DOI:
10.1039/D0NH00207K
(Focus)
Nanoscale Horiz., 2020,
5, 1355-1367
Ultrasmall Au nanoclusters for bioanalytical and biomedical applications: the undisclosed and neglected roles of ligands in determining the nanoclusters' catalytic activities
Received
11th April 2020
, Accepted 29th July 2020
First published on 29th July 2020
Abstract
Significantly different from conventional Au nanoparticles, ultrasmall Au nanoclusters (NCs) consisting of several to about a hundred Au atoms with a size below 2 nm exhibit a strong quantum confinement effect, and possess an intriguing molecular-like highest occupied molecular orbital (HOMO)–lowest unoccupied molecular orbital (LUMO) transition, quantized charging, intrinsic chirality, and special fluorescence properties, as well as high catalytic activities. In virtue of their unique molecular-like electronic structure, remarkable physicochemical properties, mild preparation conditions and good biocompatibility, Au NCs have been having a profound impact on bioanalytical and biomedical applications, such as biosensing, biological imaging, cell markers, drug delivery, photodynamic/photothermal therapy, and biomedical toxicology. As an indispensable part of Au NCs, shell ligands not only stabilize and protect the structure of Au NCs, but also have an important influence on the structure and biocatalytic activities of Au NCs. Nevertheless, the effect of shell ligands on the biocatalytic activities of Au NCs has not been paid much attention or even ignored. In this Focus article, thus, the structure and biocatalytic activities of Au NCs are discussed from the perspective of the shell ligands. Particular emphasis is directed to the discussion and exploration of the undisclosed and neglected roles of shell ligands in the biocatalytic activities of Au NCs, which are of fundamental importance to the unraveling of charge transfer behaviors and biocatalytic processes of Au NCs. In addition, the future directions to explore the mechanism of shell ligands affecting the biocatalytic activities of Au NCs, such as surface ligand engineering of Au NCs, advanced surface/interface in situ characterization techniques, theoretical analysis, and the nanobiology of Au NCs, are also put forward.
1. Introduction
In recent years, the rapid development of nanotechnology has brought new opportunities to the field of biomedicine and biological analysis. Biotechnological techniques based on nanomaterials have significantly improved the detection sensitivity and specificity. In particular, gold nanoclusters (Au NCs) have attracted particular attention due to their unique optical, electrical and chemical properties.1,2 Au NCs typically consist of several to about a hundred Au atoms, ranging in size from a single atom to a nanocrystal, with nuclear clusters less than 2 nm in diameter.3 Since the size of Au NCs is similar to the Fermi wavelength of the electrons, the continuous state density is decomposed into discrete energy levels, making them significantly different from ordinary gold nanoparticles (Au NPs) (>2 nm in diameter) and bulk gold materials (Fig. 1)4,5 in terms of their optical, chemical, and electrical properties.6,7 Thanks to the strong quantum confinement effect, Au NCs possess intriguing molecular-like highest occupied molecular orbital (HOMO)–lowest unoccupied molecular orbital (LUMO) transition,8,9 quantized charging,10 intrinsic chirality,11 and special fluorescence properties,12,13 as well as high catalytic activity.6,14 In virtue of their advantages15,16 including ultrasmall size, good fluorescence stability, fluorescence adjustability, large Stokes shift, mild preparation conditions, and biocompatibility, Au NCs have been widely studied in the bioanalytical and biomedical field, such as biosensing, biological imaging, cell markers, drug delivery, the detection of biological molecules (e.g., DNA, protein and enzyme), and photodynamic/photothermal therapy, with great application prospects. It is noteworthy that these bio-related applications are usually inseparable from the biocatalytic activities of Au NCs. Different from industrial biocatalysis,17 nevertheless, the biocatalytic activities of Au NCs in this Focus article refers to the abilities of Au NCs to speed up (catalyze) the biochemical reaction processes in a variety of biological environments.
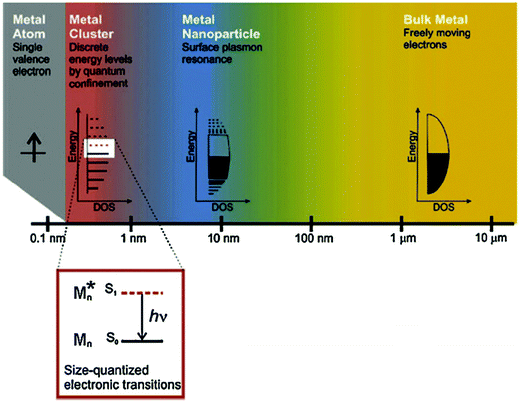 |
| Fig. 1 The effect of size on metals. Whereas bulk metal and metal nanoparticles have a continuous band of energy levels, the limited number of atoms in metal nanoclusters results in discrete energy levels, allowing interaction with light by electronic transitions between energy levels. Metal nanoclusters bridge the gap between single atoms and nanoparticles. Reprinted from ref. 4 with permission. Copyright 2010 Springer-Verlag. | |
In view of the fact that Au is a chemically stable noble metal, it was not considered to have biocatalytic activity in the early stage. With the emergence of Au NPs, especially Au NCs,10,18 the biocatalytic activity of Au has attracted extensive attention in the field of biology and has made considerable research progress. At present, the biocatalytic activity of Au NCs is mainly reflected in the following aspects. Firstly, the unique fluorescence phenomenon generated by its quantum confinement effect9,19–22 is used as a novel fluorescent probe for biomolecular detection, biological imaging and cell identification. Under a specific biological environment, the enrichment of Au NCs and the biochemical reactions involved in Au NCs will significantly affect the fluorescence intensity of Au NCs. Therefore, a change in fluorescence intensity of Au NCs can reflect to some extent the biochemical reactions in which Au NCs are involved. The second is to use its unique core–shell structure consisting of a core of zero-valent gold atoms (Au0) and a shell of monovalent gold ions (Au+) to promote the process of charge transfer.23–25 The rapid charge transfer process within the core–shell structure of Au NCs can effectively promote the biochemical reactions between Au NCs and the surrounding biological components, which would further facilitate the recognition and detection of target analyte molecules, thus leading to the improvement of the sensitivity and selectivity of biosensor technology. Thirdly, under specific conditions, Au NCs also have certain enzyme-like catalytic activity,26,27 which can simulate some reactions catalyzed by biological enzymes. In such cases, Au NCs usually participate directly in biocatalytic reactions. For example, gold nanoclusters stabilized using lysozyme show oxidase activity, which is expected to become a new substitute of peroxidase in the field of biological analysis.26 Lastly and most importantly, Au NCs produce photoacoustic or photothermal effects due to the occurrence of fluorescence resonance energy transfer (FRET) when contacting with some functional structures or molecules.16,28,29 Following light absorption, the photoacoustic or photothermal effects produced from Au NCs are generally related to the photophysical processes and photochemical reactions, in which some key processes, such as photon absorption and conversion of the absorbed pulsed or modulated radiation into phonons or heat energy, are accomplished through Au NCs. These effects have been widely used for photoacoustic imaging and photodynamic or photothermal therapy in the bioanalytical and biomedical fields especially in oncology.30 For example, Cui et al.28 developed Au NC-indocyanine green (ICG) nanoprobes by the conjugation of Au NCs with ICG for combined photoacoustic tumor imaging, therapy, and therapeutic monitoring in real time based on FRET. As is known, due to the quantum-size effect, “bare” Au NCs have very active chemical properties, which require the use of chemically synthesized molecules or biomolecules as ligands to stabilize and protect their surfaces, thus avoiding the aggregation of NCs and the loss of surface activity.14 Apparently, the biocatalytic activities of Au NCs are significantly affected by the size of the NCs, and the types and structural characteristics of the surface ligands.
However, it is worth paying attention to the point, that is, in the process of studying the biocatalytic properties of the atomically precise Au NCs, many researchers generally focus on the catalytic activities of Au NCs themselves, but often ignore the role of the ligands in the biocatalytic activities of Au NCs. In fact, the ligands have important impacts on the structural features of the NCs and have been also revealed to play an important role in other catalytic processes of Au NCs31,32 including thermal catalysis,33,34 photocatalysis35,36 and electrocatalysis.37,38 Unlike the other catalytic reactions that require external factors (e.g., heat for thermal catalysis, light for photocatalysis, and electric fields for electrocatalysis), the biocatalytic process of Au NCs usually requires no external factors and involves a biochemical reaction that takes place in a specific biological environment. Due to the lack of external factors, the biocatalytic process of Au NCs is relatively difficult to occur and is more easily influenced by the shell structure of Au NCs (e.g., the hydrophilicity, the biocompatibility, the charge transfer properties, and the stability of the ligands). Thus, the biocatalytic activities of Au NCs are closely related to the ligands on the shell structure of NCs. Unfortunately, this correlation has not been paid attention to or even ignored. In this Focus article, we will discuss the biocatalytic activities of Au nanoclusters from the perspective of ligands, and put forward some personal viewpoints.
2. Why atomically precise Au nanoclusters?
2.1 Are atomically precise nanoclusters really superior to conventional nanoparticles?
From the existing research findings, it can be deduced that the biocatalytic activity of Au NCs is significantly better than that of conventional Au NPs. In terms of reactivity, Au NCs, with an ultrasmall particle size (<2 nm), possess much larger specific surface areas than Au NPs, and thus more active sites are present in NCs. Benefiting from the high surface binding energy of Au NCs, these active sites are more likely to adsorb and bind to the surrounding reactants, which would be favorable for the biocatalytic process.39 In relation to Au NPs, Au NCs exhibit specific fluorescence properties, better water solubility and biocompatibility, which makes Au NCs more likely to react with the substances in the surrounding biological environment for the biocatalytic process. This means that Au NCs are more suited for biological analysis, biological diagnosis and therapy than Au NPs. From the perspective of biological toxicity, Au NPs probably produce severe toxicity and side effects due to their accumulation in the liver and spleen, while Au NCs can be effectively removed from the body through renal clearance with no obvious toxicity and side effects to the human body.40–42 In addition, catalytic reactions usually occur on the catalyst surface, where the reactant atoms interact with each other at the active sites for the catalytic process. Since Au NCs are ultrasmall nanoclusters composed of a small number of Au atoms, from the perspective of fundamental mechanism understanding, they are supposed to be the ideal research unit for exploring the biocatalytic mechanism of Au both in theoretical simulation and experimental methods,43 which would help to deepen the understanding of the biocatalytic reaction processes and intrinsic mechanism of Au from the atomic scale. Last but not least, in terms of cost performance, the use of Au NCs can not only achieve higher catalytic activity than Au NPs, but also reduce the amount of precious metal Au to a large extent, thus greatly reducing the application cost.44
Apparently, Au NCs exhibit superior biocatalytic activities, although there are still many problems to be solved, such as the precise regulation of Au atoms in Au NCs, the intrinsic correlation between the ligand structure and catalytic performance, as well as the catalytic mechanism.
2.2 What determines the stability of atomically precise Au nanoclusters?
The premise for the application of Au NCs is to prepare stable ultrasmall Au NCs. According to the synthetic path, the reported preparation methods of Au NCs can be classified into “bottom-up” and “top-down” approaches.45 In the former, the precursor ions (Au3+) are reduced to Au atoms (Au0) under the action of reducing agent, and the resulting Au0 atoms are further combined with the ligands and then nucleated to grow into Au NCs. In the latter, the large Au NPs are etched by appropriate ligands to obtain ultrasmall NCs. From the viewpoint of reduction methods, the Au NC preparation methods can be further divided into chemical reduction,46 photoreduction,47 ultrasonic reduction,48 microwave reduction,49 ligand exchange methods,50etc. For details on the preparation methods of Au NCs, please refer to some excellent review articles.6,45,51,52
Regardless of the synthetic method, the reactants for the preparation of Au NCs usually contain ligand molecules in addition to the Au3+ precursor. During the reaction process, the ligand molecules combine with the Au3+ precursor ions, and eventually form the Au NCs consisting of the nucleus of Au0 atoms and the shell of the semi-ring Au(I)/ligand staples or ligand molecules, as schematically illustrated in Fig. 2. For example, several groups53–55 confirmed the semi-ring Au(I)/ligand staples on the surface of Au NCs by using advanced mass spectrometric techniques. In general, the stability of Au NCs depends on both the external environment (e.g., solution pH and temperature) and the internal structure, but the latter is fundamental for its stability. Therefore, the structural stability of Au NCs is mainly governed by the geometric structure of the Au atoms, the structure of the protecting ligand molecules, and the interaction between the two. The geometric structure of Au atoms determines the nucleus of NCs,56 while the ligand structure is closely related to the surface properties (e.g., hydrophilicity,57 polarity,58 chirality,59 and functional modifiability60,61) of NCs, and the interaction between the two is reflected in the electronic and geometric properties of NCs.62
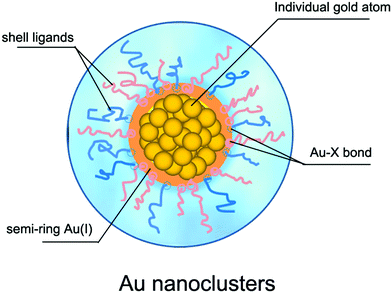 |
| Fig. 2 Schematic illustration of the structure of Au nanoclusters. | |
3. What do we really know about the role of ligands in the nanoclusters' biocatalytic activities?
3.1 Origin of the biocatalytic activity of Au nanoclusters
People have never stopped exploring the origin of the biocatalytic activities of Au NCs. In the early stage, it was generally accepted that the biocatalytic activities of Au NCs were closely related to their fluorescence performances, which were mainly dependent on the Au atomic core instead of the ligand shell because the ligands only served to protect and stabilize the core. The most direct evidence is that the naked Au NCs, only composed of several Au atoms, have been confirmed to possess an obvious photoluminescence (PL) phenomenon, and the number of Au atoms also has an important influence on their PL performance.63,64 For example, Fedrigo et al.63 detected the PL properties of small naked Au NCs under inert atmosphere. Zheng et al.65 experimentally revealed that the PL performance of Au NCs varied with the number of Au atoms, and the Jellium model (EFermi/N1/3, where EFermi is the Fermi energy of bulk gold and N is the number of Au atoms)13 could on the whole reflect the internal correlation between the number of Au atoms and the PL of small sized Au NCs, although the model would be offset to some extent when the number of Au atoms was large.66 In addition, the PL performance of Au NCs was also demonstrated to change significantly when other metal atoms (e.g., Ag67 and Cu68) partially substituted the Au atoms in the core of Au NCs, implying that the PL of Au NCs originated from the core of Au NCs. For instance, Wang et al.67 revealed that the PL intensity of AgxAu25−x (x = 13) NCs obtained by partially replacing Au atoms with Ag atoms was about 200 times that of Au25 NCs (Fig. 3). Under the influence of these early viewpoints, the role of the ligand shell in the biocatalytic activities of Au NCs has been ignored for a long time.
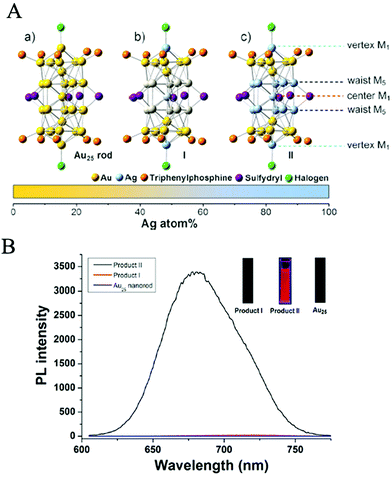 |
| Fig. 3 (A) X-ray structures of: (a) Au25 NCs, (b) Product I (Ag12Au13 NCs), and (c) Product II (Ag13Au12 NCs). M = metal atom. (B) Photoluminescence spectra of Au25NCs (bottom, blue line), Product I (Ag12Au13 NCs) (middle, red line) and Product II (Ag13Au12 NCs) (top, black line), the inset is the fluorescence digital photo of Au25, Product I and Product II excited by 365 nm UV light, respectively. Reproduced from ref. 58 with permission. Copyright 2014 Wiley-VCH. | |
With the development of research studies in recent years, it has been proven that the biocatalytic activities of Au NCs are not only determined by the Au atomic core, but also greatly influenced by the shell of the ligand molecules. For Au NCs with the same Au atomic core, the use of different shell ligands usually leads to the formation of Au NCs with isomerized structures, resulting in significantly different spectral absorption properties and ultimately affecting their catalytic activities, as exemplified in the work of Jin's group69 (Fig. 4). In some cases, the biocatalytic activities of Au NCs even come directly from the shell of the ligands. The influence of ligand shell on the biocatalytic activities of Au NCs is mainly reflected in the following aspects. Firstly, ligand molecules are bound to Au(I) via chemical bonds (e.g., Au–S) to form a semi-ring structure at the outer layer of the Au(0) core. The resulting semi-ring structure can, on the one hand, protect and stabilize the Au(0) core, and on the other hand also provide the charge transfer channel (i.e., the ligands to the metal cluster core charge transfer (LMCCCT)) for the biocatalytic process. The LMCCCT would effectively promote the interfacial charge transfer and separation, and thus change the fluorescence and biocatalytic performance of Au NCs. Link et al.,70 for example, revealed that Au25(SR)18 NCs exhibited two distinct characteristic fluorescence phenomena, in which the weak visible fluorescence emission (quantum yield (QY) = ∼10−6–10−7) originated from the Au(0) kernel structure while the other strong near-infrared fluorescence emission (QY = ∼1 × 10−3) originated from the semi-ring structure of the Au NCs. Interestingly, Miller et al.71 also observed two charge transfer processes with significantly different migration rates within the Au NCs, that is, the extremely fast charge transfer process (<200 fs) in the Au(0) kernel and the relatively slow charge transport process (∼1.2 ps) in the semi-ring structure, further revealing the important influence of the ligands on the fluorescence and biocatalytic reaction processes of Au NCs. Secondly, some ligands, as the shells of Au NCs, are rich in donor electrons, which easily provides non-localized electrons that induce fluorescence and biocatalytic activities.72,73 For example, glutathione-protected Au NCs exhibited excellent fluorescence performance, mainly because of the presence of electron-rich atoms (e.g., O, N) or atom groups (e.g., –COOH, –NH2) in the glutathione ligands that greatly promoted the LMCCCT process.73 Thirdly, some ligands, especially some proteins, used as shells have their own biocatalytic activities, while stabilizing and protecting the Au(0) core.74–77 For example, Au NCs prepared with histidine as shell ligands showed peroxidase-like catalytic activity,75 while the introduction of bilirubin oxidase (BOD) into Au NCs as the shell ligands endowed the Au NCs with the catalytic activity for the oxygen reduction reaction (ORR).76 Lastly, the shell ligands of Au NCs can be further coupled with other functional molecules with biocatalytic activity (e.g., fungicides,78 folic acid,79,80 and anticancer drugs81) to form functionally-modified Au NCs, thus enabling the biocatalytic activities of Au NCs. Based on the preparation of DT-Au NCs with 1-dodecanethiol (DT) as the shell, for instance, Chen et al.78 further introduced a bactericidal polypeptide molecule (i.e., surfactin (SFT)) on the surface of DT-Au NCs and thus prepared SFT/DT-Au NCs. The obtained SFT/DT-Au NCs possessed excellent biocompatibility and bactericidal properties, enabling the rapid healing of the methicillin-resistant S. aureus infected wounds in mice.
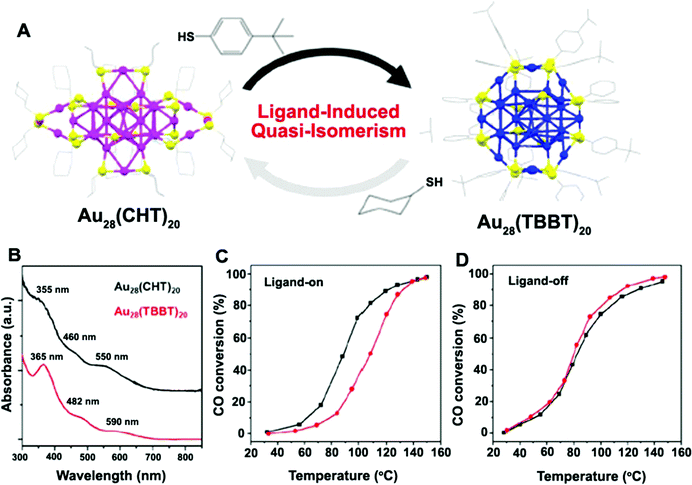 |
| Fig. 4 (A) Ligand-induced quasi-isomerism in Au28(CHT)20 and Au28(TBBT)20, (CHT: cyclohexanethiolate; TBBT: 4-tert-butylbenzenethiolate); (B) optical absorption spectra of Au28(CHT)20 (black) and Au28(TBBT)20 (red). (C and D) CO oxidation light-off curves for CeO2-supported Au28(CHT)20 (black) and Au28(TBBT)20 (red) catalysts (C) pretreated with O2 at 150 °C for 1 h or (D) pretreated with O2 at 300 °C for 1 h to remove ligands. Reproduced from ref. 60 with permission. Copyright 2016 American Chemical Society. | |
3.2 Ligand species reported so far
The utilization of the capping/stabilizing ligands has been demonstrated to reduce and arrange gold atoms into stable Au NCs. In order to obtain stable and monodisperse Au NCs, the shell ligands are indispensable and must also meet some requirements: (i) good structural stability, (ii) a relatively strong binding force with Au atoms, (iii) good solubility, and (iv) confined space. To date, most of the reported shell ligands are bound with the Au atoms through strong Au–S bonds to form Au NCs,82–84 while the other few ligands are bound with the Au atoms through weak bonds (such as phosphine,85 alkynyl86). Apparently, the weakly-bonded shell ligands of the Au-NCs have poor stability, and some of them may detach from the surface of Au atoms in solution, thus leading to the formation of “semi-naked” or “fully naked” Au atomic clusters. These naked Au atomic clusters without the protective layer are easy to agglomerate and no longer have the structural characteristics of Au NCs. For some catalytic reactions, however, the naked Au atomic clusters can instead provide more active sites, which would be conducive to the catalytic processes.6
Currently, there are many kinds of capping/protecting ligands used for the preparation of Au NCs, mainly including small organic molecules (e.g., thiolate,52,87 phosphine85,88), dendrimer or polymer,89 biological molecules (such as peptides,90 protein,19 DNA76,91), etc. In comparison, when used as shell ligands, small organic molecules are more competitive for the design and synthesis of Au NCs, because the structure of Au NCs can be easily regulated by changing the chain length, polarity or functional groups of the small organic molecules. For example, when alkanethiols are used as shell ligands, changing their chain length can produce Au NCs with different structures, thus resulting in different fluorescence effects92 (Fig. 5). When using dendrimers or polymers as shell ligands, it is difficult to regulate the structure of Au NCs because of their large and complex molecular structure. The dendrimer or polymer shell generally occupies much more space than the space occupied by the Au(0) core, resulting in poor monodispersity of Au NCs, and meanwhile the number of Au atoms in the Au NCs is not easy to determine.6 Consequently there are few reports on this type of Au NC. Since the first report on the preparation of Au NCs obtained by using bovine serum albumin (BSA) as the shell ligands,18 many biomolecules have been used as shell ligands for the preparation of Au NCs, including proteins (e.g., BSA,19,93 lysozyme,94 human transferrin,95 lactoferrin,96 trypsin,97 horseradish peroxidase98), peptides,90,99,100 DNA,76,101etc. The Au NCs protected by the bio-ligands have several unique advantages: (i) the improved biocompatibility of Au NCs, (ii) the introduction of surface functional groups (e.g., –NH2, –COOH) into the Au NCs, and (iii) the possible biocatalytic activities originated from some shell bio-ligands (e.g., enzyme98). These advantages can facilitate the applications of Au NCs in bioanalytical and biomedical fields.
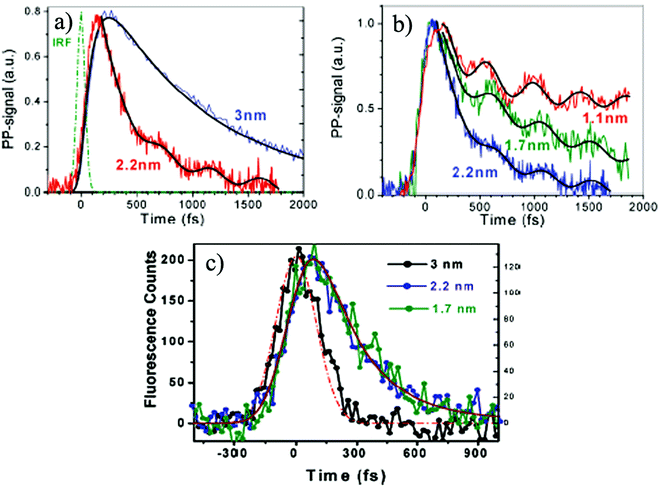 |
| Fig. 5 (a) Demonstration of different transient absorption dynamics for Au NCs with the cluster sizes of 2.2 and 3 nm; (b) decay profiles with oscillatory features for Au NCs with the cluster sizes of 1.1, 1.7 and 2.2 nm; (c) time-resolved fluorescence profiles for different Au NCs with the cluster sizes of 1.7, 2.2 and 3 nm measured at 530 nm. Reproduced from ref. 83 with permission. Copyright 2010 American Chemical Society. | |
3.3 Ligand's role in the nanoclusters' biocatalytic activities
Ligands are an indispensable part of Au NCs and play an important role in the biocatalytic activities of Au NCs. In the process of biocatalytic reaction, ligands, on one hand, are the stable and protective layers of Au NCs, and on the other hand, are the link between Au NCs and biological media for mass migration and charge transfer, which largely determines the biocompatibility and biocatalytic activities of Au NCs. Now the question is, how much do we know about the roles of the ligands?
3.3.1 What is known?.
Firstly, the shell ligands are the biocatalytic reaction interface of Au NCs, because the shell ligands are in direct contact between the Au NCs and environmental media. Generally speaking, the biocatalytic reaction occurs in solution, while the processes of mass migration and charge transfer between Au NCs dispersed in solution and the biological media are accomplished through the shell ligands. Meanwhile, the dispersity of Au NCs also depends on their shell ligands. The better the dispersity of the Au NCs, the more the shell ligands exposed to solution will be, which means that the more biocatalytic reaction interface provided by Au NCs, the more favorable the biocatalytic reaction will be.
Secondly, shell ligands provide reactive sites for the biocatalytic process of Au NCs, because shell ligands usually contain many functional groups that can participate in the biocatalytic process. When a biocatalytic process occurs, Au NCs can adsorb and interact with the biological analytes/objects in the solution through some functional groups existing on the surface of the shell ligands, and the charge transfer process between Au NCs and the biological analytes/objects is also realized. The more reactive sites provided by ligands, the higher the biocatalytic reactivity of Au NCs is.
Thirdly, shell ligands can be used to regulate the biocatalytic selectivity of Au NCs, due to the structural modulability of shell ligands. For a specific biocatalytic reaction process, specific reaction sites can be introduced into ligands by selecting appropriate shell ligands or modifying ligands with functional groups. Accordingly, it can induce a specific biocatalytic reaction process and greatly improve the biocatalytic selectivity of Au NCs. For example, the introduction of antibodies on the shell ligand surface of the Au NCs would lead to a specific biological reaction with the receptor in the biological medium, showing excellent biocatalytic selectivity.102–104
In addition, shell ligands can improve the biocompatibility of Au NCs in biocatalytic reactions. When a biocatalytic process occurs, Au NCs are bound to interact with various biological molecules, cells or tissues. Therefore, the biocompatibility of Au NCs is crucial. In recent years, the use of various biological molecules (e.g., proteins, peptides, DNA, as mentioned above) as the shell ligands of Au NCs has greatly improved the biocompatibility of Au NCs and further reduced the biological toxicity.101
3.3.2 What is not known?.
Although Au NCs show excellent biocatalytic activities and in recent years have been widely used for bioanalytical and biomedical applications, the biocatalytic mechanism of Au NCs is not clear, and there are many problems that remain to be further explored. In particular, the exploration on the effect of the shell ligands on the biocatalytic activities of Au NCs is one of the problems that needs to be addressed.
Shell ligands have diverse structural features. When a biocatalytic reaction occurs, the shell ligands of Au NCs interact with environmental media, resulting in the diversification of shell ligands. The diversity of shell ligands not only depends on their own molecular structures, but also is closely related to the environmental media (e.g., spatial charge distribution of shell ligands, polarity or solubility of ligands, and spatial distance of adjacent Au NCs). However, whether their own molecular structures or external factors determine the diversity characteristics of shell ligands has not been determined yet, and further research is needed.
As is well-known, Au NCs, different from the metallic Au NPs, have a molecular-like electronic structure, that is, the HOMO–LUMO structure,8,9 which is an essential intrinsic factor in determining their charge transfer behavior in the biocatalytic reaction. However, it is not clear how shell ligands participate in the formation of the HOMO–LUMO electronic structure of Au NCs and how they affect the electronic structure. Moreover, the influence of shell ligands on the charge transfer behavior of Au NCs during the biocatalytic reaction processes is also a blind spot, which needs to be further explored. According to Marcus Theory105 formula:
where
kET is the electron transfer rate,
AET is the rate constant, Δ
G is free energy changes,
R is the ideal gas constant, and
T is the absolute temperature.
AET declines exponentially with the distance between the donor and receptor, and thus
kET decreases exponentially. This indicates that the size of the shell ligands significantly affects the charge transfer rate of Au NCs. Theoretically, the larger the shell ligands, the smaller the charge transfer rate of Au NCs. However, when a biocatalytic reaction process occurs, the charge transfer rate of Au NCs is not only affected by the size of the ligands, but also by their surface charge. Moreover, the shell ligands are always in a dynamic process, which also affects the charge transfer process of Au NCs. Therefore, from the perspective of the reaction mechanism, it is still unknown what effect the shell ligands have on the charge transfer behavior of Au NCs and their biocatalytic reaction kinetics.
As mentioned above, when a biocatalytic reaction occurs, Au NCs usually adsorb biological analytes/objects through the shell ligands and interact with them, and at the same time the charge transfer behavior is also generated between the two. Apparently, shell ligands are the link between Au NCs and the bioanalyte/object, and also the bridge of charge transfer between the two in the biocatalytic reaction processes. Studies have shown that the charge transfer at the interface between Au NCs usually involves electron hopping106,107 or quantum tunneling behavior.108,109 However, the role of the shell ligands in the charge transfer process (e.g., electron hopping or quantum tunneling) between Au NCs and bioanalytes/objects is still unknown, and the impact of shell ligands on the charge transfer mechanism of Au NCs is also unclear. In other words, shell ligands can generally induce the surface charge redistribution of Au NCs. What needs to be clarified is the difference between the charge distribution induced by ligands and the charge transfer mechanism of Au NCs.
The spatial structure of the shell ligands determines the actual particle size of Au NCs and affects the biocatalytic activities of Au NCs. Though the reported synthesis processes can control the particle size of Au NCs to some extent, they cannot accurately regulate the amount and surface charge of shell ligands on the surface of Au NCs, resulting in poor reproducibility of Au NCs. As a result, the spatial structure of shell ligands for different Au NCs is diverse, thus affecting the biocatalytic activities of Au NCs. Therefore, it is a great challenge for Au NCs to precisely regulate the spatial structure of shell ligands through the synthetic process, so as to control the structure and biocatalytic activities of Au NCs. Undoubtedly, this is also one of the urgent problems to be solved.
The biocatalytic activities of Au NCs are closely related to the microscopic states (e.g., the distribution density, the uniformity, and the surface charge) of shell ligands on the surface of Au NCs. However, the existing characterization equipment and techniques are still unable to detect the microscopic states of the shell ligands of Au NCs, resulting in our limited understanding on the microscopic states of shell ligands. Therefore, currently it is still difficult to establish the intrinsic correlation between shell ligands and the biocatalytic activities of Au NCs. Nevertheless, this is a problem that must be solved to explore the influence of ligands on the biocatalytic activities of Au NCs.
4. Summary and future prospects
Au nanoclusters (NCs), due to their unique electronic structure, excellent fluorescence performance, good biocompatibility and ability to be removed by renal clearance, have shown more excellent biocatalytic activities than Au NPs. In recent years, Au NCs have attracted extensive attention and have been widely applied in the field of biological analysis and biomedicine. As an important part of Au NCs, shell ligands not only stabilize and protect the structure of Au NCs, but also have important effects on the structure and performance of Au NCs. In the process of biocatalytic reaction, obviously, shell ligands also affect the biocatalytic activity of Au NCs to a great extent. At present, however, the effect of ligands on the biocatalytic activities of Au NCs has not been paid much attention or even ignored. In this Focus article, from the perspective of ligands, the effect of ligands on the biocatalytic activity of Au NCs is briefly analyzed, and the important role of ligands in the biocatalytic reaction of Au NCs is also discussed. Meanwhile, some unknown problems to be further explored are put forward.
The importance of shell ligands on the structure and biocatalytic activity of Au NCs is self-evident, and thus it is of great significance to reveal the role of shell ligands in the biocatalytic activity of Au NCs. Nevertheless, research toward the effect of shell ligands on the structure and biocatalytic activities of Au NCs remains extremely challenging. Much work remains to be done in facilitating their practical applications of Au NCs and broadening the scope of their bio-related applications in the future. Specific attention should be focused on a range of current challenges, such as facile synthesis and functionalization of stable and monodisperse ligand-protected Au NCs, the determination of the structure of Au NCs at the atomic level, further exploration of the electronic structure of Au NCs, a better understanding of the effect of shell ligands on the charge transfer behavior of Au NCs, and the interpretation of the charge transport characteristics at the interface between the shell ligands and the Au(0) core of Au NCs, or the interface between the shell ligands and the bioanalytes in solution. In view of this, we believe that the following aspects are the future directions to explore the mechanism of shell ligands affecting the biocatalytic activities of Au NCs, as schematically presented in Fig. 6.
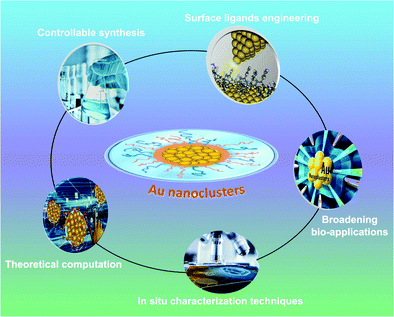 |
| Fig. 6 Schematic illustration of the future directions to explore the mechanisms of shell ligands affecting the biocatalytic activities of Au nanoclusters. | |
(1) Surface ligand engineering of Au NCs
The current synthetic processes of Au NCs seem to be able to effectively regulate the particle size of NCs to a certain extent, but it is far from enough to just regulate the particle size of NCs. This is because the inability to design and regulate the ligands on the Au NCs' surface makes it impossible to achieve the reproducibility of Au NCs' synthesis and even less to regulate their biocatalytic activities, which highlights the importance of surface ligand engineering of Au NCs. The most important content of surface ligand engineering of Au NCs is how to precisely regulate the shell ligands of Au NCs through new synthetic methodologies. The precise regulation of shell ligands is a prerequisite for the controllable synthesis of fine-structure Au NCs, and is also an indispensable condition for subsequent research on the influence mechanism of shell ligands on the biocatalytic activities of Au NCs. In order to realize the precise regulation of ligands on the surface of Au NCs, it is necessary to explore the distribution uniformity, the binding stability, the surface charge state and the three-dimensional spatial structure of ligands, etc., on the surface of Au NCs. All of these belong to the research category of surface ligand engineering of Au NCs.
(2) Development of advanced in situ characterization techniques for surface ligands
In order to understand the mechanism of ligands' influence on the biocatalytic activities of Au NCs, it is necessary to in situ analyze the microstructure and charge distribution of shell ligands. However, the currently-reported nanoscale surface/interface characterization techniques (such as high-resolution transmission electron microscopy (HRTEM), atomic force microscopy (AFM), X-ray photoelectron spectroscopy (XPS), scanning tunneling microscopy (STM), and Kelvin probe microscopy (KPM)) are commonly used to examine the microstructure and morphologies of the NPs themselves, rarely involving the in situ detection of the morphology and charge distribution of ligands on the surface of NPs. Moreover, the shell ligands change dynamically during the biocatalytic reaction of Au NCs, and there are few characterization techniques for the in situ monitoring of the dynamic changes of ligands in real time. It is worth mentioning that single-atom catalysis has become a research hotspot in the field of catalysis in recent years, while the characterization techniques on single-atom catalysts (such as high-angle annular dark field-scanning transmission electron microscopy (HAADF-STEM), X-ray absorption near edge spectroscopy (XANES), and extended X-ray absorption fine structure (EXAFS) spectroscopy) lay more emphasis on the single-atom catalysts themselves than on their surface ligands.110 Therefore, the development of advanced nano-surface/interface in-situ characterization techniques (e.g., surface enhanced Raman spectroscopy (SERS), diffuse reflectance infrared Fourier transform (DRIFT-IR), electrospray ionization mass spectrometry (ESI-MS), matrix-assisted laser desorption/ionization-mass spectrometry (MALDI-TOF-MS), XANES, EXAFS, and surface probes) is very important for the in situ characterizations on the microstructure and charge distribution of shell ligands of Au NCs in biocatalytic reactions. Meanwhile, it is also helpful to reveal the mechanism of shell ligands' influence on the biocatalytic activities of Au NCs.
(3) Theoretical analysis of the shell ligands of Au NCs
At present, there are few theoretical studies on the effect of shell ligands on the charge transfer behavior, biocatalytic activities and reaction kinetics of Au NCs. Therefore, on the basis of the building of a computational model for different ligand-based Au NCs, the theoretical exploration of the intrinsic correlation between the shell ligands and the charge transfer behavior as well as biocatalytic kinetics of Au NCs will help to deepen the understanding of the role of shell ligands in the biocatalytic process of Au NCs, which is of great significance to reveal the biocatalytic mechanism of Au NCs.
(4) Broadening the nanobiological applications of Au NCs
As mentioned above, when a biocatalytic reaction of Au NCs occurs, the shell ligands are in direct contact with the environmental media, which are usually complicated for any biological system. In some biological systems, the interaction of the shell ligands with the environmental media may induce the aggregation of Au NCs, thus leading to the loss of their original physical structures. In other biological systems, the interaction of ligands with the environmental media may contribute to the dispersion and stabilization of Au NCs and thus promote the biocatalytic process. In addition, when Au NCs in the original biological system enter into another new biological system, the interaction of shell ligands with the environmental media also changes, which may promote or inhibit the biocatalytic activities of Au NCs. In other words, the shell ligands of Au NCs largely determine the biological system applicable to Au NCs and even the extended application of Au NCs in other fields. Thus, the clear understanding of the relationship between shell ligands and the biocatalytic activities of Au NCs is of great significance to broaden the nanobiological applications of Au NCs, such as sensing analysis, nanocatalysis, drug therapy and biological toxicity.
Despite these remaining challenges, it is clear that Au NCs offer many advantages for a wide range of bio-related applications, and currently researchers are making rapid progress in this area.
Conflicts of interest
There are no conflicts to declare.
Acknowledgements
This work is financially supported by the National Natural Science Foundation of China (No. 51972294, 21621003, 51872271), National Key Research and Development Program of China (No. 2016YFA0203101), Zhejiang Provincial Natural Science Foundation of China (No. LY19E020003, LQ20F040007), and Tsinghua University Initiative Scientific Research Program.
References
- L. Y. Chen, C. W. Wang, Z. Q. Yuan and H. T. Chang, Fluorescent Gold Nanoclusters: Recent Advances in Sensing and Imaging, Anal. Chem., 2015, 87, 216–229 CrossRef CAS PubMed.
- R. C. Jin, C. J. Zeng, M. Zhou and Y. X. Chen, Atomically Precise Colloidal Metal Nanoclusters and Nanoparticles: Fundamentals and Opportunities, Chem. Rev., 2016, 116, 10346–10413 CrossRef CAS PubMed.
- Y. Z. Lu and W. Chen, Sub-nanometre sized metal clusters: from synthetic challenges to the unique property discoveries, Chem. Soc. Rev., 2012, 41, 3594–3623 RSC.
-
I. Díez and R. Ras, Advanced Fluorescence Reporters in Chemistryand Biology II, Springer, 2010, pp. 307–332 Search PubMed.
- L. B. Zhang and E. K. Wang, Metal nanoclusters: New fluorescent probes for sensors and bioimaging, Nano Today, 2014, 9, 132–157 CrossRef CAS.
- J. Fang, B. Zhang, Q. F. Yao, Y. Yang, J. P. Xie and N. Yan, Recent advances in the synthesis and catalytic applications of ligand protected,atomically precise metal nanoclusters, Coord. Chem. Rev., 2016, 322, 1–29 CrossRef CAS.
- Y. Zhao, Y. Q. Zheng, C. Y. Zhao, J. M. You and F. L. Qu, Hollow PDA-Au nanoparticles-enabled signal amplification for sensitive nonenzymatic colorimetric immune detection of carbohydrate antigen125, Biosens. Bioelectron., 2015, 71, 200–206 CrossRef CAS PubMed.
- A. Fernando, K. L. D. M. Weerawardene, N. V. Karimova and C. M. Aikens, Quantum Mechanical Studies of Large Metal, Metal Oxide, and Metal Chalcogenide Nanoparticles and Clusters, Chem. Rev., 2015, 115, 6112–6216 CrossRef CAS PubMed.
- M. Z. Zhu, C. M. Aikens, F. J. Hollander, G. C. Schatz and R. C. Jin, Correlating the Crystal Structure of A Thiol-Protected Au25 Cluster and Optical Properties, J. Am. Chem. Soc., 2008, 130, 5883–5885 CrossRef CAS PubMed.
- S. W. Chen, R. S. Ingram, M. J. Hostetler, J. J. Pietron, R. W. Murray, T. G. Schaaff, J. T. Khoury, M. M. Alvarez and R. L. Whetten, Gold Nanoelectrodes of Varied Size: Transition to Molecule-Like Charging, Science, 1998, 280, 2098–2101 CrossRef CAS PubMed.
- S. Knoppe and T. Bürgi, Chirality in Thiolate-Protected Gold Clusters, Acc. Chem. Res., 2014, 47, 1318–1326 CrossRef CAS PubMed.
- L. Shang, S. Dong and G. U. Nienhaus, Ultra-small fluorescent metal nanoclusters: Synthesis and biological applications, Nano Today, 2011, 6, 401–418 CrossRef CAS.
- N. Goswami, Q. F. Yao, Z. T. Luo, J. G. Li, T. K. Chen and J. P. Xie, Luminescent Metal Nanoclusters with Aggregation-Induced Emission, J. Phys. Chem. Lett., 2016, 7(6), 962–975 CrossRef CAS PubMed.
- G. Li and R. C. Jin, Atomically Precise Gold Nanoclusters as New Model Catalysts, Acc. Chem. Res., 2013, 46, 1749–1758 CrossRef CAS PubMed.
- W. Kurashige, Y. Niihori, S. Sharma and Y. Negishi, Precise synthesis, functionalization and application of thiolate-protected gold clusters, Coord. Chem. Rev., 2016, 320–321, 238–250 CrossRef CAS.
- Y. Liu, X. L. Li, H. Liu, Z. J. Zhou, J. P. Huang, S. L. Lei, S. H. Cai, Z. Chen, Y. L. Guo, Z. W. Chen, X. Zhou and L. M. Nie, Porous gold nanocluster-decorated manganese monoxide nanocomposites for microenvironment-activatable MR/photoacoustic/CT tumor imaging, Nanoscale, 2018, 10, 3631–3638 RSC.
- G. Hughes and J. C. Lewis, Introduction: Biocatalysis in Industry, Chem. Rev., 2018, 118, 1–3 CrossRef CAS PubMed.
- R. H. Terrill, T. A. Postlethwaite, C. Chen, C. D. Poon, A. Terzis, A. Chen, J. E. Hutchison, M. R. Clark, G. Wignall, J. D. Londono, R. Superfine, M. Falvo, C. S. Johnson Jr., E. T. Samulski and R. W. Murray, Monolayers in Three Dimensions: NMR, SAXS, Thermal, and Electron Hopping Studies of Alkanethiol Stabilized Gold Clusters, J. Am. Chem. Soc., 1995, 117, 12537–12548 CrossRef CAS.
- J. P. Xie, Y. G. Zheng and J. Y. Ying, Protein-Directed Synthesis of Highly Fluorescent Gold Nanoclusters, J. Am. Chem. Soc., 2009, 131, 888–889 CrossRef CAS PubMed.
- M. S. Devadast, J. Kim, E. Sinn, D. Lee, T. Goodson and G. Ramakrishna, Unique Ultrafast Visible Luminescence in Monolayer-Protected Au25 Clusters, J. Phys. Chem. C, 2010, 114, 22417–22423 CrossRef.
- J. Sun and Y. D. Jin, Fluorescent Au nanoclusters: recent progress and sensing applications, J. Mater. Chem. C, 2014, 2, 8000–8011 RSC.
- Y. K. Zheng, W. W. Liu, Y. Chen, H. Jiang and X. M. Wang, Mercaptopyrimidine-directed gold nanoclusters: a suitable fluorescent probe for intracellular glutathione imaging and selective cancer cell identification, J. Mater. Chem. B, 2018, 6, 3650–3654 RSC.
- X. Wen, P. Yu, Y. R. Toh and J. Tang, Structure-correlated dual fluorescent bands in BSA-protected Au25 nanoclusters, J. Phys. Chem. C, 2012, 116, 11830–11836 CrossRef CAS.
- K. R. Krishnadas, A. Ghosh, A. Baksi, I. Chakraborty, G. Natarajan and T. Pradeep, Intercluster Reactions between Au25(SR)18 and Ag44(SR)30, J. Am. Chem. Soc., 2016, 138, 140–148 CrossRef CAS PubMed.
- Q. Li, A. Das, S. X. Wang, Y. X. Chen and R. C. Jin, Highly efficient three-component coupling reaction catalysed by atomically precise ligand-protected Au38(SC2H4Ph)24 nanoclusters, Chem. Commun., 2016, 52, 14298–14301 RSC.
- Y. Tao, Y. H. Lin, Z. Z. Huang, J. S. Ren and X. G. Qu, Incorporating Graphene Oxide and Gold Nanoclusters: A Synergistic Catalyst with Surprisingly High Peroxidase-Like Activity Over a Broad pH Range and its Application for Cancer Cell Detection, Adv. Mater., 2013, 25, 2594–2599 CrossRef CAS PubMed.
- D. H. Hu, Z. H. Sheng, S. T. Fang, Y. N. Wang, D. Y. Gao, P. F. Zhang, P. Gong, Y. F. Ma and L. T. Cai, Folate Receptor-Targeting Gold Nanoclusters as Fluorescence Enzyme Mimetic Nanoprobes for Tumor Molecular Colocalization Diagnosis, Theranostics, 2014, 4, 142–153 CrossRef CAS PubMed.
- H. D. Cui, D. H. Hu, J. N. Zhang, G. H. Gao, Z. Chen, W. J. Li, P. Gong, Z. H. Sheng and L. T. Cai, Gold Nanoclusters-Indocyanine Green Nanoprobes for Synchronous Cancer Imaging, Treatment, and Real-Time Monitoring Based on Fluorescence Resonance Energy Transfer, ACS Appl. Mater. Interfaces, 2017, 9, 25114–25127 CrossRef CAS PubMed.
- X. Yu, C. X. Zhang, L. N. Zhang, Y. R. Xue, H. W. Li and Y. Q. Wu, The construction of a FRET assembly by using gold nanoclusters and carbon dots and their application as a ratiometric probe for cysteine detection, Sens. Actuators, B, 2018, 263, 327–335 CrossRef CAS.
- J. J. Yang, F. L. Wang, H. Q. Yuan, L. S. Zhang, Y. Y. Jiang, X. Zhang, C. Liu, L. Chai, H. Li and M. Stenzel, Recent advances in ultra-small fluorescent Au nanoclusters toward oncological research, Nanoscale, 2019, 11, 17967–17980 RSC.
- Q. Tang, G. X. Hu, V. Fung and D. E. Jiang, Insights into Interfaces, Stability, Electronic Properties, and Catalytic Activities of Atomically Precise Metal Nanoclusters from First Principles, Acc. Chem. Res., 2018, 51, 2793–2802 CrossRef CAS PubMed.
- J. Z. Yan, B. K. Teo and N. F. Zheng, Surface Chemistry of Atomically Precise Coinage–Metal Nanoclusters: From Structural Control to Surface Reactivity and Catalysis, Acc. Chem. Res., 2018, 51, 3084–3093 CrossRef CAS PubMed.
- Y. Wang, X. K. Wan, L. T. Ren, H. F. Su, G. Li, S. Malola, S. C. Lin, Z. C. Tang, H. Häkkinen, B. K. Teo, Q. M. Wang and N. F. Zheng, Atomically Precise Alkynyl-Protected Metal Nanoclusters as a Model Catalyst: Observation of Promoting Effect of Surface Ligands on Catalysis by Metal Nanoparticles, J. Am. Chem. Soc., 2016, 138, 3278–3281 CrossRef CAS PubMed.
- X. K. Wan, J. Q. Wang, Z. A. Nan and Q. M. Wang, Ligand effects in catalysis by atomically precise gold nanoclusters, Sci. Adv., 2017, 3, e1701823 CrossRef PubMed.
- Z. M. Li, C. Liu, H. Abroshan, D. R. Kauffman and G. Li, Au38S2(SAdm)20 Photocatalyst for One-Step Selective Aerobic Oxidations, ACS Catal., 2017, 7, 3368–3374 CrossRef CAS.
- X. F. Cui, J. Wang, B. Liu, S. Ling, R. Long and Y. J. Xiong, Turning Au Nanoclusters Catalytically Active for Visible-Light-Driven CO2 Reduction through Bridging Ligands, J. Am. Chem. Soc., 2018, 140, 16514–16520 CrossRef CAS PubMed.
- M. R. Narouz, K. M. Osten, P. J. Unsworth, R. W. Y. Man, K. Salorinne, S. Takano, R. Tomihara, S. Kaappa, S. Malola, C. T. Dinh, J. D. Padmos, K. Ayoo, P. J. Garrett, M. Nambo, J. H. Horton, E. H. Sargent, H. Häkkinen, T. Tsukuda and C. M. Crudden, N-heterocyclic carbene-functionalized magic-number gold nanoclusters, Nat. Chem., 2019, 11, 419–425 CrossRef CAS.
- S. Zhao, N. Austin, M. Li, Y. B. Song, S. D. House, S. Bernhard, J. C. Yang, G. Mpourmpakis and R. C. Jin, Influence of Atomic-Level Morphology on Catalysis: The Case of Sphere and Rod-Like Gold Nanoclusters for CO2 Electroreduction, ACS Catal., 2018, 8, 4996–5001 CrossRef CAS.
- G. X. Zhao, H. M. Liu and J. H. Ye, Constructing and controlling of highly dispersed metallic sites for catalysis, Nano Today, 2018, 19, 108–125 CrossRef CAS.
- X. D. Zhang, D. Wu, X. Shen, P. X. Liu, F. Y. Fan and S. J. Fan,
In Vivo Renal Clearance, Biodistribution, Toxicity of Gold Nanoclusters, Biomaterials, 2012, 33, 4628–4638 CrossRef CAS PubMed.
- J. B. Liu, M. X. Yu, C. Zhou, S. Y. Yang, X. H. Ning and J. Zheng, Passive Tumor Targeting of Renal-Clearable Luminescent Gold Nanoparticles: Long Tumor Retention and Fast Normal Tissue Clearance, J. Am. Chem. Soc., 2013, 135(13), 4978–4981 CrossRef CAS PubMed.
- X. R. Song, N. Goswami, H. H. Yang and J. P. Xie, Functionalization of metal nanoclusters for biomedical applications, Analyst, 2016, 141, 3126–3140 RSC.
- Q. F. Yao, X. Yuan, T. K. Chen, D. T. Leong and J. P. Xie, Engineering Functional Metal Materials at the Atomic Level, Adv. Mater., 2018, 30, 1802751 CrossRef PubMed.
- A. A. Herzing, C. J. Kiely, A. F. Carley, P. Landon and G. J. Hutching, Identification of Active Gold Nanoclusters on Iron Oxide Supports for CO Oxidation, Science, 2008, 321, 1331–1335 CrossRef CAS PubMed.
- A. Mathew and T. Pradeep, Noble Metal Clusters: Applications in Energy, Environment, and Biology, Part. Part. Syst. Charact., 2014, 31, 1017–1053 CrossRef CAS.
- Z. K. Wu, M. A. MacDonald, J. Chen, P. Zhang and R. C. Jin, Kinetic Control and Thermodynamic Selection in the Synthesis of Atomically Precise Gold Nanoclusters, J. Am. Chem. Soc., 2011, 133, 9670–9673 CrossRef CAS PubMed.
- H. Zhang, X. Huang, L. Li, G. W. Zhang, I. Hussain, Z. Li and B. Tan, Photoreductive synthesis of water-soluble fluorescent metal nanoclusters, Chem. Commun., 2012, 48, 567–569 RSC.
- H. Y. Liu, X. Zhang, X. M. Wu, L. P. Jiang, C. Burda and J. J. Zhu, Rapid sonochemical synthesis of highly luminescent non-toxic Au NCs and Au@ Ag NCs and Cu (II) sensing, Chem. Commun., 2011, 47, 4237–4239 RSC.
- Y. Yue, T. Y. Liu, H. W. Li, Z. Y. Liu and Y. Q. Wu, Microwave-assisted synthesis of BSA-protected small gold nanoclusters and their fluorescence-enhanced sensing of silver(I) ions, Nanoscale, 2012, 4, 2251–2254 RSC.
- W. W. Guo, J. P. Yuan and E. K. Wang, Organic-soluble fluorescent Au8 clusters generated from heterophase ligand-exchange induced etching of gold nanoparticles and their electrochemiluminescence, Chem. Commun., 2012, 48, 3076–3078 RSC.
- M. L. Cui, Y. Zhao and Q. J. Song, Synthesis, optical properties and applications of ultra-small luminescent gold nanoclusters, Trends Anal. Chem., 2014, 57, 73–82 CrossRef CAS.
- N. Goswami, Q. F. Yao, T. K. Chen and J. P. Xie, Mechanistic exploration and controlled synthesis of precise thiolate-gold nanoclusters, Coord. Chem. Rev., 2016, 329, 1–15 CrossRef CAS.
- N. K. Chaki, Y. Negishi, H. Tsunoyama, Y. Shichibu and T. Tsukuda, Ubiquitous 8 and 29 kDa Gold: Alkanethiolate Cluster Compounds: Mass-Spectrometric Determination of Molecular Formulas and Structural Implications, J. Am. Chem. Soc., 2008, 130, 8608–8610 CrossRef CAS PubMed.
- C. A. Fields-Zinna, J. S. Sampson, M. C. Crowe, J. B. Tracy, J. F. Parker, A. M. deNey, D. C. Muddiman and R. W. Murray, Tandem Mass Spectrometry of Thiolate-Protected Au Nanoparticles NaxAu25(SC2H4Ph)18−y(S(C2H4O)5CH3)y, J. Am. Chem. Soc., 2009, 131, 13844–13851 CrossRef CAS PubMed.
- A. Ghosh, T. Udayabhaskararao and T. Pradeep, One-Step Route to Luminescent Au18SG14 in the Condensed Phase and Its Closed Shell Molecular Ions in the Gas Phase, J. Phys. Chem. Lett., 2012, 3, 1997–2002 CrossRef CAS.
- Y. Negishi, Y. Takasugi, S. Sato, H. Yao, K. Kimura and T. Tsukuda, Magic-Numbered Aun Clusters Protected by Glutathione Monolayers (n = 18, 21, 25, 28, 32, 39):
Isolation and Spectroscopic Characterization, J. Am. Chem. Soc., 2004, 126, 6518–6519 CrossRef CAS PubMed.
- E. Porret, L. Sancey, A. Martín-Serrano, M. I. Montañez, R. Seeman, A. Yahia-Ammar, H. Okuno, F. Gomez, A. Ariza, N. Khildebrandt, J. B. Fleury, J. L. Coll and X. Le Guével, Hydrophobicity of Gold Nanoclusters Influences Their Interactions with Biological Barriers, Chem. Mater., 2017, 29, 7497–7506 CrossRef CAS.
- A. V. Shytov and P. B. Allen, Electronic polarity of nanoclusters: Quantum and many-body effects, Phys. Rev. B: Condens. Matter Mater. Phys., 2006, 74, 075419 CrossRef.
- M. Z. Zhu, H. F. Qian, X. M. Meng, S. S. Jin, Z. K. Wu and R. C. Jin, Chiral Au25 Nanospheres and Nanorods: Synthesis and Insight into the Origin of Chirality, Nano Lett., 2011, 11, 3963–3969 CrossRef CAS PubMed.
- K. Pyo, V. D. Thanthirige, S. Y. Yoon, G. Ramakrishna and D. Lee, Enhanced luminescence of Au22(SG)18 nanoclusters via rational surface engineering, Nanoscale, 2016, 8, 20008–20016 RSC.
- I. Guryanov, F. Polo, E. V. Ubyvovk, E. Korzhikov-Vlakh, T. Tennikova, A. T. Rad, M. P. Nieh and F. Maran, Polylysine-grafted Au144 nanoclusters: birth and growth of a healthy surface-plasmon-resonance-like band, Chem. Sci., 2017, 8, 3228–3238 RSC.
- A. Dass, P. R. Nimmala, V. R. Jupally and N. Kothalawala, Au103(SR)45, Au104(SR)45, Au104(SR)46 and Au105(SR)46 nanoclusters, Nanoscale, 2013, 5, 12082–12085 RSC.
- S. Fedrigo, W. Harbich and J. Buttet, Optical Response of Ag2, Ag3, Au2, and Au3 in Argon Matrices, J. Chem. Phys., 1993, 99, 5712–5717 CrossRef CAS.
- B. A. Collings, K. Athanassenas, D. Lacombe, D. M. Rayner and P. A. Hackett, Optical absorption spectra of Au7, Au9, Au11, and Au13, and their cations: gold clusters with 6, 7, 8, 9, 10, 11, 12, and 13 s-electrons, J. Chem. Phys., 1994, 101, 3506–3513 CrossRef CAS.
- J. Zheng, C. W. Zhang and R. M. Dickson, Highly fluorescent, water-soluble, size-tunable gold quantum dots, Phys. Rev. Lett., 2004, 93, 077402 CrossRef PubMed.
- J. Zheng, C. Zhou, M. Yu and J. Liu, Different Sized Luminescent Gold Nanoparticles, Nanoscale, 2012, 4, 4073–4083 RSC.
- S. Wang, X. Meng, A. Das, T. Li, Y. Song, T. Cao, X. Zhu, M. Zhu and R. Jin, A 200-fold Quantum Yield Boost in the Photoluminescence of Silver-Doped AgxAu25−x Nanoclusters: The 13 th Silver Atom Matters, Angew. Chem., Int. Ed., 2014, 53, 2376–2380 CrossRef CAS PubMed.
- C. M. Andolina, A. C. Dewar, A. M. Smith, L. E. Marbella, M. J. Hartmann and J. E. Millstone, Photoluminescent Gold–Copper Nanoparticle Alloys with Composition-Tunable Near-Infrared Emission, J. Am. Chem. Soc., 2013, 135, 5266–5269 CrossRef CAS PubMed.
- Y. X. Chen, C. Liu, Q. Tang, C. J. Zeng, T. Higaki, A. Das, D. E. Jiang, N. L. Rosi and R. C. Jin, Isomerism in Au28(SR)20 Nanocluster and Stable Structures, J. Am. Chem. Soc., 2016, 138, 1482–1485 CrossRef CAS PubMed.
- S. Link, A. Beeby, S. FitzGerald, M. A. El-Sayed, T. G. Schaaff and R. L. Whetten, Visible to Infrared Luminescence from a 28-Atom Gold Cluster, J. Phys. Chem. B, 2002, 106, 3410–3415 CrossRef CAS.
- S. A. Miller, J. M. Womick, J. F. Parker, R. W. Murray and A. M. Moran, Femtosecond Relaxation Dynamics of Au25L18− Monolayer-Protected Clusters, J. Phys. Chem. C, 2009, 113, 9440–9444 CrossRef CAS.
- Z. Wu and R. Jin, On the Ligand's Role in the Fluorescence of Gold Nanoclusters, Nano Lett., 2010, 10, 2568–2573 CrossRef CAS PubMed.
- J. Liu, H. W. Li, W. X. Wang and Y. Q. Wu, Thermally prepared ultrabright adenosine monophosphate capped gold nanoclusters and the intrinsic mechanism, J. Mater. Chem. B, 2017, 5, 3550–3556 RSC.
- G. L. Wang, L. Y. Jin and Y. M. Dong, Intrinsic enzyme mimicking activity of gold nanoclusters upon visible light triggering and its application for colorimetric trypsin detection, Biosens. Bioelectron., 2015, 64, 523–529 CrossRef CAS PubMed.
- C. X. Chen, D. Zhao, Y. Y. Jiang, P. J. Ni, C. H. Zhang, B. Wang, F. Yang, Y. Z. Lu and J. Sun, Logically Regulating Peroxidase-Like Activity of Gold Nanoclusters for Sensing Phosphate-Containing Metabolites and Alkaline Phosphatase Activity, Anal. Chem., 2019, 91, 15017–15024 CrossRef CAS PubMed.
- S. Chakraborty, S. Babanova, R. C. Rocha, A. Desireddy, K. Artyushkova, A. E. Boncella, P. Atanassov and J. S. Martinez, A Hybrid DNA-Templated Gold Nanocluster For Enhanced Enzymatic Reduction of Oxygen, J. Am. Chem. Soc., 2015, 137, 11678–11687 CrossRef CAS PubMed.
- D. J. Mikolajczak, A. A. Berger and B. Koksch, Catalytically Active Peptide–Gold Nanoparticle Conjugates: Prospecting for Artificial Enzymes, Angew. Chem., Int. Ed., 2020, 59, 8776–8785 CrossRef CAS PubMed.
- W. Y. Chen, H. Y. Chang, J. K. Lu, Y. C. Huang, S. G. Harroun, Y. T. Tseng, Y. J. Li, C. C. Huang and H. T. Chang, Self-Assembly of Antimicrobial Peptides on Gold Nanodots: Against Multidrug-Resistant Bacteria and Wound-Healing Application, Adv. Funct. Mater., 2015, 25, 7189–7199 CrossRef CAS.
- K. Pyo, N. H. Ly, S. Y. Yoon, Y. M. Shen, S. Y. Choi, S. Y. Lee, S. W. Joo and D. Lee, Highly Luminescent Folate-Functionalized Au22 Nanoclusters for Bioimaging, Adv. Healthcare Mater., 2017, 6, 1700203 CrossRef PubMed.
- Z. M. Liu, L. Turyanska, F. Zamberlan, S. Pacifico, T. D. Bradshaw, F. Moro, M. W. Fay, H. E. L. Williams and N. R. Thomas, Synthesis of folic acid functionalized gold nanoclusters for targeting folate receptor-positive cells, Nanotechnology, 2019, 30, 505102 CAS.
- H. Y. Chen, B. W. Li, X. Y. Ren, S. N. Li, Y. X. Ma, S. S. Cui and Y. Q. Gu, Multifunctional near-infrared-emitting nano-conjugates based on gold clusters for tumor imaging and therapy, Biomaterials, 2012, 33, 8461–8476 CrossRef CAS PubMed.
- M. J. Wang, C. Y. Sun, L. Y. Wang, X. H. Ji, Y. B. Bai, T. J. Li and J. H. Li, Electrochemical Detection of DNA Immobilized on Gold Colloid Particles Modified Self-assembled Monolayer Electrode with Silver Nanoparticle Label, J. Pharm. Biomed. Anal., 2003, 33, 1117–1125 CrossRef CAS PubMed.
- Y. Negishi, K. Nobusada and T. Tsukuda, Glutathione-Protected Gold Clusters Revisited:
Bridging the Gap between Gold(I)–Thiolate Complexes and Thiolate-Protected Gold Nanocrystals, J. Am. Chem. Soc., 2005, 127, 5261–5270 CrossRef CAS PubMed.
- J. Nishigaki, R. Tsunoyama, H. Tsunoyama, N. Ichikuni, S. Yamazoe, Y. Negishi, M. Ito, T. Matsuo, K. Tamao and T. Tsukuda, A New Binding Motif of Sterically Demanding Thiolates on a Gold Cluster, J. Am. Chem. Soc., 2012, 134, 14295–14297 CrossRef CAS PubMed.
- L. C. McKenzie, T. O. Zaikova and J. E. Hutchison, Structurally Similar Triphenylphosphine-Stabilized Undecagolds, Au11(PPh3)7Cl3 and [Au11(PPh3)8Cl2]Cl, Exhibit Distinct Ligand Exchange Pathways with Glutathione, J. Am. Chem. Soc., 2014, 136, 13426–13435 CrossRef CAS PubMed.
- X. K. Wan, Q. Tang, S. F. Yuan, D. Jiang and Q. M. Wang, Au19 Nanocluster Featuring a V-Shaped Alkynyl–Gold Motif, J. Am. Chem. Soc., 2015, 137, 652–655 CrossRef CAS PubMed.
- Y. Y. Zhao, Z. L. Chen, Y. F. Chen, J. Xu, J. H. Li and X. Y. Jiang, Synergy of nonantibiotic drugs and pyrimidinethiol on gold nanoparticles against superbugs, J. Am. Chem. Soc., 2013, 135, 12940–12943 CrossRef CAS PubMed.
- N. Q. Gong, X. W. Ma, X. X. Ye, Q. F. Zhou, X. L. Tan, S. K. Yao, S. D. Huo, T. B. Zhang, S. Z. Chen, X. C. Teng, X. X. Hu, J. Yu, Y. L. Gan, H. D. Jiang, J. H. Li and X. J. Liang, Carbon dot-supported atomically-dispersed gold as a mitochondrial oxidative stress amplifier for cancer treatment, Nat. Nanotechnol., 2019, 14, 379–387 CrossRef CAS PubMed.
- J. Zheng, J. T. Petty and R. M. Dickson, High Quantum Yield Blue Emission from Water-Soluble Au8Nanodots, J. Am. Chem. Soc., 2003, 125, 7780–7781 CrossRef CAS PubMed.
- D. Y. An, J. G. Su, J. K. Weber, X. Y. Gao, R. H. Zhou and J. Y. Li, A Peptide-Coated Gold Nanocluster Exhibits Unique Behavior in Protein Activity Inhibition, J. Am. Chem. Soc., 2015, 137, 8412–8418 CrossRef CAS PubMed.
- Z. P. Wang, J. Q. Hu, Y. Jin, X. Yao and J. H. Li, In Situ Amplified Chemiluminescent Detection of DNA and Immunoassay of IgG Using Special-Shaped Gold Nanoparticles as Label, Clin. Chem., 2006, 52, 1958–1961 CrossRef CAS PubMed.
- O. Varnavski, G. Ramakrishna, J. Kim, D. Lee and T. Goodson, Critical Size for the Observation of Quantum Confinement in Optically Excited Gold Clusters, J. Am. Chem. Soc., 2010, 132, 16–17 CrossRef CAS PubMed.
- D. Hu, Z. H. Sheng, P. Gong, P. F. Zhang and L. T. Cai, Highly selective fluorescent sensors for Hg2+ based on bovine serum albumin-capped gold nanoclusters, Analyst, 2010, 135, 1411–1416 RSC.
- H. Wei, Z. D. Wang, L. M. Yang, S. L. Tian, C. J. Hou and Y. Lu, Lysozyme-stabilized gold fluorescent cluster: Synthesis and application as Hg2+ sensor, Analyst, 2010, 135, 1406–1410 RSC.
- X. L. Guével, N. Daum and M. Schneider, Synthesis and characterization of human transferrin-stabilized gold nanoclusters, Nanotechnology, 2011, 22, 275103 CrossRef PubMed.
- P. L. Xavier, K. Chaudhari, P. K. Verma, S. K. Pal and T. Pradeep, Luminescent quantum clusters of gold in transferrin family protein, lactoferrin exhibiting FRET, Nanoscale, 2010, 2, 2769–2776 RSC.
- H. Kawasaki, K. Yoshimura, K. Hamaguchi and R. Arakawa, Trypsin-stabilized fluorescent gold nanocluster for sensitive and selective Hg2+ detection, Anal. Sci., 2011, 27, 591–596 CrossRef CAS PubMed.
- F. Wen, Y. H. Dong, L. Feng, S. Wang, S. C. Zhang and X. R. Zhang, Horseradish peroxidase functionalized fluorescent gold nanoclusters for hydrogen peroxide sensing, Anal. Chem., 2011, 83, 1193–1196 CrossRef CAS PubMed.
- J. J. Luo, A. Rasooly, L. Q. Wang, K. Zeng, C. C. Shen, P. Qian, M. H. Yang and F. Qu, Fluorescent turn-on determination of the activity of peptidases using peptide templated gold nanoclusters, Microchim. Acta, 2016, 183, 605–610 CrossRef CAS.
- N. Q. Gong, X. C. Teng, J. H. Li and X. J. Liang, Antisense Oligonucleotide-Conjugated Nanostructure-Targeting lncRNA MALAT1 Inhibits Cancer Metastasis, ACS Appl. Mater. Interfaces, 2019, 11, 37–42 CrossRef CAS PubMed.
- T. A. C. Kennedy, J. L. MacLean and J. W. Liu, Blue emitting gold nanoclusters templated by poly-cytosine DNA at low pH and poly-adenine DNA at neutral pH, Chem. Commun., 2012, 48, 6845–6847 RSC.
- R. C. Triulzi, M. Micic, S. Giordani, M. Serry, W. A. Chiou and R. M. Leblanc, Immunoasssay based on the Antibody-conjugated PAMAM-dendrimer-gold Quantum dot Complex, Chem. Commun., 2006, 5068–5070 RSC.
- G. H. Yang, J. J. Shi, S. Wang, W. W. Xiong, L. P. Jiang, C. Burda and J. J. Zhu, Fabrication of a Boron Nitride-gold Nanocluster Composite and its Versatile Application for Immunoassays, Chem. Commun., 2013, 49, 10757–10759 RSC.
- Y. Zhang, C. Y. Zhang, C. Xu, X. L. Wang, C. Liu, G. I. N. Waterhouse, Y. L. Wang and H. Z. Yin, Ultrasmall Au nanoclusters for biomedical and biosensing applications: A mini-review, Talanta, 2019, 200, 432–442 CrossRef CAS PubMed.
- T. P. Silverstein, Marcus Theory: Thermodynamics Can Control the Kinetics of Electron Transfer Reactions, J. Chem. Educ., 2012, 89, 1159–1167 CrossRef CAS.
- J. L. Brennan, M. R. Branham, J. F. Hicks, A. J. Osisek, R. L. Donkers, D. G. Georganopoulou and R. W. Murray, Electron Hopping Dynamics in Monolayer-Protected Au Cluster Network Polymer Films by Rotated Disk Electrode Voltammetry, Anal. Chem., 2004, 76, 5611–5619 CrossRef CAS PubMed.
- J. Kim and D. Lee, Electron Hopping Dynamics in Au38 Nanoparticle Langmuir Monolayers at the Air/Water Interface, J. Am. Chem. Soc., 2006, 128, 4518–4519 CrossRef CAS PubMed.
- J. A. Scholl, A. García-Etxarri, A. L. Koh and J. A. Dionne, Observation of Quantum Tunneling between Two Plasmonic Nanoparticles, Nano Lett., 2013, 13, 564–569 CrossRef CAS PubMed.
- A. Yu, S. W. Li, G. Czap and W. Ho, Tunneling-Electron-Induced Light Emission from Single Gold Nanoclusters, Nano Lett., 2016, 16, 5433–5436 CrossRef CAS PubMed.
- H. J. Xiang, W. Feng and Y. Chen, Single-Atom Catalysts in Catalytic Biomedicine, Adv. Mater., 2020, 1905994 CrossRef CAS PubMed.
|
This journal is © The Royal Society of Chemistry 2020 |
Click here to see how this site uses Cookies. View our privacy policy here.