Total synthesis of (−)-microcarpalide, a novel microfilament disrupting metabolite†
Received 25th July 2003, Accepted 15th October 2003
First published on 6th November 2003
Abstract
The stereoselective total synthesis of (−)-microcarpalide, a recently discovered 10-membered lactone of fungal origin displaying a remarkable disrupting action on actin microfilaments, was accomplished by using ring-closing metathesis (RCM) as the key step for the formation of the medium-sized ring. The diene ester required for the macrocyclization reaction was assembled via DCC-mediated esterification of two suitable partners, each bearing a terminal alkene group. The alcohol fragment was synthesized from n-bromohexane through a seven-step sequence entailing two consecutive stereoselective homologations of chiral boronic esters as strategic transformations for the sequential insertion of the two stereocentres with the final S absolute configuration, using (+)-pinanediol as the chiral director; final elaboration to the desired C11 framework envisaged treatment with an allyl Grignard reagent and oxidative cleavage of the boronic scaffold. In contrast, the acidic fragment was prepared in ten steps from D-tartaric acid, whose C4 backbone was elongated to the required C7 skeleton by means of two distinct Swern–Wittig oxidation–homologation sequences.
Introduction
Secondary metabolites from endophytic fungi have been receiving a great deal of attention in recent years, and a number of peculiar structures with specific bioactivities have been discovered so far.1,2Along this line, microcarpalide (1) has been recently characterised as a new secondary metabolite produced by an endophytic fungus (as yet unidentified) isolated from the bark of the tropical tree Ficus microcarpa L.3 Bioassay-guided purification of fermentation broths using immunofluorescence microscopy to test anticytoskeletal activity led to the isolation of a new substance displaying a remarkable disrupting action on actin microfilaments, to which the structure 1 was assigned (Fig. 1).3
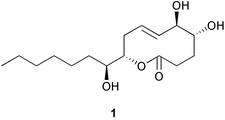 |
| Fig. 1 | |
Microcarpalide represents a novel alkyl-substituted nonenolide structurally related to a family of phytotoxins such as achaetolide,4 pinolidoxin,5 lethalotoxin,6 putaminoxins7 and herbarumins,8 from which it differs in the hydroxylation pattern and the double bond position within the 10-membered lactone, as well as in the longer side chain at C-10. At concentrations of 0.5–1 µg mL−1, microcarpalide was found to disrupt actin microfilaments in approximately 50% of A-10 cells (from rat smooth muscle);3 moreover, it displayed a weak cytotoxicity to mammalian cells,3 thus making it an attractive tool for studying cell motility and metastasis, and a potential lead structure to develop new anticancer drugs.
Owing to such a peculiar biological activity and attracted by the structural potential of microcarpalide for structure–activity relationship studies, we decided to challenge its total synthesis. In this respect, aiming also at shedding light on the mechanism of action of this novel fungal metabolite, the synthetic route ought to be versatile enough to allow the preparation of analogues thereof. With this in mind, the retrosynthetic approach outlined in Scheme 1 was devised.
 |
| Scheme 1 Retrosynthetic analysis. | |
Firstly, the macrocyclic framework of microcarpalide was retrosynthetically disassembled into fragments A and B
(Scheme 1) by means of two key disconnections, namely a ring-closing metathesis (RCM)9 for the construction of the oxecin ring, and an esterification reaction for assembling the two alkene fragments. Both fragments contain two stereogenic carbon atoms and bear a terminal alkene group that is required for the RCM macrocyclization.
Fragment A represents a dihydroxy substituted 1-undecene with S absolute configuration at both adjacent stereocentres (C-10 and C-1′ in 1) and can be ultimately disconnected to the C6 alkyl boronic acid (Scheme 1). In fact, boronic ester chemistry provides a highly stereoselective method for the sequential insertion of stereocentres into the C–B bond.10 In particular, chain elongation by means of two strategic stereoselective homologations of the corresponding (+)-pinanediol ester would allow installation of the C-1′ and C-10 atoms with the desired stereochemistry under the guide of the same chiral director, thus affording chloro derivative C. Final insertion of the allylic appendage via SN2 displacement and oxidative removal of the boronic ester would then lead to the required C11 skeleton of A, providing at the same time the free hydroxy group at C-10 to be used for the coupling reaction with partner B.
Fragment B is a 4,5-disubstituted 6-heptenoic acid bearing two contiguous hydroxy groups in a threo fashion, whose R absolute configurations can be matched by the pattern displayed by D-tartaric acid. Retrosynthetically, the C7 backbone was traced back to a suitably protected D-threitol D
(Scheme 1) by use of two different Swern–Wittig oxidation–homologation sequences (each one with an appropriate phosphorus ylide), which would allow chain elongation at both termini of the C4 chiral synthon D. In turn, threitol derivative D can be easily prepared from D-tartaric acid, as reported in the literature for the L-enantiomer.
Results and discussion
Synthesis of fragment A
For our synthesis we envisaged using the stereoselective homologation of chiral boronic esters developed by Matteson and coworkers10 to install the two adjacent stereocentres with the required S absolute configuration. In particular, (+)-pinanediol was chosen as the chiral auxiliary to direct the stereochemical outcome of the homologation reaction; in fact, the (+) isomer is known to induce the S configuration when used as the chiral director.10,11The synthetic approach to fragment A commenced from commercially available n-bromohexane (2), which was converted into the corresponding Grignard derivative; reaction between n-hexylmagnesium bromide and trimethyl borate in diethyl ether at −78 °C, followed by acidic work-up, furnished boronic acid 3 on a multigram scale in 72% yield (Scheme 2);12 acid 3 undergoes spontaneous dehydration to form the corresponding trimeric boroxine, as shown by MS analysis. Esterification with the chiral auxiliary (+)-pinanediol in dry Et2O at rt readily afforded the corresponding boronate 4
(71% yield).10 The presence of a carbon–boron bond was confirmed on the basis of the 13C NMR spectrum displaying a diagnostic broad signal at 10.5 ppm for C-1, which could be detected only in highly concentrated samples. Chiral boronate 4 is an air-stable liquid that can be easily handled and stored over time on the lab shelf.10 Hence, the stage was set to exploit Matteson's asymmetric homologation for inserting the first stereocentre. Addition of in situ-generated (dichloromethyl)lithium to chiral boronic ester 4 at −100 °C in THF13 provided α-chloro derivative 5 in good yield (64%) and high diastereoisomeric excess (d.e.
≥ 98%)
(Scheme 2). The presence of a new signal at 3.48 ppm in the 1H NMR spectrum indicated the successful insertion of a chlorine-bearing carbon atom into the C–B bond of 4, whereas the diastereoselectivity of the homologation was determined by means of the diagnostic signal of the Hendo proton of the pinanyl moiety (1.19 ppm, doublet).11 Since (+)-pinanediol is reported to direct the formation of (S)-α-chloroboronic esters,10,11 the S absolute configuration could be assigned to 1-chloroheptaneboronate 5. Subsequently, exposure to a solution of (benzyloxy)lithium (prepared by titration of benzyl alcohol with n-butyllithium in the presence of 1,10-phenanthroline as indicator) in THF resulted in the SN2 nucleophilic displacement of the chlorine atom,11 thus affording the corresponding 1-benzyloxy derivative 6 in 70% yield (Scheme 2). Compound 6 bears a protected hydroxy group with R absolute configuration,14 which corresponds to the desired 1′S stereochemistry in 1. More interestingly, benzyl ether 6 could be also obtained directly from 4 through a one-pot procedure that avoided the isolation of the α-chloroboronic ester 5 and, gratifyingly, proceeded with higher overall yield (55%).
 |
| Scheme 2 Synthesis of fragment A. Reagents and conditions: a. Mg, Et2O, reflux, then trimethyl borate, −78 °C → rt, 72%; b.
(1S,2S,3R,5S)-(+)-pinanediol, Et2O, rt, 71%; c. (dichloromethyl)lithium, THF, −100 °C → rt, 64%; d. benzyl alcohol, n-BuLi, THF, −78 °C → rt, then reflux, 70%; e. (dichloromethyl)lithium, ZnCl2, THF, −100 °C → rt, 61%; f. allylmagnesium bromide, THF, −78 °C → rt, 74%; g. H2O2, NaOH, THF, 0 °C → 45 °C, 90%. | |
By close analogy, the second asymmetric centre was introduced sequentially through stereoselective homologation of α-benzyloxy boronic ester 6. Addition of (dichloromethyl)lithium at −100 °C in THF,13 followed by treatment with zinc chloride (1 M solution in Et2O)10 provided 2-benzyloxy-1-chloroboronate 7 in good yield (61%)
(Scheme 2). In the absence of zinc chloride, the homologation reaction was found to display incomplete conversion and proceeded in lower yield, albeit with excellent diastereoselectivity. In contrast, addition of zinc chloride resulted in better conversions and yield, without affecting d.e. NMR spectroscopy confirmed the insertion of a new carbon atom (δH 3.65 ppm, doublet; δC 46.1 ppm, broad signal due to the C–B bond) and the Hendo proton was used again as diastereotopic marker to assess d.e., which was found to be greater than 98%. By use of the same chiral director, the S absolute configuration was installed.
The desired C11 backbone of fragment A was then constructed by exploiting the ease of reaction of α-halo boronic esters with C-nucleophiles, namely Grignard reagents.10 In the case of 1-chlorooctaneboronate 7, an allylic appendage was required for such a purpose. Accordingly, a solution of 7 in THF was reacted with allylmagnesium bromide at −78 °C, affording compound 8 in 74% yield (Scheme 2). Since nucleophilic displacements on α-chloroboronates are known to occur with SN2 mechanism,14 alkene 8 has opposite configuration at the boron-bearing C atom with respect to 7
(even though the same S chiral descriptor is used for priority reasons). Hence, boronic ester 8 already features the two adjacent asymmetric centres with the correct final stereochemistry required by 1. Finally, oxidative removal of the boronic scaffold by treatment with basic hydrogen peroxide in THF,10,11,15 which is reported to occur with retention of configuration,16 yielded the desired alcohol 9
(90%) with S,S absolute configuration (Scheme 2), thus releasing the free hydroxy function required for the coupling reaction with fragment B and meanwhile retaining the benzylic protection at the adjacent position (the C-1′-to-be in 1) to be cleaved off only at the end of the overall sequence.
Synthesis of fragment B
For the preparation of the acidic partner B, we devised using D-tartaric acid as the starting chiral synthon, to which a number of transformations described in the literature for the corresponding L-enantiomer were applied.Firstly, D-(−)-tartaric acid (10) was refluxed with 2,2-dimethoxypropane in the presence of p-toluenesulfonic acid to yield the corresponding dimethyl ester acetonide 11 in excellent yield (Scheme 3).17 Subsequent lithium aluminium hydride reduction in diethyl ether gave D-(−)-threitol acetonide 12
(87% yield),18 which was treated with sodium hydride in THF and reacted with tert-butyldimethylsilyl chloride at rt to afford the monosilylated derivative 13 on a multigram scale in excellent yield (91%)
(Scheme 3).19
 |
| Scheme 3 Synthesis of fragment B. Reagents and conditions: a. 2,2-dimethoxypropane, p-TsOH, reflux, 91%; b. LAH, Et2O, reflux, 87%; c. TBDMSCl, NaH, THF, 91%; d. Swern oxidation (oxalyl chloride–DMSO, CH2Cl2, triethylamine, −70 °C), 90%; e. (ethoxycarbonylmethylene)triphenylphosphorane, DMF, rt, 94%; f. H2, Pd/C, EtOH, rt, 99%; g. TBAF, THF, rt, 82%; h. Swern oxidation (oxalyl chloride–DMSO, CH2Cl2, triethylamine, −72 °C), 76%; i. methyltriphenylphosphonium bromide, n-BuLi, THF, −20 °C → rt, 42%; j. KOH, THF–MeOH–water, rt, 81%. | |
Alcohol 13 was oxidised to aldehyde 14 under Swern conditions at −70 °C;20–221H NMR analysis of the crude residue revealed the presence of the diagnostic proton at 9.78 ppm as a doublet, thus confirming the identity of the product, which was used without further purification for the next Wittig reaction. Homologation of 14 with (ethoxycarbonylmethylene)triphenylphosphorane in anhydrous DMF at rt22 smoothly afforded alkene 15 as an 85 : 15 trans–cis mixture in 85% combined yield (over two steps)
(Scheme 3); separation of the geometric isomers was not required since the newly formed double bond was to be hydrogenated in the following step. Catalytic hydrogenation of alkene 15 with 10% palladium on charcoal in ethanol under H2 atmosphere furnished hexanoate 16 in quantitative yield.23 Subsequently, removal of the TBDMS protecting group with tetra-n-butylammonium fluoride in dry THF at rt24 provided alcohol 17 in excellent yield (82%), thus releasing the hydroxy function at C-6 to be used for the final chain elongation, yet again by means of a Swern–Wittig sequence. Oxidation of 17 in the presence of oxalyl chloride–DMSO in dry CH2Cl2 at −72 °C, followed by treatment with triethylamine,20–22 gave aldehyde 18
(76%) which was immediately homologated with methylenetriphenylphosphorane in THF at −20 °C to provide 6-heptenoate derivative 19 in 42% yield (Scheme 3).25 Batty and Crich used buffered pyridinium chlorochromate (PCC) oxidation to prepare the (4S,5R) enantiomer of the L-threitol-derived aldehyde 18 in order to avoid migration of the isopropylidene group to the 5,6 site, which they experienced on the erythro analogue using other oxidation methods, including Swern's.25 In contrast, however, we did not observe any 5,6-isopropylidene shift during Swern oxidation of either alcohol 13 or 17, and both could be converted into the desired aldehyde 14 and 18, respectively. Saponification of the ester 19 with KOH in THF–MeOH–water (2 : 2 : 1) afforded the desired free acid 20 in 81% yield,25 which was directly employed for the next coupling step with alcohol 9.
Completion of the total synthesis
With the two alkene partners in hand, assembly of the dienic substrate for the crucial macrocyclization reaction via ring-closing metathesis (RCM)9 was performed by coupling alcohol 9 to acid 20 with DCC in the presence of DMAP in diethyl ether at rt, to give ester 21 in 85% yield (Scheme 4). A highly-diluted solution (0.45 mM) of diene 21 in anhydrous degassed dichloromethane was then refluxed for 48 h under argon atmosphere in the presence of Grubbs' catalyst I
(17 mol %)
(Fig. 2),9 to afford the 10-membered lactone 22 in excellent yield (92%) as a mixture of two geometric isomers in a 67 : 33 E–Z-ratio (Scheme 4). The desired trans-oxecin (E)-22 could be separated by column chromatography from the cis-analogue (Z)-22; the stereochemistry of the newly formed double bond was unambiguously assigned by comparison of the J7–8 coupling constants (15.6 against 10.3 Hz, respectively). |
| Fig. 2 Catalysts for ring-closing metathesis (RCM): I Grubbs' catalyst; II 2nd generation Grubbs' catalyst; III Fürstner's catalyst. | |
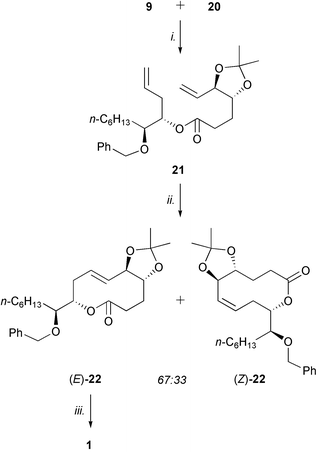 |
| Scheme 4 Completion of the total synthesis. Reagents and conditions: i., DCC, DMAP, Et2O, rt, 85%; ii., Grubbs' catalyst I, CH2Cl2, reflux, 92%; iii., TiCl4, CH2Cl2, 0 °C, 66%. | |
The same stereoselectivity in the RCM cyclization has been also reported by Marco and coworkers in the course of the first total synthesis of microcarpalide,26 by using a closely related diene ester that only differed in the protection at 1′-OH, whereas formation of the unwanted (Z)-olefin resulted upon exposure to second-generation Grubbs' catalyst II
(Fig. 2).
The lack of stereocontrol in the synthesis of medium-sized rings by RCM has been repeatedly addressed in the literature (for leading references, see ref. 27) and still represents a major drawback of this reaction. Mixtures of (E) and (Z) cycloalkenes are usually formed and, in addition, 8- to 11-membered rings are sensitive to the reverse process (ROM or ROMP) because of their intrinsic ring strain.27 Therefore, a reliable and general method of controlling the geometry of the newly formed double bond in RCM macrocyclizations has been called for. In this respect, second-generation Grubbs' catalyst II is known to favour the formation of the thermodynamically more stable (Z)-isomers.27
Within 10-membered lactones, for instance, in the total synthesis of pinolidoxin by Liu and Kozmin, the presence of an acetonide group spanning the two hydroxy functions in positions α and β to one of the alkene appendages was found to result in the stereoselective formation of the undesired (Z)-olefin in the key RCM step with catalyst II, whereas removal of the acetonide prior to metathetic ring closure determined a loss of stereocontrol in double bond formation.28 Similarly, but opposite in sense, excellent stereoselectivity in RCM was reported by Fürstner's group in the course of their recent total synthesis of herbarumins and pinolidoxin,27 a family of phytotoxic nonenolides related to 1, by using the newly developed ruthenium indenylidene catalyst III29
(Fig. 2). In particular, the stereocontrol of the key RCM macrocyclization was successfully reversed by simply switching to ruthenium complex III, resulting in the selective formation of the desired (E)-oxecin in a 10 : 1 ratio with the (Z)-isomer, which, in contrast, was formed exclusively when carbene II was employed as the catalyst.27
Prompted by such promising performances, we also exposed diene 21 to a catalytic amount of Fürstner's complex III,29 under similar reaction conditions. Although compound 21 was successfully metathesized to lactone 22, unfortunately the reaction afforded a mixture of (E) and (Z) isomers in about 2 : 1 ratio, respectively, as determined on the basis of 1H NMR analysis, by using the signal belonging to H-7 [for (E)-olefin: 5.32 ppm, doublet of doublets; for (Z): 5.50 ppm, triplet] as diagnostic marker.
In contrast, the most recent total synthesis of microcarpalide by Gurjar et al. featured an RCM reaction with Grubbs' catalyst I which proceeded with excellent stereoselectivity (10 : 1), yielding almost exclusively the required (E)-alkene from a diene ester similar to 21, except for the presence of two benzyl groups protecting the hydroxyls at positions 5 and 6, as well as for a methoxyethoxymethyl substituent at 1′-OH.30 Hence, the nature of the diene substrate that is subjected to the RCM reaction, and especially of its appendages, seems to play an important role in determining the stereochemical outcome of the metathetic process, at least for 10-membered lactones, thus making it difficult to draw up general rules for controlling selectivity in RCM reactions, even within a given catalyst.
Treatment of (E)-22 with titanium tetrachloride in CH2Cl2 at 0 °C30–31
(Scheme 4) resulted in the simultaneous removal of both protective groups, affording a single product whose spectral data matched perfectly those reported by Hemscheidt3 for the natural compound. Like natural microcarpalide, when dissolved in acetonitrile synthetic 1 appears as a mixture of two slowly interconverting conformers in a 76 : 24 ratio, as determined from the 1H NMR spectrum by using signals which displayed suitable separation, namely those for H-10 (4.84 ppm for the major conformer and 4.63 ppm for the minor one) and, in addition, 5-OH (3.09 and 3.19 ppm, respectively). This conformer ratio is identical to the 3.5 : 1 value described in the literature for the natural 1 in the same solvent.3
At present, only two total syntheses of microcarpalide have been disclosed in the literature so far.26,30 Like ours, both rely on the construction of the 10-membered skeleton by means of a ring-closing metathesis (RCM) macrocyclization using Grubbs' catalyst I as the crucial step, thus requiring the synthesis of two alkene partners, namely A and B
(Scheme 1). Subunit B was always prepared from the chiral pool, by starting either from D-tartaric acid26 or D-mannose;30 the latter, however, required a cumbersome and unduly lengthy sequence, thus making the tartrate-derived one more attractive and applicable, even though a more straightforward and, possibly, enantioselective approach would be desirable for future developments. As far as partner A is concerned, it was previously synthesized by means of diastereoselective addition of an allyltin reagent to an (R)-glycidol-derived aldehyde as the key step26 or, later,30 through asymmetric dihydroxylation of a suitable alkene precursor, followed by a long sequence of transformations. In contrast, here a completely different and original route to fragment A was followed, which fully exploited the stereoselective homologation of chiral boronic esters10 as a powerful tool to introduce sequentially stereocentres with high diastereoselectivity and desired absolute stereochemistry by use of the same chiral auxiliary.
Conclusions
In summary, a new stereoselective total synthesis of the microfilament disrupting agent microcarpalide (1), a secondary metabolite produced by an endophytic fungus, has been accomplished. Formation of the required 10-membered lactone was achieved by means of ring-closing metathesis (RCM) of a suitable diene ester that was assembled by coupling the two advanced fragments A and B
(i.e.9 and 20, respectively). Fragment A has been synthesized in 7 steps from n-bromohexane through two consecutive stereoselective homologations of chiral boronic esters 4 and 6 as key transformations, which allowed the sequential introduction of the two asymmetric centres with the final S absolute configuration, using (+)-pinanediol as the chiral auxiliary. Final elaboration entailed insertion of the allylic appendage on derivative 7 by nucleophilic displacement of the chlorine atom, followed by oxidative removal of the boronic scaffold to reveal alcohol 9. In turn, fragment B has been prepared in 10 steps from D-(−)-tartaric acid. The required C7 backbone of 20 has been constructed from the C4 starting synthon using two different Swern–Wittig oxidation–homologation sequences as key steps, each one with a suitable phosphorus ylide. Hence, D-tartrate-derived monosilylated threitol 13 was oxidized and homologated to afford alkene 15, which was hydrogenated and exposed to TBAF, thus revealing alcohol 17. Swern oxidation and Wittig methylenation furnished ester 19, whose saponification yielded 6-heptenoic acid 20. Completion of the total synthesis entailed a DCC-mediated coupling between partners 9 and 20, followed by RCM using Grubbs' catalyst I, providing the desired 10-membered macrocyclic framework with good stereoselectivity (67 : 33 E–Z) and excellent yield. Finally, cleavage of protective groups afforded a single product whose spectroscopic properties were identical with those of the natural compound 1.With a view to future structure–activity relationship (SAR) studies, the synthetic route to 1 herein disclosed appears flexible enough to allow the preparation of microcarpalide analogues, by simply varying the starting materials and/or the chiral director employed. In particular, our total synthesis features an original enantioselective approach to fragment A that allows the asymmetric insertion of suitably labelled atoms in specific positions by means of Matteson's stereoselective homologation, thus making available labelled derivatives which could be of value for shedding light on the mechanism of action of this novel fungal metabolite endowed with such a peculiar biological activity. Further applications of this methodology toward the preparation of analogues for SAR studies are being currently pursued in our laboratory and will be reported in due course.
Experimental
General
1H and 13C NMR spectra were recorded in CDCl3 solution (unless otherwise noted) on a Bruker DPX 200 or AMQ 400 MHz spectrometer; chemical shifts are reported in δ values from TMS as internal standard; coupling constants (J) are given in Hz. For mass spectral determinations a Finnigan MAT SSQ A and a Hewlett Packard HP5989A mass spectrometer were used (EI, 70 eV). Elemental analyses were performed with a Carlo Erba Elemental Analyzer 1110. Optical rotations were recorded in chloroform (unless stated otherwise) at 20 °C on a Perkin-Elmer 241 polarimeter and are in 10−1 deg cm2 g−1. All organic solvents were dried and distilled by standard methods prior to use and all reactions requiring anhydrous conditions were carried out using oven-dried and argon-flushed glassware. Chromatographic purification of compounds was performed on silica gel (particle size 0.05–0.20 mm). Analytical TLC was performed on pre-loaded (0.25 mm) glass supported silica gel plates (Merck, Kieselgel 60, F254). Compounds were visualized by exposure to UV light, I2 vapours and by dipping the plates in 1% Ce(SO4)2·4H2O, 2.5%
(NH4)Mo7O24·4H2O in 10% sulfuric acid followed by heating on a hot plate. (1S,2S,3R,5S)-(+)-Pinanediol and all other reagents were obtained from Aldrich. Grubbs' catalyst I was purchased from Strem Chemicals Ltd., whereas dichloro(3-phenyl-1H-inden-1-ylidene)bis(tricyclohexylphosphine)ruthenium(IV)
(Fürstner's catalyst, III) was prepared according to Fürstner et al.29Synthesis of fragment A
Hexaneboronic acid (3)12,32,33. Activated magnesium turnings (4.03 g, 16.58 mmol) were suspended in anhydrous diethyl ether (5 mL) in a 100-mL two-necked round bottom flask equipped with a reflux condenser and a dropping funnel. A portion of neat 1-bromohexane (0.4 mL, 2.85 mmol) was added straightaway along with a few crystals of I2 in order to initiate the reaction. Once the mixture was refluxing, the remaining halide (1.3 mL, 9.25 mmol) dissolved in Et2O (3 mL) was added dropwise, and the reaction was kept under gentle reflux for about 50 min. In a separate flask, trimethyl borate (1.41 mL, 12.10 mmol) was dissolved in the same solvent (8 mL), and the milky solution of n-hexylmagnesium bromide was slowly added via syringe at −78 °C under mechanical stirring and Ar atmosphere over 10 min, washing with a further 2 mL of ether; during addition, the temperature rose and was carefully maintained below −60 °C. The reaction mixture was stirred overnight and left to cool to rt, affording a light yellowish precipitate. After dilution with Et2O (20 mL), the mixture was cooled at 0 °C and 10% aqueous sulfuric acid (12 mL) was added dropwise; the clear solution thus obtained was warmed and stirred for 20 min at rt. The yellow ethereal phase was separated and the aqueous layer extracted with ether (3 × 12 mL); the white emulsion that formed initially upon separation was subjected to the same extraction protocol. The organic phases were pooled, washed with water and brine, and dried over MgSO4. Filtration and concentration in vacuo afforded the title compound 3
(5.69 g, 72%) as a white solid (mp 68–71 °C)
(lit.12,32,33 88–90 °C) which was used as such in the following step without further purification. 1H NMR (200 MHz, as the corresponding boroxine): δ 0.79–1.00 (5H, m, CH2B and CH3), 1.22–1.53 (8H, m, 4 × CH2). 13C NMR: δ 14.3, 23.0, 25.9, 30.0, 32.0 (CH2B not seen). MS, m/z
(as boroxine): 336 (M+, 75%), 306 (26), 278 (20), 265 (80), 250 (5), 195 (56), 167 (30), 84 (100), 69 (35), 55 (60). [Found: C, 64.3; H, 11.6. C18H39B3O3
(boroxine) requires: C, 64.4; H, 11.7%]. (+)-Pinanediol hexaneboronate (4). Boronic acid 3
(920 mg, 7.08 mmol) was dissolved in anhydrous diethyl ether (10 mL) and stirred at rt in the presence of (1S,2S,3R,5S)-(+)-pinanediol (1.21 g, 7.08 mmol), which was added portionwise over 1 h 15 min, until TLC analysis (light petroleum–ethyl acetate 80 : 20) showed no disappearance of the pinanediol itself. After rotary evaporation under reduced pressure, the desired ester 4 was separated from the unreacted pinanediol by means of column chromatography (light petroleum–ethyl acetate 80 : 20), affording 1.002 g (71%) of a colourless oil (bp 80 °C, 0.09 mbar), [α]D
+20.9 (c 0.90). 1H NMR (200 MHz): δ 0.78–0.95 (8H, m, 3 ×
H-6, 2 × CH2B and pinanyl CH3), 1.15 (1H, d, J 12.3, pinanyl Hendo), 1.24–1.56 (14H, m, H-2 to H-5 and 2 × pinanyl CH3), 1.80–2.45 (5H, m, pinanyl protons), 4.27 (1H, dd, J 8.6, 1.8, pinanyl CHOB). 13C NMR: δ 10.5 (m, BCH2), 14.4, 22.9, 24.3, 24.5, 26.8, 27.5, 29.1, 30.1, 32.0, 32.5, 36.0, 38.5, 40.0, 51.8, 77.9, 85.6. MS, m/z: 264 (M+, 11%), 249 (48), 235 (3), 223 (26), 195 (67), 181 (20), 168 (59), 134 (69), 109 (31), 83 (90), 67 (100), 55 (95). (Found: C, 72.7; H, 11.0. C16H29BO2 requires: C, 72.7; H, 11.1%).
(+)-Pinanediol (1S)-1-chloroheptaneboronate (5). A solution of anhydrous methylene chloride (183 µL, 2.85 mmol) in freshly distilled dry THF (4 mL) was cooled at −100 °C and treated with a 2.5 M solution of n-BuLi in hexanes (912 µL, 2.28 mmol) under Ar flow and mechanical stirring; n-BuLi addition took place over a 10 min period and the reaction mixture was then stirred for an additional 30 min, carefully maintaining the temperature below −100 °C. A white precipitate formed, which slowly faded. Pinanediol ester 4
(500 mg, 1.90 mmol) was dissolved in THF (3 mL) and slowly added dropwise to the (dichloromethyl)lithium solution at −100 °C. The reaction mixture was stirred under argon atmosphere and allowed to warm to rt overnight. After rotary evaporation, the residue was diluted with light petroleum (100 mL) and treated with saturated NH4Cl (25 mL). The aqueous phase was extracted with light petroleum (2 × 50 mL), the combined organic phases were dried over Na2SO4, filtered and concentrated under reduced pressure. Column chromatography on SiO2 using light petroleum–diethyl ether 97 : 3 as the eluant gave homologation product 5
(380 mg, 64%) as a clear yellow liquid, [α]D
+27.3 (c 0.87). 1H NMR (200 MHz): δ 0.88–0.94 (6H, m, 3 ×
H-7 and pinanyl CH3), 1.19 (1H, d, J 10.9, pinanyl Hendo), 1.24–1.56 (16H, m, H-2 to H-6 and 2 × pinanyl CH3), 1.80–2.45 (5H, m, pinanyl protons), 3.48 (1H, dd, J 7.7, 6.8, H-1), 4.38 (1H, dd, J 8.8, 2.0, pinanyl CHOB). 13C NMR: δ 14.4, 23.0, 24.3, 26.7, 27.4, 27.6, 28.8, 29.1, 31.8, 34.6, 35.7, 38.6, 39.8, 51.6, 78.9, 87.0 (ClCHB not seen). MS, m/z: 312 (M+, 3%), 297 (4), 217 (32), 199 (40), 173 (36), 158 (43), 145 (100), 134 (98), 118 (78), 96 (52), 83 (70), 67 (54), 55 (57). (Found: C, 65.2; H, 9.7. C17H30BClO2 requires: C, 65.3; H, 9.7%).
(+)-Pinanediol (1R)-1-benzyloxyheptaneboronate (6). Benzyl alcohol (759 µL, 7.34 mmol) was dissolved in anhydrous THF (6 mL) in a 25-mL flask equipped with a dropping funnel and a reflux condenser, and a few crystals of oven-dried (110 °C, 20 min) 1,10-phenanthroline were introduced. The solution was cooled at −78 °C and a 2.5 M solution of n-BuLi in hexanes (3.22 mL, 8.00 mmol) was added until the mixture turned dark red. After stirring for 15 min at −78 °C and for an additional 5 min at rt under argon flow, chloro derivative 5
(2.081 g, 6.67 mmol) in 6 mL THF was slowly added via syringe to the reaction flask at −78 °C. The pale yellow solution thus obtained was allowed to warm to rt overnight under Ar atmosphere. Complete conversion was reached after gentle refluxing of the reaction mixture for 2 h. After dilution with Et2O (1 : 1 v/v with respect to THF) and treatment with saturated NH4Cl (10 mL), the organic phase was separated and the aqueous layer extracted with ether (3 × 60 mL). The organic phases were pooled, dried (Na2SO4), filtered and rotary evaporated in vacuo. The residue was purified by chromatography (light petroleum–diethyl ether 95 : 5), affording benzyloxy derivative 6 as a fruit-scented bright yellow oil (1.75 g, 70%), [α]D
+4.8 (c 2.4). In separate experiments, the title compound 6 was also prepared on a gram scale directly from boronate 4 in a total 55% yield (over two steps), following an improved one-pot procedure that did not require isolation of the intermediate chloro derivative 5. 1H NMR (200 MHz): δ 0.86 (3H, s, pinanyl CH3), 0.89 (3H, t, J 5.5, 3 ×
H-7), 1.17 (1H, d, J 10.8, pinanyl Hendo), 1.24–1.52 (16H, m, H-2 to H-6 and 2 × pinanyl CH3), 1.62–2.45 (5H, m, pinanyl protons), 3.36 (1H, t, J 6.4, H-1), 4.33 (1H, dd, J 8.8, 2.0, pinanyl CHOB), 4.52 (1H, d, J 12.1, CH2Ph), 4.62 (1H, d, J 12.1, CH2Ph), 7.19–7.42 (5H, m, arom). 13C NMR: δ 14.4, 23.0, 24.4, 26.9, 27.4, 29.1, 29.8, 31.8, 32.2, 35.8, 38.5, 39.9, 51.6, 68.3 (br, CHB), 72.5, 78.5, 86.5, 127.6, 128.2, 128.5, 139.6. MS, m/z: 384 (M+, 4%), 297 (6), 235 (7), 135 (61), 107 (25), 97 (43), 91 (100), 69 (17), 55 (43). (Found: C, 74.8; H, 9.6. C24H37BO3 requires: C, 75.0; H, 9.7%).
(+)-Pinanediol (1S,2S)-2-benzyloxy-1-chlorooctaneboronate (7). By close analogy to the synthesis of 5 reported above, (dichloromethyl)lithium was prepared by treatment of dichloromethane (158 µL, 2.46 mmol) in THF (4 mL) with n-BuLi (2.5 M solution in hexanes, 676 µL, 1.64 mmol) at −100 °C. Benzyl ether 6
(591 mg, 1.54 mmol) in THF (4 mL) was then added to such a mixture at −100 °C, and after 5 min a 1 M solution of ZnCl2 in diethyl ether (923 µL, 0.92 mmol) was slowly dropped in. The reaction was stirred under Ar atmosphere and left to warm to rt overnight. The clear and colourless solution thus obtained was concentrated under reduced pressure, diluted with light petroleum (40 mL) and treated with saturated NH4Cl (10 mL). After separation of the organic layer, the aqueous phase was extracted with light petroleum (3 × 15 mL), and the organic phases were pooled, dried over MgSO4, filtered and rotary evaporated. After chromatographic purification (light petroleum–diethyl ether 95 : 5), the title compound 7 was obtained as a pale yellow liquid (374 mg, 61%), [α]D
+7.7 (c 2.3). 1H NMR (200 MHz): δ 0.83–0.95 (6H, m, 3 ×
H-8 and pinanyl CH3), 1.17 (1H, d, J 10.8, pinanyl Hendo), 1.27–1.48 (16H, m, H-3 to H-7 and 2 × pinanyl CH3), 1.60–2.45 (5H, m, pinanyl protons), 3.65 (1H, d, J 6.5, H-1), 3.81 (1H, dt, J 5.3, 6.5, H-2), 4.38 (1H, dd, J 8.8, 2.0, pinanyl CHOB), 4.62 (1H, d, J 12.0, CH2Ph), 4.72 (1H, d, J 12.0, CH2Ph), 7.20–7.45 (5H, m, arom). 13C NMR: δ 14.4, 23.0, 24.3, 25.8, 26.7, 26.8, 28.8, 29.6, 32.1, 32.5, 35.6, 38.5, 39.8, 46.1 (br, CHCl), 51.6, 72.9, 78.9, 80.9, 87.2, 127.6, 128.1, 128.5, 139.0. MS, m/z: 324 ([M − PhCH2OH]+, 30%), 309 (100), 293 (2), 247 (4), 147 (3), 133 (5), 119 (10), 91 (6). (Found: C, 69.6; H, 8.9. C25H38BClO3 requires: C, 69.4; H, 8.8%).
(+)-Pinanediol (1S,1′S)-1-(1′-benzyloxy)heptylbut-3-eneboronate (8). A 1 M solution of allylmagnesium bromide in diethyl ether (1.88 mL, 1.88 mmol) was slowly added via syringe over 10 min to a stirred solution of chloro derivative 7
(730 mg, 1.71 mmol) in anhydrous THF (20 mL) at −78 °C under Ar flow. The mixture was warmed to rt overnight, and a white precipitate began to form while the temperature was rising. The yellow solution was then diluted with light petroleum (20 mL), treated with saturated NH4Cl (20 mL) and the organic phase was separated and dried over MgSO4. Filtration and concentration in vacuo afforded an oily residue which was purified by chromatography (light petroleum–diethyl ether 95 : 5) to give 8 as a pale yellow oil (575 mg, 74%), [α]D
+12.7 (c 4.4). 1H NMR (200 MHz): δ 0.83 (3H, s, pinanyl CH3), 0.89 (3H, t, J 6.3, H-7′), 1.20 (1H, d, J 10.1, pinanyl Hendo), 1.24–1.45 (16H, m, H-2′ to H-6′ and 2 × pinanyl CH3), 1.50–2.40 (8H, m, H-1, H-2 and pinanyl protons), 3.58 (1H, q, J 5.5, H-1′), 4.26 (1H, dd, J 8.8, 2.0, pinanyl CHOB), 4.53 (2H, AB system, J 11.2, CH2Ph), 4.90–5.09 (2H, m, H-4), 5.88 (1H, ddt, J 16.9, 10.1, 6.5, H-3), 7.18–7.40 (5H, m, arom). 13C NMR: δ 14.4, 23.0, 24.4, 25.8, 26.7, 27.5, 29.1, 29.8, 31.7, 32.2, 33.1, 35.9, 38.5, 39.9, 51.6, 71.3, 78.0, 81.2, 85.8, 115.0, 127.4, 127.9, 128.4, 139.4, 139.7. The EIMS was unobtainable. (Found: C, 76.8; H, 9.8. C28H43BO3 requires: C, 76.7; H, 9.9%).
(4S,5S)-5-Benzyloxyundec-1-en-4-ol (9). Allyl boronate 8
(50 mg, 0.11 mmol) was dissolved in THF (2 mL) and treated with a 2.2 M solution of NaOH (152 µL, 0.34 mmol) at 0 °C for 10 min under magnetic stirring. 35% Hydrogen peroxide (34 µL, 0.30 mmol) was added at 0 °C and the mixture was stirred for 15 min, during which a yellow precipitate formed. The reaction was then warmed to 45 °C and the milky solution stirred for an additional 1.5 h, until TLC analysis (light petroleum–diethyl ether 95 : 5) showed disappearance of the starting material. After addition of diethyl ether (15 mL) and water (5 mL), the organic layer was separated and dried over MgSO4. Purification of the bright yellow residue obtained upon filtration and rotary evaporation was achieved by column chromatography, using light petroleum–diethyl ether 70 : 30 as the eluant, thus affording the title compound 9 as a colourless liquid (39 mg, 90%), [α]D
+17.8 (c 1.5), which upon refrigeration slowly crystallised in transparent needles. 1H NMR (200 MHz): δ 0.90 (3H, t, J 6.7, H-11), 1.22–1.81 (10H, m, H-6 to H-10), 2.15–2.45 (3H, m, OH and H-3), 3.34 (1H, q, J 5.5, H-5), 3.56–3.72 (1H, m, H-4), 4.52 (1H, d, J 11.3, CH2Ph), 4.67 (1H, d, J 11.3, CH2Ph), 5.04–5.16 (2H, m, H-1), 5.87 (1H, ddt, J 17.6, 9.6, 7.0, H-2), 7.24–7.40 (5H, m, arom). 13C NMR: δ 14.4, 22.9, 25.5, 29.9, 30.6, 32.1, 38.5, 72.4, 72.8, 81.8, 117.6, 128.1, 128.2, 128.8, 135.4, 140.1. MS, m/z
(as the corresponding TMS ether, by treatment in situ with neat MSTFA at 70 °C for 1 h): 333 ([M − 15]+, 0.5%), 307 (5), 241 (0.6), 205 (6), 152 (6), 143 (44), 91 (100), 73 (29). (Found: C, 78.2; H, 10.1. C18H28O2 requires: C, 78.2; H, 10.2%).
Synthesis of fragment B
Dimethyl 2,3-O-isopropylidene-D-(+)-tartrate (11). The title compound was prepared in 91% yield on a 50-g scale from commercial D-(−)-tartaric acid (10) according to Carmack and Kelley;17
[α]D
+42.0 (neat)
[lit.,17
(L-isomer)
−49.4]. 2,3-O-Isopropylidene-D-(−)-threitol (12)34. LAH (10.11 g, 0.266 mol) was slurried in dry ether (100 mL) and refluxed for 30 min. Dimethyl 2,3-O-isopropylidene-D-(+)-tartrate (11)
(28.742 g, 0.132 mol) was dissolved in dry ether (130 mL) and slowly added at rt under vigorous magnetic stirring. The reaction mixture was refluxed for about 3 h and stirred at rt overnight. After cooling with ice, ethyl acetate (12 mL) was carefully added dropwise, followed by water (10 mL, cautiously!), 4 M NaOH (10 mL) and, again, water (30 mL); the suspension was stirred until gas release ceased and the conspicuous ivory precipitate which had settled down was filtered off, washing with abundant ether. The filter cake was extracted thoroughly and repeatedly with ether in a Soxhlet apparatus, and the pooled ethereal extracts were dried (MgSO4), filtered and concentrated in vacuo. Claisen distillation of the residue afforded D-threitol derivative 12
(18.6 g, 87%) as a pale yellow dense oil, bp 94–96 °C (0.7 mmHg), [α]D
−2.1 (c 2.6)
(lit.,34
−3.1), which crystallised upon freezing. 1H NMR (200 MHz): δ 1.36 (6H, s, 2 × CH3), 3.40 (2 × 1H, br, 2 × OH), 3.67 (4H, br, 2 × CH2), 3.89 (2H, m, 2 × CH). 13C NMR: δ 27.3, 62.6, 78.8, 109.6. MS, m/z
(as the corresponding bisTMS ether, by derivatisation in situ with neat MSTFA at 100 °C for 3.5 h): 307 ([M + 1]+, 0.07%), 291 (90), 231 (22), 216 (36), 203 (40), 185 (18), 145 (60), 131 (69), 117 (58), 103 (88), 73 (100), 59 (14), 43 (11). (Found: C, 51.9; H, 8.8. C7H14O4 requires: C, 51.8; H, 8.7%). (2R,3R)-4-(tert-Butyldimethylsilyloxy)-2,3-(isopropylidenedioxy)butanol (13). Sodium hydride (0.66 g, 27.5 mmol; as 60% dispersion in mineral oil) was added portionwise to a stirred solution of D-(−)-threitol acetonide 12
(4.006 g, 24.70 mmol) in anhydrous THF (150 mL) cooled to 0 °C under Ar flow. When effervescence ceased, a solution of tert-butyldimethylsilyl chloride (4.105 g, 27.2 mmol) in dry THF (50 mL) was slowly added dropwise to the slurry at rt and stirred vigorously overnight under Ar atmosphere. The milky mixture was poured in water (120 mL), the yellow clear organic phase was separated and the aqueous layer extracted with ethyl acetate (3 × 50 mL). The organic phases were combined, washed with water (100 mL), dried (MgSO4) and rotary evaporated, giving a yellow residue which was chromatographed on silica gel (light petroleum–ethyl acetate from 90 : 10 to 50 : 50) to afford the desired monosilylated alcohol 13
(6.21 g, 91%) as a lemon yellow oil, [α]D
−16.1 (c 3.2)
[lit.,19,21
(2S,3S)
+16.1;19
+15.321]. 1H NMR (200 MHz): δ 0.06 (6H, s, 2 ×
tBuMe2Si), 0.88 (9H, s, 3 ×
tBuMe2Si), 1.37 (3H, s, Me2C), 1.39 (3H, s, Me2C), 2.55 (1H, t, J 6.0, OH), 3.60 (6H, m, 2 × CH and 2 × CH2). 13C NMR: δ
−4.19, −4.16, 19.6, 27.2, 28.2, 28.4, 64.1, 65.1, 79.4, 81.4, 110.4. MS, m/z: 275 ([M − 1]+, 0.07%), 261 (17), 245 (2.8), 219 (14), 201 (3.5), 187 (4.6), 173 (5.6), 161 (44), 143 (20), 131 (100), 117 (43), 105 (16), 89 (14), 75 (96), 59 (41), 43 (18). (Found: C, 56.4; H, 10.3. C13H28O4Si requires: C, 56.5; H, 10.2%). (2S,3R)-4-(tert-Butyldimethylsilyloxy)-2,3-(isopropylidenedioxy)butanal (14). Oxalyl chloride (588 µL, 6.84 mmol) was dissolved in dry CH2Cl2
(13 mL) in a three-necked 100-mL round bottom flask equipped with two dropping funnels and a bubbler, and treated at −80 °C with a solution of anhydrous DMSO (970 µL, 13.68 mmol) in CH2Cl2
(4.5 mL), which was added dropwise over 15 min. The milky solution thus obtained was stirred at −72 °C under Ar flow for an additional 30 min. Alcohol 13
(1.716 g, 6.22 mmol) was dissolved in dry CH2Cl2
(8 mL) and slowly added at −70 °C over a 15 min period. After stirring at −60 °C for a further 40 min, freshly distilled anhydrous triethylamine (4.33 mL, 31.08 mmol) was added, and the reaction mixture was stirred for 15 min at the same temperature. The cold bath was then removed, the mixture allowed to warm to rt and poured in 30 mL water. The lower organic layer was separated and the aqueous phase extracted with CH2Cl2
(3 × 20 mL); the pooled organic layers were washed with saturated NH4Cl, satd. NaHCO3 and brine (60 mL each), and dried over Na2SO4. After filtration and concentration in vacuo a yellow sticky oil was obtained (1.53 g, 90%). After being repeatedly treated with anhydrous toluene and rotary evaporated in order to remove azeotropically any trace of water left, this crude material was directly used in the next step. 1H NMR (200 MHz): δ 0.08 (3H, s, tBuMe2Si), 0.09 (3H, s, tBuMe2Si), 0.91 (9H, s, 3 ×
tBuMe2Si), 1.41 (3H, s, Me2C), 1.42 (3H, s, Me2C), 3.66–4.16 (3H, m, CHCH2 and CH2), 4.33 (1H, dd, J 7.2, 1.6, CHCHO), 9.78 (1H, d, J 1.6, CHO). MS, m/z: 259 ([M − 15]+, 4.9%), 245 (9.5), 217 (13), 199 (4.2), 187 (7.2), 171 (4.0), 159 (50), 145 (6.7), 131 (60), 117 (100), 101 (60), 89 (16), 75 (75), 59 (25), 43 (18).
(2E,4R,5R)- and (2Z,4R,5R)-6-(tert-Butyldimethylsilyloxy)-4,5-(isopropylidenedioxy)hex-2-enoic acid ethyl ester (15). The crude aldehyde 14
(1.189 g, 4.33 mmol) was dissolved in anhydrous DMF (36 mL) and (ethoxycarbonylmethylene)triphenylphosphorane (4.448 g, 12.77 mmol) was added portionwise at rt. The mixture was stirred overnight, poured in 350 mL water and thoroughly extracted with light petroleum (5 × 150 mL). After drying over MgSO4, filtration and evaporation under reduced pressure, the residue was purified by silica gel chromatography using light petroleum–diethyl ether 70 : 30 as the eluant, to afford the title alkene 15 in an 85 : 15 trans–cis mixture (as determined by GC-MS analysis) as a light yellow liquid (1.402 g, 94%). (Found: C, 59.5; H, 9.5. C17H32O5Si requires: C, 59.3; H, 9.4%).
(2E,4R,5R)-(15). 1H NMR (200 MHz): δ 0.07 (6H, s, 2 ×
tBuMe2Si), 0.90 (9H, s, 3 × Me2tBuSi), 1.29 (3H, t, J 7.1, CH2CH3), 1.42 (6H, s, 2 ×
Me2C), 3.69–3.86 (3H, m, H-5 and 2 ×
H-6), 4.20 (2H, q, J 7.1, CH2CH3), 4.45–4.56 (1H, m, H-4), 6.12 (1H, dd, J 15.7, 1.6, H-2), 6.94 (1H, dd, J 15.7, 5.1, H-3). 13C NMR: δ
−4.13, −4.07, 15.6, 19.6, 27.2, 28.1, 28.3, 61.8, 64.1, 79.2, 82.1, 111.2, 123.3, 146.0, 176.7. MS, m/z: 329 ([M − 15]+, 13%), 299 (7.3), 281 (5.9), 241 (4.0), 229 (100), 199 (20), 183 (34), 155 (34), 117 (36), 109 (51), 89 (41), 84 (29), 75 (74), 59 (21), 43 (18).
(2Z,4R,5R)-(15). 1H NMR (200 MHz): δ 0.057 (3H, s, tBuMe2Si), 0.061 (3H, s, tBuMe2Si), 0.88 (9H, s, 3 ×
tBuMe2Si), 1.28 (3H, t, J 7.1, CH2CH3), 1.43 (6H, s, 2 ×
Me2C), 3.69–3.86 (3H, m, H-5 and 2 ×
H-6), 4.17 (2H, q, J 7.1, CH2CH3), 4.76–4.84 (1H, m, H-4), 5.91 (1H, dd, J 11.7, 1.0, H-2), 6.18 (1H, dd, J 11.7, 8.6, H-3). 13C NMR: δ
−4.0, 27.3, 28.4, 28.5, 61.7, 65.2, 75.0, 83.6, 111.3, 124.2, 146.9, (C
O not seen). MS, m/z: 329 ([M − 15]+, 4.3%), 287 (26), 269 (24), 241 (17), 229 (91), 199 (9.2), 183 (68), 155 (34), 117 (52), 109 (100), 89 (15), 84 (23), 75 (62), 59 (20), 43 (18). (4R,5R)-6-(tert-Butyldimethylsilyloxy)-4,5-(isopropylidenedioxy)hexanoic acid ethyl ester (16). Alkene 15
(1.321 g, 3.84 mmol) was dissolved in absolute EtOH (20 mL) and stirred overnight with 10% wt. dry Pd on C (200 mg) at rt under H2 balloon pressure. The reaction mixture was passed through a short Celite plug, washing with diethyl ether and EtOH, and the resulting clear solution was evaporated to dryness to afford 16 as a colourless liquid (1.31 g, 99%) which was used as such for the next reaction. For analysis, a small sample was purified by silica gel chromatography, using light petroleum–diethyl ether 80 : 20 as the eluant; [α]D
+5.9 (c 1.5). 1H NMR (200 MHz): δ 0.07 (6H, s, 2 ×
tBuMe2Si), 0.89 (9H, s, 3 ×
tBuMe2Si), 1.25 (3H, t, J 7.1, CH2CH3), 1.36 (3H, s, Me2C), 1.38 (3H, s, Me2C), 1.75–2.14 (2H, m, 2 ×
H-3), 2.34–2.60 (2H, m, 2 ×
H-2), 3.63–3.82 (3H, m, H-4 and 2 ×
H-6), 3.85–3.95 (1H, m, H-5), 4.13 (2H, q, J 7.1, CH2CH3). 13C NMR: δ
−4.12, −4.06, 15.6, 19.7, 27.2, 28.3, 28.8, 28.9, 32.1, 61.6, 65.0, 79.3, 82.2, 110.0, 174.6. MS, m/z: 345 ([M − 1]+, 0.1%), 331 (36), 301 (13), 289 (33), 271 (36), 243 (42), 231 (90), 185 (100), 157 (35), 143 (73), 111 (93), 83 (27), 75 (48), 59 (15), 43 (12). (Found: C, 59.2; H, 10.1. C17H34O5Si requires: C, 58.9; H, 9.9%).
(4R,5R)-6-Hydroxy-4,5-(isopropylidenedioxy)hexanoic acid ethyl ester (17). Silylated alcohol 16
(1.26 g, 3.64 mmol) was dissolved in anhydrous THF (10 mL) and treated with a solution of tetra-n-butylammonium fluoride (1.142 g, 4.37 mmol) in 6 mL THF at rt under magnetic stirring and Ar atmosphere. After 5 h the solvent was rotary evaporated, the yellowish residue dissolved in ethyl acetate (15 mL) and washed with water (15 mL). The aqueous layer was extracted with EtOAc (15 mL) and the pooled organic phases were dried (Na2SO4) and filtered. Concentration in vacuo followed by chromatography on silica gel (light petroleum–diethyl ether, from 70 : 30 to 40 : 60) yielded free alcohol 17 as a pale yellow oil (689 mg, 82%), [α]D
+24.8 (c 1.1)
[lit.,21,25
(4S,5S)
−24.1;25
−19.521]. 1H NMR (200 MHz): δ 1.26 (3H, t, J 7.1, CH2CH3), 1.39 (3H, s, Me2C), 1.40 (3H, s, Me2C), 1.70–2.06 (2H, m, 2 ×
H-3), 2.10 (1H, t, J 5.4, OH), 2.42 (1H, ddd, J 16.4, 8.2, 7.0, H-2), 2.54 (1H, ddd, J 16.4, 8.4, 6.7, H-2), 3.59–3.68 (1H, m, H-5), 3.70–3.85 (2H, m, 2 ×
H-6), 3.91 (1H, dt, J 3.8, 7.9, H-4), 4.14 (2H, q, J 7.1, CH2CH3). 13C NMR: δ 15.5, 28.4, 28.6, 29.4, 32.0, 61.8, 63.3, 77.6, 82.4, 110.3, 174.6. MS, m/z: 217 ([M − 15]+, 62%), 187 (7.3), 171 (8.9), 156 (7.0), 143 (23), 129 (40), 115 (45), 111 (67), 101 (22), 83 (44), 59 (100). (Found: C, 56.8; H, 8.9. C11H20O5 requires: C, 56.9; H, 8.7%). (4R,5S)-4,5-(Isopropylidenedioxy)-6-oxohexanoic acid ethyl ester (18). By analogy to the preparation of aldehyde 14 described above, a solution of DMSO (404 µL, 5.70 mmol) in anhydrous CH2Cl2
(2 mL) was carefully added dropwise under Ar flow over a 3 min period to a stirred solution of oxalyl chloride (245 µL, 2.85 mmol) in the same solvent (5.5 mL) cooled at −76 °C. The mixture was allowed to react for 30 min at the same temperature, until a milky turbid appearance was observed. Thereafter, alcohol 17
(601 mg, 2.59 mmol) was dissolved in dry CH2Cl2
(3.5 mL) and slowly added at −72 °C to the white mixture over a 15 min period. After stirring for 1 h at −65 °C under Ar flow, anhydrous triethylamine (1.8 mL, 12.95 mmol) was added dropwise at −61 °C. The reaction mixture was stirred for 15 min at the same temperature, left to warm to rt over 2 h and poured into 15 mL water. The whitish clear bottom phase was separated from the aqueous layer, which was extracted with CH2Cl2
(3 × 15 mL); the organic phases were combined, washed with 1 M HCl (50 mL), saturated NaHCO3
(50 mL) and brine (50 mL), dried (Na2SO4), filtered and evaporated under reduced pressure to give the crude aldehyde 18 as a lemon yellow thick oil (455 mg, 76%), which was used for the next Wittig reaction without further purification, except for repeated treatments with anhydrous toluene followed by rotary evaporation. 1H NMR (200 MHz): δ 1.26 (3H, t, J 7.1, CH2CH3), 1.39 (3H, s, Me2C), 1.41 (3H, s, Me2C), 1.81–2.14 (2H, m, 2 ×
H-3), 2.38–2.60 (2H, m, 2 ×
H-2), 3.63–3.93 (1H, m, H-4), 3.98 (1H, dd, J 7.5, 2.1, CHCHO), 4.14 (2H, q, J 7.1, CH2CH3), 9.74 (1H, d, J 2.1, CHO). MS, m/z: 215 ([M − 15]+, 32%), 201 (13), 185 (30), 143 (70), 127 (33), 115 (100), 99 (26), 85 (50), 59 (23), 43 (70).
(4R,5R)-4,5-(Isopropylidenedioxy)hept-6-enoic acid ethyl ester (19). Methyltriphenylphosphonium bromide (1.837 g, 5.14 mmol) was slurried in 45 mL freshly distilled anhydrous THF. The white suspension was cooled to −23 °C and n-butyllithium (1.2 mL of a 2.5 M solution in hexanes) was added over 2 min under Ar flow and magnetic stirring. The bright yellow mixture was vigorously stirred and allowed to warm to rt for 1 h 40 min; gas bubbles were observed to develop and a white solid to settle. The crude aldehyde 18
(438 mg, 1.90 mmol) was dissolved in dry THF (5 mL) and added dropwise over a 3 min period to the yellow slurry at −20 °C and stirred for 30 min at the same temperature. After 2 h at rt, the orange-yellow cloudy mixture was poured in 200 mL diethyl ether and stirred for 5 min; the white suspension thus formed was passed through a short Celite pad and washed with abundant ether to obtain a clear colourless solution. Concentration in vacuo provided a crude residue which was purified by silica gel chromatography (light petroleum–diethyl ether 70 : 30 and finally 60 : 40) to afford the title alkene 19
(181 mg, 42%) as a yellow liquid, [α]D
+0.7 (c 0.8). [lit.,25
(4S,5S) 0]. 1H NMR (200 MHz): δ 1.26 (3H, t, J 7.1, CH2CH3), 1.41 (6H, s, 2 ×
Me2C), 1.73–2.06 (2H, m, 2 ×
H-3), 2.33–2.61 (2H, m, 2 ×
H-2), 3.70 (1H, dt, J 8.2, 3.9, H-4), 4.01 (1H, ddt, J 8.2, 7.2, 0.8, H-5), 4.14 (2H, q, J 7.1, CH2CH3), 5.27 (1H, ddd, J 10.1, 1.5, 0.8, CH
CH2), 5.38 (1H, ddd, J 17.1, 1.5, 0.8, CH
CH2), 5.81 (1H, ddd, J 17.1, 10.1, 7.2, CH
CH2). 13C NMR: δ 15.6, 28.2, 28.3, 28.6, 32.0, 61.8, 80.9, 83.8, 110.2, 120.6, 136.4, 174.5. MS, m/z: 213 ([M − 15]+, 21%), 195 (0.6), 171 (9.9), 125 (100), 115 (5.5), 98 (66), 83 (34), 69 (32), 55 (21), 43 (61). (Found: C, 63.2; H, 8.7. C12H20O4 requires: C, 63.1; H, 8.8%). (4R,5R)-4,5-(Isopropylidenedioxy)hept-6-enoic acid (20). The ester 19
(166 mg, 0.728 mmol) was dissolved in 8 mL THF–MeOH (1 : 1) and treated with aqueous KOH (248 mg in 2 mL) at rt under magnetic stirring. After 2.5 h TLC (diethyl ether–light petroleum 80 : 20) showed disappearance of the starting material. The pale yellow reaction mixture was poured in a separating funnel with water and diethyl ether (80 mL each), and the pH was adjusted to 4–5 with 10% HCl. The aqueous layer was further extracted with ether (3 × 20 mL) and the pooled organic phases were washed with water (2 × 100 mL) and brine (100 mL), dried over Na2SO4 and filtered. Finally, rotary evaporation afforded the desired free acid 20 as a sticky greenish oil (118 mg, 81%), which was used as such for the next DCC-mediated coupling step. 1H NMR (200 MHz): δ 1.41 (6H, s, 2 ×
Me2C), 1.70–2.06 (2H, m, 2 ×
H-3), 2.39–2.68 (2H, m, 2 ×
H-2), 3.72 (1H, dt, J 8.1, 3.9, H-4), 4.02 (1H, dd, J 8.1, 7.3, H-5), 5.27 (1H, ddd, J 10.2, 1.5, 0.8, CH
CH2), 5.38 (1H, ddd, J 17.2, 1.5, 0.9, CH
CH2), 5.81 (1H, ddd, J 17.2, 10.2, 7.3, CH
CH2). 13C NMR: δ 26.5, 26.9, 27.1, 30.3, 79.4, 82.4, 108.9, 119.1, 135.0, 178.7. MS, m/z: 200 (M+, 0.1%), 185 (64), 167 (1.4), 144 (5.1), 125 (100), 98 (93), 83 (57), 69 (47), 55 (28), 43 (84). (Found: C, 60.2; H, 7.8. C10H16O4 requires: C, 60.0; H, 8.0%). DCC-mediated coupling and final steps of the total synthesis
(4R,5R)-4,5-(Isopropylidenedioxy)hept-6-enoic acid, (1′S,1″S)-1′-(1″-benzyloxyheptyl)-3′-butenyl ester (21). A solution of acid 20
(118 mg, 0.590 mmol) in 1.5 mL anhydrous ether was added dropwise to alcohol 9
(165 mg, 0.598 mmol) in dry ether (2 mL), and DCC (122 mg, 0.590 mmol) was added at rt, along with DMAP (7 mg, 0.057 mmol). After stirring for 5.5 h, the white precipitate was filtered off and the yellow clear solution evaporated to dryness. The turbid yellow oil was chromatographed on silica gel with light petroleum–diethyl ether 95 : 5 to afford ester 21 as a liquid (205 mg, 85%), [α]D
−1.9 (c 4.2), along with 19 mg of unreacted 9. 1H NMR (200 MHz): δ 0.89 (3H, t, J 6.5, 3 ×
H-7″), 1.26 (8H, br m, H-3″ to H-6″), 1.39 (3H, s, Me2C), 1.41 (3H, s, Me2C), 1.44–1.62 (2H, m, 2 ×
H-2″), 1.72–2.07 (2H, m, 2 ×
H-3), 2.17–2.62 (4H, m, 2 ×
H-2 and 2 ×
H-2′), 3.45 (1H, dt, J 7.1, 4.5, H-1″), 3.69 (1H, dt, J 8.1, 3.9, H-4), 4.00 (1H, dd, J 8.1, 7.2, H-5), 4.60 (2H, s, CH2Ph), 4.97–5.18 (3H, m, H-1′ and 2 ×
H-4′), 5.25 (1H, ddd, J 10.2, 1.5, 0.8, H-7), 5.38 (1H, ddd, J 17.1, 1.5, 0.9, H-7), 5.63–5.79 (1H, m, H-3′), 5.80 (1H, ddd, J 17.1, 10.2, 7.2, H-6), 7.23–7.40 (5H, m, arom). 13C NMR: δ 14.0, 22.5, 25.5, 26.9, 27.2, 29.3, 29.9, 30.8, 31.7, 34.4, 72.4, 73.5, 79.0, 82.4, 108.8, 117.5, 118.9, 127.6, 127.8, 128.3, 134.1, 135.1, 138.5, 172.6. MS, m/z: 458 (M+, 0.4%), 443 (0.8), 361 (0.8), 294 (11), 275 (3.5), 259 (5.6), 223 (3.8), 205 (11), 183 (6.8), 143 (9.7), 125 (70), 98 (35), 91 (100), 83 (10), 69 (9.2), 55 (9.0), 43 (12). (Found: C, 73.4; H, 9.1. C28H42O5 requires: C, 73.3; H, 9.2%).
(5R,6R,7E,10S)- and (5R,6R,7Z,10S)-10-[(1′S)-1′-Benzyloxyheptyl]-5,6-isopropylidenedioxy-3,4,5,6,9,10-hexahydro-2H-oxecin-2-one, (E-22 and Z-22). Ester 21
(50 mg, 0.109 mmol) was dissolved in freshly distilled degassed anhydrous CH2Cl2
(230 mL) and Grubbs' catalyst I was added (15.5 mg, 0.0188 mmol). The mixture was refluxed for 2 days under Ar flow until TLC (light petroleum–diethyl ether 80 : 20) showed complete disappearance of the starting material. Most of the solvent was then distilled off and the concentrated solution left to stir at rt for 2 h under air bubbling in order to decompose the catalyst. Evaporation to dryness gave a brown residue which was purified by chromatography on silica gel; slow elution with light petroleum–diethyl ether mixtures (from 97 : 3 to 80 : 20) allowed separation of the desired trans stereoisomer (E)-22
(29 mg) from cis derivative (Z)-22
(14 mg)
(combined 92% yield).
(5R,6R,7E,10S)-22. [α]D
−37.9 (c 3.0). 1H NMR (200 MHz): δ 0.90 (3H, t, J 6.5, 3 ×
H-7′), 1.27 (8H, br envelope, H-3′ to H-6′), 1.41 (6H, s, 2 ×
Me2C), 1.51–1.80 (2H, m, 2 ×
H-2′), 1.87–2.71 (6H, m, 2 ×
H-3, H-4 and H-9), 3.47 (1H, m, H-1′), 3.64 (1H, m, H-5), 3.92 (1H, t, J 9.2, H-6), 4.56 (1H, d, J 11.6, CH2Ph), 4.65 (1H, d, J 11.6, CH2Ph), 4.95 (1H, m, H-10), 5.32 (1H, dd, J 15.6, 9.2, H-7), 5.78 (1H, ddd, J 15.6, 11.0, 4.7, H-8), 7.29–7.40 (5H, m, arom). 13C NMR: δ 14.0, 22.5, 25.4, 25.6, 26.9, 27.1, 29.4, 29.7, 31.7, 34.2, 72.5, 73.4, 79.8, 80.4, 84.4, 108.8, 127.8, 127.9, 128.4, 129.2, 130.3, 138.2, 171.8. MS, m/z: 430 (M+, 0.22%), 415 (0.33), 373 (0.60), 328 (1.1), 298 (0.71), 237 (3.3), 220 (1.8), 205 (4.3), 203 (7.0), 179 (1.8), 123 (6.2), 113 (14), 91 (100), 85 (23), 79 (6.2), 65 (3.1), 55 (5.5). (Found: C, 72.4; H, 9.0. C26H38O5 requires: C, 72.5; H, 8.9%).
(5R,6R,7Z,10S)-22. [α]D
+4.5 (c 1.6). 1H NMR (200 MHz): δ 0.89 (3H, t, J 6.5, 3 ×
H-7′), 1.27 (8H, br envelope, H-3′ to H-6′), 1.40 (3H, s, Me2C), 1.42 (3H, s, Me2C), 1.50–1.77 (2H, m, 2 ×
H-2′), 2.01–2.77 (6H, m, 2 ×
H-3, H-4 and H-9), 3.49 (1H, m, H-1′), 3.66 (1H, ddd, J 10.2, 9.5, 2.3, H-5), 4.52 (1H, dd, J 9.5, 8.0, H-6), 4.58 (1H, d, J 11.6, CH2Ph), 4.66 (1H, d, J 11.6, CH2Ph), 5.10 (1H, ddd, J 11.8, 4.4, 2.2, H-10), 5.50 (1H, t, J 10.3, H-7), 5.74 (1H, dt, J 10.3, 7.0, H-8), 7.28–7.44 (5H, m, arom). 13C NMR: δ 14.0, 22.5, 25.4, 26.8, 27.0, 29.3, 29.6, 30.5, 31.7, 32.1, 72.6, 72.8, 77.1, 79.7, 81.5, 107.6, 127.7, 128.4, 130.3, 130.9, 138.0, 176.6. MS, m/z: 430 (M+, 0.69%), 415 (2.3), 373 (0.30), 328 (1.1), 298 (0.60), 265 (1.7), 237 (3.0), 220 (1.7), 205 (3.9), 203 (6.3), 179 (2.5), 123 (5.1), 113 (13), 91 (100), 85 (16), 79 (6.6), 65 (3.1), 55 (6.6). (Found: C, 72.7; H, 9.1. C26H38O5 requires: C, 72.5; H, 8.9%).
(5R,6R,7E,10S)-5,6-Dihydroxy-10-[(1′S)-1′-hydroxyheptyl]-3,4,5,6,9,10-hexahydro-2H-oxecin-2-one (microcarpalide, 1)3. Titanium tetrachloride (66 µL, 0.605 mmol) in anhydrous CH2Cl2
(0.5 mL) was slowly added dropwise over 10 min to a stirred solution of trans derivative E-22
(26 mg, 0.0605 mmol) in dry CH2Cl2
(2.5 mL) cooled to 0 °C. After 1.5 h the ochre-yellow cloudy mixture was poured in water (5 mL), diluted with CH2Cl2
(4 mL) and treated with satd. NaHCO3
(8 mL), brine (5 mL) and EtOAc (15 mL) in a separating funnel. After settling, the upper milky layer was discarded, whereas the clear lower phase was separated, dried (Na2SO4), filtered and concentrated under reduced pressure; silica gel chromatography of the crude residue using ethyl acetate as the eluant afforded microcarpalide 1 as a beige oil (12 mg, 66%), [α]D
−23.6 (c 1.0, MeOH)
(lit.,3
−22). NMR analysis clearly showed the presence of two slowly interconverting conformers in a 76 : 24 ratio (in CD3CN), which is identical to the value described in the literature for the natural compound in the same solvent.31H NMR (400 MHz, CD3CN; referenced to the residual proton at 1.96 ppm): δ 0.91 (3H, t, J 6.8, 3 ×
H-7′), 1.26–1.38 (8H, br envelope, H-3′ to H-6′), 1.41–1.47 (2H, br m, 2 ×
H-2′), 1.80 (1H, br dddd, H-4), 2.02 (1H, br ddd, H-4 minor conformer), 2.11–2.23 (3H, br m, H-3, H-4 and H-9), 2.27–2.34 (1H, br m, H-9), 2.36 (1H, ddd, J 5.2, 2.7, 1.1, H-9 minor), 2.47–2.58 (1H, m, H-3), 2.83 (1H, d, J 5.8, 1′-OH), 2.86 (1H, d, J 6.4, 6-OH), 3.09 (1H, d, J 4.1, 5-OH), 3.19 (1H, d, J 3.2, 5-OH minor), 3.28 (1H, br dt, H-5 minor), 3.54–3.60 (1H, br m, H-1′), 3.64 (1H, dt, J 3.1, 9.1, H-6 minor), 3.80 (1H, br m, H-5), 4.13 (1H, br m, H-6), 4.63 (1H, ddd, J 8.4, 4.5, 2.7, H-10 minor), 4.84 (1H, ddd, J 11.3, 4.9, 3.3, H-10), 5.08 (1H, dd, J 15.7, 9.4, H-7 minor), 5.53 (1H, dddd, J 15.8, 10.3, 5.3, 2.2, H-8), 5.69 (1H, m, H-8 minor), 5.73 (1H, dd, J 15.8, 2.5, H-7). 13C NMR (100 MHz, CD3CN; referenced to CD3CN at 118.26 ppm): δ 14.4 (C-7′), 23.3 (C-6′), 26.1 (C-3′), 26.5 (C-4), 29.3 (C-3), 30.0 (C-4′), 32.2 (C-9, minor conformer), 32.3 (C-5′, minor), 32.6 (C-5′), 33.9 (C-2′, minor), 34.3 (C-2′), 35.9 (C-3, minor), 36.7 (C-9), 72.5 (C-6), 72.9 (C-1′), 73.5 (C-5), 73.8 (C-1′, minor), 76.4 (C-10, minor), 77.0 (C-5, minor), 79.5 (C-6, minor), 79.7 (C-10), 126.7 (C-8), 130.0 (C-8, minor), 133.8 (C-7, minor), 134.6 (C-7), 173.5 (C-2, minor), 176.4 (C-2)
[see also electronic supporting information (ESI) for original 1H and 13C NMR spectra of synthetic 1]. MS, m/z: 301 ([M + 1]+, 1.4%), 283 (0.55), 265 (0.78), 198 (6.2), 180 (55), 162 (1.2), 151 (3.5), 141 (10), 129 (30), 113 (13), 110 (16), 95 (39), 84 (100), 73 (44), 70 (80), 55 (64). (Found: C, 63.9; H, 9.2. C16H28O5 requires: C, 64.0; H, 9.4%). Acknowledgements
We are grateful to the Università di Modena e Reggio Emilia (“Progetto Giovani Ricercatori” to P.D.) for financial support. Spectroscopic assistance from the staff at Centro Interdipartimentale Grandi Strumenti (Università di Modena) has been greatly appreciated throughout the project. We thank Stefania Morandi and Chiara Danieli for critically examining NMR assignments of pinanediol boronates. We are also grateful to Dr Claudia Zucchi for kindly providing Fürstner's catalyst III.References
-
(a) J. Gusman and M. Vanhaelen, Recent Res. Dev. Phytochem., 2000, 4, 187–206 Search PubMed;
(b) R.-X. Tan and W.-H. Zou, Nat. Prod. Rep., 2001, 18, 448–459 RSC;
(c) B. Schulz, C. Boyle, S. Draeger, A. K. Rommert and K. Krohn, Mycol. Res., 2002, 106, 996–1004 Search PubMed.
- For recent examples:
(a) D. B. Stierle, A. A. Stierle and T. Bugni, J. Org. Chem., 2003, 68, 4966–4969 CrossRef CAS;
(b) G. Y. Chen, Y. C. Lin, L. Wen, L. L. P. Vrijmoed and E. B. G. Jones, Tetrahedron, 2003, 59, 4907–4909 CrossRef CAS;
(c) J. A. Findlay, G. Q. Li, J. D. Miller and T. O. Womiloju, Can. J. Chem., 2003, 81, 284–292 CrossRef CAS;
(d) A. Abdel-Lateff, C. Klemke, G. M. König and A. D. Wright, J. Nat. Prod., 2003, 66, 706–708 CrossRef CAS;
(e) P. Kongsaeree, S. Prabpai, N. Sriubolmas, C. Vongvein and S. Wiyakrutta, J. Nat. Prod., 2003, 66, 709–711 CrossRef CAS;
(f) B. Köpke, R. W. S. Weber and H. Anke, Phytochemistry, 2002, 60, 709–714 CrossRef;
(g) G. Strobel, E. Ford, J. Worapong, J. K. Harper, A. M. Arif, D. M. Grant, P. C. W. Fung and R. M. W. Chau, Phytochemistry, 2002, 60, 179–183 CrossRef CAS;
(h) S. F. Brady, S. M. Bondi and J. Clardy, J. Am. Chem. Soc., 2001, 123, 9900–9901 CrossRef CAS;
(i) J. Y. Li, J. K. Harper, D. M. Grant, B. O. Tombe, B. Bashyal, W. M. Hess and G. A. Strobel, Phytochemistry, 2001, 56, 463–468 CrossRef CAS.
- A. S. Ratnayake, W. Y. Yoshida, S. L. Mooberry and T. Hemscheidt, Org. Lett., 2001, 3, 3479–3481 CrossRef CAS.
- B. Bodo, L. Molho, D. Davoust and D. Molho, Phytochemistry, 1983, 22, 447–451 CrossRef CAS.
-
(a) A. Evidente, R. Lanzetta, R. Capasso, M. Vurro and A. Bottalico, Phytochemistry, 1993, 34, 999–1003 CrossRef CAS;
(b) A. Evidente, R. Capasso, M. A. Abouzeid, R. Lanzetta, M. Vurro and A. Bottalico, J. Nat. Prod., 1993, 56, 1937–1943 CrossRef CAS.
- A. Arnone, G. Assante, M. Montorsi, G. Nasini and E. Ragg, Gazz. Chim. Ital., 1993, 123, 71–73 CAS.
-
(a) A. Evidente, R. Lanzetta, R. Capasso, A. Andolfi, A. Bottalico, M. Vurro and M. C. Zonno, Phytochemistry, 1995, 40, 1637–1641 CrossRef CAS;
(b) A. Evidente, R. Lanzetta, R. Capasso, A. Andolfi, M. Vurro and M. C. Zonno, Phytochemistry, 1997, 44, 1041–1045 CrossRef CAS;
(c) A. Evidente, R. Capasso, A. Andolfi, M. Vurro and M. C. Zonno, Phytochemistry, 1998, 48, 941–945 CrossRef CAS.
-
(a) J. F. Rivero-Cruz, G. García-Aguirre, C. M. Cerda-García-Rojas and R. Mata, Tetrahedron, 2000, 56, 5337–5344 CrossRef;
(b) J. F. Rivero-Cruz, M. Macías, C. M. Cerda-García-Rojas and R. Mata, J. Nat. Prod., 2003, 66, 511–514 CrossRef CAS.
- Reviews:
(a) T. M. Trnka and R. H. Grubbs, Acc. Chem. Res., 2001, 34, 18–29 CrossRef CAS;
(b) A. Fürstner, Angew. Chem., Int. Ed., 2000, 39, 3012–3043 CrossRef CAS;
(c) R. H. Grubbs and S. Chang, Tetrahedron, 1998, 54, 4413–4450 CrossRef CAS.
-
(a) D. S. Matteson, Acc. Chem. Res., 1988, 21, 294–300 CrossRef CAS;
(b) D. S. Matteson, Chem. Rev., 1989, 89, 1535–1551 CrossRef CAS;
(c) D. S. Matteson, J. Organomet. Chem., 1999, 581, 51–65 CrossRef CAS.
- D. S. Matteson, K. M. Sadhu and M. L. Peterson, J. Am. Chem. Soc., 1986, 108, 810–819 CrossRef CAS.
- H. R. Snyder, J. A. Kuck and J. R. Johnson, J. Am. Chem. Soc., 1938, 60, 105–111 CrossRef CAS.
-
(a) D. S. Matteson and D. Majumdar, J. Am. Chem. Soc., 1980, 102, 7588–7590 CrossRef CAS;
(b) D. S. Matteson and D. Majumdar, Organometallics, 1983, 2, 1529–1535 CrossRef CAS.
-
(a) M. M. Midland, A. R. Zolopa and R. L. Halterman, J. Am. Chem. Soc., 1979, 101, 248–249 CrossRef CAS;
(b) D. S. Matteson, Acc. Chem. Res., 1970, 3, 186–193 CrossRef CAS.
-
(a) D. S. Matteson and R. Ray, J. Am. Chem. Soc., 1980, 102, 7590–7591 CrossRef CAS;
(b) D. S. Matteson, R. Ray, R. R. Rocks and D. J. Tsai, Organometallics, 1983, 2, 1536–1543 CrossRef CAS.
-
(a) H. C. Brown, in Boranes in Organic Chemistry, Cornell University Press, Ithaca, NY, 1972, pp. 317–409 Search PubMed;
(b) G. W. Kabalka, R. J. Newton, Jr. and J. Jacobus, J. Org. Chem., 1978, 43, 1567–1569 CrossRef CAS.
- M. Carmack and C. H. Kelley, J. Org. Chem., 1968, 33, 2171–2173 CrossRef CAS.
- P. W. Feit, J. Med. Chem., 1964, 7, 14–17 CrossRef CAS.
- J. Taunton, J. L. Collins and S. L. Schreiber, J. Am. Chem. Soc., 1996, 118, 10412–10422 CrossRef CAS.
- H. Iida, N. Imazaki and C. Kibayashi, J. Org. Chem., 1987, 52, 3337–3342 CrossRef CAS.
- S. Clough, M. E. Raggatt, T. J. Simpson, C. L. Willis, A. Whiting and S. K. Wrigley, J. Chem. Soc., Perkin Trans. 1, 2000, 2475–2481 RSC.
- P. Schnurrenberger, E. Hungerbühler and D. Seebach, Liebigs Ann. Chem., 1987, 733–744 Search PubMed.
- During a preliminary approach to fragment B, diol 12 was treated with NaH and benzyl bromide in DMF at −40 °C to afford the corresponding monobenzyl derivative.21 In fact, a benzyl protection seemed convenient since it could have been removed en route, during the hydrogenation step that was planned to follow the first Wittig homologation. Surprisingly and unexpectedly, however, cleavage of the benzyl group by catalytic hydrogenation was found rather troublesome, even under higher pressures. Hence, annoyingly, the problem was circumvented by switching to a different protecting group for diol 12, namely TBDMS, and the synthetic sequence had to be lengthened by one step, i.e., deprotection with TBAF.
- E. J. Corey and A. Venkateswarlu, J. Am. Chem. Soc., 1972, 94, 6190–6191 CrossRef CAS.
-
(a) D. Batty and D. J. Crich, J. Chem. Soc., Perkin Trans. 1, 1992, 3193–3204 RSC;
(b) D. Batty and D. J. Crich, Tetrahedron Lett., 1992, 33, 875–878 CrossRef CAS.
- J. Murga, E. Falomir, J. García-Fortanet, M. Carda and J. A. Marco, Org. Lett., 2002, 4, 3447–3449 CrossRef CAS.
- A. Fürstner, K. Radkowski, C. Wirtz, R. Goddard, C. W. Lehmann and R. Mynott, J. Am. Chem. Soc., 2002, 124, 7061–7069 CrossRef.
- D. Liu and S. A. Kozmin, Org. Lett., 2002, 4, 3005–3007 CrossRef CAS.
- A. Fürstner, O. Guth, A. Düffels, G. Seidel, M. Liebl, B. Gabor and R. Mynott, Chem.-Eur. J., 2001, 7, 4811–4820 CrossRef CAS.
- M. K. Gurjar, R. Nagaprasad and C. V. Ramana, Tetrahedron Lett., 2003, 44, 2873–2875 CrossRef CAS.
- E. J. Corey, J. L. Gras and P. Ulrich, Tetrahedron Lett., 1976, 11, 809–812 CrossRef.
- M. F. Lappert, Chem. Rev., 1956, 56, 959–1064 CrossRef CAS.
- H. C. Brown, N. G. Bhat and V. Somayaji, Organometallics, 1983, 2, 1311–1316 CrossRef CAS.
- L. J. Rubin, H. A. Lardy and H. O. L. Fischer, J. Am. Chem. Soc., 1952, 74, 425–428 CrossRef CAS.
|
This journal is © The Royal Society of Chemistry 2004 |
Click here to see how this site uses Cookies. View our privacy policy here.