Aquatic ecosystems: effects of solar ultraviolet radiation and interactions with other climatic change factors†
First published on 10th January 2003
Abstract
Aquatic ecosystems are a key component of the Earth's biosphere. A large number of studies document substantial impact of solar UV radiation on individual species, yet considerable uncertainty remains with respect to assessing impacts on ecosystems. Several studies indicate that the impact of increased UV radiation appears relatively low when considering overall ecosystem response, while, in contrast, effects on individual species show considerable responses. Ecosystem response to climate variability incorporates both synergistic and antagonistic processes with respect to UV-related effects, significantly complicating understanding and prediction at the ecosystem level. The impact of climate variability on UV-related effects often becomes manifest via indirect effects such as reduction in sea ice, changes in water column bio-optical characteristics, changes in cloud cover and shifts in oceanographic biogeochemical provinces.
Introduction
Life on Earth has developed in the absence of a stratospheric ozone layer with much higher UV levels than today.1–3 Surviving populations of organisms likely possessed efficient strategies and physiological mechanisms to prevent and repair UV-induced damage4,5 including biosynthesis of UV-absorbing substances, DNA repair mechanisms and enzymes that reduce photooxidative stress.6,7 It has been postulated that some of the first screening pigments such as scytonemin may have evolved in cyanobacteria during the Precambrian and allowed colonization of exposed, shallow-water and terrestrial habitats.8 However, protection is not perfect, and UV-B can cause molecular damage to lipids, proteins and nucleic acids and it may exert indirect effects through oxidative stress due to molecular reactions of UV-B with cellular targets.9
Recent results continue to confirm the general consensus that solar UV negatively affects aquatic organisms.10–13 Reductions in productivity, impaired reproduction and development and increased mutation rate have been shown for phytoplankton,14,15 macroalgae,16,17 fish eggs and larvae18,19 zooplankton20 and primary and secondary consumers exposed to UV radiation.21–23 Decreases in biomass productivity due to enhanced UV-B are relayed through all levels of the intricate food web, possibly resulting in reduced food production for humans,21 reduced sink capacity for atmospheric carbon dioxide,24 as well as changes in species composition and ecosystem integrity.25 However, quantitative assessments of UV-related effects in natural waters are complex because species respond differentially to increased solar radiation and other environmental stress factors. This, in turn, affects physiological functions such as growth, reproduction and behaviour26 and the consequent population fitness and species interactions (Fig. 1). Consequently, community structure and trophic interaction will change with time and this ultimately will also alter biogeochemical cycling.27,28
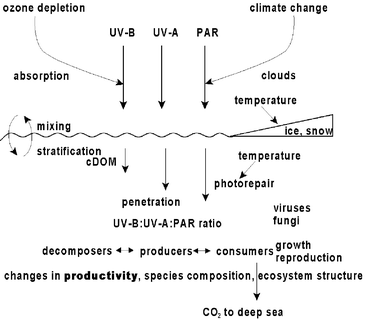 |
| Fig. 1 Concept of the aquatic food web affected by ozone depletion and climate change. PAR, photosynthetic active radiation (400–700 nm); CDOM, coloured dissolved organic material. | |
For many aquatic ecosystems, pre-ozone depletion conditions are not known; consequently effects caused by ozone depletion are difficult to evaluate.29 Impacts of environmental factors are evaluated mostly on the basis of specific species which should be carefully selected for long-term monitoring of environmental change.30 Species interactions and ecosystem dynamics are more difficult to evaluate, model and predict. Feedback mechanisms between aquatic ecosystems, physical factors and atmospheric and oceanic circulation have significant impact on primary productivity and ecosystem integrity, but are not well understood and are difficult to predict. Changing environmental conditions may have positive or negative effects on populations and species responses, and interact either positively or negatively with respect to UV-related changes,28,31 thus confounding quantitative assessment.32
Solar UV radiation and penetration in aquatic ecosystems
Incident solar UV radiation and the depth of penetration into the water column are key factors in assessing the potential for damage to aquatic organisms. In addition to the Antarctic ozone hole,33 increasing ozone loss has been observed over the Arctic during spring and early summer months.34 Areas with reduced ozone concentrations35 separate from the Arctic ozone hole and pass over Northern and Mid-Europe and sometimes even cross the European Alps,36 which increases the UV stress due to gradual ozone loss over mid-latitudes37 with clearly demonstrated trends of increasing UV-B radiation.38 Sabziparvar et al.39 have developed a model to describe the global climatology of the UV irradiation at the Earth's surface to predict future UV trends on a global scale.
Aquatic ecosystems differ tremendously in their transparency and thus the depth of solar UV penetration.40 Absorbing and scattering substances reduce the transparency of the water, especially in eutrophic freshwater systems and coastal areas of the oceans,41,42 while UV penetrates to greater depths in clear oceanic waters. Often there is a pronounced variability and seasonal changes in the transparency.43,44 In addition to inorganic particulate matter, dissolved and particulate organic carbon (DOC and POC) and various humic substances contribute considerably to the attenuation of short wavelength radiation.45 DOC is only slowly degraded in the water column but is broken down by solar UV to smaller subunits,46 which can be taken up by bacterioplankton, which consequently increases the transparency of the water column leading to a deeper penetration of solar UV.47 As a consequence, the concentration of DOC and the mechanisms that influence its abundance will have an important impact on the penetration of UV and the subsequent UV-related effects.48 Another consequence is a shift from autotrophic (photosynthetic) to heterotrophic (consumers) organisms under UV stress.49 Penetration of solar UV into the water column can be measured with a number of instruments.50 Recently, the attenuation of biologically effective UV radiation has been measured using biochemical dosimeters based on the formation of cyclobutane dimers in isolated DNA or on the behaviour of microorganisms.51,52 The impact of solar UV is modified by the depth and rate of the mixing layer.53
Bacterioplankton and picoplankton
Bacterioplankton and picoplankton are major constituents in the production and recycling of energy and nutrients within aquatic ecosystems. Disrupting the function of these critical components would have far-reaching impacts on organisms, including humans, many of which depend on aquatic ecosystems for their food supply.
Bacteria and small planktonic organisms (nano- and picoplankton) usually are too small to effectively protect themselves against solar UV by absorbing substances, since there is an upper limit of the concentration of these substances due to osmotic effects. These populations overcome the stress of solar UV (as well as predation and other adverse effects) by fast cell division and growth. In clear oceanic waters with high UV penetration, bacterioplankton are affected in the top layers of the water column. Growth and survival are impaired, and the activity of enzymes is inhibited. Solar UV damages the DNA mainly through the formation of pyrimidine dimers54 which may cause mutagenesis and cell death.55 As long as the repair mechanisms keep up with the damage,56 the population is not at stake; it is only when the dimers accumulate under strong ambient radiation, that the population will decrease.
Solar and artificial UV radiations were found to have little effect on the composition of coastal marine bacterioplankton communities in the North Sea.57 DNA showed only minor changes under the different radiation regimes. Some of the species were more sensitive than others, but only about 10% of the species appeared to be affected by UV radiation. The resistance of bacterioplankton to solar UV in these coastal areas is due to high turbidity and indicates the presence of an efficient DNA repair system, which has made bacterial evolution possible.58
Cyanobacteria
Cyanobacteria have a cosmopolitan distribution in both aquatic and terrestrial ecosystems ranging from hot springs to the Antarctic and Arctic regions. The significant role of these N2-fixing microorganisms in improving the fertility of rice paddy fields and other soils is well documented.59 Cyanobacteria are also prominent constituents of marine ecosystems and account for a significant percentage of oceanic primary productivity.
UV-B radiation is known to impair motility and photoorientation60 and to affect a number of other physiological and biochemical processes in cyanobacteria,21,61 resulting in reduced overall productivity, germination and differentiation.62 Photosynthetic pigments can be bleached by UV-B and the structure of the light harvesting complexes is affected resulting in impaired photosynthesis.63
Proteins and DNA are the main targets of UV-B.64 Enzymes of nitrogen metabolism show differential sensitivity towards UV-B. In contrast to the inhibition of nitrogenase and glutamine synthetase activity, there was an induction in nitrate reductase activity by artificial UV-B.62 The primary photosynthetic reactions and CO2 uptake are affected by UV-B.65Synechococcus resists UV-B by rapidly exchanging alternate protein forms in the photosynthetic apparatus. This molecular plasticity may be an important element in community-level responses to UV-B, where susceptibility to UV-B inhibition of photosynthesis changes diurnally.66 However, photosynthesis may be reactivated by UV-A/blue light exposure.67
Cyanobacteria have developed protective strategies to counteract the damaging effects of UV-B. These include (a) production of photoprotective compounds such as mycosporine-like amino acids (MAAs) and scytonemin,68,69
(b) escape from UV radiation by migration into habitats with reduced light exposure,21
(c) production of quenching agents such as carotenoids70 and superoxide dismutase,71
(d) repair mechanisms such as photoreactivation and light-independent nucleotide excision repair of DNA72 and (e) activity of a number of antioxidant enzymes.73 UV-B induces synthesis of MAAs in a number of cyanobacteria.74 A polychromatic action spectrum for the induction of MAAs in Anabaena sp. shows a single prominent peak at 290 nm.75 In addition to having photo-protective functions, MAAs also play an important role as osmotic regulators and antifreeze compounds.76 Other UV-A-absorbing compounds were found to be induced by UV-A.77 A database on photoprotective compounds in cyanobacteria and algae78 is available (www.biologie.uni-erlangen.de/botanik1/index.html).
Cyanobacteria form large mat communities in e.g. Antarctica. A Leptolyngbya mat showed significant photochemical inhibition under supplemental UV-B, while inhibition was less prominent in a Phormidium mat.79 The latter contained 25 times the concentration of UV protecting MAAs and double the concentration of carotenoids compared to the Leptolyngbya mat showing the ameliorating action of screening pigments. Rai and coworkers80 studied the interactive effects of UV-B and heavy metal pollution on nitrogen-fixing cyanobacteria and found synergistic effects of the two stress factors.
Phytoplankton
Phytoplankton are by far the major biomass producers in the oceans and thus represent the base of the food web. Their productivity matches that of all terrestrial ecosystems taken together.81 A large number of recent studies indicate a considerable sensitivity to solar ambient UV of phytoplankton communities distributed from polar to tropical habitats.82,83 Satellite studies over the last decade indicate a significant global decrease in phytoplankton.84 The reasons for this are not known, but the authors suggest increased stratification. Solar UV impairs photosynthesis, bleaches photosynthetic pigments, nitrogen metabolism and induces DNA damage.85,86 However, there are efficient protection and repair mechanisms in these organisms, including the xanthophyll cycle in photosynthesis, screening pigment production, synthesis of antioxidants and DNA repair.87–89 While the effectiveness of screening pigments was postulated but not demonstrated in the past, recent experiments showed that vital physiological functions were protected by the presence of MAAs.90
Large-scale quantification and assessment of phytoplankton photochemical characteristics can be determined by pigment analysis91 or by airborne monitoring using laser pump-and-probe techniques.92 Systematic monitoring revealed strong daytime declines in photosynthetic quantum yield under ambient light in the near-surface water layer over large aquatic areas.
Blooms of diatoms or Phaeocystis are common during Austral spring. Phaeocystis has a high UV absorption, but there can be a 10-fold variability in the screening pigment to chlorophyll ratio. Its sensitivity to UV is not clear: several experimental approaches have suggested that it is more sensitive to UV than diatoms, which have a significantly lower UV absorption.93 In contrast, other studies show changes in species composition favouring Phaeocystis.94 Colonial Phaeocystis antarctica produce a number of MAAs which strongly absorb in the UV.95
The repair mechanism of the photosynthetic apparatus was studied in UV-B sensitive and tolerant species of several alga groups.96 Application of an inhibitor of chloroplast protein synthesis aggravated the UV-B induced inhibition of photosynthesis in UV-B tolerant species while it did not in the sensitive species. Thus, UV-B tolerance of photosynthesis is associated with recovery capacity and repair. Phytoplankton are also affected by changing ambient CO2 concentrations. However, they can acclimate to a wide range of CO2 concentrations.97 It should be mentioned that many results are based on short-term studies (days). This is quite appropriate, since the organisms have short generation times on the order of hours or days. However, genetic adaptation to increased UV stress is expected to occur on much longer time scales.
Models have been developed to predict the productivity of phytoplankton communities98 taking into account the variability in exposure and differences in sensitivity. Another model evaluates the role of 3D currents, vertical mixing and turbulence on the growth and dispersion of marine phytoplankton.99
Studies in Patagonia (Argentina), which is occasionally under the influence of the Antarctic ozone hole, showed that photosynthetic inhibition in phytoplankton varies considerably between different environments and it depends on the optical depth of the water column. The contributions to decreased photosynthesis of UV-A and UV-B were approximately equal and maximum inhibition was about 35% at the surface.100 Freshwater phytoplankton seem to be more inhibited by solar UV than marine phytoplankton. There was also significant DNA damage (cyclobutane pyrimidine dimers, CPD), which was higher than in tropical seawater.101 However, in evaluating the impact of solar UV it is necessary to monitor other variables such as changes in cloud cover, species composition including cell size distribution, and depth of the mixed layer.
The importance of cloudiness has been shown by an analysis of solar irradiance measurements from Ushuaia (Argentina) and Palmer (Antarctica). Calculations showed that the biologically effective daily UV doses changed to a larger degree due changing cloud cover than with ozone depletion.102
Sensitivity to natural UV radiation varies considerably between dominant phytoplankton species as shown in three Arctic lakes.103 Growth of small chlorophytes, diatoms and cyanobacteria was impaired mainly by short wavelength UV while the larger colony forming species were stimulated. Since the latter species are not preferred food for Daphnia they dominated in the end of the experiment.
Macroalgae and seagrasses
Macroalgae and seagrasses are important biomass producers along the coastlines and on the continental shelves. They are exploited commercially on a large scale and form habitats for larval stages of fish, shrimp, crustaceans and other ecologically and economically important animals. Both short- and long-term exposure to solar radiation inhibits growth in adult stages of several species of macroalgae.104 Photosynthesis is seriously impaired in red, brown and green algae105–107 resulting in reduced oxygen production.108 Susceptibility of marine macroalgae to UV is highly variable among species which results in a specific depth distribution of species.109 Deep-water species are generally more sensitive to UV radiation than intertidal species.110 This can be easily demonstrated by transplantation experiments of algae from deep to shallow waters.111 Eulittoral and upper sublittoral species generally tolerate or acclimate to UV.112
In King George Island, Antarctica, biomass productivity and species diversity decreased at 20 m, probably due to limited light conditions.113 Also in the Arctic fjords primary productivity of seaweeds is strongly affected by the availability of solar radiation.114 Even though the downwelling solar UV-B radiation never exceeded 0.27 W m−2, UV radiation penetrated deeply into the water column and affected primary productivity to a depth of 5–6 m. The harmful effects were ameliorated during summer following the influx of turbid fresh water from snow and glacier ice into the fjord water, increasing the light attenuation in the top water layer.
Many macroalgae produce one or several UV absorbing substances.115 Most MAA-producing species belong to the red algae, followed by brown algae, and only a few green algae produce MAAs.78 Three different types of protection by UV screening pigments have been found: one group (sublittoral algae not likely to be exposed to higher doses of solar UV) does not synthesize UV absorbing pigments at all; another group produces high amounts of MAAs, but they cannot be further induced by exposure to any radiation.78 This group includes supralittoral species with high natural exposure to solar UV. In the third group, MAA production can be induced by solar radiation. MAAs are very stable compounds and are not easily modified by heat, UV or extreme pH.116,117 A polychromatic action spectrum was determined for the induction of MAAs in the chlorophyte Prasiola stipitata showing a clear maximum at 300 nm.118 In contrast, in the red alga Chondrus crispus blue light and UV-A radiation control the synthesis of MAAs, but the induction by UV was not investigated.119 While most algae use MAAs, a few produce different types of UV-absorbing compounds.120
Young developmental stages of algae (zoospores, gametes, zygotes and young germlings) are extremely susceptible to UV radiation stress:121 Mortality of zoospores of kelp species from southern Spain was induced by UV radiation (more by UV-B than by UV-A).122 In kelp zoospores there is loss of viability, cellular disintegration and impairment of motility and phototaxis.123 DNA damage (pyrimidine dimers) increased linearly with UV-B dose.122 Zoospores of the shallow water species Chordaria flagelliformis need higher UV doses than the mid-sublittoral species Laminaria saccharina to suffer mortality.122 Polarity formation, mitosis and cytokinesis during development of brown algae zygotes are affected.
The green alga Chara is regarded as a link to higher plants, which makes it interesting for research on UV-related responses. Under elevated UV-B there was an increased vegetative reproduction while generative reproduction was suppressed. UV-B did not induce the synthesis of protective pigments in this alga.124
Zooplankton
Zooplankton are primary consumers in the aquatic food web, providing a vital link to populations, including humans, that depend upon aquatic ecosystems for their food supply. In earlier research the vertical migration of zooplankton into lower and darker water layers was explained by the avoidance of visually oriented predators (e.g., fish). Today UV exposure is assumed to be a contributing, hazardous factor.125
The sensitivity of zooplankton to UV radiation was tested for several species of Daphnia that differed in their pigmentation.126 Both melanin and carotenoid pigments protect these organisms from UV. Under UV, all Daphnia species moved deeper into the water, but the extent of the response was inversely correlated with the pigmentation—the lighter pigmented species migrating deeper into the water column.127 In another experiment Daphnia was found to migrate away from the surface at midday and in late afternoon, while animals in UV shielded controls remained closer to the surface. However, the vertical swimming behaviour itself, as well as their phototactic orientation, is impaired by exposure to full spectrum solar radiation.128,129
Large variability was found in the concentration of the photoprotective compounds, MAAs, among zooplankton from lakes located along an elevation gradient.130 The concentration of these photoprotective compounds was inversely related to the attenuation coefficient of the water and in the lakes. MAAs, together with other photoprotective compounds, play a major role in minimizing the damaging effects of solar UV radiation in zooplankton from transparent lakes. UV-B is a major stress factor for zooplankton, particularly in high mountain lakes, which have high transparency, especially above the tree line.40
The copepod Calanus is a key component in marine zooplankton communities in the Gulf of St. Lawrence (Fig. 2). Eggs are released in spring and summer in shallow water and incubate for 1–3 days. At current levels, UV radiation has a negative impact on eggs residing in the first few meters of the water column.131 Again, variability in cloud cover, water transparency and vertical distribution may have a greater effect on UV-B exposure than ozone depletion. Kuhn and coworkers132 have modeled the UV induced mortality in the early life stages of Calanus finmarchicus. The lowest modeled daily survival was 59% under ambient ozone and 49% under simulated 50% ozone loss.
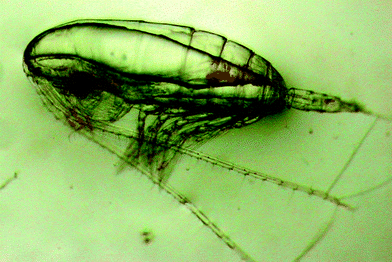 |
| Fig. 2 Photograph of the fifth copepodite of Calanus marshallae Frost. (Photo by Jaime Gomez-Gutiérrez.) | |
The copepod Diaptomus showed no increased mortality during food shortage when incubated at 0.5 m in a clear-water lake, but food-replete animals did. These results show that UV tolerance is not affected by short-term lack of energy or nutrients. Under artificial UV the animals showed high mortality, which could not be improved by feeding.133
Usually organisms tolerate damaging UV better if they are exposed to low irradiances for a longer time (in the presence of UV-A or visible light) than to high irradiances for a short time (indicating the lack of reciprocity), because their photorepair system keeps up with the damage.134 However, in some strains there is reciprocity, due to a lack of photorepair. In Asplancha and Daphnia the offspring died if no photorepair-inducing radiation was provided.
Secondary consumers
Although humans use about 8% of the productivity of the oceans, that fraction increases to more than 25% for upwelling areas and to 35% for temperate continental shelf systems. For about one-sixth of the world's population (primarily developing nations), the oceans provide more than one-third of their animal protein. Many of the fisheries that depend upon the oceanic primary productivity are unsustainable. Although the primary causes for a decline in fish populations are predation and poor food supply for larvae, overfishing of adults, increased water temperature and pollution and disease; exposure to increased UV-B radiation may also contribute to that decline. The eggs and larvae of many fish are sensitive to UV-B exposure. However, imprecisely defined habitat characteristics and the unknown effect of small increases in UV-B exposure on the naturally high mortality rates of fish larvae are major barriers to a more accurate assessment of ozone depletion on marine fish populations.
In the Gulf of Maine, UV-A penetrates to 23 m and UV-B to 7–12 m where the embryos and larvae of the Atlantic cod develop. Exposure to UV equivalent to 10 m depth resulted in a significant mortality of developing embryos and a significant decrease in length of larvae.135 These irradiances occur in many temperate latitudes where these ecologically and commercially important fish spawn.
After exposure to solar UV larvae had lower concentrations of UV absorbing compounds and greater DNA damage. However, they had higher activities of the antioxidant superoxide dismutase and transcriptional activator p53. p53 is expressed in response to DNA damage and can result in cellular growth arrest during the cell cycle or to programmed cell death (apoptosis). Cellular death caused by apoptosis is the most likely cause of mortality in embryos and larvae in these experiments, while the smaller size at hatching in those larvae that survived is caused by permanent cellular arrest in response to DNA damage.
In another experiment, the effect of UV on the success of early life history stages of bluegills was tested for fish from two lakes with different underwater UV environment. Survival was as low as 20% at 0.1 m, but higher at 1 m depth. Embryos responded similarly to UV exposure regardless of the lake of origin. UV-B is an important factor in the success of early life history stages especially in high transparency lakes. Bluegills constructed their nests deeper in a lake with high UV transparency than in a less transparent lake.136
Fingerling channel catfish were found to be quite sensitive to solar UV-B.137 After a 24 h exposure, thinning of the dorsal epidermis was observed accompanied by edema and sunburn cells. After 48 h exposure, cell death and sloughing of the outer epidermal layer were widespread.
The impact of UV on Atlantic cod eggs and larvae was studied in the estuary and Gulf of St. Lawrence22 in comparison with the ambient levels of UV radiation and penetration in this subarctic marine ecosystem. Exposure to UV-B produced a significant negative effect; however, these direct effects are likely to be minimal within the context of all the other environmental factors that produce the very high levels of mortality typically observed in their planktonic early life stages.
Also in the Gulf of St. Lawrence, certain larval stages of the American lobster spend the daylight hours in the top two meters of the water column throughout the breeding season. Lobster larvae may therefore be exposed to high doses of UV radiation; yet larvae exposed to radiation had a mortality rate comparable to that of protected larvae.138 This outcome suggests that lobster larvae are tolerant to UV radiation. A combination of adaptations to planktonic life near the surface, such as production of light-blocking pigments and other effective mechanisms, may account for the relative resistance of lobster larvae to UV radiation.
There is quantitative evidence for global amphibian population declines.139 One extensive study was based on large-scale temporal and spatial variations in amphibian population trends from 936 populations in North America and Western Europe.140 On a global scale, the data indicate rapid declines from the late 1950s to the late 1960s with subsequent slower declines. These declines have complex causes, including pathogen outbreaks, interannual variability in precipitation, climate-induced changes and possibly UV-B exposure.141 Climate-induced reductions in water depth at sites where eggs are laid have caused high mortality of embryos due to increased exposure to solar UV-B radiation and subsequent vulnerability to infection. Precipitation is strongly linked to El Niño events underscoring the role of large-scale climatic patterns. Elevated surface temperatures affect the climate over much of the world and if warming continues this could be responsible for future pathogen-mediated amphibian declines in many regions. While a number of studies have not found evidence for negative effects of UV radiation on amphibian early embryonic development,142 sublethal effects that can manifest themselves at later developmental stages can occur.143 In addition, another study on frogs did not find clear evidence for reduced hatchability or increased frequency of developmental anomalies of embryos exposed to ambient UV-B.144 However, hatchling size was significantly larger when UV-B was blocked, indicating that solar UV-B has a negative effect on early hatchling growth. In yet another study, enhanced UV-B was found to induce high mortality and damage to the skin and ocular system of two other species of tadpoles.145 After 1 month of hatching only 3–18% survived under enhanced UV-B as compared to 52–55% at ambient radiation and 44–65 % in the controls. A series of careful studies tried to assess the risk of solar ultraviolet radiation to the northern leopard frog (Rana pipiens) in 26 North American wetlands.146,147 Full sunlight caused approximately 50% mortality of the frogs during early larval development and about 97% hindlimb malformations.146 Filtering the UV-B wavelengths out almost completely eliminated the effects. The difficulty arises when one tries to evaluate the radiation doses perceived by the organisms in their natural habitat. This depends on shading, the attenuation in the water column and the behaviour of the animals.147 On the basis of radiation monitoring over the year and behavioural observations, the authors conclude that estimated UV-B doses never exceeded detrimental levels in 21 of the 26 wetlands in Minnesota and Wisconsin.147 However, continued reduction of ozone and other climate change effects may increase UV doses above the damage threshold.
The catastrophic declines in corals and sea urchins on a global scale are only partially offset by some local recoveries.148,149 Multiple factors seem to be responsible for these declines, including rising temperatures, pollution and other anthropogenic causes. While protection by UV absorbing pigments has been shown for many species,150 a primary effect of increased solar radiation on survival and growth is still uncertain.
Ecosystems
All ecosystems are affected by gradual changes in important environmental factors including climate, nutrient loading, habitat fragmentation or biotic exploitation.151 In addition, ecosystems are now being subjected to relatively rapid anthropogenic climate and UV-related changes which may lead to large shifts in the system.148 Also, it is at the ecosystem level where assessment of anthropogenic climate change and UV-related effects are confounded and where there is the potential for both synergistic and antagonistic effects. Recent studies have shown that these changes may lead to loss of ecosystem resilience that often drives the system into a different structure. This is particularly important when strategies for sustainable management of ecosystems are developed. The seasonal timing of anthropogenic changes is critical. For example, in the Arctic and the Antarctic ecosystems as well as many temperate freshwater biotopes the onset of spring phytoplankton blooms and spawning in invertebrates as well as vertebrates coincides with ozone depletion as well as shifts in several climate-related parameters. Ozone monitoring and ground-based measurements show a clear downward trend of stratospheric ozone, with attendant increases in UV-B, over both polar regions.
Freshwater ecosystems
Olesen and Maberly152 have measured oxygen production in natural phytoplankton populations from a freshwater lake in situ at mid-summer. They show that fluxes of PAR, UV-A and UV-B accounted for 81% of the variation in gross photosynthesis and that UV-A was more inhibitory than UV-B radiation. In another study the impact of UV-B on food quality in four western boreal toad breeding ponds was carried out.153 These workers found that ambient solar UV-B exposure decreased protein concentration, shifted the community composition of algae and reduced the food quality within the ponds.
Williamson and co-workers have shown that in order to evaluate anthropogenic impacts on lakes a variety of parameters need to be assessed. These parameters should include acid precipitation, heavy metal and toxic organic contaminants, and the concentration of CDOC (coloured dissolved organic carbon), in addition to increased UV radiation and changes due to global warming.154 Further, the anthropogenic acidification often causes changes in zooplankton community structure, which in turn also affects zooplanktivourous predators.155
The Antarctic aquatic ecosystem
An assessment of UV-B effects for polar aquatic ecosystems is complex and requires consideration of the ecosystem as a whole (Fig. 3). In particular, climate variability has been shown to have important synergistic influences on UV-B. Because of being close to freezing temperatures, polar ecosystems are particularly sensitive to change because the freeze/thaw boundary applies critical limits to subsequent environmental responses including: air and water temperature, the timing, extent and duration of ice and snow cover, changes in the surface albedo, changes in water column CDOM concentrations and the level of solar radiation and its penetration depth. Such changes, driven by climate variability, may be more important for UV-B exposure levels and spectral balance between UV-B and PAR than ozone depletion. In addition, the known increases in UV-B from reduction of stratospheric ozone may be augmented or reduced by changes in cloud cover related to climate variability. A summary of nearly a decade of ship and satellite observations along the western Antarctic Peninsula showed a very large (nearly an order of magnitude) interannual variability both spatially and temporally.156 From the perspective of trying to understand the possible influence of a multitude of environmental factors, such as ozone-related enhanced UV-B, cloud cover and sea ice season, this large interannual variability makes it difficult do attribute cause and effect.
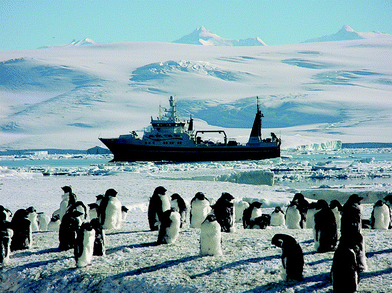 |
| Fig. 3 Antarctic ecosystem with research vessel Tangaroa, New Zealand, in the background. | |
Recent work confirms changes in UV effects consistent with the physiological effects of temperature, both directly on photosynthesis as well as indirectly through the enzymes involved in repair of UV damage.98 These authors concluded that changes in temperature can have strong effects on UV sensitivity, and point out the importance of repair processes. Thus, ecological response to climate variability with attendant indirect UV-related effects may mask direct ozone-related UV effects. While the ecological significance of enhanced ozone-related UV radiation continues to be debated, the ecological response to a statistically significant warming trend in the western Antarctic Peninsula region over the past half century, with corresponding reduction in sea ice extent, has been demonstrated for several trophic levels.157,158 It is well documented that changes in underwater UV exposure have the capacity to directly affect the species composition of aquatic biota at various trophic levels and to cause effects that may cascade throughout the trophic structure.159 It is also clear that this complex combination of direct and indirect effects will result in future changes in aquatic ecosystem structure. However, the individual contribution of climate variability and ozone-related increases in UV radiation are extremely difficult to untangle and/or predict.
Marine plankton can be used as sensitive indicators of UV-B fluctuations at the Earth's surface. Significant UV-B penetration occurs to 20–30 m.160 A first report indicated a close correlation between DNA damage and UV-B irradiance in Antarctica.161 DNA dosimeters were 2–4 times more sensitive than small phytoplankton, indicating that photoprotective and DNA repair mechanisms reduce DNA damage in bacterioplankton and phytoplankton. However, phytoplankton had higher DNA damage levels after daily exposure than bacterioplankton. This is in contrast to the findings by Jeffrey et al. in the Gulf of Mexico.162 This could indicate a more efficient photorepair in bacteria, depending on temperature.
The Arctic aquatic ecosystem
Ozone monitoring and ground-based measurements show a clear downward trend of total column ozone of −6.1% per decade for April and −3% per decade for June (1979–1999); however, there is large variability.163 Calculations using a multiple scattering radiation transfer model for the period from 1979 to 1999 predict increases in ground-based UV of 8% per decade (April); however, high surface albedo and cloud cover may strongly affect the UV level.
Aas and co-workers have characterized the attenuation of solar radiation both in Atlantic and coastal waters of the Barents Sea and Nordic Seas.164 They have documented both the spatial and seasonal variability of water transparency and the attenuation of UV radiation for these waters. Available data suggest that Arctic marine phytoplankton populations may be more sensitive to solar UV radiation than their Antarctic counterparts;165 however, the role of long-term acclimation processes is not clear. In both areas there was a shift in species composition to diatom-dominated assemblages, which are capable of synthesizing UV screening compounds. Available results indicate that currently measured UV levels do not seriously affect macroalgal communities in high Arctic coastal ecosystems. While growth and photosynthesis are affected by solar UV-B, all species studied so far have sufficient acclimation potential to cope with moderately increased UV levels.166
Sea ice and snow are highly scattering and absorbing and therefore their presence or absence has a very large influence on the penetration of UV radiation. Perovich has measured the spectral transmittance of snow and sea ice.167 He shows, for example, that 10 cm of snow reduces UV-B transmittance by about a factor of 40, which protects the biota from UV but which allows a substantial amount of visible radiation to penetrate. The potential risk is that there is a large step-up in UV impact on non-adapted organisms when the ice melts. UV albedos can range from above 0.9 for fresh snow to 0.7 for ice and 0.2 for ice with melted surface areas (ponded ice).
Due to the extreme climatic conditions in combination with anthropogenic contamination, increased solar UV-B may have a considerable impact on freshwater ecosystems.168 Most Arctic freshwater ecosystems are characterized by low nutrient concentration, low temperature—and consequently low productivity and low DOC—making them particularly vulnerable to UV stress. These characteristics are also found in high alpine lakes.169 Changes in underwater UV exposure directly affect species composition of the biota at each trophic level. The abundance of cyanobacteria in microbial mats indicates their efficient defense mechanisms including absorbing pigments and vertical migration.170 In the future the largest changes in UV exposure in the Arctic may be associated with changes in water quality and vegetation linked with climate change, and there is evidence of substantial warming over the last 30 years in some regions of the Arctic.171 In addition, this warming has lead to a statistically significant reduction in the temporal period of ice cover in many circumarctic lakes which results in increasing periods of UV exposure in the water column.172 Changes in UV exposure may influence not only species composition but also cause a shift in the balance between benthic and pelagic primary production as has been shown by paleo-optical studies of subarctic lakes during the Holocene.173
The zooplankton food chains are rather simple with only few species dominated by melanic (pigmented) morphs of Daphnia species.174 While a number of potential effects can be deduced from laboratory and field studies, well-founded conclusions on UV-induced community effects are premature at this stage. Synergistic effects of UV and other stress factors as well as lack of food due to low primary productivity or change in food quality under increased UV radiation may make zooplankton more vulnerable.175
Interactions between UV-B increases and other environmental factors
In addition to higher levels of solar UV-B radiation, aquatic ecosystems are confronted with other environmental stress factors including increased nutrient input, pollution, acidification and global climate change. In turn, climate change will result in water temperature and sea level change; shifts in the timing and extent of sea ice cover; changes in salinity and altered stratification of the water column, wave climate and ocean circulation; and these effects will be linked by feedback mechanisms which are not yet completely understood.176 These complex changes are likely to have significant impacts that will vary both spatially and temporally, including biological production (including human marine resources) as well as changes in the global hydrological cycle, vertical mixing and efficiency of CO2 uptake by the ocean. Ozone-related increases in UV-B are an important additional ecological stress that will have both positive and negative impacts in association with the other factors.
Several recent reviews have tackled the interaction between climate change and ozone depletion.174,177–179 Ozone depletion has occurred only during the last few decades,180 exceeding 50% of the pre-ozone hole concentrations at some locations. Because of the strict control measures imposed, the concentrations of ozone depleting substances will decrease during the next decades.181 However, increasing CO2 concentrations will result in warming of the troposphere and simultaneous cooling of the stratosphere, which favours further ozone destruction.182,183 One of the possible feedback mechanisms is changes in cloud cover and increased rainfall.
Direct monitoring of the ambient and yearly average temperatures are restricted to about 120 years in Europe and much less in other parts of the world,184–186 although information on past temperatures can be extracted from ice core and sediment records.187 Non-anthropogenic changes of ambient temperature fluctuated over millennia, while the recent global climate change has occurred on the order of a few decades. Expected and already measured temperature changes are not uniform over the surface of the earth. While on the Antarctic Peninsula a decrease in sea ice was observed over the last two decades,188 increases in ice cover were monitored over most of the Antarctic continent.189 Temperature changes significantly affect carbon assimilation, and even small changes could alter species competition, timing of reproduction and hatching success.190–192
Changing temperatures can lead to sea ice variability, altered nutrient cycling, food availability and trophic interactions.32 Melting of sea ice, with relatively fresh water, provides water column stability, thus enhancing springtime phytoplankton blooms. Recent work has shown that glacial melt water (enhanced due to the past century's warming trend in the Antarctic peninsula) is associated with enhanced productivity, extending over 100 km offshore.193 Field experiments have shown that higher temperatures increase colonization by cyanobacteria and increase arthropod populations. Changes in species composition and expansions of macroalgae populations have been reported in response to local temperature increases.194 Changes in ice cover will modify gas and heat exchange between the ocean and the atmosphere,195,196 and incident light and UV penetration into the water column will be affected. Consequently carbon fluxes and photosynthesis of phytoplankton and ice communities will be affected.29 Thus, changing temperatures affect primary consumers as well as secondary consumers, trophic dynamics and biogeochemical cycling.197–200
There are major feedback loops between climate change and other environmental variables and primary productivity. The primary producers are responsible for large uptake of atmospheric CO2, part of which is sunk into the marine sediments as oceanic snow. Because of physiological differences in the substrate affinity of the CO2 fixing enzymes in different organisms, changes in the partial pressure will alter autotroph diversity.201 With phytoplankton productivity being affected by climate change, this will simultaneously modify the degree of climate change.199,202–204 Increasing temperatures enhance rainfall and melting of glaciers and ice shelves augmenting the runoff of melt water and stabilizing the stratification of the water column, which in turn increases the sedimentation of particulate organic matter. Pronounced stratification decreases nutrient concentrations of coastal waters and increases UV-B exposure of phytoplankton.205,206
Conclusions and consequences
All ecosystems are likely to be affected by gradual changes in important environmental factors, including climate, nutrient loading, habitat fragmentation or biotic exploitation. As with other environmental stress factors, UV-B elicits species-specific responses with a high degree of intraspecies variation. Potential consequences of enhanced levels of exposure to UV-B radiation, demonstrated in recent experiments, include loss of biomass, including food sources for humans; changes in species composition; decrease in availability of nitrogen and other nutrients; and reduced uptake capacity for atmospheric carbon dioxide, resulting in the potential augmentation of global warming. Temperature changes in Antarctica can, for example, significantly affect carbon assimilation, and even small changes could alter species competition, timing of reproduction, and hatching success. Changing temperatures also have indirect effects, such as changes in sea ice variability, and altered nutrient cycling and food availability within the food web. In addition, there is emerging evidence that global warming and acid precipitation may allow increased penetration of UV-B and UV-A radiation into aquatic environments, predominantly through decreases in attenuation of radiation by dissolved organic carbon. Although there is significant evidence that increased UV-B exposure is harmful to aquatic organisms, damage at the whole ecosystem level is still uncertain. In the Arctic, however, while growth and photosynthesis are affected by solar UV-B, all species studied so far have sufficient acclimation potential to cope with moderately increased UV levels. One of the most important concepts for assessing the impacts of enhanced levels of UV-B exposure on aquatic ecosystems is that complex rather than simple responses are likely to be the rule. Responses will not be limited to simple decreases in primary production. In fact, shifts in community structure may initially be more common and result in small, yet detectable differences in ecosystem biomass.
References
-
L. J. Rothschild, in Origin, Evolution and Versatility of Microorganisms, ed. J. Seckbach, Kluwer, Dordrecht, The Netherlands, 1999, pp. 551–562 Search PubMed.
- F. Garcia-Pichel, Solar ultraviolet and the evolutionary history of cyanobacteria, Origins Life Evol. Biosphere, 1998, 28, 321–347 Search PubMed.
-
C. S. Cockell, in Ecosystems, Evolution and Ultraviolet Radiation, eds. C. S. Cockell and A. R. Blaustein, Springer-Verlag, New York, 2001, pp. 1–35 Search PubMed.
- L. J. Rothschild and R. L. Mancinelli, Life in extreme environments, Nature, 2001, 409, 1092–1101 CrossRef CAS.
- E. G. Nisbet and N. H. Sleep, The habitat and nature of early life, Nature, 2001, 409, 1083–1091 CrossRef CAS.
- K. Hoyer, U. Karsten, T. Sawall and C. Wiencke, Photoprotective substances in Antarctic macroalgae and their variation with respect to depth distribution, different tissues and developmental stages, Mar. Ecol. Progr. Ser., 2001, 211, 117–129 Search PubMed.
- S. J. Newman, W. C. Dunlap, S. Nicol and D. Ritz, Antarctic krill (Euphausia superba) acquire a UV-absorbing mycosporine-like amino acid from dietary algae, J. Exp. Mar. Biol. Ecol., 2000, 255, 93–100 CrossRef CAS.
- J. G. Dillon and R. W. Castenholz, Scytonemin, a cyanobacterial sheath pigment protects against UVC radiation: implications for early photosynthetic life, J. Phycol., 1999, 35, 673 CrossRef CAS.
- Y.-Y. He and D.-P. Häder, Involvement of reactive oxygen species in the UV-B damage to the cyanobacterium Anabaena sp., J. Photochem. Photobiol., B, 2002, 66, 73–80 Search PubMed.
-
A. R. Blaustein, L. K. Belden, A. C. Hatch, L. B. Kats, P. D. Hoffman, J. B. Hays, A. Marco, D. P. Chivers and J. M. Kiesecker, in Ecosystems, Evolution and Ultraviolet Radiation, eds. C. S. Cockell and A. R. Blaustein, Springer-Verlag, New York, 2001, pp. 63–79 Search PubMed.
- A. G. J. Buma, E. W. Helbling, M. K. de Boer and V. E. Villafañe, Patterns of DNA damage and photoinhibition in temperate South-Atlantic picophytoplankton exposed to solar ultraviolet radiation, J. Photochem. Photobiol., B, 2001, 62, 9–18 Search PubMed.
-
F. L. Figueroa, in Role of Solar UV-B Radiation on Ecosystems, Ecosystem Research Report No. 30, eds. C. V. Nolan and D.-P. Häder, European Communities, Belgium, 1998, pp. 121–133 Search PubMed.
-
D.-P. Häder, in Ecosystems, Evolution and Ultraviolet Radiation, eds. C. S. Cockell and A. R. Blaustein, Springer-Verlag, New York, 2001, pp. 150–169 Search PubMed.
- A. U. Bracher and C. Wiencke, Simulation of the effects of naturally enhanced UV radiation on photosynthesis of Antarctic phytoplankton, Mar. Ecol. Progr. Ser., 2000, 196, 127–141 Search PubMed.
-
B. B. Prezelin, M. A. Moline and H. A. Matlick, in Antarctic Sea Ice Biological Processes, Interactions, and Variability,Antarctic Research Series, Vol. 73, eds. M. P. Lizotte and K. R. Arrigo, American Geophysical Union, Washington D.C., 1998, pp. 45–83 Search PubMed.
- P. S. Huovinen, A. O. J. Oikari, M. R. Soimasuo and G. N. Cherr, Impact of UV radiation on the early development of the giant kelp (Macrocystis pyrifera) gametophytes, Photochem. Photobiol., 2000, 72, 308–313 Search PubMed.
-
U. Karsten, D. Hanelt, K. Bischof, H. Tüg, P. E. M. Brouwer and C. Wiencke, in The Arctic and Global Change, Proceedings from the Fourth Ny-Alesund Seminar, eds. R. CasacchiaH. Koutsileos, M. Morbidoni, P. D. Petrelli, M. R. Pettersen, R. Salvatori, R. Sparapani and E. S. Larsen, Ravello, Italy, 1998, pp. 177–180 Search PubMed.
- V. Dethlefsen, H. von Westernhagen, H. Tüg, P. D. Hansen and H. Dizer, Influence of solar ultraviolet-B on pelagic fish embryos: osmolality, mortality and viable hatch, Helgol. Mar. Res., 2001, 55, 45–55 Search PubMed.
- J. H. M. Kouwenberg, H. I. Browman, J. J. Cullen, R. F. Davis, J.-F. St-Pierre and J. A. Runge, Biological weighting of ultraviolet (280–400 nm) induced mortality in marine zooplankton and fish.
I. Atlantic cod (Gadus morhua) eggs, Mar. Biol., 1999, 134, 269–284 CrossRef.
- J. H. M. Kouwenberg, H. I. Browman, J. A. Runge, J. J. Cullen, R. F. Davis and J.-F. St-Pierre, Biological weighting of ultraviolet (280–400 nm) induced mortality in marine zooplankton and fish. II. Calanus finmarchicus
(Copepoda) eggs, Mar. Biol., 1999, 134, 285–293 CrossRef.
- D.-P. Häder, H. D. Kumar, R. C. Smith and R. C. Worrest, Effects on aquatic ecosystems, J. Photochem. Photobiol., B, 1998, 46, 53–68 Search PubMed.
- H. I. Browman, C. A. Rodriguez, F. Bèland, J. J. Cullen, R. F. Davis, J. H. M. Kouwenberg, P. S. Kuhn, B. McArthur, J. A. Runge, J.-F. St-Pierre and R. D. Vetter, Impact of ultraviolet radiation on marine crustacean zooplankton and ichthyoplankton: a synthesis of results from the estuary and Gulf of St. Lawrence, Canada, Mar. Ecol. Prog. Ser., 2000, 199, 293–311 Search PubMed.
-
D. F. Gleason, in Ecosystems, Evolution and Ultraviolet Radiation, eds. C. S. Cockell and A. R. Blaustein, Springer-Verlag, New York, 2001, pp. 118–149 Search PubMed.
- T. Takahashi, R. A. Feely, R. F. Weiss, R. H. Wanninkhof, D. W. Chipman, S. C. Sutherland and T. T. Takahashi, Global air–sea flux of CO2: an estimate based on measurements of sea–air pCO2 difference, Proc. Natl. Acad. Sci. USA, 1997, 94, 8292–8299 CrossRef CAS.
- R. Sommaruga and A. G. J. Buma, UV-induced cell damage is species-specific among aquatic phagotrophic protists, J. Eukar. Microbiol., 2000, 47, 450–455 Search PubMed.
- M. E. Feder, A. F. Bennett and R. B. Huey, Evolutionary physiology, Ann. Rev. Ecol. Syst., 2000, 31, 315–341 Search PubMed.
- D. Tilman, The ecological consequences of changes in biodiversity: a search for general principles, Ecology, 1999, 80, 1455–1474 Search PubMed.
- D. S. Woodruff, Declines of biomes and biotas and the future of evolution, Proc. Natl. Acad. Sci. USA, 2001, 98, 5471–5476 CrossRef CAS.
- P. G. Falkowski, R. T. Barber and V. Smetacek, Biogeochemical controls and feedbacks on ocean primary production, Science, 1998, 281, 200–206 CrossRef CAS.
- J. Hilty and A. Merenlender, Faunal indicator taxa selection for monitoring ecosystem health, Biol. Cons., 2000, 92, 185–197 Search PubMed.
- S. Naeem, F. S. Chapin III, R. Costanza, P. R. Ehrlich, F. B. Golley, D. U. Hooper, J. H. Lawton, R. V. O'neill, H. A. Mooney, O. E. Sala, A. J. Symstad and D. Tilman, Biodiversity and ecosystem functioning: maintaining natural life support processes, Issues Ecol., 1999, 4, 1–14 Search PubMed.
- K. Reid and J. P. Croxall, Environmental response of upper trophic-level predators reveals a system change in an Antarctic marine ecosystem, Proc. R. Soc. Lond. B, 2001, 268, 377–384 CrossRef CAS.
- J. E. Frederick, Z. Qu and C. R. Booth, Ultraviolet radiation at sites on the Antarctic coast, Photochem. Photobiol., 1998, 68, 183–190 Search PubMed.
- A. E. Waibel, T. Peter, K. S. Carslaw, H. Oelhaf, G. Wetzel, P. J. Crutzen, U. Pöschl, A. Tsias, E. Reimer and H. Fischer, Arctic ozone loss due to denitrification, Science, 1999, 283, 2064–2069 CrossRef CAS.
- M. Lebert, M. Schuster and D.-P. Häder, The European Light Dosimeter Network: four years of measurements, J. Photochem. Photobiol., B, 2002, 66, 81–87 Search PubMed.
-
EC, European research in the stratosphere 1996–2000, Vol. EUR 19867, European Commission, 2001 Search PubMed.
- C. S. Zerefos, C. Meleti, D. S. Balis, A. F. Bais and D. Gillotay, On changes of spectral UV-B in the 90's in Europe, Adv.
Space Res., 2000, 26, 1971–1978 CrossRef CAS.
- D.-P. Häder, M. Lebert, R. Marangoni and G. Colombetti, ELDONET—European Light Dosimeter Network hardware and software, J. Photochem. Photobiol., B, 1999, 52, 51–58 Search PubMed.
- A. A. Sabziparvar, K. P. Shine and P. M. F. de Forster, A model-derived global climatology of UV irradiation at the earth's surface, Photochem. Photobiol., 1999, 69, 193–202 Search PubMed.
- I. Laurion, M. Ventura, J. Catalan, R. Psenner and R. Sommaruga, Attenuation of ultraviolet radiation in mountain lakes: factors controlling the among- and within-lake variability, Limnol. Oceanogr., 2000, 45, 1274–1288 Search PubMed.
- P. Kuhn, H. Browman, B. McArthur and J.-F. St-Pierre, Penetration of ultraviolet radiation in the waters of the estuary and Gulf of St. Lawrence, Limnol. Oceanogr., 1999, 44, 710–716 Search PubMed.
- D. Conde, L. Aubriot and R. Sommaruga, Changes in UV penetration associated with marine intrusions and freshwater discharge in a shallow coastal lagoon of the Southern Atlantic Ocean, Mar. Ecol. Prog. Ser., 2000, 207, 19–31 Search PubMed.
- M. J. Dring, A. Wagner, L. A. Franklin, R. Kuhlenkamp and K. Lüning, Seasonal and diurnal variations in ultraviolet-B and ultraviolet-A irradiances at and below the sea surface at Helgoland (North Sea) over a 6-year period, Helgol. Mar. Res., 2001, 55, 3–11 Search PubMed.
- V. S. Kuwahara, H. Ogawa, T. Toda, T. Kikuchi and S. Taguchi, Variability of bio-optical factors influencing the seasonal attenuation of ultraviolet radiation in temperate coastal waters of Japan, Photochem. Photobiol., 2000, 72, 193–199 Search PubMed.
- M. T. Arts, R. D. Robarts, F. Kasai, M. J. Waiser, V. P. Tumber, A. J. Plante, H. Rai and H. J. de Lange, The attenuation of ultraviolet radiation in high dissolved organic carbon waters of wetlands and lakes on the northern Great Plains, Limnol. Oceanogr., 2000, 45, 292–299 Search PubMed.
- H. E. Zagarese, M. Diaz, F. Pedrozo, M. Ferraro, W. Cravero and B. Tartarotti, Photodegradation of natural organic matter exposed to fluctuating levels of solar radiation, J. Photochem. Photobiol., B, 2001, 61, 35–45 Search PubMed.
-
G. J. Herndl, J. M. Arrieta, I. Obernosterer and C. Pausz, in Role of Solar UV-B Radiation on Ecosystems,Ecosystem Research Report No. 30, eds. C. V. Nolan and D.-P. Häder, European Communities, Belgium, 1998, pp. 69–77 Search PubMed.
-
D. O. Hessen and P. J. Faerovig, in Plant Ecology. Special Issue: Responses of Plants to UV-B Radiation, Vol. 154, eds. J. Rozema, Y. Manetas and L. O. Björn, Kluwer Academic Publishers, Dordrecht, Boston, London, 2001, pp. 263–273 Search PubMed.
- R. F. Whitehead, S. deMora, S. Demers, M. Gosselin, P. Monfort and B. Mostajir, Interactions of ultraviolet-B radiation, mixing, and biological activity on photobleaching of natural chromophoric dissolved organic matter: A mesocosm study, Limnol. Oceanogr., 2000, 45, 278–291 Search PubMed.
- H. Piazena, E. Perez-Rodrigues, D.-P. Häder and F. Lopez-Figueroa, Penetration of solar radiation into the water column of the central subtropical Atlantic Ocean—optical properties and possible biological consequences, Deep-Sea Res. II, 2002, 49, 3513–3528 Search PubMed.
- R. Sommer, A. Cabaj, T. Sandu and M. Lhotsky, Measurement of UV radiation using suspensions of microorganisms, J. Photochem. Photobiol., B, 1999, 53, 1–6 Search PubMed.
-
K. Koussoulaki, D. Danielidis, D.-P. Häder and R. Santas, Assessment of Euglena gracilis as a biological dosimeter for solar UVA and UVB under field conditions. Proceedings of the First Internet Conference on Photochemistry and Photobiology. Reviewed publication, in Proceeding of the First InternetConference on Photochemistry and Photobiology, 1998 Search PubMed.
- Y. Huot, W. H. Jeffrey, R. F. Davis and J. J. Cullen, Damage to DNA in bacterioplankton: a model of damage by ultraviolet radiation and its repair as influenced by vertical mixing, Photochem. Photobiol., 2000, 72, 62–74 Search PubMed.
- T. Douki, M. Court, S. Sauvaigo, F. Odin and J. Cadet, Formation of the main UV-induced thymine dimeric lesions within isolated and cellular DNA as measured by high performance liquid chromatography-tandem mass spectrometry, J. Biol. Chem., 2000, 275, 11678–11685 CrossRef CAS.
- T. Douki and J. Cadet, Individual determination of the yield of the main UV-induced dimeric pyrimidine photoproducts in DNA suggests a high mutagenicity of CC photolesions, Biochemistry, 2001, 40, 2495–2501 CrossRef CAS.
- F. Thoma, Light and dark in chromatin repair: repair of UV-induced DNA lesions by photolyase and nucleotide excision repair, Embo J., 1999, 18, 6585–6598 CrossRef CAS.
- C. Winter, M. M. Moeseneder and G. J. Herndl, Impact of UV radiation on bacterioplankton community composition, Appl. Environ. Microbiol., 2001, 67, 665–672 CrossRef CAS.
- M. Radman, F. Taddei and I. Matic, DNA repair systems and bacterial evolution, Cold Spring Harbor Symp. Quant. Biol., 2000, 65, 1–9 Search PubMed.
- A. Vaishampayan, R. P. Sinha, D.-P. Häder, T. Dey, A. K. Gupta, U. Bhan and A. L. Rao, Cyanobacterial biofertilizers in rice agriculture, Bot. Rev., 2002, 67, 453–516 Search PubMed.
- C. Kruschel and R. W. Castenholz, The effect of solar UV and visible irradiance on the vertical movements of cyanobacteria in microbial mats of hypersaline waters, FEMS Microbiol. Ecol., 1998, 27, 53–72 CrossRef CAS.
-
M. A. Moran and R. G. Zepp, in Microbial Ecology of the Oceans, ed. D. L. Kirchman, Wiley-Liss Inc., New York, 2000, pp. 201–228 Search PubMed.
- R. P. Sinha, M. Klisch, A. Gröniger and D.-P. Häder, Responses of algae and cyanobacteria to solar UV-B, Plant Ecol., 2001, 154, 189–204 Search PubMed.
-
R. P. Sinha and D.-P. Häder, in Advances in Phycology, eds. B. N. Verma, A. N. Kargupta and S. K. Goyal, APC Publ., New Delhi, 1998, pp. 71–80 Search PubMed.
- R. P. Sinha, M. Dautz and D.-P. Häder, A simple and efficient method for the quantitative analysis of thymine dimers in cyanobacteria, phytoplankton and macroalgae, Acta Protozool., 2001, 40, 187–195 Search PubMed.
- B. K. Kolli, S. Tiwari and P. Mohanty, Ultraviolet-B induced damage to photosystem II in intact filaments of Spirulina platensis, Z. Naturf., 1998, 53c, 369–377 Search PubMed.
- D. Campbell, M.-J. Eriksson, G. Öquist, P. Gustafsson and A. K. Clarke, The cyanobacterium Synechococcus resists UV-B by exchanging photosystem II reaction-center D1 proteins, Proc. Natl. Acad. Sci. USA, 1998, 95, 364–369 CrossRef CAS.
- T. Han, R. P. Sinha and D.-P. Häder, UV-A/blue light-induced reactivation of photosynthesis in UV-B irradiated cyanobacterium, Anabaena sp., J. Plant Physiol., 2001, 158, 1403–1413 Search PubMed.
- S. W. Hansucker, B. M. Tissue, M. Potts and R. F. Helm, Screening protocol for the ultraviolet-photoprotective pigment scytonemin, Analyt. Biochem., 2001, 288, 227–230 Search PubMed.
- D. D. Wynn-Williams, H. G. M. Edwards and F. Garcia-Pichel, Functional biomolecules of Antarctic stromatolitic and endolithic cyanobacterial communities, Eur. J. Phycol., 1999, 34, 381–391 Search PubMed.
- S. Steiger, L. Schäfer and G. Sandmann, High-light-dependent upregulation of carotenoids and their antioxidative properties in the cyanobacterium Synechocystis PCC 6803, J. Photochem. Photobiol., B, 1999, 52, 14–18 Search PubMed.
- A. Canini, D. Leonardi and M. G. Caiola, Superoxide dismutase activity in the cyanobacterium Microcystis aeruginosa after bloom formation, New Phytol., 2001, 152, 107–116 Search PubMed.
- T. Lindahl and R. D. Wood, Quality control by DNA repair, Science, 1999, 286, 1897–1905 CrossRef CAS.
- R. Araoz and D.-P. Häder, Enzymatic antioxidant activity in two cyanobacteria species exposed to solar radiation, Recent Res. Devel. Photochem. Photobiol., 1999, 3, 123–132 Search PubMed.
- R. Sommaruga and F. Garcia-Pichel, UV-absorbing mycosporine-like compounds in planktonic and benthic organisms from a high-mountain lake, Arch. Hydrobiol., 1999, 144, 255–269 Search PubMed.
- R. P. Sinha, J. P. Sinha, A. Gröniger and D.-P. Häder, Polychromatic action spectrum for the induction of a mycosporine-like amino acid in a rice-field cyanobacterium, Anabaena sp., J. Photochem. Photobiol., B, 2002, 66, 47–53 Search PubMed.
- J. Rozema, L. O. Björn, J. F. Bornman, A. Gaberšèik, D.-P. Häder, T. Trošt, M. Germ, M. Klisch, A. Gröniger, R. P. Sinha, M. Lebert, Y.-Y. He, R. Buffoni-Hall, N. V. J. de Bakker, J. van de Staaij and B. B. Meijkamp, The role of UV-B radiation in aquatic and terrestrial ecosystems—an experimental and functional analysis of the evolution of UV-absorbing compounds, J. Photochem. Photobiol., B, 2002, 66, 2–12 Search PubMed.
- A. Yamazawa, H. Takeyama, D. Takeda and T. Matsunaga, UV-A-induced expression of GroEL in the UV-A-resistant marine cyanobacterium Oscillatoria sp. NKBG 091600, Microbiol., 1999, 145, 949–954 Search PubMed.
- A. Gröniger, R. P. Sinha, M. Klisch and D.-P. Häder, Photoprotective compounds in cyanobacteria, phytoplankton and macroalgae—a database, J. Photochem. Photobiol., B, 2000, 58, 115–122 Search PubMed.
- A. L. George, A. W. Murray and P. O. Montiel, Tolerance of Antarctic cyanobacterial mats to enhanced UV radiation, FEMS Microbiol. Ecol., 2001, 37, 91–101 CrossRef CAS.
- L. C. Rai, B. Tyagi, P. K. Rai and N. Mallick, Interactive effects of UV-B and heavy metals (Cu and Pb) on nitrogen and phosphorus metabolism of a N2-fixing cyanobacterium Anabaena doliolum, Environ. Exp. Bot., 1998, 39, 221–231 CrossRef CAS.
- P. Falkowski, The ocean's invisible forest, Sci. Am., 2002,(August), 38–45 Search PubMed.
- S.-A. Wängberg, J.-S. Selmer and K. Gustavson, Effects of UV-B radiation on carbon and nutrient dynamics in marine plankton communities, J. Photochem. Photobiol., B, 1998, 45, 19–24 Search PubMed.
- M. S. Estevez, G. Malanga and S. Puntarulo, UV-B effects on Antarctic Chlorella sp. cells, J. Photochem. Photobiol., B, 2001, 62, 19–25 Search PubMed.
- W. W. Gregg and M. E. Conkwright, Decadal changes in global ocean chlorophyll, Geophys. Res. Lett., 2002, 29 Search PubMed.
- F. Ghetti, H. Hermann, D.-P. Häder and H. K. Seidlitz, Spectral dependence of the inhibition of photosynthesis under simulated global radiation in the unicellular green alga Dunaliella salina, J. Photochem. Photobiol., B, 1999, 48, 166–173 Search PubMed.
- M. Klisch and D.-P. Häder, Effects of solar radiation on phytoplankton, Recent Res. Devel. Photochem. Photobiol., 1999, 3, 113–121 Search PubMed.
- W. M. Bandaranayake, Mycosporines: are they nature's sunscreens?, Nat. Prod. Rep., 1998, 59–172 RSC.
- N. Noguchi, A. Watanabe and H. Shi, Diverse functions of antioxidants, Free Rad. Res., 2000, 33, 809–817 Search PubMed.
- S. W. Jeffrey, H. S. MacTavish, W. C. Dunlap, M. Vesk and K. Groenewoud, Occurrence of UVA- and UVB-absorbing compounds in 152 species (206 strains) of marine microalgae, Mar. Ecol. Progr. Ser., 1999, 189, 35–51 Search PubMed.
- M. Klisch, R. P. Sinha, P. R. Richter and D.-P. Häder, Mycosporine-like amino acids (MAAs) protect against UV-B-induced damage in Gyrodinium dorsum Kofoid, J. Plant Physiol., 2001, 158, 1449–1454 Search PubMed.
- A. C. Sigleo, P. J. Neale and A. M. Spector, Phytoplankton pigments at the Weddell-Scotia confluence during the 1993 austral spring, J. Plankt. Res., 2000, 22, 1989–2006 Search PubMed.
- A. M. Chekalyuk, F. E. Hoge, C. W. Wright, R. N. Swift and J. K. Yungel, Airborne
test of laser pump-and-probe technique for assessment of phytoplankton photochemical characteristics, Photosynth. Res., 2000, 66, 45–56 CrossRef CAS.
- D. Karentz and H. J. Spero, Response of a natural Phaeocystis population to ambient fluctuations of UVB radiation caused by Antarctic ozone depletion, J. Plankt. Res., 1995, 17, 1771–1789 Search PubMed.
-
A. T. Davidson and H. J. Marchant, in Antarctic Research Series. Ultraviolet Radiation in Antarctica: Measurements and Biological Effects, Vol. 62, eds. C. S. Weiler and P. A. Penhale, 1994, pp. 187–205 Search PubMed.
- T. A. Moisan and B. G. Mitchell, UV absorption by mycosporine-like amino acids in Phaeocystis antarctica Karsten induced by photosynthetically available radiation, Mar. Biol., 2001, 138, 217–227 Search PubMed.
- F. Xiong, Evidence that UV-B tolerance of the photosynthetic apparatus in microalgae is related to the D1-turnover mediated repair cycle in vivo, J. Plant Physiol., 2001, 158, 285–294 Search PubMed.
- A. Kaplan, Y. Helman, D. Tchernov and L. Reinhold, Acclimation of photosynthetic microorganisms to changing ambient CO2 concentration, Proc. Natl. Acad. Sci. USA, 2001, 98, 4817–4818 CrossRef CAS.
- P. J. Neale, Modeling the effects of ultraviolet radiation on estuarine phytoplankton production: impact of variations in exposure and sensitivity to inhibition, J. Photochem. Photobiol., B, 2001, 62, 1–8 Search PubMed.
- O. Arino, K. Boushaba and A. Boussouar, Modelization of the role of currents and turbulence on the growth and dispersion of marine phytoplankton, C. R. Acad. Sci. Paris, Sciences de la vie/ Life Sciences, 2000, 323, 113–118 Search PubMed.
- V. E. Villafañe, E. W. Helbling and H. E. Zagarese, Solar ultraviolet radiation and its impact on aquatic systems of Patagonia, South America, Ambio, 2001, 30, 112–117 Search PubMed.
- E. W. Helbling, V. E. Villafane, A. G. J. Buma, M. Andrade and F. Zaratti, DNA damage and photosynthetic inhibition by solar ultraviolet radiation in tropical phytoplankton (Lake Titicaca, Bolivia), Eur. J. Phycol., 2001, 36, 157–166 Search PubMed.
- I. Sobolev, Effect of column ozone on the variability of biologically effective UV radiation at high southern latitudes, Photochem. Photobiol., 2000, 72, 753–765 Search PubMed.
-
E. van Donk, B. A. Faafeng, H. J. de Lange and D. O. Hessen, in Plant Ecology. Special Issue: Responses of Plants to UV-B Radiation, Vol. 154, eds. J. Rozema, Y. Manetas and L. O. Björn, Kluwer Academic Publishers, Dordrecht, Boston, London, 2001, pp. 249–259 Search PubMed.
- C. W. Grobe and T. M. Murphy, Solar ultraviolet-B radiation effects on growth and pigment composition of the intertidal alga Ulva expansa
(Setch.) S. & G. (Chlorophyta), J. Exp. Mar. Biol. Ecol., 1998, 225, 39–51 CrossRef CAS.
- M. J. Dring, A. Wagner and K. Lüning, Contribution of the UV component of natural sunlight to photoinhibition of photosynthesis in six species of subtidal brown and red seaweeds, Plant Cell Environ., 2001, 24, 1153–1164 CrossRef CAS.
-
D.-P. Häder, in Landmarks in Photobiology, Proceedings of the 12th Congress on Photobiology (ICP '96), OEMF spa, Italy, eds. H. Hönigsmann, R. M. Knobler, F. Trautinger and G. Jori, Milano, 1998, pp. 31–33 Search PubMed.
- A. Gröniger, C. Hallier and D.-P. Häder, Influence of UV radiation and visible light on Porphyra umbilicalis: photoinhibition and MAA concentration, J. Appl. Phycol., 1999, 11, 437–445 Search PubMed.
- J. A. Segui, V. Maire, I. S. Gabashvili and M. Fragata, Oxygen evolution loss and structural transitions in photosystem II induced by low intensity UV-B radiation of 280 nm wavelength, J. Photochem. Photobiol., B, 2000, 56, 39–47 Search PubMed.
- W. H. van de Poll, A. Eggert, A. G. J. Buma and A. M. Breeman, Effects of UV-B-induced DNA damage and photoinhibition on growth of temperate marine red macrophytes: habitat-related differences in UV-B tolerance, J. Phycol., 2001, 37, 30–37 CrossRef CAS.
- D.-P. Häder, M. Lebert and E. W. Helbling, Effects of solar radiation on the Patagonian macroalga Enteromorpha linza
(L.) J. Agardh—Chlorophyceae, J. Photochem. Photobiol., B, 2001, 62, 43–54 Search PubMed.
- U. Karsten, K. Bischof and C. Wiencke, Photosynthetic performance of Arctic macroalgae after transplantation from deep to shallow waters, Oecologia, 2001, 127, 11–20 Search PubMed.
- M. Altamirano, A. Flores-Moya, F. Conde and F. L. Figueroa, Growth seasonality, photosynthetic pigments, and carbon and nitrogen content in relation to environmental factors: a field study of Ulva olivascens
(Ulvales, Chlorophyta), Phycologia, 2000, 39, 50–58 Search PubMed.
- M. L. Quartino, H. Klöser, I. R. Schloss and C. Wiencke, Biomass and associations of benthic marine macroalgae from the inner Potter Cove (King George Island, Antarctica) related to depth and substrate, Polar Biol., 2001, 24, 349–355 Search PubMed.
- D. Hanelt, H. Tüg, K. Bischof, C. Groß, H. Lippert, T. Sawall and C. Wiencke, Light regime in an Arctic fjord: a study related to stratospheric ozone depletion as a basis for determination of UV effects on algal growth, Marine Biol., 2001, 138, 649–658 Search PubMed.
- U. Karsten and C. Wiencke, Factors controlling the formation of UV-absorbing mycosporine-like amino acids in the marine red alga Palmaria palmata from Spitsbergen (Norway), J. Plant Physiol., 1999, 155, 407–415 Search PubMed.
- F. R. Conde, M. S. Churio and C. M. Previtali, The photoprotector mechanism of mycosporine-like amino acids. Excited-state properties and photostability of porphyra-334 in aqueous solution, J. Photochem. Photobiol., B, 2000, 56, 139–144 Search PubMed.
- A. Gröniger and D.-P. Häder, Stability of mycosporine-like amino acids, Recent Res. Devel. Photochem. Photobiol., 2000, 4, 247–252 Search PubMed.
- A. Gröniger and D.-P. Häder, Induction of the synthesis of an UV-absorbing substance in the green alga Prasiola stipitata, J. Photochem. Photobiol., B, 2002, 66, 54–59 Search PubMed.
- L. A. Franklin, G. Kräbs and R. Kuhlenkamp, Blue light and UV-A radiation control the synthesis of mycosporine-like amino acids in Chondrus crispus
(Florideophyceae), J. Phycol., 2001, 37, 257–270 CrossRef CAS.
- E. Perez-Rodriguez, I. Gomez, U. Karsten and F. L. Figueroa, Effects of UV radiation on photosynthesis and excretion of UV-absorbing compounds of Dasycladus vermicularis
(Dasycladales, Chlorophyta) from southern Spain, Phycologia, 1998, 37, 379–387 Search PubMed.
- S. Coelho, J. W. Rijstenbil, I. Sousa-Pinto and M. T. Brown, Cellular responses to elevated light levels in Fucus spiralis embryos during the first days after fertilization, Plant Cell Environ., 2001, 24, 801–810 CrossRef CAS.
- C. Wiencke, I. Gómez, H. Pakker, A. Flores-Moya, M. Altamirano, D. Hanelt, K. Bischof and F. L. Figueroa, Impact of UV radiation on viability, photosynthetic characteristics and DNA of brown algal zoospores: implications for depth zonation, Mar. Ecol. Progr. Ser., 2000, 197, 217–229 Search PubMed.
- M. J. Dring, V. Makarov, E. Schoshina, M. Lorenz and K. Lüning, Influence of ultraviolet radiation on chlorophyll fluorescence and growth in different life history stages of three species of Laminaria
(Phaeophyta), Mar. Biol., 1996, 126, 183–191 CrossRef CAS.
- N. de Bakker, J. Rozema and R. Aerts, UV effects on a charophycean algae, Chara aspera, Plant Ecol., 2001, 154, 205–212 Search PubMed.
- M. P. Vega and R. A. Pizarro, Oxidative stress and defence mechanisms of the freshwater cladoceran Daphnia longispina exposed to UV radiation, J. Photochem. Photobiol., B, 2000, 54, 121–125 Search PubMed.
- S. C. Rhode, M. Pawlowski and R. Tollrian, The impact of ultraviolet radiation on the vertical distribution of zooplankton of the genus Daphnia, Nature, 2001, 412, 69–72 CrossRef CAS.
-
L. De Meester, P. Dawidowicz, E. van Gool and C. J. Loose,, eds. R. Tollrian and C. D. Harvell, Princeton University Press, Princeton, NJ, 1999, pp. 160–176.
- U. C. Storz and R. J. Paul, Phototaxis in water fleas (Daphnia magna) is differently influenced by visible and UV light, J. Comp. Physiol. A, 1998, 183, 709–717 Search PubMed.
- D. M. Leech and C. E. Williamson, In situ exposure to ultraviolet radiation alters the depth distribution of Daphnia, Limnol. Oceanogr., 2001, 46, 416–420 Search PubMed.
- B. Tartarotti, I. Laurion and R. Sommaruga, Large variability in the concentration of mycosporine-like amino acids among zooplankton from lakes located across an altitude gradient, Limnol. Oceanogr., 2001, 46, 1546 Search PubMed.
- C. A. Rodriguez, H. I. Browman, J. A. Runge and J.-F. St-Pierre, Impact of solar ultraviolet radiation on hatching of a marine copepod, Calanus finmarchicus, Mar. Ecol. Prog. Ser., 2000, 193, 85–93 Search PubMed.
- P. S. Kuhn, H. I. Browman, R. F. Davis, J. J. Cullen and B. L. McArthur, Modeling the effects of ultraviolet radiation on embryos of Calanus finmarchicus and Atlantic cod (Gadus morhua) in a mixing environment, Limnol. Oceanogr., 2000, 45, 1797–1806 Search PubMed.
- P. L. Stutzman, An examination of the potential effects of food limitation on the ultraviolet radiation (UVR) tolerance of Diaptomus minutus, Freshwater Biol., 2000, 44, 271–277 CrossRef.
- G. Grad, C. E. Williamson and D. M. Karapelou, Zooplankton survival and reproduction responses to damaging UV radiation: a test of reciprocity and photoenzymatic repair, Limnol. Oceanogr., 2001, 46, 584–591 Search PubMed.
- M. P. Lesser, J. H. Farrell and C. W. Walker, Oxidative stress, DNA damage and p53 expression in the larvae of Atlantic cod (Gadus morhua) exposed to ultraviolet (290–400 nm) radiation, J. Exp. Biol., 2001, 204, 157–164 Search PubMed.
- C. Gutiérrez-Rodrìguez and C. E. Williamson, Influence of solar ultraviolet radiation on early life-history stages of the bluegill sunfish, Lepomis macrochirus, Environ. Biol. Fish., 1999, 55, 307–319 Search PubMed.
- M. S. Ewing, V. S. Blazer, D. L. Fabacher, E. E. Little and K. M. Kocan, Channel catfish response to ultraviolet-B radiation, J. Aquat. Anim. Health, 1999, 11, 192–197 Search PubMed.
- C. A. Rodriguez, H. I. Browman and J.-F. St-Pierre, High survival of neustonic zoea I larvae of American lobster Homarus americanus following short-term exposure to ultraviolet radiation (280 to 400 nm), Mar. Ecol. Progr. Ser., 2000, 193, 305–309 Search PubMed.
- R. A. Alford, P. M. Dixon and J. H. K. Pechmann, Global amphibian population declines, Nature, 2001, 412, 499–500 CrossRef CAS.
- J. E. Houlahan, C. S. Findlay, B. R. Schmidt, A. H. Meyer and S. L. Kuzmin, Quantitative evidence for global amphibian population declines, Nature, 2001, 404, 752–755 CrossRef.
- J. M. Kiesecker, A. R. Blaustein and L. K. Belden, Complex causes of amphibian population declines, Nature, 2001, 410, 681–683 CrossRef CAS.
- R. Hofer and C. Mokri, Photoprotection in tadpoles of the common frog, Rana temporaria, J. Photochem. Photobiol., B, 2000, 59, 48–53 Search PubMed.
- M. Pahkala, A. Laurila and J. Merilä, Carry-over effects of ultraviolet-B radiation on larval fitness in Rana temporaria, Proc. R. Soc. London, Ser. B, 2001, 268, 1699–1706 Search PubMed.
- M. Pahkala, A. Laurila and J. Merilä, Ambient ultraviolet-B radiation reduces hatchling size in the common frog Rana temporaria, Ecography, 2000, 23, 531–538 CrossRef.
- I. N. Flamarique, K. Ovaska and T. M. Davis, UV-B induced damage to the skin and ocular system of amphibians, Biol. Bull., 2000, 199, 187–188 Search PubMed.
- G. T. Ankley, S. A. Diamond, J. E. Tietge, G. W. Holcombe, K. M. Jensen, D. L. Defoe and R. Peterson, Assessment of the risk of solar ultraviolet radiation to amphibians. I. Hindlimb malformations in the Northern Leopard frog (Rana pipiens), Environ. Sci. Technol., 2002, 36, 2853–2858 CrossRef CAS.
- G. S. Peterson, L. B. Johnson, R. P. Axler and S. A. Diamond, Assessment of the risk of solar ultraviolet radiation to amphibians. II. In situ characterization of exposure in amphibian habitats, Environ. Sci. Technol., 2002, 36, 2859–2865 CrossRef CAS.
- B. E. Brown, R. P. Dunne, M. S. Goodson and A. E. Douglas, Bleaching patterns in reef corals, Nature, 2000, 404, 142–143 CrossRef CAS.
- N. Knowlton, Sea urchin recovery from mass mortality: new hope for Caribbean coral reefs?, Proc. Natl. Acad. Sci. USA, 2001, 98, 4822–4824 CrossRef CAS.
- A. Salih, A. Larkum, G. Cox, M. Kühl and O. Hoegh-Guldberg, Fluorescent pigments in corals are photoprotective, Nature, 2000, 408, 850–853 CrossRef CAS.
- M. Scheffer, S. Carpenter, J. A. Foley, C. Folke and B. Walker, Catastrophic shifts in ecosystems, Nature, 2001, 413, 591–596 CrossRef CAS.
- B. Olesen and S. C. Maberly, The effect of high levels of visible and ultra-violet radiation on the photosynthesis of phytoplankton from a freshwater lake, Arch. Hydrobiol., 2001, 151, 301–315 Search PubMed.
- K. Rogers, A. Schmidt, J. Wilkinson and T. Merz, Effects of incident UV-B radiation on periphyton in four alpine freshwater ecosystems in central Colorado: impacts on boreal toad tadpoles (Bufo boreas), J. Freshwater Ecol., 2001, 16, 283–301 Search PubMed.
- C. E. Williamson, D. P. Morris, M. L. Pace and O. G. Olson, Dissolved organic carbon and nutrients as regulators of lake ecosystems: resurrection of a more integrated paradigm, Limnol. Oceanogr., 1999, 44, 795–803 Search PubMed.
- C. E. Williamson, B. R. Hargreaves, P. S. Orr and P. A. Lovera, Does UV play a role in changes in predation and zooplankton community structure in acidified lakes?, Limnol. Oceanogr., 1999, 44, 774–783 Search PubMed.
- R. C. Smith, K. S. Baker, H. M. Dierssen, S. E. Stammerjohn and M. Vernet, Variability of primary production in an Antarctic marine ecosystem as estimated using muli-scale sampling strategy, Am. Zool., 2001, 41, 40–56 Search PubMed.
- R. C. Smith and S. E. Stammerjohn, Variations of surface air temperature and sea-ice extent in the western Antarctic Peninsula region, Ann. Glaciol., 2001, 33, 493–500 Search PubMed.
- W. C. Quayle, L. S. Peck, H. Peat, J. C. Ellis-Evans and P. R. Harrigan, Extreme responses to climate change in Antarctic lakes, Science, 2002, 295, 645 CrossRef CAS.
-
S. de Mora, S. Demers and M. Vernet, The effect of UV radiation in the marine environment, Vol. 10, Cambridge University Press, Cambridge, 2000 Search PubMed.
- R. C. Smith, B. B. Prezelin, K. S. Baker, R. R. Bidigare, N. P. Boucher, T. Coley, D. Karentz, S. MacIntyre, H. A. Matlick, D. Menzies, M. Ondrusek, Z. Wan and K. J. Waters, Ozone depletion: ultraviolet radiation and phytoplankton biology in Antarctic waters, Science, 1992, 255, 952–959 CAS.
-
J. Meador, W. H. Jeffrey, J. P. Kase, J. D. Pakulski, S. Chiarello and D. L. Mitchell, Seasonal fluctuation of DNA photodamage at Palmer Station, Antarctica, UNEP 2002 Search PubMed.
- W. H. Jeffrey, R. J. Pledger, P. Aas, S. Hager, R. B. Coffin, R. Von Haven and D. L. Mitchell, Diel and depth profiles of DNA photodamage in bacterioplankton exposed to ambient solar ultraviolet radiation, Mar. Ecol. Prog. Ser., 1996, 137, 283–291 Search PubMed.
-
A. Dahlback, in UV Radiation and Arctic Ecosystems. Ecological Studies, Vol. 153 ed.: D. Hessen, Springer-Verlag, Berlin, Heidelberg, 2002, pp. 3–22 Search PubMed.
-
E. Aas, J. Høkedal, N. K. Højerslev, R. Sanvik and E. Sakshaug, in UV Radiation and Arctic Ecosystems. Ecological Studies, Vol. 153 ed.: D. Hessen, Springer-Verlag, Berlin, Heidelberg, 2002, pp. 23–56 Search PubMed.
-
W. E. Helbling and V. E. Villafañe, in UV Radiation and Arctic Ecosystems. Ecological Studies, Vol. 153 ed.: D. Hessen, Springer-Verlag, Berlin, Heidelberg, 2002, pp. 204–226 Search PubMed.
-
K. Bischof, D. Hanelt and C. Wiencke, in UV Radiation and Arctic Ecosystems. Ecological Studies, Vol. 153 ed.: D. Hessen, Springer Verlag, Berlin, Heidelberg, 2002, pp. 227–243 Search PubMed.
-
D. K. Perovich, in UV Radiation and Arctic Ecosystems. Ecological Studies, Vol. 153 ed.: D. Hessen, Springer-Verlag, Berlin, Heidelberg, 2002, pp. 73–89 Search PubMed.
-
W. F. Vincent and C. Belzile, in UV Radiation and Arctic Ecosystems. Ecological Studies, Vol. 153 ed.: D. Hessen, Springer-Verlag, Berlin, Heidelberg, 2002, pp. 137–155 Search PubMed.
- R. Sommaruga, The role of solar UV radiation in the ecology of alpine lakes, J. Photochem. Photobiol., B, 2001, 62, 35–42 Search PubMed.
-
W. F. Vincent, in The Ecology of Cyanobacteria, eds. B. A. Whitton and M. Potts, Kluwer Academic Publishers, 2000, pp. 321–340 Search PubMed.
- G. Weller, Regional impacts of climate change in the Arctic and Antarctic, Ann. Glac., 1998, 27, 543–552 Search PubMed.
- J. J. Magnuson, D. M. Robertson, B. J. Benson, R. H. Wynne, D. M. Livingstone, T. Arai, R. A. Assel, R. G. Barry, V. Card, E. Kuusisto, N. G. Granin, T. D. Prowse, K. M. Stewart and V. S. Vuglinski, Historical trends in lake and river ice cover in the Northern hemisphere, Science, 2000, 289, 1743–1746 CrossRef CAS.
- R. D. Vinnebrooke and P. R. Leavitt, Phytobenthos and phytoplankton as potential indicators of climate change in mountain lakes and ponds: an HPLC-based pigment approach, J. North Am. Benth. Assoc., 1999, 10, 1065–1081 Search PubMed.
-
D. Hessen, UV Radiation and Arctic Ecosystems. Ecological Studies, Vol. 153, Springer-Verlag, Berlin, Heidelberg, 2002 Search PubMed.
- D. O. Hessen and N. A. Rukke, Increased UV-susceptibility in Daphnia at low calcium concentrations, Limnol. Oceanogr., 2000, 45, 1834–1838 Search PubMed.
- R. Pienitz and W. F. Vincent, Effect of climate change relative to ozone depletion on UV exposure in subarctic lakes, Nature, 2000, 404, 484–487 CrossRef CAS.
- R. T. Watson, M. C. Zinyowera and R. H. Moss, IPCC Special Report on the Regional Impacts of Climate Change: An Assessment of Vulnerability, Intergovernmental Panel on Climate Change (IPCC) Report No..
- D. Karentz and I. Bosch, Influence of ozone-related increases in ultraviolet radiation on Antarctic marine organisms, Am. Zool., 2001, 41, 3–16 Search PubMed.
-
H. D. Kumar and D.-P. Häder, Global Aquatic and Atmospheric Environment, Springer-Verlag, Berlin, Heidelberg, New York, 1999 Search PubMed.
- J. Staehelin, N. R. P. Harris, C. Appenzeller and J. Eberhard, Ozone trends: a review, Rev. Geophys., 2001, 39, 231–290 Search PubMed.
- S. A. Montzka, J. H. Butler and L. T. Lock, Present and future trends in the atmospheric burden of ozone-depleting halogens, Nature, 1999, 398, 690–694 CrossRef CAS.
- D. L. Hartmann, J. M. Wallace, V. Limpasuvan, D. W. J. Thompson and J. R. Holton, Can ozone depletion and global warming interact to produce rapid climate change, Proc. Natl. Acad. Sci. USA, 2000, 97, 1412–1417 CrossRef CAS.
- W. J. Randel and F. Wu, Cooling of the Arctic and Antarctic polar stratospheres due to ozone depletion, J. Climate, 1999, 12, 1467–1479 Search PubMed.
- W. F. Vincent, I. Laurion and R. Pienitz, Arctic and Antarctic lakes as optical indicators of global change, Ann. Glac., 1998, 27, 691–696 Search PubMed.
- J. R. Petit, J. Jouzel, D. Raynaud, N. I. Barkov, J.-M. Barnola, I. Basile, M. Benders, J. Chappellaz, M. Davis, G. Delaygue, M. Delmotte, V. M. Kotlyakov, M. Legrand, V. Y. Lipenkov, C. Lorius, L. Pepin, C. Ritz, E. Saltzman and M. Stievenard, Climate and atmospheric history of the past 420,000 years from the Vostok ice core, Antarctica, Nature, 1999, 399, 429–436 CrossRef CAS.
- K. Alverson and F. Oldfield, Pages—past global changes and their significance for the future: an introduction, Quat. Sci. Rev., 2000, 19, 3–7 CrossRef.
- S. D. Emslie, W. Fraser, R. C. Smith and W. Walker, Abandoned penguin colonies and environmental change in the Palmer Station area, Anvers Island, Antarctic Peninsula, Antarctic Sci., 1998, 10, 257–268 Search PubMed.
- M. van den Broeke, On the interpretation of Antarctic temperature trends, J. Climate, 2000, 13, 3385–3389 Search PubMed.
- R. Bindschadler and P. Vornberger, Changes in the West Antarctic ice sheet since 1963 from declassified satellite photography, Science, 1998, 279, 689–692 CrossRef CAS.
- M. Simon, F. O. Glockner and R. Amann, Different community structure and temperature optima of heterotrophic picoplankton in various regions of the Southern Ocean, Aquat. Micro. Ecol., 1999, 18, 275–284 Search PubMed.
- S. L. Chown and K. J. Gaston, Exploring links between physiology and ecology at macroscales: the role of respiratory metabolism in insects, Biol. Rev., 1999, 74, 87–120 CrossRef.
- J. Cuzin-Roudy, Seasonal reproduction, multiple spawning, and fecundity in northern krill, Meganyctiphanes norvegica, and Antarctic krill, Euphausia superba, Can. J. Fish. Aquat. Sci., 2000, 57 suppl. 3, 6–15 CrossRef.
- H. M. Dierssen, R. C. Smith and M. Vernet, Glacial meltwater dynamics in coastal waters west of the Antarctic peninsula, Proc. Nat. Acad. Sci. USA, 2002, 99, 1790–1795 Search PubMed.
- C. W. Grobe, C. T. Ruhland and T. A. Day, A new population of Colobanthus quitensis near Arthur Harbor, Antarctica: correlating recruitment with warmer summer temperatures, Arctic Alpine Res., 1997, 29, 217–221 Search PubMed.
- D. H. Bromwich, B. Chen and K. M. Hines, Global atmospheric impacts induced by year-round open water adjacent to Antarctica, J. Geophys. Res. D, 1998, 103, 11173–11189 Search PubMed.
- B. S. Britton and R. F. Keeling, The influence of Antarctic sea ice on glacial—interglacial CO2 variations, Nature, 2000, 404, 171–174 CrossRef.
- R. G. Zepp, T. V. Callaghan and D. J. Erickson, Effects of enhanced solar ultraviolet radiation on biogeochemical cycles, J. Photochem. Photobiol., B, 1998, 46, 69–82 Search PubMed.
- P. Falkowski, R. J. Scholes, E. Boyle, J. Canadell, D. Canfield, J. Elser, N. Gruber, K. Hibbard, P. Högberg, S. Linder, F. T. Mackenzie, B. Moore III, T. Pedersen, Y. Rosenthal, S. Seitzinger, V. Smetacek and W. Steffen, The global carbon cycle: a test of our knowledge of earth as a system, Science, 2000, 290, 291–296 CrossRef CAS.
- P. G. Falkowski and Y. Rosenthal, Biological diversity and resource plunder in the geological record: casual correlations or causal relationships?, Proc. Natl. Acad. Sci. USA, 2001, 98, 4290–4292 CrossRef CAS.
- A. S. Brierley and J. L. Watkins, Effects of sea ice cover on the swarming behaviour of Antarctic krill, Euphausia superba, Can. J. Fish. Aquat. Sci., 2000, 57 (suppl. 3), 24–30 CrossRef.
- P. D. Tortell, Evolutionary and ecological perspectives on carbon acquisition in phytoplankton, Limnol. Oceanogr., 2000, 45, 744–750 Search PubMed.
- J. L. Sarmiento, T. M. C. Hughes, R. J. Stouffer and S. Manabe, Simulated response of the ocean carbon cycle to anthropogenic climate warming, Nature, 1998, 393, 245–249 CrossRef CAS.
- F. Louanchi and M. Hoppema, Interannual variations of the Antarctic Ocean CO2 uptake from 1986 to 1994, Mar. Chem., 2000, 72, 103–114 CrossRef CAS.
- J. Beardall, S. Beer and J. A. Raven, Biodiversity of marine plants in an era of climate change: some predictions based on physiological performance, Bot. Mar., 1998, 41, 113–123 Search PubMed.
- P. J. Neale, R. F. Davis and J. J. Cullen, Interactive effects of ozone depletion and vertical mixing on photosynthesis of Antarctic phytoplankton, Nature, 1998, 392, 585–589 CrossRef CAS.
- K. R. Arrigo, D. H. Robinson, D. L. Worthen, R. B. Dunbar, G. R. DiTullio, M. VanWoert and M. P. Lizotte, Phytoplankton community structure and the drawdown of nutrients and CO2 in the Southern Ocean, Science, 1999, 283, 365–367 CrossRef CAS.
Footnote |
† This article is published as part of the United Nations Environmental Programme: Environmental effects of ozone depletion and its interactions with climate change: 2002 assessment. |
|
This journal is © The Royal Society of Chemistry and Owner Societies 2003 |