DOI:
10.1039/B002950P
(Paper)
J. Mater. Chem., 2001,
11, 212-216
The widespread occurrence of negative thermal
expansion in zeolites†
Received
25th April 2000
, Accepted 29th June 2000
First published on 6th October 2000
Abstract
Powder X-ray and neutron diffraction studies of the calcined
siliceous zeolites ITQ-7, ITQ-9 and CIT-5, and the aluminophosphates
AlPO4-31 and Mg-doped AlPO4-17 have been
carried out as a function of temperature in order to determine their thermal
expansivity properties. Of these, ITQ-7, ITQ-9 and MAPO-17 show
negative thermal expansivity over a wide temperature range, in common with
several other previously studied microporous materials. CIT-5 and AlPO4-31
are anomalous in showing an expansion on heating. A detailed study of CIT-5
highlights the key structural parameters which determine its positive expansivity.
From expansivity data currently available on seventeen microporous materials,
it is clear that negative thermal expansivity must be considered the norm
rather than the exception. Positive expansivity is encouraged by the structure
having a relatively high framework density and a one-dimensional channel
system.
Introduction
There has recently been renewed interest in materials exhibiting the unusual
phenomenon of negative thermal expansion (NTE), i.e. materials
which contract on heating. This phenomenon arises, in general terms,
from a secondary structural or dynamic mechanism which overrides the normal
thermal expansivity of chemical bonds. In detail, the mechanism of this behaviour
is not yet understood for all the systems in which it occurs, but several
contributing effects have been highlighted, for example, ferroelectric (e.g.
PbTiO3) and magnetostrictive (e.g. Invar)
phase transitions and low-frequency phonon modes, specifically transverse
vibrations of two-coordinated atoms and cooperative motions of linked
quasi-rigid polyhedral units (so-called ‘rigid-unit modes’
or RUMs, for example, in β-quartz). An excellent introduction
to some of these phenomena, and examples of some of the materials exhibiting
them, has recently been given by Evans.1 Among
the materials exhibiting NTE, framework oxides represent a large sub-class;
mixed oxides such as ZrW2O8,2,3
ZrP2O74 and the Nasicon
or NZP family5,6 have recently been studied
in this respect. A further class of oxidic materials are the microporous framework
materials encompassing silicate zeolites and aluminophosphates. Our interest
in these materials arose from the theoretical predictions of NTE in zeolites
by Tschaufeser and Parker.7 Experimental verification
of NTE in some of these materials has been provided by Couves et al.8 for zeolite X, Park et al. for several pure
silica zeolites,9 Attfield and Sleight for
siliceous faujasite10 and AlPO4-17,11 and our own work on the pure silica polymorphs
ITQ-1, ITQ-3 and SSZ-2312 and
also siliceous chabazite and ITQ-4.13
In this paper we report further examples of NTE, determined by powder X-ray
and neutron diffraction methods, in the new SiO2 polymorphs ITQ-7
and ITQ-9 and in a magnesium-doped AlPO4-17 (MAPO-17)
phase, and contrast these with the observation of positive thermal expansivity
in the large-pore SiO2 polymorph CIT-5 and AlPO4-31.
In all of these systems, except zeolite X and MAPO-17, there are no extra-framework
cations or other species, and the frameworks themselves are electrostatically
neutral. The cumulative data so far point towards the conclusion that NTE
in microporous oxides is the norm rather than the exception.
Experimental
Pure polycrystalline samples of the SiO2 polymorphs ITQ-7,14 ITQ-915 and
CIT-516 were provided by Dr M. A. Camblor
and co-workers (Valencia). AlPO4-31 was prepared
by literature methods.17 A magnesium-substituted
AlPO4-17 derivative of framework composition Mg0.1Al0.9PO4
was prepared from a gel of composition 0.4R(OH)2∶0.1Mg(OAc)2∶0.9Al(OH)3∶H3PO4∶40H2O, heated at 190
°C
for 48 h {R represents the diquinuclidinium cation [(C7H13N)–(CH2)3–(NC7H13)]2+}.18 All samples were calcined in flowing O2
at 500
°C for several hours and subsequently stored under anhydrous
conditions.
Preliminary X-ray powder diffraction data were recorded in sealed 0.5 mm
glass capillaries on a Stoe STADI/P transmission-geometry diffractometer
using monochromated Cu-Kα1 radiation. For AlPO4-31,
additional high-temperature X-ray data were collected at 50
°C
intervals for 50
°C < T < 550
°C
over the 2θ range 7–45°. The variation of lattice
parameters versus temperature was determined by Rietveld refinement (GSAS
program19) using a fixed structural model (rhombohedral, R
20) and refining scale factor, lattice parameters,
detector zero-point, background and peak shape only. In the cases of the
remaining four samples, powder neutron diffraction data were used for the
thermal expansivity studies. Data for ITQ-7, CIT-5 and MAPO-17
were collected on the OSIRIS instrument21
at the ISIS facility of the CCLRC Rutherford Appleton Laboratory. Polycrystalline
samples weighing approximately 2 g were placed in vanadium cans. Measurements
were recorded at 30
°C and in 100
°C steps from 100
to 700
°C for CIT-5, at 50
°C and from 100 to 500
°C
in 100
°C steps for MAPO-17 and at 60 and 120
°C,
and from 200 to 600
°C in 100
°C steps for ITQ-7.
Problems with the incident neutron flux at 300
°C for ITQ-7
led to this measurement being discarded. A measurement of an empty can was
recorded and subtracted from the initial data before starting the refinements.
Refinements were carried out using the GSAS program. For both CIT-5
and ITQ-7, unit cell parameters, scale factor, background, peak shape (Gaussian
double exponential), atomic co-ordinates and isotropic temperature
factors, which were constrained by atom type, were refined. For the CIT-5
data, 57 variables and 4757 reflections over the range 1.0 to 7.0 Å
were refined, and for the ITQ-7 data, 59 variables and 1860 reflections
over the same d-spacing range. Soft constraints for Si–O (1.61 ± 0.005)
and O–O (2.58 ± 0.005) distances were used.
Due to poorer data quality, MAPO-17 refinements were of scale factor,
background and unit cell parameters only, using 7 variables and 4373 reflections
over the range 1.0 to 7.0 Å. For ITQ-9, data were collected
in a vanadium can on the POLARIS instrument at 20
°C, and from
200 to 600
°C in 100
°C steps. A background measurement
was subtracted. The same refinement strategy was used as in the CIT-5
and ITQ-7 experiments, although data from the low-angle, 90° and backscattering
banks were combined for greater precision. This resulted in an overall d-spacing
range of 0.7 to 8.3 Å and refinements using 104 variables and
6135 reflections.
Results and discussion
Some basic structural features of the zeolites under study are given in Table 1. It can be seen that the complexity
of these structures [in terms of the number of crystallographic variables,
or number of unique tetrahedral (T) atom sites] is relatively large.
For this reason, it was, unfortunately, not possible to carry out full unrestrained
refinements of all the crystallographic variables and derive reliable geometric
and thermal parameters. The most precise refinements were obtained in the
case of CIT-5, using the restrained model described above. In this case,
systematic trends can be seen in the derived structural parameters, which
will be further described later. However, the most important fundamental information
to be gained from these studies is the thermal expansivity data itself, in
terms of axial changes in the crystallographic cell parameters. These parameters
are derived very precisely and reliably from powder diffraction experiments.
Typical plots of unit cell parameters versus temperature for CIT-5
and MAPO-17 are shown in Fig. 1 and
2. In most cases, the axial coefficients of thermal expansivity (α)
were non-linear functions of temperature, but a reasonable feeling for
their behaviour can be obtained by approximating to a simpler linear fit.
Values of α for the relevant unit cell parameters, together
with those obtained for other microporous materials from earlier powder diffraction
experiments, are given in Table 2.
As can be seen from these figures, the vast majority of the materials studied
exhibit overall NTE throughout the temperature range studied, with most showing
NTE along all three crystallographic axes. Dramatically different behaviour
is, however, observed in the cases of CIT-5 and AlPO4-31,
which both show large positive axial α values. From Table 1, it can be seen that both of these
materials adopt one-dimensional channel systems and also have relatively
high framework densities; it is tempting to suggest that these may be significant
factors in determining their behaviour. Indeed, Tschaufeser and Parker7 originally predicted this possibility on the basis
of computational studies of the one-dimensional AlPO4 systems
AlPO4-5, AlPO4-8 and VPI-5. They suggested
that the relatively high framework density along the channel direction
would make NTE unfavourable. This also appears to be borne out by our previous
observation of a positive thermal expansivity along the channel axis (average αc ≈ +7 × 10−6 over the range 95 K < T < 510 K)
in the one-dimensional 12-MR system ITQ-4 (framework density ≈ 17.0),
which overall displays NTE.13 However, the
pitfalls in comparing simulations with experimental results must be borne
in mind; for example, although AlPO4-5 (AFI) was
predicted to show positive thermal expansivity,7
the experimental data of Park et al.9
demonstrate positive thermal expansivity only as high as 424 K, whence
strong NTE ensues (Table 2).
The temperature range over which the expansivity behaviour is studied, both
experimentally and theoretically, is a key factor—our recent results
on ITQ-4, for example, which extend the previously available data13 to higher temperatures, show a turnaround in the αc
coefficient from positive to negative. It is also interesting to note that,
for both AlPO4-31 and CIT-5 (Fig. 1),
the expansivity tends towards zero at the highest temperatures studied—this
corresponds to a reduction of the framework density of CIT-5 from 18.3
to 17.8, and of AlPO4-31 from 19.2 to 18.9 T atoms per
1000 Å3.
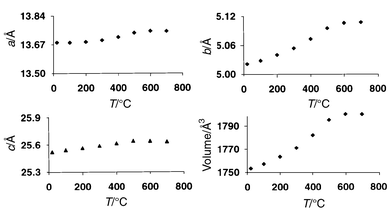 |
| Fig. 1
Thermal evolution of
lattice parameters for CIT-5.
| |
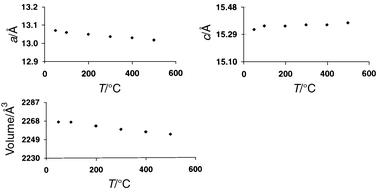 |
| Fig. 2
Thermal evolution of
lattice parameters for MAPO-17 (Note: for easy comparison of the
expansivites, all the graphs in Fig. 1 and
2 are plotted on the same percentage Δy/y-scale).
| |
Table 1
Salient structural data for the materials
studied
Material |
Typea |
Space group |
Channel systemb |
Approx. unit cell (a, b, c)/Å |
Unique T sites |
Framework densityc |
IZC structure code.26
nMR: n-membered
ring (i.e.n T-sites per ring).
No. of T sites per 1000 Å3.
|
AlPO4-31 |
ATO |
R![[3 with combining macron]](https://www.rsc.org/images/entities/char_0033_0304.gif) |
1D-12MR [001] |
20.8, 20.8, 5.0 (hexagonal) |
2 |
19.2 |
MAPO-17 |
ERI |
P63/m |
1D-12MR [001] |
13.1, 13.1, 15.3 |
4 |
15.9 |
ITQ-7 |
ISV |
P42/mmc |
3D-12MR |
12.8, 12.8, 25.2 |
5 |
15.4 |
ITQ-9 |
STF |
I![[1 with combining macron]](https://www.rsc.org/images/entities/char_0031_0304.gif) |
1D-10MR [001] |
14.7, 18.2, 7.4 |
8 |
17.2 |
CIT-5 |
CFI |
Im2a |
1D-14MR [010] |
13.7, 5.0, 25.5 |
5 |
18.3 |
Table 2
Known coefficients of thermal expansion for
a variety of microporous solidsa
Sample |
αa
b
|
αb
|
αc
|
αV
|
Range (T/K) |
F.D.c |
Reference |
The quoted values are
taken as the average linear expansivities—in some cases the true behaviour
is significantly non-linear.
αl
is defined as Δl/lΔT in units of 10−6 °C−1.
F.D.: framework density (no. of T sites per 1000 Å3)
at room temp.
|
ITQ-1 |
−4.23 |
−4.23 |
−3.21 |
−12.1 |
323–773 |
16.6 |
12
|
ITQ-3 |
−0.29 |
−2.06 |
−10.1 |
−11.4 |
323–823 |
16.2 |
12
|
ITQ-4 |
−11.5 |
−7.47 |
+7.19 |
−9.1 |
95–510 |
17.0 |
13
|
ITQ-7 |
−2.28 |
−2.28 |
−1.05 |
−5.6 |
473–873 |
15.4 |
This work |
ITQ-9 |
−5.58 |
−2.37 |
−2.19 |
−10.0 |
293–873 |
17.2 |
This work |
SSZ-23 |
−6.09 |
−3.21 |
−0.73 |
−10.3 |
323–773 |
16.7 |
12
|
MFI |
−5.5 |
−6.9 |
−2.8 |
−15.1 |
393–975 |
17.9 |
9
|
AFI |
−5.1 |
−5.1 |
−3.7 |
−14.5 |
424–774 |
17.6 |
9
|
DOH |
−0.6 |
−0.6 |
−3.1 |
−3.1 |
573–996 |
18.4 |
9
|
MTN |
−1.7 |
−1.7 |
−1.7 |
−5.0 |
463–1002 |
18.7 |
9
|
DDR |
−2.8 |
−2.8 |
−3.1 |
−8.7 |
492–1185 |
17.8 |
9
|
FAU |
−4.2 |
−4.2 |
−4.2 |
−12.6 |
25–573 |
13.5 |
10
|
MAPO-17 |
−9.16 |
−9.16 |
+4.66 |
−4.6 |
323–773 |
15.9 |
This work |
AlPO4-17 |
−15.3 |
−15.3 |
−4.52 |
−35.1 |
18–300 |
15.8 |
11
|
CHA |
−8.24 |
−8.24 |
−13.3 |
−28.5 |
293–873 |
15.4 |
13
|
CIT-5 |
+8.57 |
+28.2 |
+8.41 |
+14.9 |
373–973 |
18.3 |
This work |
AlPO4-31 |
+9.72 |
+9.72 |
+17.3 |
+32.8 |
323–823 |
19.2 |
This work |
It is interesting to compare our results on MAPO-17 with those for
AlPO4-17 (both adopting the ERI structure type) obtained
by Attfield and Sleight.11 Tschaufeser and
Parker7 originally predicted that the NTE in
AlPO4-17 itself should be stronger at higher temperatures,
at least up to their limit of 500 K, with values ranging from about α(average) ≈ −12 × 10−6
°C−1 at 50 K to −30 × 10−6
°C−1 at 500 K. Attfield and Sleight reported a nearly constant α(average)
(= 1/3αv) of −11.7 × 10−6
°C−1
over the range 18–300 K, although αc
does appear to become less negative at the higher limit. Our own data on MAPO-17 (Table 2) over the temperature range 323–773 K
are in marked contrast to either of these reports, with approximately linear
behaviour of both axes, and an α(average) of only −1.5 × 10−6
°C−1; the most significant difference is that the c-axis
actually expands throughout this temperature range. This difference,
at least in part, must be ascribed to the presence of 10% divalent
Mg2+ substituting for Al3+ throughout the
framework in MAPO-17. After calcination, the charge deficiency that results
is largely made up of charge-balancing protons on bridging oxygens (so-called ‘bridging
hydroxyls’). Clearly, subtle differences in framework composition
can have a considerable influence on expansivity behaviour, and this must
be considered in future work.
Thermal expansion in CIT-5
As we have shown above, siliceous CIT-5 represents a rare example of
a microporous solid which actually expands on heating, having a particularly
large expansivity along the one-dimensional channel axis, b.
Our restrained refinements from powder neutron diffraction data produce well-behaved
structural parameters and, we believe, permit us to identify some of the key
parameters underlying the structural origin of this perhaps anomalous behaviour.
The calcined form of CIT-5 was first reported to adopt the centrosymmetric
space group Imma,21 however, a subsequent
refinement from high-resolution synchrotron X-ray powder diffraction
data16 suggested lowering of symmetry to the
non-centrosymmetric Im2a; loss of the mirror plane perpendicular
to the short axis allows elimination of some unreasonable Si–O–Si
bond angles. We have chosen to adopt this lower symmetry space group in our
own refinements. A typical Rietveld plot is given in Fig. 3.
Final refined atomic parameters at the lowest and highest temperatures studied
are given in Tables 3 and 4.
The structure of CIT-5 comprises 14-membered ring channels running
along the short axis [010]. Labelled plots of the framework along [010]
and [001] are given in Fig. 4
and 5, respectively. Treatment of the SiO4 tetrahedra
as semi-rigid, by the application of restraints, allows flexibility in
the Si–O–Si bond angles, and it is these parameters which may
be anticipated to show the most variation versus temperature. They
are plotted in Fig. 6. It can be clearly
seen that the angles Si2–O1–Si4 and Si2–O10–Si4 vary
the most. Fig. 4 shows that these angles
are situated along the b-axis, and are largely responsible for
driving its expansivity. Also significant is the behaviour of the Si3–O2–Si5,
Si1–O3–Si1, Si1–O7–Si1 and Si3–O9–Si5
angles (Fig. 6). At first
glance, these angles appear to have little correlation with the b-axis.
However, the angle between each of the Si–Si vectors and the b-axis
has been calculated, and the results are given in Table 5.
From this, it is clear that the b-axis expansion is driven by
the behaviour of the angles Si2–O1–Si4, Si3–O2–Si5,
Si1–O3–Si1, Si1–O7–Si1, Si3–O9–Si5 and
Si2–O10–Si4, whereas the angles Si4–O5–Si5, Si2–O5–Si3,
Si1–O6–Si5 and Si3–O8–Si3 lie almost perpendicular
to the b-axis, in the ac plane. The a and c-axis
expansion is driven largely by the Si1–O6–Si5 angle.
![Portion of the CIT-5
structure viewed along [010].](/image/article/2001/JM/b002950p/b002950p-f4.gif) |
| Fig. 4
Portion of the CIT-5
structure viewed along [010].
| |
![14-Membered ring
in CIT-5 viewed along [001].](/image/article/2001/JM/b002950p/b002950p-f5.gif) |
| Fig. 5
14-Membered ring
in CIT-5 viewed along [001].
| |
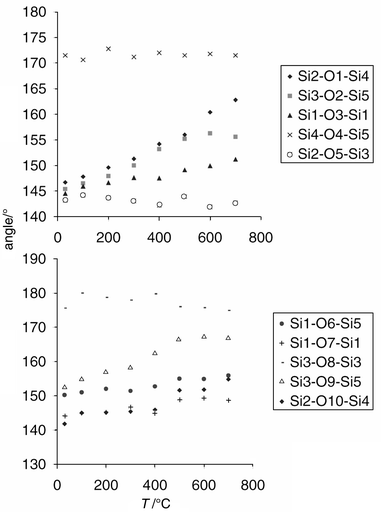 |
| Fig. 6
Thermal behaviour of
the bridging Si–O–Si bond angles in CIT-5.
| |
Table 3
Final refined atomic parameters for CIT-5
at 30
°C; space group Im2a, a = 13.6824(2), b = 5.0214(1), c = 25.5223(4) Å, V = 1753.50(4) Å3, χ2 = 2.71, Rwp = 0.051, Rp = 0.11, RF2 = 0.055
|
x
|
y
|
z
|
U
iso × 100/Å2 |
Multiplicity |
Si1 |
0.6378(3) |
0.506(1) |
0.7162(2) |
−0.09(7) |
8c |
Si2 |
0.25 |
0.019(1) |
0.5240(2) |
−0.09(7) |
4b |
Si3 |
0.4588(3) |
−0.0959(9) |
0.5580(1) |
−0.09(7) |
8c |
Si4 |
0.25 |
0.522(1) |
0.4564(3) |
−0.09(7) |
4b |
Si5 |
0.5537(3) |
0.4093(9) |
0.6050(2) |
−0.09(7) |
8c |
O1 |
0.25 |
0.3207(9) |
0.5037(2) |
0.07(5) |
4b |
O2 |
0.4747(3) |
−0.3859(9) |
0.5820(2) |
0.07(5) |
8c |
O3 |
0.75 |
0.4754(9) |
0.6981(2) |
0.07(5) |
4b |
O4 |
0.6563(2) |
0.4703(8) |
0.5786(1) |
0.07(5) |
8c |
O5 |
0.3441(2) |
−0.0349(9) |
0.5600(2) |
0.07(5) |
8c |
O6 |
0.5690(3) |
0.4451(9) |
0.6666(2) |
0.07(5) |
8c |
O7 |
0.6154(3) |
0.7987(9) |
0.7375(2) |
0.07(5) |
8c |
O8 |
0.5 |
−0.0839(9) |
0.5 |
0.07(5) |
4a |
O9 |
0.5172(3) |
0.1125(8) |
0.5933(2) |
0.07(5) |
8c |
O10 |
0.25 |
0.3254(9) |
0.0259(3) |
0.07(5) |
4b |
Table 4
Final refined atomic parameters for CIT-5
at 700
°C; space group Im2a, a = 13.7481(3), b = 5.1068(1), c = 25.6350(7) Å, V = 1799.81(7) Å3, χ2 = 4.18, Rwp = 0.056, Rp = 0.059
|
x
|
y
|
z
|
U
iso × 100/Å2 |
Multiplicity |
Si1 |
0.6390(4) |
0.4683(9) |
0.7177(3) |
3.7(2) |
8c |
Si2 |
0.25 |
−0.0076(9) |
0.5253(3) |
3.7(2) |
4b |
Si3 |
0.4599(5) |
−0.0568(9) |
0.5574(2) |
3.7(2) |
8c |
Si4 |
0.25 |
0.4953(9) |
0.4563(4) |
3.7(2) |
4b |
Si5 |
0.5515(5) |
0.4494(9) |
0.6061(3) |
3.7(2) |
8c |
O1 |
0.25 |
0.2713(9) |
0.4982(4) |
3.1(1) |
4b |
O2 |
0.4889(7) |
−0.3055(9) |
0.5910(4) |
3.1(1) |
8c |
O3 |
0.75 |
0.4668(9) |
0.7023(3) |
3.1(1) |
4b |
O4 |
0.6544(4) |
0.4847(9) |
0.5783(2) |
3.1(1) |
8c |
O5 |
0.3436(4) |
−0.0422(9) |
0.5601(2) |
3.1(1) |
8c |
O6 |
0.5742(4) |
0.4366(9) |
0.6664(2) |
3.1(1) |
8c |
O7 |
0.6103(4) |
0.7358(9) |
0.7449(4) |
3.1(1) |
8c |
O8 |
0.5 |
−0.0705(9) |
0.5 |
3.1(1) |
4a |
O9 |
0.4974(7) |
0.1948(9) |
0.5875(4) |
3.1(1) |
8c |
O10 |
0.25 |
0.2821(9) |
0.0204(4) |
3.1(1) |
4b |
Table 5
Location, thermal behaviour and directional
nature of the Si–O–Si bond angles in CIT-5
Angle |
Description of location |
Tilt from [010] of Si–Si vector |
Change in Si–O–Si vs.T |
Si2–O1–Si4 |
b-axis |
34.8° |
+16.1° |
Si3–O2–Si5 |
ring |
35.2° |
+10.2° |
Si1–O3–Si1 |
bridging |
34.6° |
+6.7° |
Si4–O4–Si5 |
bridging |
78.8° |
0° |
Si2–O5–Si3 |
ring |
78.8° |
−0.5° |
Si1–O6–Si5 |
ring |
80.1° |
+5.7° |
Si1–O7–Si1 |
ring |
34.6° |
+4.6° |
Si3–O8–Si3 |
bridging |
88.7° |
−0.7° |
Si3–O9–Si5 |
ring |
35.2° |
+14.2° |
Si2–O10–Si4 |
b-axis |
35.8° |
+13° |
Conclusions
The growing list of thermal expansivity data available on microporous solids
clearly suggests that negative thermal expansivity is the norm rather than
the exception. Of the 17 materials so far characterised, 15 show a volume
contraction on heating, of which 13 show NTE along all three crystallographic
axes. Only two materials, CIT-5 and AlPO4-31, show an overall
volume expansion. Based on gross structural features, it appears that positive
thermal expansivity is favoured by a high framework density (over 18 T
atoms per 1000 Å3) and a one-dimensional
channel system. However, this empirical rule remains to be borne out by further
studies of axial expansivities for a wider variety of framework types and
compositions, including comparisons of as-made and calcined materials,
and also by more detailed structural studies by single crystal diffraction
methods and further computational studies. The complexity of the problem is
illustrated by the observation of a positive expansivity along the channel
axis in the one-dimensional 12MR system ITQ-4, but a corresponding
negative expansivity in the one-dimensional 10MR system ITQ-9 (Table 2), both structures having similar
framework density. The mechanism of NTE in specific microporous materials,
therefore, remains unclear, although recent computational developments have
suggested that certain cases can be modelled with reasonable reliability.
The RUM models which have been successfully applied to β-quartz,23 for example, have recently been extended to microporous
polymorphs.24 Gale has also recently developed
analytical free energy minimisation methods25
which he used to predict NTE in MCM-22 (MWW structure, as for ITQ-1)
and SSZ-42 (IFR structure, as for ITQ-4). Further developments
in these methods, alongside experimental data, will be essential for the full
understanding of this unusual behaviour.
In the case of CIT-5, where positive expansivity is observed along
all three crystallographic axes, the particularly large expansivity along
the 14MR channel axis (αb = 28.2 × 10−6
°C−1) is shown to be driven by dramatic changes in the interpolyhedral Si–O–Si
angles. This effect obviously cannot, and need not, be explained merely by
a dynamic rocking of tetrahedra, or a transverse vibrational mode. However,
with the limited structural data available so far (specifically the powder
X-ray study of AlPO4-1711
and the powder neutron study of chabazite13)
we still cannot say whether the mechanism driving NTE in these materials has
a static or dynamic origin. To discriminate between these options, higher
quality, single crystal diffraction data are required. Such studies are currently
ongoing.
Acknowledgements
We would like to that the EPSRC for provision of a Project
Studentship (D. A. W.). Samples of the siliceous zeolites were prepared
by L. A. Villaescusa and M.-J. Díaz Cabañas, in the laboratory
of Dr M. A. Camblor (Valencia). We are grateful to Drs R. I. Smith
and D. Engberg for help with collection of neutron diffraction data at ISIS.
References
- J. S. O. Evans, J. Chem. Soc., Dalton Trans., 1999, 3317 RSC.
- J. S. O. Evans, T. A. Mary, T. Vogt, M. A. Subramanian and A. W. Sleight, Chem. Mater., 1996, 8, 2809 CrossRef CAS.
- J. S. O. Evans, W. I. F. David and A. W. Sleight, Acta Crystallogr.,
Sect. B, 1999, 55, 333 CrossRef.
- V. Korthuis, N. Khosrovani, A. W. Sleight, N. Roberts, R. Dupree and W. W. Warren, Chem. Mater., 1995, 7, 412 CrossRef CAS.
- D. A. Woodcock, P. Lightfoot and C. Ritter, Chem.
Commun., 1998, 107 RSC.
- D. A. Woodcock and P. Lightfoot, J. Mater. Chem., 1999, 9, 2907 RSC.
- P. Tschaufeser and S. C. Parker, J. Phys. Chem., 1995, 99, 10609 CrossRef CAS.
- J.
W. Couves, R. H. Jones, S. C. Parker, P. Tschaufeser and C. R. A. Catlow, J.
Phys.: Condens. Matter, 1993, 5, L329 Search PubMed.
- S. H. Park, R.
W. G. Kuntsleve, H. Graetsch and H. Gies, Stud. Surf. Science Catal., 1997, 105, 1989 Search PubMed.
- M. P. Attfield and A. W. Sleight, Chem.
Commun., 1998, 601 RSC.
- M. P. Attfield and A. W. Sleight, Chem. Mater., 1998, 10, 2013 CrossRef CAS.
- D. A. Woodcock, P. Lightfoot, P. A. Wright, L.
A. Villaescua, M.-J. Díaz Cabañas and M.
A. Camblor, J. Mater. Chem., 1999, 9, 349 RSC.
- D. A. Woodcock, P. Lightfoot, L. A. Villaescusa, M.-J. Díaz Cabañas, M. A. Camblor and D. Engberg, Chem.
Mater., 1999, 11, 2508 CrossRef CAS.
- L. A. Villaescusa, P.
A. Barrett and M. A. Camblor, Angew. Chem., Int. Ed.., 1999, 38, 1997 CrossRef CAS.
- L.
A. Villaescusa, P. A. Barrett and M. A. Camblor, Chem. Commun., 1998, 2329 RSC.
- P. A. Barrett, M.-J. Díaz Cabañas, M.
A. Camblor and R. H. Jones, J. Chem. Soc., Faraday Trans., 1998, 94, 2475 RSC.
- H.
L. Zubowa, M. Richter, U. Roost, B. Parlitz and R. Fricke, Catal. Lett., 1993, 19, 67 Search PubMed.
- G. W. Noble, P. A. Wright, P. Lightfoot, R. E. Morris, K.
J. Hudson, Å. Kvick and H. Graafsma, Angew. Chem., Int. Ed. Engl., 1997, 36, 81 CrossRef CAS.
-
A. C. Larson
and R. B. Von Dreele,
Los Alamos National Laboratory Report No. LA-UR-86-748, 1987.
- J. M. Bennett and R. M. Kirchner, Zeolites, 1992, 12, 338 CrossRef CAS.
- D. Martin and D. Engberg, Physica
B, 1999, 134, 268.
- P. Wagner, M. Yoshikawa, M. Lovallo, K. Tsuji, M. Tsapatsis and M.
E. Davis, Chem. Commun., 1997, 2179 RSC.
- M. B. Smirnov, Phys. Rev. B, 1999, 59, 4036 CrossRef CAS.
- K. D. Hammonds, V. Heine and M. T. Dove, J. Phys. Chem. B, 1998, 102, 1759 CrossRef CAS.
- J. D. Gale, J. Phys. Chem. B, 1998, 102, 5423 CrossRef CAS.
- International Zeolite Association structure code, see: http://www.kristall.ethz.ch/IZA-SC/.
Footnote |
† Basis
of a presentation given at Materials Discussion No. 3, 26–29
September, 2000, University of Cambridge, UK. |
|
This journal is © The Royal Society of Chemistry 2001 |