Reactivity of multi-site bound allenylidene towards alkynes and silica gel
†
Received
(in Cambridge, UK)
18th August 2000
, Accepted 19th October 2000
First published on 13th December 2000
Introduction
The recent interest in allenylidene complexes stems partly from the proposed involvement of these ligands in carbon chain growth processes at a metal surface.1 Cluster complexes containing allenylidene ligands bound to more than two metal atoms offer the potential to model the surface-mediated reactivity of such organic fragments. However, examples of multi-site bound allenylidene complexes in the literature are rare
1–3 and accounts of their carbon–carbon bond forming reactions limited to the work of Bruce and co-workers on phenyl migration in the dppm-substituted complex [Ru3(CO)7(μ-CO)(μ-dppm)(μ3-CCCPh2)]
4 and the reactivity of [Ru3(CO)9(μ-OH)(μ-H)(μ3-CCCPh2)] 1 towards terminal alkynes HC
CR (R = Ph or SiMe3).1 Interestingly, reaction of 1 with HC
CSiMe3 was found to proceed via carbon–carbon bond formation between the alkyne and the α-carbon of the allenylidene ligand and hydride migration to afford the μ-hydroxide species [Ru3(CO)9(μ-OH){μ3-CH(SiMe3)C(H)CC
CPh2}] 2 (see Scheme 1). Complex 2 may add a second molecule of alkyne, resulting in loss of water from the cluster and formation of [Ru3(CO)8{μ3-C(SiMe3)C(H)C(SiMe3)C(H)CC
CPh2}] 3a. Employing phenylacetylene in the reaction does not allow isolation of an intermediate species, instead the bis-alkyne derivative 3b is formed directly along with an isomer derived from tail to tail linkage of the incoming alkynes. It should be noted that each of the two steps in the above reaction involves either the μ-H or the μ-OH of the allenylidene cluster 1 in some way. The high yield synthesis of the μ3-allenylidene complex [Ru3(CO)9(μ-CO)(μ3-CCCPh2)] 4a, described previously,3 presents an ideal opportunity to explore the reactivity of this ligand when co-ordinated to an unsubstituted ruthenium carbonyl centre and to make a comparison with the work of Bruce et al., described above. We report here on the reactivity of 4a towards the internal alkynes but-2-yne and diphenylacetylene, which leads to the formation of metallacyclic complexes containing C5 ligands bridging three ruthenium atoms in an unprecedented manner. In addition, the reaction with diphenylacetylene affords small amounts of a novel ‘flat-butterfly’ tetraruthenium complex containing a C5 chain. We also include an account of the ‘surface-mediated’ transformations of the complexes [Ru3(CO)9(μ-CO){μ3-CCC(Ph)R}] (R = Ph 4a or R = Me 4b) that occur on silica gel and vary according to the nature of the R substituent.
 |
| Scheme 1 | |
Results and discussion
Heating complex 4a at 40 °C in the presence of an excess of alkyne afforded [Ru3(CO)9{μ3-C(Me)C(Me)C2CPh2}] 5a (30%), in the case of but-2-yne, and [Ru3(CO)9{μ3-C(Ph)C(Ph)C2CPh2}] 5b (20%) and [Ru4(CO)12{μ4-C(Ph)C(Ph)CCCPh2}] 6 (5%) in the case of diphenylacetylene (see Scheme 2). The molecular structures of the new complexes were all determined by X-ray diffraction studies and are discussed later. The spectroscopic data are consistent with the solid state structures. Thus, the IR spectra of complexes 5 show the presence of eight terminal carbonyl bands and a stretch at about 1950 cm−1 indicative of an asymmetrically bridging carbonyl unit.5 The 1H NMR spectra each show the presence of phenyl protons in the region δ 7.76–6.74 and a doublet of doublets (δ 6.06 5a; 6.18 5b) for the proton attached to the metal-bound ortho-carbon of the phenyl group. This slight up-field shift from the normal aromatic phenyl region compares well with those seen for the corresponding protons of di-6 and tri-ruthenium
7 complexes containing similar metal–phenyl π interactions. The IR spectrum of complex 6 also indicates the presence of a semi-bridging carbonyl ligand (1916 cm−1), as well as containing bands for terminal carbonyls, whilst the 1H NMR spectrum showed only a multiplet due to phenyl protons centered at δ 7.45. Owing to the low yield of 6 no satisfactory 13C NMR data could be obtained but the FAB mass spectrum and elemental analysis were consistent with the solid state structure.
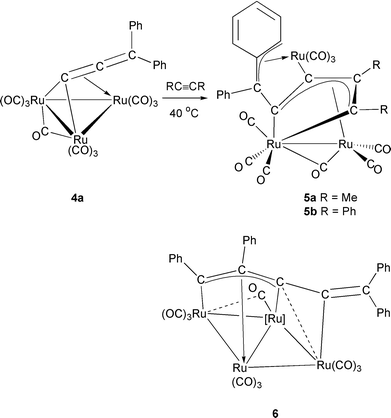 |
| Scheme 2 | |
The mechanism by which complexes 5 are formed is unclear but formally involves the following processes: loss of one molecule of CO; cleavage of two Ru–Ru bonds; insertion of an alkyne unit into one of the metal–allenylidene σ bonds of 4a; co-ordination of Cγ and a phenyl group of the original allenylidene ligand to a ruthenium atom in η3 fashion. Di- and tri-nuclear complexes containing metallacyclopentadienyl ligands are common products from reaction of metal carbonyl complexes of the iron triad with alkynes
8 and this ligand type has also been observed in complexes containing transition metals other than those of Group 8.9 However, the complexes 5 show some noteworthy features. First, they represent extremely rare examples of metallacyclopentadienyl ligands in which one of the ring carbons has been metallated. Secondly, we could find only three examples in the literature of metallacyclopentadienyl ligands containing ligating substituents at the 1 or 2 positions, the complexes [Ru3Pt(CO)7(dppe){μ4-C(But)C(H)C(CHCBut)C(CBut)}{μ-C(H)C(H)(But)}],10 [Ru3(CO)8{RCC(Ph)C(Ph)CR}] (R = C(H)C(H)Ph)
11 and [Os3(CO)9{C(SiMe3)C(Me)C(H)CR}] (R = aryl or ferrocenyl).12 The first two examples each contain an exocyclic vinyl group co-ordinated to a metal atom. The last example represents a series of complexes containing ortho-metallated ferrocenyl or aryl substituents on one of the C1 carbons. Thirdly, this is the first time such a metallacyclopentadienyl unit has been obtained directly from allenylidene. Interestingly, the complex [Fe2(CO)6{μ-Ph(H)CCCHC(OMe)O}], containing a penta-atomic metallacycle, was reported in 1997 from reaction of the allenylidene species [Fe3(CO)9(μ-CO){μ3-CCC(Ph)H}] with methanol in the presence of silica gel.13 Further, closo-pentagonal bipyramidal clusters [Fe3(CO)6(μ-CO)2(RC2R1)2] and ‘ferrole’ complexes [Fe2(CO)6(RC2R1)2] are common by-products in the thermolysis of propargyl alcohols and [Fe3(CO)12], leading to formation of allenylidene complexes [Fe3(CO)9(μ-CO){μ3-CCC(R)R1}].8,13–15 It was assumed that these metallacyclopentadienyl by-products are the result of alkynol dimerisation and cluster fragmentation. However, in the light of the above results it is tempting to suggest that at least some of these products are a direct result of the desired allenylidene complex reacting with the excess of alkynol under the reaction conditions.
It was mentioned in the introduction that one of the reasons for the interest in allenylidene ligands is their proposed involvement in carbon chain-growth processes. Thus, it is noteworthy that metallacyclopentadienyl ligands have been found as intermediates in a range of such processes, including Ziegler–Natta catalysis,16 olefin polymerisation,17 alkyne oligomerisation,18 hydro-oligomerisation of alkynes,19 alkyne cycloaddition
20 and the synthesis of tropones.21
Of the products obtained from our allenylidene–alkyne linking reactions, only the tetraruthenium complex 6 bears any resemblance to those products obtained by Bruce and co-workers in that it contains a C5 chain similar to that seen in 2 (formed from alkyne linkage with the α-carbon of the allenylidene ligand). However, the formation of the chain in complex 2 involved hydrogen migration from the cluster to one of the alkyne carbons, a process which may not occur for the unsubstituted cluster 4a. Instead, formation of 6 has involved cluster expansion so that the pentacarbon backbone of the ligand now bridges four ruthenium atoms in a hitherto unseen manner. Formally, complex 6 may be derived from 5bvia addition of one unit of Ru(CO)3 and so we decided to investigate whether 6 was a simple decomposition product of 5b. However, thermolysis of 5b under a range of reaction conditions led to isolation of starting material or decomposition products other than 6 which were not identified.
Molecular structures of complexes 5
The molecular structures of complexes 5a and 5b are shown in Figs. 1 and 2 respectively. Selected bond lengths and angles for 5a are given in Table 1 whereas those of 5b are available as supplementary data (ESI
†). The two sets of structural parameters were extremely similar and all of the bond lengths and angles used in the discussion below are those of 5a, unless otherwise stated.
Table 1 Selected bond lengths [Å] and angles [°] for complex 5a
Ru(1)–C(15) |
2.095(3) |
Ru(3)–C(18) |
2.390(3) |
Ru(1)–C(11) |
2.113(3) |
C(6)–O(6) |
1.147(4) |
Ru(1)–Ru(2) |
2.7140(6) |
C(10)–C(11) |
1.509(4) |
Ru(1) ⋯ C(6) |
2.813(4) |
C(11)–C(12) |
1.414(5) |
Ru(2)–C(6) |
1.904(4) |
C(12)–C(14) |
1.430(4) |
Ru(2)–C(15) |
2.233(3) |
C(12)–C(13) |
1.522(4) |
Ru(2)–C(11) |
2.273(3) |
C(14)–C(15) |
1.415(4) |
Ru(2)–C(14) |
2.292(3) |
C(15)–C(16) |
1.526(4) |
Ru(2)–C(12) |
2.303(3) |
C(16)–C(17) |
1.445(4) |
Ru(3)–C(14) |
2.095(3) |
C(16)–C(23) |
1.515(4) |
Ru(3)–C(16) |
2.260(3) |
C(17)–C(18) |
1.439(4) |
Ru(3)–C(17) |
2.341(3) |
|
|
|
|
|
|
C(15)–Ru(1)–C(11) |
76.43(13) |
C(10)–C(11)–Ru(2) |
128.2(2) |
C(15)–Ru(1)–Ru(2) |
53.48(9) |
Ru(1)–C(11)–Ru(2) |
76.37(10) |
C(11)–Ru(1)–Ru(2) |
54.48(9) |
C(11)–C(12)–C(14) |
113.8(3) |
Ru(2)–Ru(1)–C(6) |
40.24(8) |
C(11)–C(12)–C(13) |
125.1(3) |
C(15)–Ru(2)–C(11) |
70.55(11) |
C(14)–C(12)–C(13) |
121.1(3) |
C(15)–Ru(2)–C(14) |
36.42(11) |
C(11)–C(12)–Ru(2) |
70.87(19) |
C(11)–Ru(2)–C(14) |
62.90(12) |
C(14)–C(12)–Ru(2) |
71.48(18) |
C(15)–Ru(2)–C(12) |
63.94(11) |
C(13)–C(12)–Ru(2) |
128.0(2) |
C(11)–Ru(2)–C(12) |
35.99(12) |
C(15)–C(14)–C(12) |
115.2(3) |
C(14)–Ru(2)–C(12) |
36.27(11) |
C(15)–C(14)–Ru(3) |
100.6(2) |
C(6)–Ru(2)–Ru(1) |
72.67(11) |
C(12)–C(14)–Ru(3) |
143.5(2) |
C(15)–Ru(2)–Ru(1) |
48.92(8) |
C(15)–C(14)–Ru(2) |
69.52(18) |
C(11)–Ru(2)–Ru(1) |
49.15(8) |
C(12)–C(14)–Ru(2) |
72.25(19) |
C(14)–Ru(2)–Ru(1) |
73.30(8) |
Ru(3)–C(14)–Ru(2) |
130.43(15) |
C(12)–Ru(2)–Ru(1) |
73.75(8) |
C(14)–C(15)–C(16) |
104.5(3) |
C(14)–Ru(3)–C(16) |
64.45(12) |
C(14)–C(15)–Ru(1) |
116.6(2) |
O(6)–C(6)–Ru(2) |
170.3(3) |
C(16)–C(15)–Ru(1) |
135.6(2) |
O(6)–C(6)–Ru(1) |
122.6(3) |
C(14)–C(15)–Ru(2) |
74.06(19) |
Ru(2)–C(6)–Ru(1) |
67.09(11) |
C(16)–C(15)–Ru(2) |
132.2(2) |
C(12)–C(11)–C(10) |
118.1(3) |
Ru(1)–C(15)–Ru(2) |
77.60(11) |
C(12)–C(11)–Ru(1) |
117.0(2) |
C(17)–C(16)–C(15) |
114.9(3) |
C(10)–C(11)–Ru(1) |
124.3(3) |
C(15)–C(16)–Ru(3) |
90.44(19) |
C(12)–C(11)–Ru(2) |
73.14(19) |
|
|
 |
| Fig. 1 Molecular geometry of complex 5a; all hydrogen atoms have been omitted for clarity (as in all the present structures).
| |
 |
| Fig. 2 Molecular geometry of complex 5b.
| |
The complexes 5 each contain three ruthenium atoms of which only Ru(1) and Ru(2) are within bonding distance [Ru(1)–Ru(2) 2.7140(6) Å], with Ru(3) having non-bonding distances of 4.761 and 3.984 Å to Ru(1) and Ru(2), respectively. Rutheniums (1) and (3) each are bonded to three terminal carbonyl ligands and Ru(2) to two. In addition, the Ru(1)–Ru(2) bond is asymmetrically bridged by a carbonyl unit [Ru(1)–C(6) 2.813(4), Ru(2)–C(6) 1.904(4) Å; Ru(2)–C(6)–O(6) 170.3°], with the six terminal carbonyls around the metal–metal bond held in a non-sawhorse arrangement. The asymmetric nature of the bridging carbonyl unit is more pronounced in complex 5b, having an Ru(2)–C(6)–O(6) angle of 161.5° and Ru(1)–C(6) and Ru(2)–C(6) bond lengths of 2.603(4) and 1.921(5) Å respectively. The C5 fragments in each complex are clearly formed through linkage of C(12) of an alkyne molecule (but-2-yne 5a; diphenylacetylene 5b) with the α-carbon [C(14)] of the allenylidene unit in 4a, and bridge all three metal atoms. Thus, carbons C(11)–C(15) form a metallacyclic unit through two σ-type bonds to Ru(1) [Ru(1)–C(11) 2.113(3) and Ru(1)–C(15) 2.095(3) Å], all four carbons of which interact with Ru(2) in an η4 manner [with all Ru–C bonds in the range 2.203(3)–2.333(3) Å]. The C(11)–C(12), C(12)–C(14) and C(14)–C(15) bond lengths of 1.414(5), 1.430(4) and 1.415(4) Å respectively suggest some delocalisation of the π-electron density over these three bonds. The γ-carbon [C(16)] of the original allenylidene unit, and the ortho [C(17)] and meta [C(18)] carbons of one of its phenyl substituents, form an allylic type fragment [C(16)–C(17) 1.445(4), C(17)–C(18) 1.439(4) Å] which interacts in an η3 fashion with Ru(3) [Ru(3)–C(16) 2.260(3), Ru(3)–C(17) 2.314(3) and Ru(3)–C(18) 2.390(3) Å]. Related interactions have previously been observed in some dimetallic aryl-substituted μ-carbene complexes.5,22 The involvement of the phenyl ring in bonding to Ru(3) results in some delocalisation of the π-electron density in the remaining intra-ring C–C bonds such that two [C(19)–C(20) 1.354(5), C(21)–C(22) 1.351(5) Å] are shorter than the other three [all greater than 1.415(5) Å]. A strong σ bond from C(14) to Ru(3) [2.095(3) Å] completes the bonding. The above, along with the spectroscopic data, suggests complexes 5 are best represented as shown in Scheme 2, i.e. as μ3-butadienyl species with a diphenylcarbene substituent which binds in η3-allyl fashion to one of the ruthenium atoms. Considering 5a and 5b as simple ‘ruthenole’-type complexes in which the metallacyclopentadienyl ring has been metallated allows us to assign them as having nido-pentagonal bipyramidal geometries.
Molecular structure of complex 6
The structure of complex 6 is shown in Fig. 3 and selected bond lengths and angles in Table 2. The tetraruthenium complex has 62 valence electrons and adopts the planar rhomboid (‘flat butterfly’) arrangement observed previously for such cluster compounds as [Ru4(CO)8(μ-PPh2)2(C4But2)].23 Thus, the fold angle between the wings is 4.1° and the four rutheniums form a plane (mean deviation 0.045 Å). Some distortion of the metal framework is suggested by the long peripheral Ru(3)–Ru(4) bond distance of 3.0306(11) Å. The longest of the remaining peripheral Ru–Ru bonds, the Ru(1)–Ru(2) vertex, is asymmetrically bridged by the C(4)O(4) carbonyl unit. The main point of interest in complex 6 stems from the presence of an unusual bridging moiety that binds to the metal centre in a manner not previously reported. Thus, carbons C(13)–(17) form the C5 backbone of a μ4-organic unit derived from carbon–carbon bond formation between C(14) of a molecule of diphenylacetylene and the α-carbon [C(15)] of the allenylidene unit in complex 4a. The ligand formally donates a total of six electrons to the metal centre via three σ bonds [Ru(1)–C(13) 2.122(3), Ru(2)–C(15) 2.091(3) and Ru(4)–C(16) 2.113(3) Å] and four π-interactions, of which the Ru(3)–C(15) and Ru(3)–C(14) distances [2.414(3) and 2.324(3) Å, respectively] are significantly longer than those of Ru(3)–C(13) and Ru(3)–C(14) [2.323(3) and 2.324(3) Å], possibly reflecting the multi co-ordinated nature of C(15). The bonds between C(13)–C(16) span the narrow range 1.416(4)–1.443(4) Å suggesting delocalisation of the electron density across this four-carbon chain, whereas the C(16)–C(17) distance of 1.341(4) Å is characteristic of an unco-ordinated double bond.
Table 2 Selected bond lengths [Å] and angles [°] for complex 6
Ru(1)–C(13) |
2.122(3) |
Ru(3)–C(15) |
2.414(3) |
Ru(1) ⋯ C(4) |
2.585(3) |
Ru(3)–Ru(4) |
3.0306(11) |
Ru(1)–Ru(3) |
2.7883(9) |
Ru(4)–C(16) |
2.113(3) |
Ru(1)–Ru(2) |
2.8178(10) |
Ru(4)–C(15) |
2.516(3) |
Ru(2)–C(4) |
1.899(4) |
C(4)–O(4) |
1.150(4) |
Ru(2)–C(15) |
2.091(3) |
C(13)–C(14) |
1.416(4) |
Ru(2)–Ru(4) |
2.7733(9) |
C(14)–C(15) |
1.443(4) |
Ru(2)–Ru(3) |
2.7970(8) |
C(15)–C(16) |
1.435(4) |
Ru(3)–C(13) |
2.323(3) |
C(16)–C(17) |
1.341(4) |
Ru(3)–C(14) |
2.324(3) |
|
|
|
|
|
|
C(13)–Ru(1)–Ru(3) |
54.43(8) |
C(16)–Ru(4)–Ru(2) |
72.92(9) |
C(13)–Ru(1)–Ru(2) |
84.50(8) |
C(15)–Ru(4)–Ru(2) |
46.25(7) |
C(4)–Ru(1)–Ru(2) |
40.87(7) |
C(16)–Ru(4)–Ru(3) |
81.55(9) |
Ru(3)–Ru(1)–Ru(2) |
59.86(2) |
C(15)–Ru(4)–Ru(3) |
50.57(7) |
C(15)–Ru(2)–Ru(4) |
60.39(9) |
Ru(2)–Ru(4)–Ru(3) |
57.42(2) |
C(15)–Ru(2)–Ru(3) |
57.03(8) |
O(4)–C(4)–Ru(2) |
160.2(3) |
Ru(4)–Ru(2)–Ru(3) |
65.92(3) |
O(4)–C(4)–Ru(1) |
123.4(3) |
C(4)–Ru(2)–Ru(1) |
62.96(10) |
Ru(2)–C(4)–Ru(1) |
76.17(11) |
C(15)–Ru(2)–Ru(1) |
86.14(9) |
C(14)–C(13)–Ru(1) |
125.2(2) |
Ru(4)–Ru(2)–Ru(1) |
125.33(2) |
C(14)–C(13)–Ru(3) |
72.30(17) |
Ru(3)–Ru(2)–Ru(1) |
59.55(2) |
Ru(1)–C(13)–Ru(3) |
77.57(10) |
C(13)–Ru(3)–C(14) |
35.48(10) |
C(13)–C(14)–C(15) |
119.9(3) |
C(13)–Ru(3)–C(15) |
62.96(10) |
C(13)–C(14)–Ru(3) |
72.21(17) |
C(14)–Ru(3)–C(15) |
35.41(10) |
C(15)–C(14)–Ru(3) |
75.72(17) |
C(13)–Ru(3)–Ru(1) |
48.00(8) |
C(16)–C(15)–C(14) |
123.2(3) |
C(14)–Ru(3)–Ru(1) |
75.69(8) |
C(16)–C(15)–Ru(2) |
112.4(2) |
C(15)–Ru(3)–Ru(1) |
81.09(7) |
C(14)–C(15)–Ru(2) |
124.1(2) |
C(13)–Ru(3)–Ru(2) |
81.50(7) |
C(16)–C(15)–Ru(3) |
124.1(2) |
C(14)–Ru(3)–Ru(2) |
74.91(7) |
C(14)–C(15)–Ru(3) |
68.87(16) |
C(15)–Ru(3)–Ru(2) |
46.59(7) |
Ru(2)–C(15)–Ru(3) |
76.38(10) |
Ru(1)–Ru(3)–Ru(2) |
60.60(2) |
C(16)–C(15)–Ru(4) |
57.06(17) |
C(13)–Ru(3)–Ru(4) |
116.57(7) |
C(14)–C(15)–Ru(4) |
133.14(19) |
C(14)–Ru(3)–Ru(4) |
85.10(8) |
Ru(2)–C(15)–Ru(4) |
73.37(9) |
C(15)–Ru(3)–Ru(4) |
53.61(8) |
Ru(3)–C(15)–Ru(4) |
75.82(9) |
Ru(1)–Ru(3)–Ru(4) |
117.14(2) |
C(17)–C(16)–C(15) |
133.5(3) |
Ru(2)–Ru(3)–Ru(4) |
56.663(19) |
C(17)–C(16)–Ru(4) |
137.5(2) |
C(16)–Ru(4)–C(15) |
34.74(10) |
C(15)–C(16)–Ru(4) |
88.2(2) |
 |
| Fig. 3 Molecular geometry of complex 6.
| |
Transformations of [Ru3(CO)9(μ-CO){μ3-CCC(Ph)R}] (R = Ph 4a or Me 4b) on silica gel
There are several reports on the effect of supports, such as silica gel and alumina, in promoting hydration and dehydration reactions.7,24 We have previously shown that the splitting and addition of water
25 or methanol
13 to ene-yne or allenylidene tri-iron derivatives occurs very easily on TLC silica. More recently, our investigations into the behaviour of triruthenium carbonyl complexes containing alkynol units (and ligands derived therefrom) have led to some interesting results in this area of ‘surface organometallic chemistry’. Thus, the parallel bound hydroxyalkyne complexes [Ru3(CO)9(μ-CO){μ3-C(H)
CC(Ph)(R)OH}] 7 are found to undergo decarbonylation and oxidative addition of the alkyne on alumina to yield the corresponding acetylide species [Ru3(CO)9(μ-H){μ3-C
CC(Ph)(R)OH}] 8 (see Scheme 3).3 Further, adsorption of 8b (R = Me) onto silica gel and heating at 100 °C was found to facilitate dehydration of the acetylide unit, yielding small amounts of the ene-yne-containing species [Ru3(CO)9(μ-H){μ3-C
CC(Ph)
CH2}] 9.27 We have now found that similar processes may occur for the allenylidene complexes [Ru3(CO)9(μ-CO){μ3-C
C
C(Ph)R}] 4. Thus, complex 4a (R = Ph) was adsorbed onto silica gel and left at room temperature for 24 h, after which time chromatography afforded the acetylide species [Ru3(CO)9(μ-H){μ3-C
CC(Ph)2OH}] 8a in 20% yield. Employing 4b (R = Me) in the same reaction yielded instead [Ru3(CO)9(μ-H){μ3-C
CC(Ph)
CH2}] 9 (30%), which contains an ene-yne ligand. The complexes 8a and 9 were readily characterised by comparison of their spectroscopic data with those in the literature.26,27 It is clear from the above results that the nature of the alkyne substituents plays a role in determining the manner in which complexes 4 are transformed on silica gel. For complex 4a (R = Ph) the overall process involves loss of a carbonyl unit and addition of water (split into its component parts). However, 4b (R = Me) has formally undergone decarbonylation and hydrogen migration from the alkyl substituent to the cluster edge. Although we have no mechanistic details for the processes our previous results in this area allow us to rule out some of the more likely pathways. It has previously been shown that protonation of the acetylide complex [Ru3(CO)9(μ-H){μ3-C
CC(Me)(Ph)OH}] 8b affords the ene-yne species 9
26,27 and so one might suggest that initially the complexes 4 undergo identical transformations on silica gel to afford the corresponding species [Ru3(CO)9(μ-H){μ3-C
CC(Ph)(R)OH}] 8, with the methyl-substituted complex 8b allowing a further process to occur, yielding the observed ene-yne product 9. However, as mentioned earlier, transformation of 8b to 9 on silica gel has been shown to require high temperatures and affords only trace amounts of product. Similarly, the initial conversion of complexes 4 into the corresponding alkynol species [Ru3(CO)9(μ-CO){μ3-C(H)
CC(Ph)(R)OH}] 7 may be ruled out on the grounds that complexes 7 are stable on silica gel and rearrange only on alumina. Further, if this were the case one would expect employment of shorter reaction times to yield small amounts of 7, which is not the case. We suggest, therefore, that the two silica gel-mediated transformations occur via independent pathways.
 |
| Scheme 3 | |
Experimental
Reactions and general manipulations were performed under a nitrogen atmosphere using deoxygenated solvents, dried by distillation over an appropriate drying agent. Column chromatography was performed on silica gel. Reactions were routinely monitored by IR spectroscopy. Solution IR spectra were recorded on a Perkin-Elmer 1710 Fourier Transform Spectrometer using calcium fluoride cells of 1 mm path length, FAB mass spectra using a Fisons Autospec instrument, proton and carbon-13 NMR spectra on JEOL GX 270 and GX 400 spectrometers. All NMR spectrometers operated in the Fourier Transform mode, with field stability maintained by an external lock system. Elemental analyses were performed by the Microanalytical Laboratory of the School of Chemistry, University of Bristol.
The alkynes but-2-yne (Aldrich) and diphenlyacetylene (Lancaster) were used as supplied. Silica gel 60 (Fluka) of Brockmann Activity 2–3 and particle size 0.2–0.5 mm (35–70 mesh ASTM) was used as supplied in the surface-mediated reactions. The complexes [Ru3(CO)9(μ-CO){μ3-C
C
CPh(R)}] (R = Ph 4a or Me 4b) were prepared by the literature methods.3
Reaction of [Ru3(CO)9(μ-CO)(μ3-C
C
CPh2)] 4a with but-2-yne
A dichloromethane solution (20 cm3) of complex 4a (175 mg, 0.23 mmol) and MeC
CMe (110 μl, 1.40 mmol) was heated at reflux for 5 h. The solvent was removed, under reduced pressure, and the product chromatographed. A yellow band, eluted with CH2Cl2–hexane (1∶5), yielded [Ru3(CO)9{μ3-C(Me)C(Me)C2CPh2}] 5a (54 mg, 30%) as yellow crystals after recrystallisation from heptane at −20 °C [Found: C, 42.36; H, 2.12%; M (mass spectrometry) 800. C28H16O9Ru3 requires C, 42.06; H, 2.02%; M 800]. IR: νCO (heptane) 2081s, 2066vs, 2041vs, 2020m, 2008m, 1998 (sh)m, 1983s, 1968m and 1950w cm−1. 1H NMR (CD2Cl2): δ 7.76–6.74 (9H, m, Ph), 6.06 (1H, dd, J = 2 and 5 Hz), 2.38 (3H, s, Me) and 2.29 (3H, s, Me). 13C NMR (CD2Cl2): δ 200.0, 199.5, 197.9, 197.6, 197.3, 196.5, 188.7 (CO), 172.2 (C15), 171.1 (C11), 141.8 (C14), 137.0, 139.0, 132.8, 128.8, 127.8 (all Ph), 126.0 (C16), 124.7 (C12), 91.2 (C17), 76.0 (C18), 30.7 (μ-CMe) and 16.9 (CMe). Assignments were aided by a DEPT 135° experiment.
Reaction of [Ru3(CO)9(μ-CO)(μ3-C
C
CPh2)] 4a with diphenylacetylene
A dichloromethane solution (35 cm3) of complex 4a (200 mg, 0.26 mmol) and PhC
CPh (138 mg, 0.78 mmol) was heated at reflux for 3 h. Removal of solvent and chromatography, eluting with CH2Cl2–hexane (1∶9), afforded both a yellow and a dark purple band. Recrystallisation of the yellow band from a CH2Cl2 solution layered with hexane afforded [Ru3(CO)9{μ3-C(Ph)C(Ph)C2CPh2}] 5b (48 mg, 20%) as a crystalline solid. Recrystallisation of the dark purple band from a CH2Cl2 solution layered with hexane afforded [Ru4(CO)12(μ4-C(Ph)C(Ph)CCCPh2)] 6 (14 mg, 5%) as a crystalline solid.
Complex 5b.
IR (νCO) in heptane: 2084s, 2071vs, 2044vs, 2025m, 2009m, 1995 (sh)m, 1985s, 1970m and 1951w cm−1. 1H NMR (CD2Cl2): δ 7.74–6.81 (19H, m, Ph) and 6.18 (1H, dd, J = 2 and 5 Hz). 13C NMR (CD2Cl2): δ 201.3, 199.3, 197.6, 195.7, 193.8, 193.5, 189.0 (CO), 171.2 (C15), 169.1 (C11), 142.2 (C14), 141.7, 139.5, 133.1, 132.0, 129.4, 128.7, 128.2, 127.9, 127.8, 127.6, 126.1, 123.8, 121.3 (all Ph), 136.7 (C16), 125.4 (C12), 89.4 (C17) and 74.8 (C18). Assignments were aided by a DEPT 135° experiment. FAB MS: m/z 924 (M+) and 868–672 (M − nCO)+ (n = 2–9). Found: C, 49.36; H, 2.16. Calc. C, 49.41; H, 2.18%.
Complex 6.
IR (νCO) in heptane: 2096s, 2074vs, 2046vs, 2038s, 2028s, 1986m, and 1916w cm−1. 1H NMR (CD2Cl2): δ 7.83–6.92 (m, Ph). FAB MS: m/z 1108 (M+) and 1080–772 (M − nCO)+ (n = 1–12). Found: C, 44.46; H, 1.85. Calc: C, 44.41; H, 1.82%.
Silica gel-mediated transformations of [Ru3(CO)9(μ-CO){μ3-C
C
C(Ph)R}] (R = Ph 4a or Me 4b)
In each of the reactions described below the complexes were adsorbed onto silica gel by mixing a dichloromethane solution of the complex with silica gel in a flask and allowing the solvent to evaporate.
Complex 4a (55 mg, 0.07 mmol) was adsorbed and left at room temperature for 24 h. Chromatography of the reaction mixture, eluting with CH2Cl2–hexane (1∶9), afforded the yellow complex [Ru3(CO)9(μ-H){μ3-C
CC(Ph)2OH}] 8a (11 mg) in a yield of 20%. IR (νCO) in heptane: 2101w, 2075s, 2055s, 2025vs and 1991m cm−1. 1H NMR (CDCl3): δ 7.77–7.24 (m, Ph), 2.64 (s, 1H) and −21.11 (s, 1H).
Complex 4b (50 mg, 0.07 mmol) was adsorbed and left at room temperature for 24 h. Chromatography of the reaction mixture, eluting with CH2Cl2–hexane (1∶8), afforded the yellow complex [Ru3(CO)9(μ-H){μ3-C
CC(Ph)
CH2}] 9 (14 mg) in a yield of 30%. IR (νCO) in heptane: 2099w, 2074s, 2055s, 2047m, 2026s and 1992m. 1H NMR (CD2Cl2): δ 7.64–7.27 (m, Ph), 5.72 (d, 1H), 5.61 (d, 1H) and −20.83 (s, 1H).
Crystal structure determinations of complexes 5a, 5b and 6
Single crystals of complexes 5a, 5b and 6 were grown by diffusion of a hexane layer into dichloromethane solutions. Data were collected at −100 °C on a Bruker SMART diffractometer using graphite-monochromated Mo-Kα radiation. Other important experimental details are collected in Table 3.
|
5a
|
5b
|
6
|
Formula |
C28H16O9Ru3 |
C38H20O9Ru3 |
C41H20O12Ru4 |
M/g mol−1 |
799.6 |
923.8 |
1108.9 |
Crystal system |
Triclinic |
Monoclinic |
Triclinic |
Space group |
P![[1 with combining macron]](https://www.rsc.org/images/entities/char_0031_0304.gif) |
P21/n |
P![[1 with combining macron]](https://www.rsc.org/images/entities/char_0031_0304.gif) |
a/Å |
9.244(1) |
13.279(2) |
9.232(3) |
b/Å |
10.611(1) |
17.374(2) |
14.127(5) |
c/Å |
14.171(2) |
15.161(2) |
15.478(3) |
α/° |
82.23(1) |
|
83.66(2) |
β/° |
84.14(1) |
102.73(1) |
82.26(2) |
γ/° |
78.16(1) |
|
74.02(2) |
U/Å3 |
1344.0(3) |
3411.7(8) |
1917.1(9) |
Z
|
2 |
4 |
2 |
μ/mm−1 |
1.72 |
1.37 |
1.61 |
Total data |
10016 |
35148 |
19421 |
Unique data |
4668 |
7834 |
8649 |
R
int (%) |
3.0 |
3.7 |
3.6 |
Observed data, No |
3783 |
6324 |
6102 |
R1 (observed data) (%) |
2.3 |
2.2 |
2.6 |
wR2 (all data) (%) |
4.8 |
5.2 |
5.5 |
CCDC reference number 186/2248.
See http://www.rsc.org/suppdata/dt/b0/b006780f/ for crystallographic files in .cif format.
Acknowledgements
We acknowledge the European Union (Socrates award to R. Q.-P.) for financial support.
References
- M. I. Bruce, Chem. Rev., 1998, 98, 2797 CrossRef CAS; M. I. Bruce, B. W. Skelton, A. H. White and N. N. Zaitseva, J. Chem. Soc., Dalton Trans., 2000, 881 RSC.
- C. S. Lau and W. T. Wong, J. Chem. Soc., Dalton Trans., 1998, 3391 RSC.
- J. P. H. Charmant, P. Crawford, P. J. King, R. Quesada-Pato and E. Sappa, J. Chem. Soc., Dalton Trans., 2000, 4390 RSC.
- M. I. Bruce, B. W. Skelton, A. H. White and N. N. Zaitseva, Inorg. Chem. Commun., 1999, 2, 17 CrossRef CAS.
- F. A. Cotton and J. M. Troup, J. Am. Chem. Soc., 1974, 96, 1233 CrossRef CAS; F. A. Cotton and J. M. Troup, , 1974, 96, 123 Search PubMed.
- L. Messerle and M. D. Curtis, J. Am. Chem. Soc., 1980, 102, 7789 CrossRef CAS.
- See, for example: J. P. H. Charmant, G. Davies, P. J. King, J. R. Wigginton and E. Sappa, Organometallics, 2000, 19, 2330 CrossRef CAS; M. I. Bruce, P. A. Humphrey, B. W. Skelton, A. H. White, K. Costuas and J.-F. Halet, J. Chem. Soc., Dalton Trans., 1999, 479 RSC; S. Rivomanana, C. Mongin and G. Lavigne, Organometallics, 1996, 15, 1195 CrossRef CAS.
- E. Sappa, J. Organomet. Chem., 1999, 573, 139 CrossRef CAS and refs. therein..
- See, for example: I. Moldes, T. Papworth, J. Ros, A. A. Larena and J. F. Piniella, J. Organomet. Chem., 1995, 489, C65 CrossRef CAS; M. I. Bruce, P. J. Low, B. W. Skelton and A. H. White, J. Organomet. Chem., 1994, 464, 191 CrossRef CAS; F. E. Hong, I. R. Lue, S. C. Lo and C. C. Lin, J. Organomet. Chem., 1995, 495, 97 CrossRef CAS.
- L. J. Farrugia, N. MacDonald and R. D. Peacock, Polyhedron, 1998, 17, 2877 CrossRef CAS.
- A. A. Koridze, V. I. Zdanovich, F. M. Dolgushin, A. I. Yanovsky, Y. T. Struchkov and P. V. Petrovskii, Izv. Akad. Nauk SSSR, Ser. Khim., 1994, 2049 Search PubMed.
- A. A. Koridze, A. M. Sheloumov, F. M. Dolgushin, A. I. Yanovsky, Y. T. Struchkov and P. V. Petrovskii, Russ. Chem. Bull., 1996, 45, 702 Search PubMed and refs. therein..
- G. Gervasio, D. Marabello and E. Sappa, J. Chem. Soc., Dalton Trans., 1997, 1851 RSC.
- G. Gervasio and E. Sappa, Organometallics, 1993, 12, 1458 CrossRef CAS.
- E. Sappa, G. Predieri, A. Tiripicchio and F. Ugozzoli, Gazz. Chim. Ital., 1995, 125, 51 Search PubMed.
- R. J. McKinney, J. Chem. Soc., Chem. Commun., 1980, 490 RSC.
- C. O. Arean, E. E. Platero, G. Spoto and A. Zecchina, J. Mol. Catal., 1989, 56, 211 CrossRef.
- See, for example: R. S. Dickson, P. J. Fraser and B. M. Gatehouse, J. Chem. Soc., Dalton Trans., 1972, 2287 RSC; R. E. Colborn and K. P. C. Vollhart, J. Am. Chem. Soc., 1981, 103, 6259 CrossRef CAS; C. H. Patterson, J. M. Munclenar, P. Y. Timbrell, A. J. Gellman and R. M. Lambert, Surf. Sci., 1989, 208, 93 CrossRef CAS; L. A. Brady, A. F. Dyke, S. E. Garner, S. A. R. Knox, A. Irving, S. M. Nicholls and A. G. Orpen, J. Chem. Soc., Dalton Trans., 1993, 487 RSC.
- F. Biagini, A. M. Caporusso, T. Funailoli and C. Fachinetti, Angew. Chem., Int. Ed. Engl., 1989, 28, 1009 CrossRef.
- N. E. Shore, Chem. Rev., 1988, 88, 1081 CrossRef CAS.
- See, for example: S. A. R. Knox, B. R. Lloyd, D. A. V. Morton, A. G. Orpen, M. L. Turner and G. Hogarth, Polyhedron, 1995, 14, 2723 CrossRef CAS; R. Giordano, E. Sappa and G. Predieri, Inorg. Chim. Acta, 1995, 228, 139 CrossRef CAS and refs. therein..
- D. L. Davies, S. A. R. Knox, K. A. Mead, M. J. Morris and P. Woodward, J. Chem. Soc., Dalton Trans., 1984, 2293 RSC.
- Y. Chi, A. J. Carty, P. Blenkiron, E. Delgado, G. D. Enright, W. Wang, S.-M. Peng and G.-H. Lee, Organometallics, 1996, 15, 5269 CrossRef CAS.
-
S. Deabate
, P. J. King
and E. Sappa
, in Metal Clusters in Chemistry, eds. P. Braunstein, L. Al Oro and P. R. Raithby, Wiley-VCH, Weinheim, 1999, vol. 2, pp. 796–843.
Search PubMed.
- G. Gervasio and E. Sappa, J. Organomet. Chem., 1995, 498, 73 CrossRef CAS.
- G. Gervasio, R. Gobetto, P. J. King, D. Marabello and E. Sappa, Polyhedron, 1998, 17, 2937 CrossRef CAS.
- S. Ermer, R. Karpelus, S. Miurs, E. Rosenberg, A. Tiripicchio and A. M. M. Lanfredi, J. Organomet. Chem., 1980, 187, 81 CrossRef CAS.
|
This journal is © The Royal Society of Chemistry 2001 |