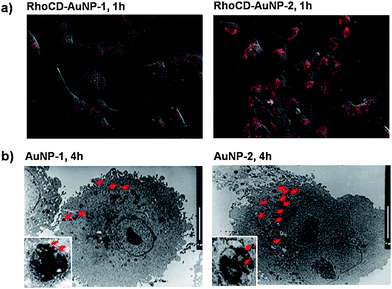 |
| Fig. 4 (a) Enhanced intracellular release: CLSM images of A549 cells incubated with Rho-AuNP-1 or Rho-AuNP-2 for 1 h. (b) Enhanced intracellular uptake of AuNP carriers: TEM images of A549 cells incubated with AuNP-1 or AuNP-2 for 4 h (scale bars: 10 µm). | |
2.4 The release of β-lapachone from AuNP carriers
The release of β-lapachone from AuNP-1/lap in the HEPES buffer solution containing either 10 mM or no glutathione is shown in Fig. 5a. The percent release of β-lapachone from AuNP-1/lap was significantly greater in the presence of glutathione. The mass analysis showed that both SH-CD and β-lapachone were present in the buffer solution containing 10 mM glutathione, but not in the buffer solution without glutathione (Fig. S9). It was evident that glutathione induced the release of SH-CD from RhoCD-AuNP-1, followed by release of β-lapachone from the cavity of CD. These results indicated that the intracellular glutathione concentration significantly influences the release of β-lapachone from AuNP/lap in the cells.
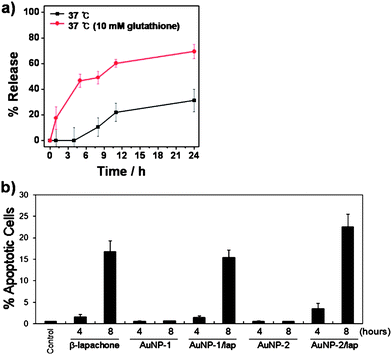 |
| Fig. 5 (a) The effect of glutathione concentration on the release of β-lapachone from AuNP-1/lap in HEPES buffer solution (pH 7.4). (b) The percent apoptosis of A549 cells as determined by flow cytometry after incubation with free β-lapachone, AuNP-1, AuNP-1/lap, AuNP-2, or AuNP-2/lap. Means of 5 experiments with duplicated cultures ± S.E. are shown. | |
2.5
In vitro cytotoxicity study of AuNP carriers with β-lapachone
The effectiveness of 15 µM β-lapachone, AuNP-1/lap, and AuNP-2/lap at inducing apoptosis in A549 cells was investigated using flow cytometry (Fig. 5b). The percent of apoptosis caused by AuNP-2/lap was slightly greater than that caused by free β-lapachone after 4 h or 8 h treatment. Importantly, the apoptosis caused by AuNP-2/lap was slightly greater than that caused by AuNP-1/lap, indicating that the presence of anti-EGFR antibody on the surface of AuNP increased the cellular uptake of AuNP/lap (Fig. 4).
In the above in vitro cytotoxicity study, cells were exposed to 15 µM β-lapachone in the form of free β-lapachone, AuNP-1/lap, and AuNP-2/lap for identical lengths of time. It has recently been reported that AuNPs are cleared from blood circulation with a half-life of 2–6 h after intravenous injection.20 Therefore, it may be expected that the prolonged circulation of AuNP/lap would result in the exposure of tumor cells to a higher concentration of β-lapachone for a longer period, as compared with the situation when free β-lapachone is administered. Furthermore, the presence of anti-EGFR would cause preferential accumulation of AuNPs in tumor cells relative to normal cells.
3. Conclusions
In summary, we have developed a new type of Au nanocarrier which is covered with SH-CDs, PEG, and a targeting antibody, and evaluated its capability as a carrier vehicle for a hydrophobic anti-cancer drug. It was found that AuNPs are a potentially useful carrier of the anti-cancer agent β-lapachone, which can be loaded in the cavity of CD on the surface of AuNPs. Since a variety of hydrophobic drugs can be easily encapsulated into the hydrophobic pocket of CDs, the multifunctional AuNP carriers described here can serve as a versatile nanoplatform for the delivery of other anti-cancer drugs as well. The use of AuNPs as a drug carrier has the potential to prolong the blood circulation time of the drug, thereby increasing the accumulation of the drug in target tumors. Our results also indicated, as illustrated in Fig. 6, that attaching anti-EGFR to AuNPs increased the cellular uptake of drug-carrying AuNPs. The release of a cytotoxic drug from the surface of AuNPs is greatly influenced by the glutathione concentration in the cells. It was concluded that AuNPs covered with SH-CD and a tumor-targeting ligand are potentially useful carriers of hydrophobic anti-cancer drugs. Furthermore, this approach may open up novel possibilities in the development of the next generation of diagnostic and therapeutic modalities based on inorganic nanoparticles.
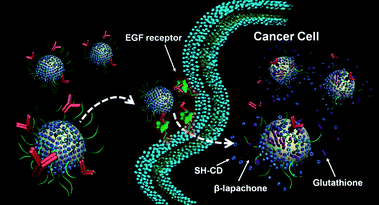 |
| Fig. 6 Schematic illustration for the enhanced cellular uptake of AuNPs by anti-EGFR antibody and glutathione-mediated intracellular release of AuNP-2 carriers. | |
4. Experimental
4.1 Materials and equipment
HAuCl4, trisodium citrate, β-cyclodextrin, iodine, NaOH, N-hydroxysuccinimide, 1,3-diisopropylcarbodiimide, 4-(dimethylamino)pyridine, lipoic acid, mouse monoclonal anti-epidermal growth factor receptor (anti-EGFR), and KHSO4 from Aldrich were used as received. Methoxy-poly(ethylene glycol sulfhydryl) (mPEG-SH, MW 2000 Da) from SunBio and α-carboxy ω-hydroxy terminated poly(ethylene glycol) from Polymer Source were used as received. All solvents were purified by a literature procedure.211H and 13C NMR spectra were recorded on a Varian UNITY INOVA 400 at 400 and 100 MHz respectively. FT-IR spectra were obtained using VERTEX 80V vacuum FT-IR spectrometer. UV–vis absorption spectra were obtained using a Hewlett-Packard 8453A spectrophotometer. All the fluorescence measurements were performed using a Shimadzu RF-5301PC spectrofluorometer.
4.2
Cell lines and culture conditions
A549 human lung cancer cells and MCF human breast cancer cells were used. The cells were cultured in 25 cm2 plastic tissue culture flasks with Dulbecco's modified Eagle's medium (DMEM, Hyclone Laboratories Inc., Logan, Utah) supplemented with 10% fetal bovine serum (FBS, Hyclone Laboratories Inc.) and 1% penicillin/streptomycin (Hyclone Laboratories Inc.) in a humidified 5% CO2/95% air incubator at 37 °C. The cells in exponential growth phase were treated with β-lapachone, AuNP-1, Au-NP-1/lap, AuNP-2 or Au-NP-2/lap.
Control and drug-treated A549 cells were harvested using trypsin. The cells were then fixed with 70% ethanol and maintained at −20 °C overnight, washed with PBS, and resuspended in 1 mL PBS containing 30 units of DNase free RNase. After incubation with propidium iodide (PI; Sigma-Aldrich, Inc., 5 µg in 100 µL) in the dark at 37 °C for 30 min, the cells were subjected to apoptosis study with a FACSCalibur™ flow cytometer (Becton Dickinson Bioscience, San Jose, CA). A total of 10
000 cells were counted for each sample.
A549 cells were grown in eight-well chamber slides (or 24-well coverslips) and treated with 15 µM free β-lapachone, AuNP-1, Au-NP-1/lap, AuNP-2 or Au-NP-2/lap at 37 °C for 0–24 h. Cells were washed with PBS, fixed using 4% (v/v) paraformaldehyde (Sigma–Aldrich) at 4 °C for 30 min, and permeabilized with 0.25% (w/v) Triton X-100 (Sigma–Aldrich) at room temperature for 2 min. After several washing with PBS, the nuclei were stained with DAPI solution containing ProLong Gold antifade reagent (Invitrogen, Eugene, OR). The glass slides were covered with cover glasses, and the cells were examined with a fluorescence microscope.
4.5 Live cell imaging
For live cell time-lapse imaging, cells were plated on Lab-Tek II coverglass bottom dishes and analyses were performed with a Nikon TE200E inverted device 8 h after the addition of AuNPs into the cultures. Single-plane confocal picture sequences were taken every 15 min for confocal stacks and used for simultaneous two-color-wide field imaging.
4.6
Intracellular
glutathione concentration
The GSH concentration was measured using a glutathione assay kit from Sigma (St. Louis, MO) following the manufacturer's instructions. Briefly, cells were seeded in a six-well plate and either left untreated or treated with drugs before assaying for GSH. Cell lysate was treated with 5% 5-sulfosalicylic acid and centrifuged to remove protein precipitate. The supernatant was then treated with 5,5-dithiobis(2-nitrobenzoic acid) (DTNB). GSH reduced DTNB to generate TNB, and the total TNB formed was measured by absorption at 412 nm in a Beckman DU640 spectrophotometer (Beckman Coulter, Fullerton, CA).
Acknowledgements
This work was supported by grants from the National R&D Program for Cancer Control, Ministry of Health & Welfare, Republic of Korea (0620340-1), the National Nuclear Technology Program (2007) from KOSEF, and the Korea Health 21 R&D Project (A062254).
References
-
(a) N. L. Rosi and C. A. Mirkin, Chem. Rev., 2005, 105, 1547 CrossRef CAS;
(b) M. Ferrari, Nat. Rev. Cancer, 2005, 5, 161 CrossRef CAS;
(c) R. Weissleder, Science, 2006, 312, 1168 CrossRef CAS.
-
(a) R. Gref, Y. Minamitake, M. T. Peracchia, V. Trubetskoy, V. Torchilin and R. Langer, Science, 1994, 263, 1600 CrossRef CAS;
(b) R. Langer and D. A. Tirrell, Nature, 2004, 428, 487 CrossRef CAS;
(c) V. P. Torchilin, Nat. Rev. Drug Discovery, 2005, 4, 145 CrossRef CAS;
(d) R. Duncan, Nat. Rev. Drug Discovery, 2003, 2, 347 CrossRef CAS;
(e) R. Duncan, Nat. Rev. Cancer, 2006, 6, 688 CrossRef CAS.
-
(a) C. Barbe, J. Bartlett, L. Kong, K. Finnie, H. Q. Lin, M. Larkin, S. Calleja, A. Bush and G. Calleja, Adv. Mater., 2004, 16, 1959 CrossRef CAS;
(b) R. Langer, Acc. Chem. Res., 1993, 26, 537 CrossRef CAS;
(c) X. Guo and F. C. Szoka, Jr., Acc. Chem. Res., 2003, 36, 335 CrossRef CAS;
(d) K. Kataoka, A. Harada and Y. Nagasaki, Adv. Drug Delivery Rev., 2001, 47, 113 CrossRef CAS;
(e) M. Jones and J. Leroux, Eur. J. Pharm. Biopharm., 1999, 48, 101 CrossRef CAS.
-
(a) J. Yang, C.-H. Lee, H.-J. Ko, J.-S. Suh, H.-G. Yoon, K. Lee, Y.-M. Huh and S. Haam, Angew. Chem., Int. Ed., 2007, 46, 8836 CrossRef CAS;
(b) N. Nasongkla, E. Bey, J. Ren, H. Ai, C. Khemtong, J. S. Guthi, S.-F. Chin, A. D. Sherry, D. A. Boothman and J. Gao, Nano Lett., 2006, 6, 2427 CrossRef CAS;
(c) J. Kim, J. E. Lee, S. H. Lee, J. H. Yu, J. H. Lee, T. G. Park and T. Hyeon, Adv. Mater., 2008, 20, 478 CrossRef CAS;
(d) J. F. Hainfeld, D. N. Slatkin, T. M. Focella and H. M. Smilowitz, Br. J. Radiol., 2006, 79, 248 Search PubMed;
(e) V. Kattumuri, K. Katti, S. Bhaskaran, E. J. Boote, S. W. Casteel, G. M. Fent, D. J. Robertson, M. Chandrasekhar, R. Kannan and K. V. Katti, Small, 2007, 3, 333 CrossRef CAS;
(f) D. Kim, S. Park, J. H. Lee, Y. Y. Jeong and S. Jon, J. Am. Chem. Soc., 2007, 129, 7661 CrossRef CAS;
(g) J. H. Lee, Y. M. Huh, Y. W. Jun, J. W. Seo, J. T. Jang, H. T. Song, S. Kim, E. J. Cho, H. G. Yoon, J. S. Suh and J. Cheon, Nat. Med., 2006, 13, 95;
(h) X. Gao, Y. Cui, R. M. Levenson, L. W. Chung and S. Nie, Nat. Biotechnol., 2004, 22, 969 CrossRef CAS;
(i) S. Kim, Y. T. Lim, E. G. Soltesz, A. M. Grand, J. Lee, A. Nakayama, J. A. Parker, T. Mihaljevic, R. G. Laurence, D. M. Dor, L. H. Cohn, M. G. Bawendi and J. V. Frangioni, Nat. Biotechnol., 2004, 22, 93 CrossRef;
(j) O. Rabin, J. M. Perez, J. Grimm, G. Wojtkiewicz and R. Weissleder, Nat. Mater., 2006, 5, 118 CrossRef CAS.
-
(a) G. Han, P. Ghosh and V. M. Rotello, Nanomedicine, 2007, 2, 113 CrossRef CAS;
(b) G. Han, P. Ghosh, M. De and V. M. Rotello, Nanobiotechnology, 2007, 3, 40 CrossRef CAS.
-
(a) G. F. Paciotti, D. G. I. Kingston and L. Tamarkin, Drug. Dev. Res., 2006, 67, 47 CrossRef CAS;
(b) G. F. Paciotti, L. Myer, D. Weinreich, D. Goia, N. Pavel, R. E. McLaughlin and L. Tamarkin, Drug Delivery, 2004, 11, 169 CrossRef CAS.
- R. Hong, G. Han, J. M. Fernández, B.-J. Kim, N. S. Forbes and V. M. Rotello, J. Am. Chem. Soc., 2006, 128, 1078 CrossRef CAS.
- A. Verma, J. M. Simard, W. E. Worrall and V. M. Rotello, J. Am. Chem. Soc., 2004, 126, 13987 CrossRef CAS.
- J. D. Gibson, B. P. Khanal and E. R. Zubarev, J. Am. Chem. Soc., 2007, 129, 11653 CrossRef CAS.
- P. Podsiadlo, V. A. Sinani, J. H. Bahng, N. W. S. Kam, J. Lee and N. A. Kotov, Langmuir, 2008, 24, 568 CrossRef CAS.
- F. M. Veronese, Biomaterials, 2001, 22, 405 CrossRef CAS.
- M. E. Davis and M. E. Brewster, Nat. Rev. Drug Discovery, 2004, 3, 1023 CrossRef CAS.
- N. Nasongkla, A. F. Wiedmann, A. Bruening, M. Beman, D. Ray, W. G. Bornmann, D. A. Boothman and J. Gao, Pharm. Res., 2003, 20, 1626 CrossRef CAS.
- E. K. Choi, K. Terai, I. M. Ji, Y. H. Kook, K. H. Park, E. T. Oh, R. J. Griffin, B. U. Lim, J. S. Kim, D. S. Lee, D. A. Boothman, M. Loren, C. W. Song and H. J. Park, Neoplasia, 2007, 9, 634 CrossRef CAS.
- M. T. Rojas, R. Koeniger, J. F. Stoddart and A. E. Kaifer, J. Am. Chem. Soc., 1995, 117, 336 CrossRef CAS.
- G. Frens, Nat. Phys. Sci., 1973, 241, 20 Search PubMed.
- J. Liu, S. Mendoza, E. Román, M. J. Lynn, R. Xu and A. E. Kaifer, J. Am. Chem. Soc., 1999, 121, 4304 CrossRef CAS.
- R. Xu, M. A. Winnik, F. R. Hallett, G. Riess and M. D. Croucher, Macromolecules, 1991, 24, 87 CrossRef CAS.
- M. Ono and M. Kuwano, Clin. Cancer Res., 2006, 12, 7242 CrossRef CAS.
- R. K. Visaria, R. J. Griffin, B. W. Williams, E. S. Ebbini, G. F. Paciotti, C. W. Song and J. C. Bischof, Mol. Cancer Ther., 2006, 5, 1014 CrossRef CAS.
-
W. L. F. Armarego and D. D. Perrin, Purification of Laboratory Chemicals, Butterworth–Heinemann, Oxford, 4th edn, 1996 Search PubMed.
Footnotes |
† Electronic supplementary information (ESI) available: Synthesis of AuNPs, FT-IR spectra, TGA, dot blot analysis, DLS, fluorescence analysis and ESI-MS results. See DOI: 10.1039/b816209c |
‡ These authors contributed equally to this work. |
|
This journal is © The Royal Society of Chemistry 2009 |