Behaviour of titanium dioxide and zinc oxide nanoparticles in the presence of wastewater-derived organic matter and implications for algal toxicity†
Received
10th October 2014
, Accepted 23rd November 2014
First published on 24th November 2014
Abstract
The properties of engineered nanomaterials (ENMs), such as their small size and increased reactivity, enable their use in a wide range of products; however, these attributes may also pose an environmental hazard. Wastewater effluent is expected to be a significant source of ENMs to the aquatic environment. Little is known about the behaviour and effect of ENMs in this complex matrix. The aim of this study was to assess the effect of titanium dioxide (TiO2) and zinc oxide (ZnO) ENMs on microalgae Pseudokirchneriella subcapitata in wastewater effluent and compare with more commonly tested matrices, specifically Talaquil growth media and Suwannee River humic acid. To better understand the toxicity results, ENM concentration, size and streaming potential in the different experimental matrices were assessed. The different media types had a significant influence on TiO2 behaviour, with TiO2 settling out of solution within the first 24 hours in wastewater. However, as TiO2 was not toxic to algae at the studied concentration, no difference in effect was observed between media, humic acid and wastewater. In contrast, media types had less of an influence on ZnO behaviour, with growth inhibition observed in all three media types at 72 hours, although the presence of organic matter delayed inhibition slightly. The results demonstrate that organic matter properties can have a significant influence on ENM behaviour, and while this did not translate into an effect on algae in the current study, it is still important to consider ENM behaviour and fate when working in different matrices.
Nano impact
ENMs are expected to enter the aquatic environment via wastewater effluent; however, many toxicity studies are not conducted under environmentally realistic conditions. Here, we assessed the behaviour of TiO2 and ZnO ENMs in wastewater-derived organic matter and their effect on algae for the first time and compared their behaviour in humic acid and media. Organic matter type had a significant effect on TiO2 stability and aggregation, but TiO2 did not have an adverse effect on algae under any studied conditions. In contrast, the behaviour and effect of ZnO was not significantly influenced by organic matter. Given the significant difference in TiO2 behaviour, this study demonstrates the importance of using environmentally relevant matrices for ENM toxicity assessment.
|
Introduction
Engineered nanomaterials (ENMs), such as zinc oxide (ZnO), titanium dioxide (TiO2), silver nanoparticles and carbon nanotubes, are increasingly used in areas such as energy and electronics, as well as everyday consumer products including cosmetics, paints and clothing. The worldwide annual production of TiO2 was estimated to be up to 10
000 tonnes, while 100–1000 tonnes of ENMs such as ZnO and carbon nanotubes are expected to be produced per year.1 Nanomaterials are defined2 as having any external dimensions or internal or surface structure on the nanoscale, i.e., the size ranges from approximately 1 nm to 100 nm, and this means that they often exhibit properties different from their bulk form. For example, ENMs have a larger surface area to volume ratio than the bulk material, which can cause increased reactivity and this may increase the formation of reactive oxygen species and cause oxidative stress in exposed organisms.3 Consequently, the same properties that have enabled ENMs to be applied in a wide range of products may pose a toxicological hazard.
ENMs are expected to enter the environment during product manufacturing, use and disposal.4 A number of studies have demonstrated that ENMs can be released from products including clothing5 and painted facades.6,7 Further, the use of ENMs in sunscreens and cosmetics suggests that ENMs will reach wastewater treatment plants (WWTPs), with loads expected to increase with greater ENM usage. Pilot and full scale WWTP studies indicate that the majority of ENMs will be removed by conventional wastewater treatment processes (literature summarised by Neale et al.8 and Brar et al.9); however, some fraction of ENMs are still passing through WWTPs and have been detected in wastewater effluent.10,11 Due to difficulties with detecting low concentrations of ENMs in complex matrices, much of the ENM exposure data comes from modelling studies. The predicted levels in wastewater effluent range from nanogram per litre levels for ENMs such as silver and carbon nanotubes to microgram per litre levels for more extensively used ENMs such as TiO2 and ZnO.12,13
In recent years, numerous studies have explored the effect of a range of ENMs on aquatic organisms, including fish, crustaceans, bivalves and algae.14–16 Reported effects are wide ranging and include mortality, malformation, growth inhibition, immobilisation and reduced reproductive success. However, observed effects often occur at concentrations two to five orders of magnitude greater than detected or expected ENM environmental concentrations.8 Further, the observed effect can vary significantly depending on factors such as ENM preparation method, experimental protocol and ENM coating.17
The majority of ENM effect studies are conducted in pure water or media, with only a limited number conducted in the presence of organic matter.18,19 Organic matter, including humic and fulvic acids, has been shown to alter the fate and behaviour of ENMs, altering stability and leading to heteroaggregation20–22 or alternatively promoting disagglomeration.23 Changes in aggregation and/or agglomeration can have implications for toxicity. For example, the presence of commercial humic acid changed the charge properties of TiO2 ENMs, reducing physical interactions between TiO2 and the test organism, algae, and consequently decreasing TiO2 toxicity.24 In contrast, Wang et al. showed that Suwannee River fulvic acid increased the toxicity of copper oxide (CuO) ENMs to algae by reducing agglomeration/aggregation and facilitating increased release of free copper ions.25 Many studies on this area focus on reference organic matter, including International Humic Substances Society standards, but, to the authors' knowledge, no studies have been conducted in wastewater, with the exception of Kang et al. who studied the effect of carbon based ENMs on microbial communities in wastewater effluent.26 Wastewater-derived organic matter can differ greatly from reference organic matter due to its microbial origin,27 and this may influence ENM behaviour and fate, which in turn can have implications for toxicity.
The aim of this study was to investigate the behaviour and effect of ENMs in wastewater effluent. This was compared with experimental media commonly used for toxicity experiments and reference humic acid. TiO2 and ZnO ENMs were selected for this study as they are used in a wide range of products and have been detected in wastewater effluent at microgram per litre levels.11,28 Further, ZnO ENMs can release free zinc ions,29 while TiO2 ENMs are relatively insoluble, and this can influence toxicity. ENM behaviour was assessed by measuring concentration over time and assessing changes in particle diameter and streaming potential, while growth and photosynthesis inhibition of microalgae Pseudokirchneriella subcapitata was studied in the presence of ENMs. Microalgae were selected as the test species as they represent the bottom of the aquatic food chain; thus effects on algae could have implications for higher organisms.
Materials and methods
Chemicals
All chemicals were of analytical grade. The herbicide terbutryn (Chem Service Inc, West Chester, USA) was used as a positive control for the photosynthesis inhibition experiments. ZnCl2 (Sigma Aldrich, Castle Hill, Australia) was used as an experimental control in the ZnO experiments. Suprapur nitric acid (65%) (Merck-Millipore, Kilsyth, Australia) was used for inductively coupled plasma mass spectrometry (ICP-MS) analysis.
Engineered nanomaterials (ENMs)
Two different ENMs were investigated in this study, TiO2 and ZnO, all in dry powder form. The TiO2 sample was Aeroxide P25 (Sigma Aldrich, Castle Hill, Australia), which is a mix of the crystallographic forms anatase and rutile (78% anatase, 14% rutile) and has a nominal particle diameter of 21 nm (manufacturer's information). Two different ZnO samples (NM-110 and NM-112) were studied, which consisted of materials recently analysed in an OECD project.30 The particle size distribution (PSD) of NM-110 ranges from 70 to 200 nm, with a mean size of 100 nm, while NM-112 has a nominal PSD ranging from 30 to 50 nm. Further details about NM-110 and NM-112 can be found in Singh et al.31
The ENM stock suspensions were prepared in algae growth media by stirring in a darkened environment for approximately 18 hours. The concentration of the TiO2 stock was 1000 mg L−1, and the concentrations of the ZnO stocks were 5000 mg L−1, which were diluted to working stocks of 200 mg L−1 (normalised to Zn concentration). Stirring was selected over other preparation methods as it is considered more environmentally relevant.32 Sonication is often used for stock preparation, but it can cause artefacts in toxicity testing.33 The influence of the preparation method, including overnight stirring and sonication, on TiO2 particle diameter is shown in Fig. S1 of the ESI.† Freshly prepared stocks were used for each experiment to minimise the effect of changes (e.g. aggregation/agglomeration, dissolution) during storage. An initial comparison of the stability of the TiO2 dispersions after different preparation methods was performed by visual observation in wastewater, Talaquil growth media and ultrapure water (18 MΩ cm) (Fig. S2 to S4†). These results indicated that the majority of the particles in wastewater and Talaquil growth media sedimented out of the suspension rapidly, whereas the sample suspended in ultrapure water remained stable for much longer.
Organic matter
Sixty litres of secondary treated effluent were collected from a wastewater treatment plant in South East Queensland (April 2013). On the day of collection, the water was filtered with a 1.6 μm glass fibre filter followed by a 0.45 μm nylon filter. The pH, which was 8.1, was adjusted to 2 using HCl and the water was stored at 4 °C in acid washed 20 L plastic containers for up to 8 months. Prior to experiments the water was sterile filtered with a 0.22 μm polyethersulfone filter and readjusted to the original pH using NaOH. The organic carbon concentration of the wastewater after 0.22 μm filtration was 9.25 mgC L−1 and was analysed using an Analytik Jena Multi N/C 310. Further wastewater characterisation can be found in Section S3 of the ESI.† Suwannee River humic acid (2S101H) (International Humic Substances Society, St Paul, US) was selected as the reference organic matter.
ENM Characterisation
Laser diffraction.
The particle diameter of TiO2 and ZnO was assessed in the presence of growth media, humic acid and wastewater using a Malvern Mastersizer 3000 (Malvern, UK). The wastewater experiments were conducted in the presence or absence of P. subcapitata (1 × 104 cells mL−1). For the data analysis, a refractive index of 1.33 was used for the solutions, while refractive indices of 2.49 and 2.01 were used for the TiO2 and ZnO ENMs, respectively. The samples were stirred at 2000 RPM during the entire measurement cycle to ensure that the particles remained suspended and ENMs were added until a suitable obscuration of the laser signal was reached (4 to 6.5% depending on the ENM type). Measurements were taken every 10 minutes over a period of an hour.
Streaming potential.
To assess if the stability of the suspension could be further quantified, qualitative measurement of the streaming potential of TiO2 and ZnO ENMs in media, humic acid and wastewater at a fixed pH was assessed using a Stabisizer PMX 200C (Microtrac Europe GmbH, Meerbusch, Germany). The instrument can perform surface potential measurements of particles at higher concentrations and larger sizes than electrophoretic mobility based techniques.34 TiO2 and ZnO ENM stock solutions with a concentration of 10 mg mL−1 were prepared for the streaming potential experiments. Prior to each measurement, 1 mL of stock solution was added to 9 mL of media, humic acid or wastewater. As there are no reference materials available for verification of the streaming potential measurements, an undiluted suspension of commercially available zeta potential quality control material (polystyrene latex particles, Malvern, UK), known to exhibit a stable surface charge, was also measured. A 200 μm gap piston was used and the streaming potential of each sample was measured for 10 minutes, with data collected every 3 seconds.
Inductively coupled plasma mass spectrometry (ICP-MS).
The concentration of ENMs in media (TiO2 only), humic acid and wastewater in the presence of algae over 72 hours was analysed using an Agilent 7500 series ICP-MS. All samples were spiked with an internal standard containing lithium, germanium, indium, beryllium, rhodium, yttrium and scandium. A wastewater effluent certified reference material (BCR-713, European Commission, Geel, Belgium) was also included for analysis. The limits of detection were 0.60 μg L−1 for Ti and 0.64 μg L−1 for Zn and the percent recovery of the spiked compounds in the working range (10–100 μg L−1) ranged from 94–111%.
Highly insoluble Ti was digested using an ammonium persulphate method developed by Khosravi et al.35 Briefly, 2 mL of the sample was collected from the supernatant at the top of the flask at different time points and evaporated to dryness in porcelain crucibles on a hotplate, then covered with 1 g of ammonium persulphate and fusion was performed using a Bunsen burner. The products of the fusion were soaked in 2% HNO3 for 30 minutes and then diluted to a final volume of 20 mL. Ammonium persulphate and wastewater controls were included.
Total Zn concentration in wastewater and humic acid over time was assessed using microwave digestion. Briefly, 1 mL of the sample was collected from the supernatant at different time points and added to 4 mL of 65% HNO3. The sample was digested using the following microwave program at 1400 W: ramp to 125 °C over 10 minutes, ramp up to 175 °C over 15 minutes, then hold at 175 °C for 15 minutes. The sample was then diluted with ultrapure water to a final HNO3 concentration of 2%. Wastewater without ZnO was also digested as an experimental control.
ZnO dissolution to dissolved Zn ions in humic acid and wastewater over time was also assessed by ICP-MS. Briefly, 4.9 mL of the sample was filtered through a 0.1 μm PVDF filter and 0.1 mL of 65% HNO3 was added to give a final HNO3 concentration of 1.3%. While 100 nm is the upper end of the nominal ENM size range, the laser diffraction results indicated that the size of ZnO in solution was much larger than 100 nm (see Results and discussion), thus the particulate ZnO should be retained by the filter while dissolved Zn should pass through. Similar methods to assess Zn dissolution have been applied previously by Franklin et al.29 and Fabrega et al.36
Algae toxicity testing
Green microalgae P. subcapitata was selected to assess growth and photosynthesis inhibition by the studied ENMs. The algae were cultured in Talaquil growth media37 in an incubator (22 °C, 90 RPM) under constant fluorescent lighting. The experiments were conducted in 100 mL autoclaved Erlenmeyer flasks with 50 mL of Talaquil growth media, humic acid or wastewater. A starting algal density of 1 × 104 cells mL−1 was added to each flask. To facilitate algae growth, concentrated humic acid stock was diluted in Talaquil growth media to achieve a final humic acid concentration of 10 mgC L−1, while the wastewater was supplemented with 5 mL of 10 times enriched Talaquil growth media. This diluted the wastewater concentration to 8.3 mgC L−1. For the ZnO experiments the Talaquil media were prepared without ethylenediaminetetraacetic acid (EDTA). Details on the differences in Talaquil media between TiO2 and ZnO ENM experiments can be found in Section S4.† Concentration range finder experiments in Talaquil growth media were conducted with TiO2 concentrations from 0.1 to 10 mg L−1 and ZnO concentrations from 0.05 to 2 mg L−1 (Fig. S6 and S7†), with the majority of experiments in the study conducted at 1 mg L−1 TiO2 and 0.1 mg L−1 ZnO (normalised to Zn concentration). All experiments were conducted in three replicates.
Algae growth was assessed using an Accuri C6 flow cytometer (BD, Franklin Lakes, US). Due to the presence of chlorophyll, P. subcapitata is autofluorescent above λ = 600 nm. Therefore, fluorescence detector 3 (λ > 670 nm) was used and the algae peak was gated to separate it from interference from ENMs or organic matter (example shown in Fig. S8†). Cell count was assessed at 0, 24, 48 and 72 hours. The data were reported as growth inhibition relative to the control, which was calculated using eqn (1):
| 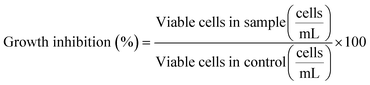 | (1) |
Photosynthesis inhibition was assessed using Maxi-Imaging-PAM (IPAM, Walz GmbH, Germany). The photosynthetic yield (Y) of the samples and controls was measured at 24, 48 and 72 hours. The data were reported as photosynthesis inhibition relative to the control, which was calculated using eqn (2):
|  | (2) |
Results and discussion
Behaviour of TiO2 ENMs in wastewater, media and humic acid
The fate and behaviour of TiO2 ENMs in the different matrices was assessed by monitoring concentration over time and changes in particle size (due to agglomeration and/or aggregation) and streaming potential. The concentration experiments were conducted under identical conditions as the toxicity tests, while the additional characterisation experiments were conducted over shorter time periods, but were used to provide further information about the behaviour of the ENMs in media, humic acid and wastewater. The different experimental matrices had a dramatic effect on TiO2 ENM behaviour, with both ICP-MS analysis and laser diffraction indicating that TiO2 ENMs were not stable in wastewater. The results shown in Fig. 1A illustrate that after 24 hours in wastewater the majority of Ti was lost from the supernatant, with the Ti concentration the same as the wastewater background levels (Fig. S9A†). In contrast, as little as 25% of Ti was lost from the supernatant over 72 hours in the presence of humic acid (Fig. 1A). The laser diffraction results showed that the median TiO2 particle diameter (D50) increased rapidly over time in wastewater, and in the presence of algae particle diameter further increases (Fig. 2A and S10†). In contrast, the TiO2 appeared to be more stable in humic acid compared to media. Laser diffraction has been previously used to assess ENM aggregation in the presence of organic matter.38 Despite the nominal nanometre size range of the ENMs, the laser diffraction analysis indicated that in all studied samples, particles were likely to form agglomerates and/or aggregates in the micrometre size range under the different experimental conditions (Fig. 2). Results from Taurozzi et al.39 indicated that a significant amount of ultrasonic energy is required to break up the agglomerates that are likely to be present. As sonication was not applied in the current study, it was not possible to differentiate between agglomerates and aggregates.
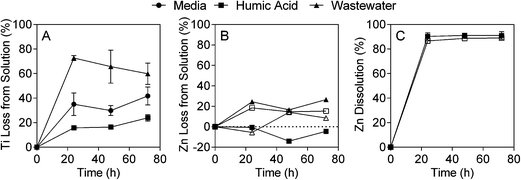 |
| Fig. 1 ENM percent loss and percent dissolution over time in the algae toxicity tests. A) Ti loss from solution (%) in media, humic acid and wastewater; B) Zn loss from solution (%) in humic acid and wastewater and C) Zn dissolution (%) in humic acid. In B) and C) the closed symbols are NM-110 and the open symbols are NM-112. | |
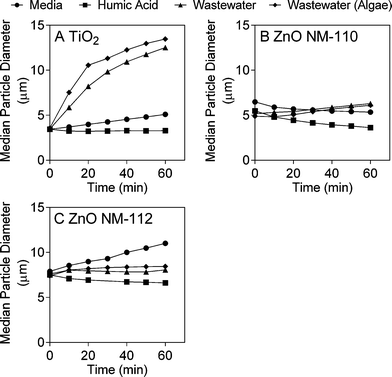 |
| Fig. 2 Median particle diameter (D50) in media, humic acid and wastewater (with and without algae) of A) TiO2, B) ZnO NM-110 and C) ZnO NM-112. | |
The lack of stability of TiO2 ENMs in wastewater was further supported by the streaming potential measurements. While the surface potential of TiO2 ENMs in media and humic acid remained relatively constant over the 10 minute measurement, there was a larger difference in streaming potential for TiO2 in wastewater, compared to wastewater alone, which indicates that the TiO2 ENMs were unstable and likely to aggregate (Table S2†). Keller et al. explored the behaviour of TiO2 in a number of water sources and found that stabilisation was promoted by high organic carbon content and low ionic strength.40 In the current study the organic carbon concentration was slightly higher in the humic acid experiments than in the wastewater experiments (10 vs. 8.3 mgC L−1). Previous studies have suggested that humic acid can coat TiO2 particles, increasing electrostatic repulsion and preventing aggregation/agglomeration.24 The organic carbon properties of wastewater are very different from those of humic acid, with more low molecular weight neutrals and biopolymers,27 and these may have a reduced affinity for TiO2. Further, the presence of ions in the wastewater may shield the TiO2 negative charge, leading to increased aggregation/agglomeration. The conductivity of the wastewater was 2.3 mS cm−1 (after pH adjustment), while conductivity of the algae growth media was 1.0 mS cm−1. The presence of divalent ions, such as calcium, has previously been shown to have a significant influence on TiO2 stability.41 Divalent ion concentrations were not measured in the current study, but based on past analysis of wastewater effluent in Eastern Australia,42 the concentration of divalent ions in wastewater is likely to be higher than in the other studied matrices.
Behaviour of ZnO ENMs in wastewater, media and humic acid
In contrast to the TiO2 ENMs, both types of ZnO ENMs studied were more stable in wastewater, with around 80% of added Zn remaining in the supernatant after 72 hours (Fig. 1B). It was not clear whether Zn was present in particulate or dissolved form in the ICP-MS experiments as only total Zn was measured. NM-112 had a greater tendency to aggregate over time compared to NM-110, particularly in the growth media experiment, and had a larger average particle size at time zero (Fig. 2B and C). No significant change in surface potential was observed during the 10 minute streaming potential measurements for the ZnO ENMs (Table S2†), which is also reflected in the results from the laser diffraction measurements at 10 minutes, where no discernible size difference was detected (Fig. 2). Singh et al. also found that NM-112 formed larger aggregates in deionised water, seawater and Daphnia magna media compared to NM-110 using differential centrifugal sedimentation.31 The same study also found distinct differences in the zeta potential of the ENMs in the different media types, but there was no significant difference between NM-110 and NM-112, which agrees with the observations in the current study. It should be noted that the sample preparation applied by Singh et al. included ultrasonication, which was not performed in the current study.31
It should also be noted that due to the large dilution required after microwave digestion, the ZnO ENM fate experiments were conducted at 20 mgZn L−1 (200 times higher than the toxicity experiments), in contrast to the TiO2 ENM fate experiments, which were conducted at the same concentration as the toxicity experiments (1 mg L−1).
ZnO ENM dissolution experiments in humic acid indicated that around 90% of dissolved Zn was released from both NM-110 and NM-112, with the majority of dissolution occurring within the first 24 hours (Fig. 1C). The rate of ZnO ENM dissolution in algal toxicity experiments varies substantially within the literature (e.g. 4–50%) and can be influenced by media composition, algae concentration, pH and ZnO ENM concentration.43,44 For example, Zhao et al. found that ZnO ENM dissolution increased with decreasing ZnO ENM concentrations in experiments with D. magna.45 Many studies conduct dissolution experiments at concentrations many times higher than the toxicity experiments, but in the current study, both the dissolution and effect studies were conducted at 0.1 mgZn L−1. Zn dissolution was also assessed in wastewater, but the high wastewater content appeared to interfere with the analysis, leading to apparent elevated Zn concentrations (Fig. S9C†).
Effect of TiO2 ENMs in wastewater, media and humic acid
Despite the differences in stability, TiO2 ENMs had no adverse effect on algae growth or photosynthesis in media, humic acid or wastewater, with some experiments even showing slightly increased growth relative to the control (Fig. 3). Kulacki and Cardinale also observed increased growth of a range of algal species in the presence of TiO2 ENMs.46 To validate the experimental setup, the experiments were conducted in parallel with positive control herbicide terbutryn, which inhibits photosynthesis with a 50% effect concentration (EC50) of 7.24 μg L−1.47 Fig. S11† shows significant growth and photosynthesis inhibition by terbutryn in media, humic acid and wastewater, indicating that the different test matrices were not interfering with the measurement technique.
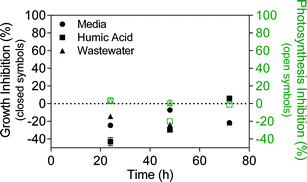 |
| Fig. 3 Algae growth inhibition (closed symbols) and photosynthesis inhibition (open symbols) in the presence of 1 mg L−1 TiO2 in Talaquil growth media, humic acid and wastewater. Growth and photosynthesis inhibition were calculated using eqn (1) and (2), respectively. | |
The experiments were conducted at a concentration of 1 mg L−1 TiO2, which is around 50 times higher than detected TiO2 ENM concentrations in wastewater effluent.11 TiO2 ENM toxicity towards P. subcapitata varies within the literature, with 72 hour growth EC50 values ranging from 2.5 to 241 mg L−1 TiO2.43,48–50 The considerable variation may be related to preparation method, extent of TiO2 aggregation/agglomeration, media type and TiO2 crystal form (anatase, rutile), although some studies lack appropriate characterisation of TiO2 ENMs, making the results difficult to interpret. Further, TiO2 is known to be photoreactive and may induce formation of reactive oxygen species (ROS) under light conditions.51 This can be problematic for algal toxicity testing, given that algae require light for growth. However, Miller et al. found that TiO2 ENMs were only toxic to marine phytoplankton under UV light conditions, with most artificial fluorescent lights emitting little UV light.52
Effect of ZnO ENMs in wastewater, media and humic acid
In contrast to the TiO2 ENMs, both types of ZnO ENMs caused growth inhibition in all studied matrices (Fig. 4). The effect was similar to that of the positive control ZnCl2. To compare the effect of ZnO to ZnCl2, all results for ZnO ENMs were normalised to Zn concentration. Hence 0.1 mgZn L−1 translates to 0.12 mg L−1 for ZnO, which is around 80 times higher than the concentration of ZnO ENMs previously detected in wastewater effluent.28 The presence of organic matter appeared to initially delay growth inhibition of both ZnO ENMs and ZnCl2 in the humic acid and wastewater experiments, but growth inhibition is similar to media after 72 hours. Photosynthesis inhibition relative to the control was much lower than growth inhibition, indicating that photosynthesis inhibition was not an important toxicity mechanism. Instead, an indirect toxicity mechanism, specifically the release of dissolved Zn, is more likely to explain the observed growth inhibition, given the similarity in effect between ZnO ENMs and ZnCl2. This is also supported by the ZnO ENM dissolution experiments in humic acid. Dissolved Zn can interfere with the cell membrane of green algae by disrupting calcium uptake.53 A number of studies have attributed ZnO ENM toxicity to P. subcapitata to the release of dissolved Zn,29,48 with EC50 values around 0.04 to 0.07 mg L−1.
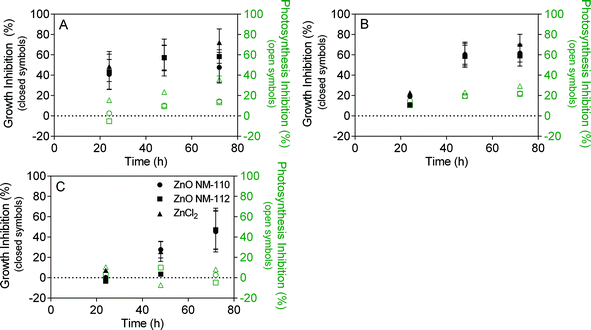 |
| Fig. 4 Algae growth inhibition (closed symbols) and photosynthesis inhibition (open symbols) in the presence of 0.1 mgZn L−1 ZnO (NM-110 or NM-112) in A) Talaquil growth media, B) humic acid, and C) wastewater. 0.1 mgZn L−1 ZnCl2 acted as a positive control. Growth and photosynthesis inhibition were calculated using eqn (1) and (2), respectively. | |
There was considerably more variability associated with the results of the effect study for ZnO ENMs compared to TiO2 ENMs due to the absence of EDTA from the test media. EDTA is often used in algae growth media as it can improve the test performance,54 but EDTA is able to complex free metal ions and thus can underestimate toxicity from dissolved Zn. The algae doubling time in the control experiments with and without EDTA was similar for media and humic acid, but algae doubling time was greatly increased in wastewater without EDTA, meaning lower algae counts after 72 hours (Fig. S12 and Table S3†). This is most likely due to the presence of trace metals in the secondary treated effluent that were bound by EDTA in the TiO2 experiments, but were bioavailable in the EDTA free experiments. ICP-MS analysis of wastewater control after microwave digestion indicated that most of the targeted metals (e.g. lead and arsenic) were similar to the experiment blank (2% HNO3 in ultrapure water), with the exception of manganese, which was over 10 times higher than the blank.
Conclusions
This study aimed to explore the influence of wastewater-derived organic matter and reference organic matter on the behaviour and effect of two common ENMs, TiO2 and ZnO. The findings are ENM specific. TiO2 ENMs behaved very differently in wastewater compared to humic acid. While the majority of TiO2 remained in solution in humic acid after 72 hours (only 25% reduction in concentration), the TiO2 concentration in the wastewater supernatant was similar to background levels after 24 hours. Additional characterisation confirmed that TiO2 was not stable in wastewater, with aggregation and/or agglomeration and subsequent sedimentation likely to contribute to the loss from solution. However, as TiO2 was non-toxic to P. subcapitata at the studied concentration, no difference in effect was observed between humic acid and wastewater, despite humic acid stabilising the TiO2 ENMs.
In contrast, both studied ZnO ENM types caused growth inhibition of algae, but this was attributed to the release of dissolved Zn, rather than the nanoparticle form. Both humic acid and wastewater initially suppressed the effect of ZnO ENMs compared to media, but growth inhibition was similar in all matrices after 72 hours. ZnO ENMs appeared to be slightly more stable in humic acid than in wastewater, but this did not translate to a significant difference in effect.
In conclusion, while organic matter did have an influence on ENM fate, the current study did not observe any difference in effect on algae. However, the altered behaviour in wastewater may have implications for the toxicity of other ENMs and this warrants further investigation. In addition, a limitation of this study is that pristine ENMs were used whereas ENMs that reach WWTPs are likely to be transformed by chemical, physical and biological processes.55 Nevertheless, this study clearly demonstrates the importance of working with realistic environmental matrices when investigating ecotoxicity of ENMs.
Acknowledgements
The National Research Centre for Environmental Toxicology (Entox) is a joint venture of The University of Queensland and Queensland Health. This study was supported by the Australian Research Council (ARC) Linkage Project funding scheme (LP100200276) with industry partners Seqwater, Water Research Australia Limited and Veolia Water Australia and The University of Queensland Collaboration and Industry Engagement Fund. Elissa O'Malley was supported by a Water Research Australia scholarship. Victoria Coleman (National Measurement Institute Australia) is acknowledged for useful discussions. Lixia Qi (Entox) is thanked for assistance with ICP-MS analysis.
References
- F. Piccinno, F. Gottschalk, S. Seeger and B. Nowack, J. Nanopart. Res., 2012, 14 Search PubMed.
- ISO, ISO/TS 80004–1:2010, Nanotechnologies -- Vocabulary -- Part 1: Core terms, International Organization for Standardization, Geneva, 2010 Search PubMed.
- T. Xia, M. Kovochich, J. Brant, M. Hotze, J. Sempf, T. Oberley, C. Sioutas, J. I. Yeh, M. R. Wiesner and A. E. Nel, Nano Lett., 2006, 6, 1794–1807 CrossRef CAS PubMed.
- B. Nowack, J. F. Ranville, S. Diamond, J. A. Gallego-Urrea, C. Metcalfe, J. Rose, N. Horne, A. A. Koelmans and S. J. Klaine, Environ. Toxicol. Chem., 2012, 31, 50–59 CrossRef CAS PubMed.
- T. M. Benn and P. Westerhoff, Environ. Sci. Technol., 2008, 42, 4133–4139 CrossRef CAS.
- R. Kaegi, B. Sinnet, S. Zuleeg, H. Hagendorfer, E. Mueller, R. Vonbank, M. Boller and M. Burkhardt, Environ. Pollut., 2010, 158, 2900–2905 CrossRef CAS PubMed.
- R. Kaegi, A. Ulrich, B. Sinnet, R. Vonbank, A. Wichser, S. Zuleeg, H. Simmler, S. Brunner, H. Vonmont, M. Burkhardt and M. Boller, Environ. Pollut., 2008, 156, 233–239 CrossRef CAS PubMed.
- P. A. Neale, A. K. Jamting, B. I. Escher and J. Herrmann, Water Sci. Technol., 2013, 68, 1440–1453 CrossRef CAS PubMed.
- S. K. Brar, M. Verma, R. D. Tyagi and R. Y. Surampalli, Waste Manage., 2010, 30, 504–520 CrossRef CAS PubMed.
- D. M. Mitrano, E. K. Lesher, A. Bednar, J. Monserud, C. P. Higgins and J. F. Ranville, Environ. Toxicol. Chem., 2012, 31, 115–121 CrossRef CAS PubMed.
- P. Westerhoff, G. X. Song, K. Hristovski and M. A. Kiser, J. Environ. Monit., 2011, 13, 1195–1203 RSC.
- A. A. Keller and A. Lazareva, Environ. Sci. Technol. Lett., 2013, 1, 65–70 CrossRef.
- T. Y. Sun, F. Gottschalk, K. Hungerbuhler and B. Nowack, Environ. Pollut., 2014, 185, 69–76 CrossRef CAS PubMed.
- F. Schwab, T. D. Bucheli, L. P. Lukhele, A. Magrez, B. Nowack, L. Sigg and K. Knauer, Environ. Sci. Technol., 2011, 45, 6136–6144 CrossRef CAS PubMed.
- A. J. Bone, B. P. Colman, A. P. Gondikas, K. M. Newton, K. H. Harrold, R. M. Cory, J. M. Unrine, S. J. Klaine, C. W. Matson and R. T. Di Giulio, Environ. Sci. Technol., 2012, 46, 6925–6933 CrossRef CAS PubMed.
- L. Canesi, R. Fabbri, G. Gallo, D. Vallotto, A. Marcomini and G. Pojana, Aquat. Toxicol., 2010, 100, 168–177 CrossRef CAS PubMed.
- R. D. Handy, G. Cornelis, T. Fernandes, O. Tsyusko, A. Decho, T. Sabo-Attwood, C. Metcalfe, J. A. Steevens, S. J. Klaine, A. A. Koelmans and N. Horne, Environ. Toxicol. Chem., 2012, 31, 15–31 CrossRef CAS PubMed.
- K. Van Hoecke, K. A. C. De Schamphelaere, S. Ramirez-Garcia, P. Van der Meeren, G. Smagghe and C. R. Janssen, Environ. Int., 2011, 37, 1118–1125 CrossRef CAS PubMed.
- A. J. Edgington, A. P. Roberts, L. M. Taylor, M. M. Alloy, J. Reppert, A. M. Rao, J. D. Mao and S. J. Klaine, Environ. Toxicol. Chem., 2010, 29, 2511–2518 CrossRef CAS PubMed.
- A. Praetorius, M. Scheringer and K. Hungerbühler, Environ. Sci. Technol., 2012, 46, 6705–6713 CrossRef CAS PubMed.
- J. T. K. Quik, I. Velzeboer, M. Wouterse, A. A. Koelmans and D. van de Meent, Water Res., 2014, 48, 269–279 CrossRef CAS PubMed.
- L. Chekli, S. Phuntsho, M. Roy, E. Lombi, E. Donner and H. K. Shon, Water Res., 2013, 47, 4585–4599 CrossRef CAS PubMed.
- F. Loosli, P. Le Coustumer and S. Stoll, Environ. Sci.: Nano, 2014, 1, 154–160 RSC.
- D. H. Lin, J. Ji, Z. F. Long, K. Yang and F. C. Wu, Water Res., 2012, 46, 4477–4487 CrossRef CAS PubMed.
- Z. Wang, J. Li, J. Zhao and B. Xing, Environ. Sci. Technol., 2011, 45, 6032–6040 CrossRef CAS PubMed.
- S. Kang, M. S. Mauter and M. Elimelech, Environ. Sci. Technol., 2009, 43, 2648–2653 CrossRef CAS.
- P. A. Neale, A. Antony, W. Gernjak, G. Leslie and B. I. Escher, Water Res., 2011, 45, 4227–4237 CAS.
- S. M. Majedi, H. K. Lee and B. C. Kelly, Anal. Chem., 2012, 84, 6546–6552 CrossRef CAS PubMed.
- N. M. Franklin, N. J. Rogers, S. C. Apte, G. E. Batley, G. E. Gadd and P. S. Casey, Environ. Sci. Technol., 2007, 41, 8484–8490 CrossRef CAS.
- OECD, Safety of manufactured nanomaterials, <http://www.oecd.org/env/ehs/nanosafety/>, accessed 29th May 2014.
-
C. Singh, S. Friedrichs, M. Levin, R. Birkedal, K. A. Jensen, G. Pojana, W. Wohlleben, S. Schulte, K. Wiench, T. Turney, O. Koulaeva, D. Marshall, K. Hund-Rinke, W. Kördel, E. Van Doren, P.-J. De Temmerman, M. Francisco, J. Mast, J. Gibson, N. R. Koeber, T. Linsinger and C. L. Klein, NM-Series of Representative Manufactured Nanomaterials: Zinc Oxide NM-110, NM-111, NM-112, NM-113 Characterisation and Test Item Preparation, EUR 25066 EN - 2011, 2011 Search PubMed.
- E. Oberdorster, S. Q. Zhu, T. M. Blickley, P. McClellan-Green and M. L. Haasch, Carbon, 2006, 44, 1112–1120 CrossRef PubMed.
- E. J. Petersen, T. B. Henry, J. Zhao, R. I. MacCuspie, T. L. Kirschling, M. A. Dobrovolskaia, V. Hackley, B. Xing and J. C. White, Environ. Sci. Technol., 2014, 48, 4226–4246 CrossRef CAS PubMed.
- Particle Metrix GmbH, Particle Metrix, <http://www.particle-metrix.de/en/home.html>, accessed 9th October 2014.
- K. Khosravi, M. E. Hoque, B. Dimock, H. Hintelmann and C. D. Metcalfe, Anal. Chim. Acta, 2012, 713, 86–91 CrossRef CAS PubMed.
- J. Fabrega, R. Tantra, A. Amer, B. Stolpe, J. Tomkins, T. Fry, J. R. Lead, C. R. Tyler and T. S. Galloway, Environ. Sci. Technol., 2012, 46, 1128–1135 CrossRef CAS PubMed.
- S. Le Faucheur, R. Behra and L. Sigg, Environ. Toxicol. Chem., 2005, 24, 1731–1737 CrossRef CAS.
- A. Praetorius, J. Labille, M. Scheringer, A. Thill, K. Hungerbühler and J.-Y. Bottero, Environ. Sci. Technol., 2014, 48, 10690–10698 CrossRef CAS PubMed.
- J. S. Taurozzi, V. A. Hackley and M. R. Wiesner, Nanotoxicology, 2013, 7, 389–401 CrossRef CAS PubMed.
- A. A. Keller, H. T. Wang, D. X. Zhou, H. S. Lenihan, G. Cherr, B. J. Cardinale, R. Miller and Z. X. Ji, Environ. Sci. Technol., 2010, 44, 1962–1967 CrossRef CAS PubMed.
- J. A. Gallego-Urrea, J. Perez Holmberg and M. Hassellöv, Environ. Sci.: Nano, 2014, 1, 181–189 RSC.
- A. Antony, N. Subhi, R. K. Henderson, S. J. Khan, R. M. Stuetz, P. Le-Clech, V. Chen and G. Leslie, J. Membr. Sci., 2012, 407–408, 8–16 CrossRef CAS PubMed.
- W.-M. Lee and Y.-J. An, Chemosphere, 2013, 91, 536–544 CrossRef CAS PubMed.
- S. W. Y. Wong, P. T. Y. Leung, A. B. Djurisic and K. M. Y. Leung, Anal. Bioanal. Chem., 2010, 396, 609–618 CrossRef CAS PubMed.
- X. Zhao, S. Wang, Y. Wu, H. You and L. Lv, Aquat. Toxicol., 2013, 136, 49–59 CrossRef PubMed.
- K. J. Kulacki and B. J. Cardinale, PLoS One, 2012, 7, e47130 CAS.
- J. Y. M. Tang and B. I. Escher, Environ. Toxicol. Chem., 2014, 33, 1427–1436 CrossRef CAS PubMed.
- V. Aruoja, H.-C. Dubourguier, K. Kasemets and A. Kahru, Sci. Total Environ., 2009, 407, 1461–1468 CrossRef CAS PubMed.
- N. B. Hartmann, F. Von der Kammer, T. Hofmann, M. Baalousha, S. Ottofuelling and A. Baun, Toxicology, 2010, 269, 190–197 CrossRef CAS PubMed.
- D. B. Warheit, R. A. Hoke, C. Finlay, E. M. Donner, K. L. Reed and C. M. Sayes, Toxicol. Lett., 2007, 171, 99–110 CrossRef CAS PubMed.
- H. Ma, A. Brennan and S. A. Diamond, Environ. Toxicol. Chem., 2012, 31, 2099–2107 CrossRef CAS PubMed.
- R. J. Miller, S. Bennett, A. A. Keller, S. Pease and H. S. Lenihan, PLoS One, 2012, 7, e30321 CAS.
- K. L. Wilde, J. L. Stauber, S. J. Markich, N. M. Franklin and P. L. Brown, Arch. Environ. Contam. Toxicol., 2006, 51, 174–185 CrossRef CAS PubMed.
-
U.S. Environmental Protection Agency, Short-Term Methods for Estimating the Chronic Toxicity of Effluents and Receiving Waters to Freshwater Organisms (Fourth Edition), 2002 Search PubMed.
- G. V. Lowry, K. B. Gregory, S. C. Apte and J. R. Lead, Environ. Sci. Technol., 2012, 46, 6893–6899 CrossRef CAS PubMed.
Footnotes |
† Electronic supplementary information (ESI) available. See DOI: 10.1039/c4en00161c |
‡ Current address: Smart Water Research Centre, Griffith University, Southport QLD 4222, Australia. |
§ Current address: Cell Toxicology, Helmholtz Centre for Environmental Research – UFZ, Leipzig, Germany. |
|
This journal is © The Royal Society of Chemistry 2015 |