DOI:
10.1039/B401867M
(Paper)
New J. Chem., 2004,
28, 912-918
2,5-Di(aryleneethynyl)pyrazine derivatives: synthesis, structural and optoelectronic properties, and light-emitting device†
Received
(in Montpellier, France)
5th February 2004
, Accepted 18th March 2004
First published on 6th July 2004
Abstract
A series of 2,5-di(aryleneethynyl)pyrazine derivatives has been synthesised in 23–41% yields by two-fold reaction of 2,5-dibromo-3,6-dimethylpyrazine 3 with ethynylarenes (arene
=
phenyl, 2-pyridyl, 4-ethylphenyl, 4-chlorophenyl, 4-biphenyl) under standard Sonogashira conditions [CuI, Pd(PPh3)2Cl2, NEt3, THF]. Compound 3 has been converted into 2,5-diethynyl-3,6-dimethylpyrazine, which reacts with 2-iodothiophene to yield 2,5-bis(thien-2-ylethynyl)-3,6-dimethylpyrazine. In the X-ray crystal structure of 2,5-di(phenylethynyl)-3,6-dimethylpyrazine 4 the two phenyl rings are parallel and the pyrazine ring is inclined to their planes by 14.2°. Quantum chemical calculations establish that the HOMO–LUMO gap for 4
(3.56 eV) is lower than that of di(phenylethynyl)benzene 12
(3.72 eV). The nitrogen atoms of 4 serve to localise the HOMO on the central ring’s carbon atoms, resulting in a quinoidal-type population, in contrast to 12. Cyclic voltammetric studies establish that 4 undergoes a reduction to the radical anion at ca.
−1.9 V (vs. Ag/Ag+ in MeCN), which is almost reversible at high scan rates (500 mV s−1). The UV-vis absorption and photoluminescence profiles of 4 in cyclohexane are similar to those of 12; the emission for 4
(λmax 379 and 395 nm) is red-shifted compared to 12. Single-layer OLEDs using MEH-PPV as the emissive polymer show significantly enhanced external quantum efficiencies (up to 0.07%) when 20% by weight of 2,5-di(biphenyl-4-ethynyl)-3,6-dimethylpyrazine 8 is added as a dopant: this is ascribed to the enhanced electron-transporting properties of the pyrazine system.
Introduction
There is widespread interest in the synthesis and optoelectronic properties of new conjugated oligoarylenes containing electron-deficient heterocycles in the backbone or as pendant groups.1 1,3,4-Oxadiazole derivatives have been prominent in this respect2 and it has also been established that pyridine,3 pyrimidine,4 quinoline5 and 1,3,5-triazine6 units improve electron transport characteristics compared to their phenylene analogues. This has led to their use as new materials with tailored photoluminescence and electroluminescence properties, for example as the emitting layer or as an electron-transport, hole-blocking (ETHB) layer in organic light emitting devices (OLEDs).7 Pyrazine derivatives have been somewhat neglected in this context, although the symmetry of 2,5-disubstituted pyrazines has obvious attractions. Orange-red luminescence has been reported from thin films of polyimides containing distyrylypyrazine units in the backbone.8 Electron injection is significantly improved in OLEDs made with a PPV–copolymer (PPV
=
p-phenylenevinylene) that contains pyrazine units in the backbone,9 and we have shown that p-phenylene-2,5-dimethylpyrazine co-oligomers are efficient blue emitters in photoluminescence and electroluminescence studies.10
Herein we describe our work on the synthesis, structure determination and optoelectronic properties of novel 2,5-di(aryleneethynyl)pyrazines. We targeted these molecules because there is considerable current interest in the structural, optoelectronic and photophysical properties of highly conjugated oligoaryleneethynylenes.11 For example, 1,4-bis(phenylethynyl)benzene has been thoroughly studied12 as a model for poly(phenyleneethynylene) derivatives that are known emitters in OLEDs.13 Oligo(9,9-dihexyl-2,7-fluoreneethynylene)s have also been used as the emitting layer in blue OLEDs.14 Hitherto 2,5-diethynylpyrazine units have not been studied.
Results and discussion
Synthesis
Although 2,5-dibromopyrazine is known,15 in our hands the literature synthesis (namely, a Sandmeyer reaction on 2-amino-5-bromopyrazine)15a did not routinely provide sufficient material for further reactions. We, therefore, used 2,5-dibromo-3,6-dimethylpyrazine 3 as our starting reagent. An outline synthesis has been reported for this compound, although details are lacking.16 The reaction of alanine anhydride 1 with a mixture of phosphoryl chloride and phosphorus pentachloride at 105
°C is reported to give 2,5-dichloro-3,6-dimethylpyrazine 2 in 12% yield.17 With minor modifications to this procedure we obtained 2 in 31% yield. Conversion of compound 2 into the dibromo analogue 3 using phosphorus tribromide at 170
°C proceeded in 35% yield. In a typical reaction we obtained ca. 3 g of analytically pure compound 3, and this appears to be the optimum scale for this reaction: larger-scale reactions resulted in incomplete conversion to 3. Two-fold Sonogashira reactions18 on 3 using the appropriate ethynylarene derivative under standard conditions [copper iodide, triethylamine, Pd(PPh3)2Cl2 in refluxing THF] gave products 4–8 in 23–41% yields (Scheme 1). The analogous reaction of 3 with 2-methyl-3-butyn-2-ol gave product 9, which was deprotected under standard basic conditions19 with loss of acetone to afford 10
(76% yield for the two steps from 3). As an alternative route to 2,5-di(aryleneethynyl)pyrazine derivatives, we explored the Sonogashira reactions of compound 10 with iodobenzene and 2-iodothiophene. The reactions were not clean and products 4 and 11 were isolated in ca. 20% yields. (2-Bromothiophene gave 11 in only 10% yield). It is preferable, therefore, to use compound 3 rather than compound 10 as the pyrazine reagent in these syntheses.
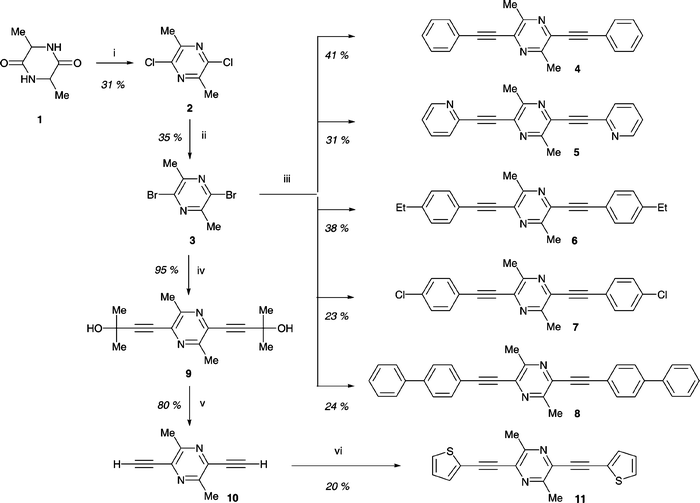 |
| Scheme 1 Synthesis of 2,5-(diaryleneethynyl)pyrazines. Reagents and conditions: (i) POCl3/PCl5, 105 °C, 24 h; (ii) PBr3, 170 °C, 24 h; (iii) ArC CH, CuI, Et3N, Pd(PPh3)2Cl2, THF, 20 °C/1 h, 65 °C/1.5 h; (iv) Me2C(OH)–C CH, CuI, Et3N, THF, 20 °C, 1 h, reflux; (v) NaOH, toluene, reflux; (vi) 2-iodothiophene, CuI, Et3N, Pd(PPh3)2Cl2, THF, 20 °C/1 h, 65 °C/1.5 h. | |
X-Ray crystal structure of 4
The molecule of 4
(Fig. 1) is located at a crystallographic inversion centre. Thus, the two phenyl rings are parallel with the pyrazine ring inclined to their planes by 14.2°. Molecules in the crystal form a layer of a brickwork pattern (Fig. 1) parallel to the (0 0 1) plane. The long axes of all molecules belonging to the same layer are strictly parallel, while those in the adjacent layers are inclined to the latter by ca. 45°. Packing within the layer is not particularly close due to the non-planar conformation of the molecule. The separation between the mean planes of contacting molecules (3.49 Å) and the shortest intramolecular C⋯C distances (3.46–3.48 Å) correspond to normal Van der Waals' contacts. There is partial overlap between the phenyl ring of one molecule and the pyrazine ring of another.
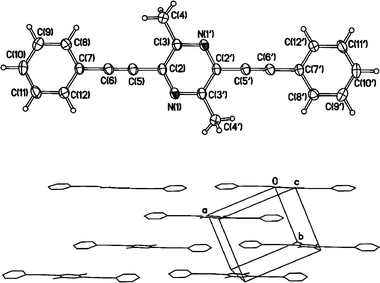 |
| Fig. 1 (top) Molecular structure of 4. Thermal ellipsoids are drawn at 50% probability level. Atoms generated by the inversion centre are primed. (bottom) Layer of molecules in the crystal structure; projection on the (0 0 1) plane. | |
Quantum chemical calculations
We performed quantum mechanical calculations of the geometry and electronic structure of compounds 4–8 and 11 by DFT methods at the B3LYP/6-31G(d) level of theory, and for compound 4 also at the B3LYP/6-311G(2d,p) level (for optimised geometries see Tables S1–S9 in the Electronic supplementary information). For reference the known phenylene analogue 1212
(Chart 1) was calculated at the same levels and the results of B3LYP/6-311G(2d,p) calculations for 4 and 12 were compared with X-ray diffraction single crystal structures for these compounds.20 The calculated structures of both 4 and 12 are essentially flat and all three aromatic rings lie in the plane. There is generally a good agreement between the calculated and the X-ray experimental bond distances: mean discrepancies are 0.006 and 0.007 Å, respectively (Fig. 2). The calculated triple bond lengths are somewhat longer (by 0.003–0.008 Å) and the single bonds (between the aromatic rings and sp-hybrid carbon atoms) are somewhat shorter (by 0.008–0.012 Å) than the experimental data, but these are expected differences because the calculated values are inter-nuclear distances whereas X-ray gives interatomic distances based on mean electronic density, which for the fragment
C– is shifted towards the triple bond.
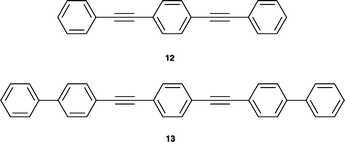 |
| Chart 1 | |
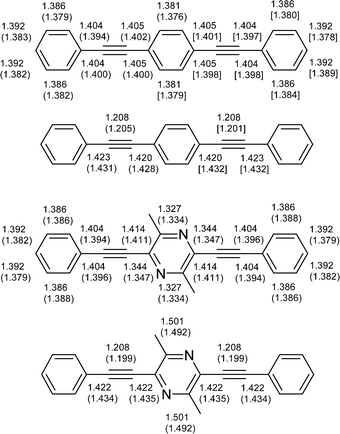 |
| Fig. 2 B3LYP/6-311G(2d,p) calculated bond distances in phenylene 12 and dimethylpyrazine 4 derivatives. Bond distances from X-ray analysis are given in parentheses for comparison (distances for the two independent molecules from the X-ray crystal structure of phenylene derivative 12 are given in parentheses and square brackets, respectively). The structures at the top show bond distances in the rings and while those at the bottom show acyclic bond distances. | |
Orbital energy level analysis for compounds 4–8, 11 and 12 at the B3LYP/6-31G(d) level is summarised in Table 1, indicating that all pyrazine derivatives are stronger electron acceptors than compound 12
(their LUMO energies are lower than that in 12 by 0.26–0.60 eV). Although their HOMO energy levels are higher than that in 12, in total all pyrazine derivatives show a narrower HOMO–LUMO gap as compared to 12.
Calculations performed for compounds 4 and 12 at the higher B3LYP/6-311G(2d,p) level for more precise estimation of orbital energies showed ca. 0.21–0.26 eV lower energies as compared to B3LYP/6-31G(d) calculations, although the general trend remains the same and HOMO–LUMO gap energies calculated by both methods are very close (3.53 and 3.56 eV for 4, 3.70 and 3.72 eV for 12, respectively). For pyrazine derivative 4
(as compared to 12) the energies of both HOMO and LUMO orbitals decrease (by 0.17 and 0.33 eV, respectively) with, as expected, more pronounced changes in the LUMO energy due to the electronegative nitrogen atoms (Fig. 3). As a result the HOMO–LUMO gap contracts by 0.16 eV, from 3.72 eV for 12 to 3.56 eV for 4. For compound 12 the HOMO is located on the C–C triple bonds as well as on the central 1,4-phenylene ring, with only minor population on the outer phenyl moieties. The main population at the central ring is on C–1 and C–4, which form bonding orbitals with adjacent carbons. In 4, the HOMO is also located on the triple C–C bonds and on the central ring. The electronegative nitrogen atoms in 4 result in localisation of the HOMO on the central ring's carbon atoms, giving a quinoidal-type population, in contrast to 12. The LUMO in 12 populates the exocyclic C–C single bond and C–2,3, C–5,6 bonds of the central phenylene ring, resulting in a quinoidal structure. Similar localisation of the LUMO on the exocyclic C–C single bonds is observed for 4, whereas for the pyrazine ring the LUMO is localised on the nitrogen atoms (Fig. 4).
Table 1 HOMO/LUMO energy levels for compounds 4–8, 11 and 12 from B3LYP/6-31G(d) calculations
Compound |
HOMO/eV |
LUMO/eV |
ΔEHOMO–LUMO/eV |
4
|
−5.62 |
−2.09 |
3.53 |
5
|
−5.86 |
−2.30 |
3.56 |
6
|
−5.48 |
−2.00 |
3.48 |
7
|
−5.81 |
−2.34 |
3.47 |
8
|
−5.48 |
−2.15 |
3.33 |
11
|
−5.47 |
−2.20 |
3.27 |
12
|
−5.43 |
−1.74 |
3.70 |
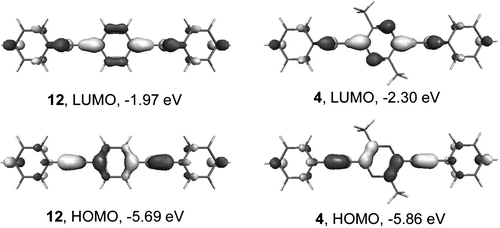 |
| Fig. 3 Frontier orbitals of compounds 12 and 4 calculated by B3LYP/6-311G(2d,p) DFT method. | |
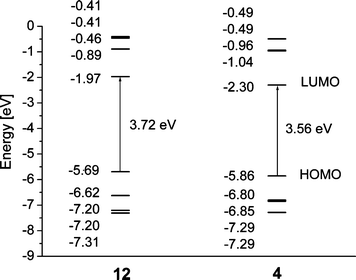 |
| Fig. 4 B3LYP/6-311G(2d,p) orbital energy level diagrams for phenylene 12 and dimethylpyrazine 4 derivatives. | |
The population of the unoccupied molecular orbitals LUMO
+
1 to LUMO
+
4 is similar for both molecules 4 and 12
(Fig. S1 in ESI): LUMO
+
1 is shifted to the outer phenyl rings, which become essentially quinoidal; LUMO
+
2 is localised on the central ring, forming antibonding orbitals between C2–C3, C5–C6 and N1–C6, C3–N4 for 12 and 4, respectively. Structures LUMO
+
3 and LUMO
+
4 for both compounds are nearly the same, with population on the terminal phenyl rings. For occupied HOMO
−
1 to HOMO
−
4 orbitals, the structures of HOMO
−
1 and HOMO
−
3 orbitals for both compounds are quite similar, whereas a large difference is observed for HOMO
−
2, which is quinoidally localised on the end phenyls in 12 and on the central pyrazine ring in 4, involving participation of methyl groups and the nitrogen lone pairs.
Solution electrochemical properties
The redox properties of compound 4, studied by cyclic voltammetry (CV) in dry deoxygenated acetonitrile solution, show an irreversible oxidation to the radical cation at +1.62 V vs. Ag/Ag+
(Fig. 5). Reduction of 4 to its radical anion occurs at quite high negative potentials of ca.
−1.87 V (vs. Ag/Ag+). At slow scan rates (20–50 mV s−1) the reduction is an irreversible process (not shown on Fig. 5). On increasing the scan rate to 100 mV s−1 it becomes partly reversible (ic
≫
ia) and, remarkably, at a high scan rate of 500 mV s−1 the process is almost reversible (ic
≈
ia; ΔEpa–pc
=
77 mV). The electrochemical band gap, estimated from the oxidation and reduction onsets (+1.45 V and −1.78 V) is ECVg
=
3.23 eV, which is in reasonable agreement with the optical band gap estimated from the red edge of the longest wavelength absorption (λ
=
382 nm, Eoptg
=
3.25 eV) and close to the calculated value of the HOMO–LUMO gap (3.56 eV). No clear reduction peak was observed in the CV of compound 12 within the acetonitrile solvent window, under the same experimental conditions, demonstrating the enhanced electron-accepting ability of compound 4, due to the presence of the pyrazine ring.
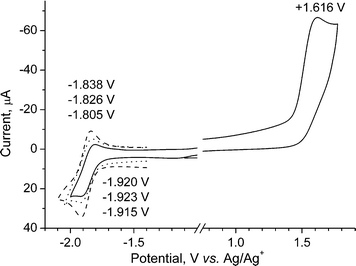 |
| Fig. 5 Cyclic voltammogram for derivative 4 in acetonitrile/0.1 M Bu4NPF6
(20 °C) at different scan rates: (—) 100 mV s−1 (E1/2red = −1.863 V; ΔEpa-pc = 115 mV); (⋯) 200 mV s−1 (E1/2red = −1.874 V; ΔEpa-pc = 97 mV); ( ) 500 mV s−1 (E1/2red = −1.876 V; ΔEpa–pc = 77 mV). | |
Absorption and photoluminescence studies on 4 and 6
The absorption and steady state fluorescence spectra in cyclohexane were recorded at ambient temperature for compounds 4 and 6
(Fig. 6 and S2 in ESI). The major absorption, emission and excitation maxima are given in Table 2, along with data for compound 12.12 The absorbances of these compounds were recorded between 230 and 400 nm and are characterised by two broad bands, with the longer wavelength bands at λmax 354 and 359 nm for 4 and 6, respectively, showing a similar profile to that observed for 12 at ambient temperature. It is believed that these spectral profiles represent the time-averaged spectra of the various rotamers present at these temperatures.12
Compound |
λ
abs/nm |
λ
PL/nm |
λ
ex/nm |
br – broad, sh – shoulder.
|
4
|
286 (br), 354 (br) |
379, 395 (br) |
283 (br), 357 (br) |
6
|
291, 359 (br), 376 (sh) |
384, 403, 418 |
287, 360, 374 (sh) |
12
|
320, 345 (sh) |
346, 362, 375 |
319, 340 (sh) |
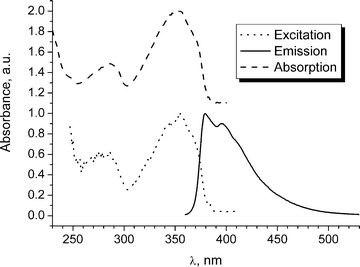 |
| Fig. 6 Normalised absorbance, excitation (for 432 nm emission wavelength) and emission (for 354 nm excitation wavelength) spectra of compound 4 in cyclohexane. | |
The emission spectra of 4 and 6 were independent of excitation wavelength. The fluorescence profiles and the excitation spectra coincide well with the corresponding absorption spectra. There is a small Stokes shift between the excitation and emission spectra. The emission spectra have some vibrational fine structure and resemble that of 12, although the maxima for 4 and 6 are red-shifted compared to 12. The difference in the excitation and emission profiles is attributed to differences in the geometric arrangement of the nuclei in the ground and excited states, resulting from the low barrier to rotation about the alkynyl–aryl single bond.11b,12 The vibrational structure in the emission spectra is indicative of an S1 state with a larger barrier to rotation from the lowest energy planar orientation of the rings. The ethyl substituents in 6 only slightly lower the S1 excited state.
Electroluminescent device studies
The potential of 2,5-di(aryleneethynyl)pyrazine derivatives 4, 5 and 8 to act as electron-transport materials in OLEDs has been explored by blending the compound as a dopant into thin films of poly[2-(2-ethylhexyloxy)-5-methoxy-1,4-phenylenevinylene]
(MEH-PPV), following the precedent set by Heeger's group.21 Analogous experiments using the phenylene analogues 12 and 13 (Chart 1) were performed for comparison. Preliminary data establish that the electroluminescence (EL) characteristics of single-layer devices in the configuration ITO/MEH-PPV + dopant/Al were unchanged compared to an MEH-PPV reference device (i.e., no added dopant) by addition of compounds 4, 5, 12 and 13. On the other hand, using compound 8 as the dopant led to a marked increase in the external quantum efficiency of the device (0.07% at a current density of 10 mA cm−3) compared to the reference device (ca. 2
×
10−3%) although more rapid deterioration in the EL output was observed for the MEH-PPV
+
8 layers. The EL spectra of all the device structures are very similar, which suggests that, as expected, emission is due to the MEH-PPV polymer [λELmax
=
575, 640 (sh) nm]. The non-planar biphenyl units of 8 probably serve to reduce crystallisation (and hence segregation) of the dopant in the blend. A comparison between 8 and 13 demonstrates the benefit of the electron-deficient pyrazine unit. We have previously reported similar levels of enhancement of the external quantum efficiencies of MEH-PPV devices doped with oxadiazole-pyridine hybrid compounds as the electron-transport material.22 Further studies on single blended-layer OLEDs will be published shortly.
Conclusions
2,5-Di(aryleneethynyl)pyrazine derivatives have been studied for the first time. In particular, the structural, electrochemical and photophysical properties of derivative 4 have been compared with those of di(phenylethynyl)benzene 12. The presence of the pyrazine ring leads to a significant enhancement of electron-accepting properties, as revealed by cyclic voltammetric data. Quantum chemical calculations confirm these observations. Preliminary experiments establish the potential for diethynylpyrazine derivative 8 to act as an electron-transporting material as a blend with MEH-PPV in single-layer OLEDs. The compounds reported herein are attractive for photophysical studies and compound 10 should be a very useful and versatile building block for the synthesis of new, more highly functionalised pyrazine derivatives, including conjugated oligomers for optoelectronic device applications.
Experimental
General
1H NMR spectra were recorded on Varian VXR 400s and Varian Inova 500 instruments, operating at 400 and 500 MHz, respectively. Chemical shifts are quoted in ppm, relative to tetramethylsilane (TMS), using TMS or the residual solvent as internal reference. 13C NMR spectra were recorded using broad band decoupling, on the Varian VXR 400s or Varian Inova 500 at 100 MHz and 125 MHz, respectively. Electron impact (EI) mass spectra were recorded on a Micromass AutoSpec spectrometer operating at 70 eV with the ionisation mode as indicated. An Ati Unicam UV/VIS UV2 spectrometer was used to record the absorption spectra of the samples, which were held in 1 cm path length cuvettes. Emission and excitation spectra were recorded using a Jobin–Yvon Horiba Fluorolog 3-22 Tau-3 spectrofluorimeter. The spectra of dilute solutions with absorbance of less than 0.1 in the 200 to 400 nm range were recorded using conventional 90 degree geometry.
Device fabrication
For OLED studies a mixed solution containing MEH-PPV and 20% by weight of the dopant was prepared using p-xylene and chloroform as solvents (1∶1 v/v) and films of ca. 100 nm thickness were produced by spin-coating. ITO-coated glass was used as the anode, and an Al top electrode was evaporated onto the organic film.
X-Ray crystallography
The experiment was carried out on a Bruker 3-circle diffractometer with a SMART 6K CCD area detector, using graphite-monochromated Mo-Kα radiation (![[small lambda, Greek, macron]](https://www.rsc.org/images/entities/i_char_e0cc.gif)
=
0.71073 Å). The crystal was maintained at low temperature (T
=
120 K), using a Cryostream (Oxford Cryosystems) open-flow N2 cryostat. The structure was solved by direct methods and refined by full-matrix least squares against F2 of all data, using SHELXTL software. Compound 4 crystal data: C22H16N2, MW
=
308.37, monoclinic, space group P21/c
(No. 14), a
=
9.059(1), b
=
7.515(1), c
=
12.102(4)
Å, β
=
92.33(1)°, U
=
823.2(3)
Å3, Z
=
2, Dc
=
1.244 g cm−3, μ
=
0.07 mm−1. Full sphere of reciprocal space was covered by four sets of 0.3°
ω scans, each set with different φ and/or 2θ angles, yielding 10,887 reflections with 2θ
≤55°, of which 1891 were independent (Rint
=
0.039). All non-hydrogen atoms were refined in anisotropic approximation; all H atoms were located in a difference Fourier map and refined in isotropic approximation. The refinement of 240 parameters converged at R
=
0.038 [for 1465 reflections with F2
≥
σ(F2)] and wR(F2)
=
0.115 (for all data).§
Electrochemistry
Cyclic voltammetry experiments were performed on a BAS CV50W electrochemical analyser with iR compensation. Platinum wire, platinum disk (ø 1.6 mm) and Ag/Ag+
(0.01 AgNO3 in acetonitrile) were used as counter, working, and reference electrodes, respectively. CV experiments were performed in dry acetonitrile with 0.1 M Bu4NPF6 as supporting electrolyte. The scan rate was varied from 50 to 2000 mV s−1. The potentials were referred to the Fc/Fc+ couple as the internal reference, which showed a potential of +0.08 V vs. Ag/Ag+ in our conditions.
Computational procedures
The ab initio computations of compounds 4–9 and 12 were carried out with the Gaussian 9823 package of programs at the density functional theory (DFT) level using Pople's 6-31G and 6-311G split valence basis sets supplemented by one or two d-functions on heavy atoms and p-polarisation functions on hydrogens (or without them). DFT calculations were carried out using Becke's three-parameter hybrid exchange functional24 with Lee–Yang–Parr gradient-corrected correlation functional (B3LYP).25 Thus, the geometries were optimised with B3LYP/6-31G(d) and for compounds 4 and 12 also with B3LYP/6-311G(2d,p); electronic structures were calculated at the same level. Contours of the five highest occupied and five lowest unoccupied molecular orbitals were visualised using the Molekel v.4.2 program.26 No constraints to bonds/angles/dihedral angles were applied in the calculations and all the atoms were free to optimise.
Syntheses
2,5-Dichloro-3,6-dimethylpyrazine 2.
To alanine anhydride 1
(25.0 g, 0.176 mol) in POCl3
(150 cm3), PCl5
(9.0 g) was added and the mixture was heated (oil bath temperature 105
°C) for 24 h. The mixture was cooled to room temperature and excess POCl3 was removed by vacuum distillation. Cold brine was added slowly with stirring and a white solid formed after standing for 12 h. Then the mixture was extracted with dichloromethane and the organic layer was dried with MgSO4. The solvent was removed by vacuum evaporation and the residue was chromatographed on silica eluted with dichloromethane, followed by crystallisation from hexane to yield compound 2 as a white solid (9.3 g, 31%), m.p. 73–74
°C (lit.17 m.p. 72
°C). Found C, 40.12; H, 3.78; N, 15.63%. C6H6Cl2N2 requires C, 40.71; H, 3.42; N, 15.82%. MS (EI): m/z 176 (M+, 100%). 1H NMR (CDCl3): δ 2.61 (6H, s, CH3). 13C NMR (CDCl3): δ 21.24, 145.60, 149.77.
2,5-Dibromo-3,6-dimethylpyrazine 3.
A mixture of 2
(5.37 g 30 mmol) and PBr3
(15 cm3) was stirred at reflux (oil bath temperature 170
°C) for 24 h then cooled to 20
°C and excess PBr3 removed by vacuum distillation. Work up and purification as described for 2 gave compound 3
(2.8 g, 35%) as a white solid, m.p. 84.4–85.8
°C. Found C, 26.76; H, 2.52; N, 10.35%. C6H6Br2N2 requires C, 27.10; H, 2.27; N, 10.53%. MS (EI): m/z 266 (M+ 100%). 1H NMR (CDCl3): δ 2.58 (6H, s, CH3). 13C NMR (CDCl3): δ 22.97, 138.80, 152.4.
Preparation of 4-8: general procedure.
To the solution of 2,5-dibromo-3,6-dimethylpyrazine 3
(0.51 g, 2.0 mmol) and the alkynylarene (4.8 mmol) in dry THF (7.0 cm3), CuI powder (10.6 mg) was added. Triethylamine (0.9 cm3) was added and the solution was stirred at 20
°C for 10 min to obtain a clear solution. Pd(PPh3)2Cl2
(32 mg) was added in one portion and the mixture was stirred at 20
°C for 1 h, followed by reflux at 65
°C for another 1.5 h. The mixture was cooled to room temperature and the solid that formed during the reaction was removed by vacuum filtration. The filtrate was chromatographed on silica, eluted with DCM, followed by crystallisation from hexane. The compounds were thereby obtained as yellow solids.
2,5-Dimethyl-3,6-bis(phenylethynyl)pyrazine 4.
41% yield, m.p. 169–170
°C. Found: C, 85.44; H, 5.21; N, 9.15%. C22H16N2 requires C, 85.69; H, 5.23; N, 9.08%. MS (EI): m/z 308, (M+, 100%). 1H NMR (CDCl3): δ 2.69 (6H, s, CH3) 7.32 (6H, s, CH) 7.58 (4H, s, CH). 13C NMR (CDCl3): δ 21.75, 86.45, 96.72, 121.85, 128.51, 129.49, 132.06, 136.24, 153.02.
2,5-Dimethyl-3,6-bis(pyridin-2-ylethynyl)pyrazine 5.
31% yield, m.p. 201–202
°C. Found: C, 77.24; H, 4.57; N, 17.89%. C20H14N4 requires C, 77.40; H, 4.55; N, 18.05%. MS (EI): m/z 310 (M+, 100%). 1H NMR (CDCl3): δ 2.60 (6H, s, CH3), 7.31 (2H, s, CH), 7.48 (2H, s, CH), 8.56 (2H, s, CH). 13C NMR (CDCl3): δ 20.13, 72.46, 123.31, 127.26, 135.34, 137.42, 149.41, 153.97.
2,5-Bis(4-ethylphenylethynyl)-3,6-dimethylpyrazine 6.
38% yield, m.p. 196.5–198.3
°C. MS (EI): m/z 364, (M+, 100%). HRMS (EI): (M+) calcd. for C26H24N2 364.19395; found 364.19400. 1H NMR (CDCl3): δ 1.19 (6H, t, J
=
7.6 Hz, CH3), 2.62 (4H, q, J
=
7.6 Hz, CH2), 2.68 (6H, s, CH3), 7.15 (4H, d, J
=
8 Hz, CH), 7.48 (4H, d, J
=
8 Hz, CH). 13C NMR (CDCl3): δ 15.89, 22.15, 29.78, 86.20, 97.81, 119.73, 128.22, 132.31, 136.23, 148.34, 152.68.
2,5-Bis(4-chlorophenylethynyl)-3,6-dimethylpyrazine 7.
23% yield, m.p. 190.5–191
°C. MS (EI): m/z 376 (M+, 58%), 270 (100%). HRMS (EI): (M+) calcd. for C22H14Cl2N2 376.05340; found 376.05329. 1H NMR (CDCl3): δ 2.69 (6H, s, CH3), 7.31 (4H, s, CH), 7.48 (4H, s, CH). 13C NMR (CDCl3): δ 20.93, 74.66, 81.84, 120.63, 127.20, 133.21, 141.56, 156.45.
2,5-Bis(biphenyl-4-ylethynyl)-3,6-dimethylpyrazine 8.
24% yield, m.p. 188.0–188.7
°C. MS (EI): m/z 460, (M+ 100%). HRMS (EI): (M+) calcd. for C34H24N2 460.19395; found 460.19385. 1H NMR (CDCl3): δ 2.62 (6H, s, CH3), 7.18 (2H, s, CH), 7.29 (4H, s, CH), 7.38 (8H, s, CH), 7.61 (4H, s, CH). 13C NMR (CDCl3): δ 14.68, 74.66, 81.84, 120.63, 127.07, 127.14, 127.88, 128.93, 132.97, 140.10, 141.97, 156.91.
4-[5-(3-Hydroxy-3-methyl-but-1-ynyl)-3,6-dimethylpyrazin-2-yl]-2-methylbut-3-yn-2-ol 9.
Compound 3
(810 mg, 3.04 mmol), 2-methylbut-3-yn-2-ol (1.54 g, 18.24 mmol), CuI (58 mg), Pd(PPh3)2Cl2
(213 mg) and triethylamine (10 cm3) were mixed in dry THF (15 cm3) and stirred at room temperature for 1 h, then at reflux under Ar to obtain a brownish suspension. The mixture was filtered and the precipitate was washed with diethyl ether. The filtrate was evaporated under reduced pressure. The residue was purified by column chromatography on silica [eluent: ethyl acetate–petroleum ether (b.p. 40–60
°C), 7∶3 (v/v)] to give compound 9 as white solid (780 mg, 95%), m.p. 170–171
°C. Found: C, 70.43; H, 7.67; N, 10.02%. C16H20N2O2 requires C, 70.56; H, 7.40; N, 10.29%. MS (EI): m/z 272 (M+, 100%). 1H NMR (CDCl3): δ 1.59 (12H, s, CH3), 2.10 (2H, s, OH), 2.55 (6H, s, CH3). 13C NMR (CDCl3): δ 21.77, 31.34, 65.85, 79.40, 101.48, 136.18, 153.10.
2,5-Diethynyl-3,6-dimethylpyrazine 10.
Compound 9
(200 mg, 0.735 mmol) was dissolved in dry toluene (15 cm3). Sodium hydroxide powder (freshly ground from pellets; 64 mg) was added and the mixture was refluxed under Ar until 9 was completely consumed (TLC monitoring). The mixture was cooled to room temperature then suction filtered through a celite pad. The filtrate was evaporated in vacuo and residue was purified by column chromatography on silica [eluent: ethyl acetate–petroleum ether (b.p. 40–60
°C), 5∶4 (v/v)] to give compound 10 as a pale-yellow solid (88 mg, 80%), m.p. 175–176
°C. Found: C, 76.67; H, 5.34; N, 17.74%. C10H8N2 requires C, 76.90; H, 5.16; N, 17.94%. MS (EI): m/z 156 (M+, 100%). 1H NMR (CDCl3): δ 2.59 (6H, s, CH3), 3.48 (2H, s, CH). 13C NMR (CDCl3): δ 21.92, 80.32, 85.74, 136.08, 153.64.
2,5-Bis(thien-2-ylethynyl)-3,6-dimethylpyrazine 11.
Compound 10
(200 mg, 1.28 mmol), 2-iodothiophene (1.08 mg, 5.12 mmol), CuI (24 mg), Pd(PPh3)2Cl2
(89 mg) and triethylamine (10 cm3) were mixed in dry THF (15 cm3) and reacted according to the general procedure above. Column chromatography [silica, dichloromethane–hexane, 1∶1 (v/v)] gave compound 11 as a yellow solid (81 mg, 20% yield), m.p. 145–147
°C (dec). MS (EI): m/z 319.98 (M+, 100%). HRMS (EI): (M+) calcd. C18H12N2S2 320.0450; found 320.0441. 1H NMR (CDCl3): δ 7.36 (2H, d, J
=
4.8 Hz), 7.34 (2H, d, J
=
6.4 Hz), 6.99 (2H, t, J
=
8.8 Hz), 2.61 (6H, s). 13C NMR (CDCl3): δ 22.17, 90.47, 110.00, 121.91, 127.66, 129.48, 134.10, 136.21, 153.04.
Compounds 12 and 13 were obtained analytically pure using standard procedures.12
Acknowledgements
We thank the Royal Society and the Royal Society of Chemistry Journals Grant for International Authors for funding visits to Durham (I.F.P.), and One North-East via the County Durham Sub-regional Partnership project SP/082 for funding the purchase of equipment used in this work.
References
- M. Thelakkat and H.-W. Schmidt, Polym. Adv. Technol., 1998, 9, 429 CrossRef CAS.
-
(a) K. Chondroudis and D. B. Mitzi, Chem. Mater., 1999, 11, 3028 CrossRef CAS;
(b) C. Wang, M. Kilitziraki, L.-O. Palsson, M. R. Bryce, A. P. Monkman and I. D. W. Samuel, Adv. Funct. Mater., 2001, 11, 47 CrossRef CAS;
(c) C. Wang, G.-Y. Jung, A. S. Batsanov, M. R. Bryce and M. C. Petty, J. Mater. Chem., 2002, 12, 173 RSC;
(d) Y.-Y. Chien, K.-T. Wong, P.-T. Chou and Y.-M. Cheng, Chem. Commun., 2002, 2874 RSC;
(e) J. H. Kim, J. H. Park and H. Lee, Chem. Mater., 2003, 15, 3414 CrossRef CAS.
-
(a) J. A. Irvin, C. J. DuBois and J. R. Reynolds, Chem. Commun., 1999, 2121 RSC;
(b) S.-C. Ng, H.-F. Lu, H. S. O. Chan, A. Fujii, T. Laga and K. Yoshino, Adv. Mater., 2000, 12, 1122 CrossRef CAS;
(c) C. Wang, M. Kilitziraki, J. A. H. MacBride, M. R. Bryce, L. Horsburgh, A. Sheridan, A. P. Monkman and I. D. W. Samuel, Adv. Mater., 2000, 12, 217 CrossRef CAS;
(d) A. P. Monkman, L.-O. Palsson, R. W. T. Higgins, C. Wang, M. R. Bryce and J. A. K. Howard, J. Am. Chem. Soc., 2002, 124, 6049 CrossRef CAS.
-
(a) K.-T. Wong, T. S. Hung, Y. Lin, C.-C. Wu, G.-H. Lee, S.-M. Peng, C. H. Chou and Y. O. Su, Org. Lett., 2002, 4, 513 CrossRef CAS;
(b) C. C. Wu, Y. T. Lin, H. H. Chiang, T. Y. Cho, C. W. Chen, K. T. Wong, Y. L. Liao, G. H. Lee and S. M. Peng, Appl. Phys. Lett., 2002, 81, 577 CrossRef CAS;
(c) G. Hughes, C. Wang, A. S. Batsanov, M. Fearn, S. Frank, M. R. Bryce, I. F. Perepichka, A. P. Monkman and B. P. Lyons, Org. Biomol. Chem., 2003, 1, 3069 RSC.
- X. Zhang and S. A. Jenekhe, Macromolecules, 2000, 33, 2069 CrossRef CAS.
-
(a) J. M. Lupton, L. R. Hemingway, I. D. W. Samuel and P. L. Burn, J. Mater. Chem., 2000, 10, 867 RSC;
(b) J. Pang, Y. Tao, S. Freiberg, X.-P. Yang, M. D'Iorio and S. Wang, J. Mater. Chem., 2002, 12, 206 RSC.
-
(a) Reviews: A. Kraft, A. C. Grimsdale and A. B. Holmes, Angew. Chem., Int. Ed., 1998, 37, 402 Search PubMed;
(b) Y. Shirota, J. Mater. Chem., 2000, 10, 1 RSC;
(c) U. Mitschke and P. Bäuerle, J. Mater. Chem., 2000, 10, 1471 RSC.
- A. Wu, T. Akagi, M. Jikei, M. Kakimoto, Y. Imai, S. Ukishima and Y. Takahashi, Thin Solid Films, 1996, 273, 214 CrossRef CAS.
- Z. Peng and M. E. Galvin, Chem. Mater., 1998, 10, 1785 CrossRef CAS.
- F. Türksoy, G. Hughes, A. S. Batsanov and M. R. Bryce, J. Mater. Chem., 2003, 13, 1554 RSC.
-
(a) U. H. F. Bunz, Chem. Rev., 2000, 100, 1605 CrossRef CAS;
(b) M. I. Sluch, A. Godt, U. H. F. Bunz and M. A. Berg, J. Am. Chem. Soc., 2001, 123, 6447 CrossRef CAS.
- A. Beeby, K. Findlay, P. J. Low and T. B. Marder, J. Am. Chem. Soc., 2002, 124, 8280 CrossRef CAS and references therein.
-
(a) A. Montali, P. Smith and C. Weder, Synth. Met., 1998, 97, 123 CrossRef CAS;
(b) C. Schmitz, P. Pösh, M. Thelakkat, H.-W. Schmidt, A. Montali, K. Feldman, P. Smith and C. Weder, Adv. Funct. Mater., 2001, 11, 41 CrossRef CAS.
- S. H. Lee, T. Nakamura and T. Tsutsui, Org. Lett., 2001, 3, 2005 CrossRef CAS.
-
(a) R. C. Ellingson and R. L. Henry, J. Am. Chem. Soc., 1949, 71, 2798 CrossRef CAS;
(b) K. Pieterse, J. A. J. M. Vekemans, H. Kooijman, A. J. Spek and E. J. Meijer, Chem.-Eur. J., 2000, 6, 4597 CrossRef CAS.
- F. Ogura, Y. Hama, Y. Aso and T. Otsubo, Synth. Met., 1988, 27, B295 CrossRef CAS.
- K. W. Blake and P. G. Sammes, J. Chem. Soc. C, 1970, 1070 RSC.
-
(a) Reviews: K. Sonogashira, in Comprehensive Organic Synthesis, ed. B. M. Trost and I. Fleming, Pergamon Press, Oxford, 1991, vol. 3, p. 521 Search PubMed;
(b) K. Sonogashira, J. Organomet. Chem., 2002, 653, 46 CrossRef CAS.
- D. E. Ames, D. Bull and C. Takunda, Synthesis, 1981, 364 CrossRef CAS.
- Single crystal X-ray diffraction data for compound 12 are from T. B. Marder (private communication).
- Y. Cao, I. D. Parker, G. Yu, C. Zhang and A. J. Heeger, Nature (London), 1999, 397, 414 CrossRef CAS.
-
(a) P. Cea, Y. Hua, C. Pearson, C. Wang, M. R. Bryce, M. C. López and M. C. Petty, Mater. Sci. Eng., C, 2002, 22, 87 Search PubMed;
(b) P. Cea, Y. Hua, C. Pearson, C. Wang, M. R. Bryce, F. M. Royo and M. C. Petty, Thin Solid Films, 2002, 408, 275 CrossRef CAS.
-
M. J. Frisch, G. W. Trucks, H. B. Schlegel, G. E. Scuseria, M. A. Robb, J. R. Cheeseman, V. G. Zakrzewski, J. A. Montgomery, Jr., R. E. Stratmann, J. C. Burant, S. Dapprich, J. M. Millam, A. D. Daniels, K. N. Kudin, M. C. Strain, O. Farkas, J. Tomasi, V. Barone, M. Cossi, R. Cammi, B. Mennucci, C. Pomelli, C. Adamo, S. Clifford, J. Ochterski, G. A. Petersson, P. Y. Ayala, Q. Cui, K. Morokuma, D. K. Malick, A. D. Rabuck, K. Raghavachari, J. B. Foresman, J. Cioslowski, J. V. Ortiz, A. G. Baboul, B. B. Stefanov, G. Liu, A. Liashenko, P. Piskorz, I. Komaromi, R. Gomperts, R. L. Martin, D. J. Fox, T. Keith, M. A. Al-Laham, C. Y. Peng, A. Nanayakkara, M. Challacombe, P. M. W. Gill, B. G. Johnson, W. Chen, M. W. Wong, J. L. Andres, C. Gonzalez, M. Head-Gordon, E. S. Replogle and J. A. Pople, GAUSSIAN 98 (Revision A.9), Gaussian, Inc., Pittsburgh, PA, 1998 Search PubMed.
- A. D. Becke, Phys. Rev. A, 1988, 38, 3098 CrossRef CAS; A. D. Becke, J. Chem. Phys., 1993, 98, 5648 CrossRef CAS.
- C. Lee, W. Yang and R. G. Parr, Phys. Rev. B, 1988, 37, 785 CrossRef CAS.
-
(a)
P. Flükiger, H. P. Lüthi, S. Portmann and J. Weber, Molekel, Version 4.2, Swiss Center for Scientific Computing, Manno, Switzerland), 2000 (http://www.cscs.ch/molekel/) Search PubMed;
(b) S. Portmann and H. P. Lüthi, Chimia, 2000, 54, 766 CAS.
|
This journal is © The Royal Society of Chemistry and the Centre National de la Recherche Scientifique 2004 |