DOI:
10.1039/D3BM01338C
(Review Article)
Biomater. Sci., 2024,
12, 2521-2560
The unexplored role of alkali and alkaline earth elements (ALAEs) on the structure, processing, and biological effects of bioactive glasses
Received
16th August 2023
, Accepted 15th February 2024
First published on 26th March 2024
Abstract
Bioactive glass has been employed in several medical applications since its inception in 1969. The compositions of these materials have been investigated extensively with emphasis on glass network formers, therapeutic transition metals, and glass network modifiers. Through these experiments, several commercial and experimental compositions have been developed with varying chemical durability, induced physiological responses, and hydroxyapatite forming abilities. In many of these studies, the concentrations of each alkali and alkaline earth element have been altered to monitor changes in structure and biological response. This review aims to discuss the impact of each alkali and alkaline earth element on the structure, processing, and biological effects of bioactive glass. We explore critical questions regarding these elements from both a glass science and biological perspective. Should elements with little biological impact be included? Are alkali free bioactive glasses more promising for greater biological responses? Does this mixed alkali effect show increased degradation rates and should it be employed for optimized dissolution? Each of these questions along with others are evaluated comprehensively and discussed in the final section where guidance for compositional design is provided.
1. Introduction
Glass-based materials have been developed for medical applications since the discovery of their bone bonding capabilities by Larry Hench in 1969.1 Bioactive glasses (BGs) have then been expanded to several applications in medicine outside of hard tissue. These new applications required a new definition of BGs to be proposed: “a non-equilibrium, non-crystalline material that has been designed to induce specific biological activity”.2
BGs have been developed from network formers such as SiO2, B2O3, and P2O5 and have been modified with nearly every biocompatible element on the periodic table. Varying chemical compositions of BGs has been the best method to tailor bioactivity, dissolution rates, and other properties to the desired application. 45S5 Bioglass®, the original parent composition, was designed as belonging to the quaternary 24.5Na2O–24.5CaO–45SiO2–6P2O5 (wt%) oxide system. Invented by Larry Hench, 45S5 Bioglass® has been employed as the most used composition so far and has been implemented in several commercially available medical devices mainly addressed to bone repair. Other glass systems such as 13-93B3, a borate-based composition of 5.5Na2O–11.2K2O–4.6MgO–18.5CaO–56.5B2O3–3.7P2O5 (wt%), have been shown effective in soft tissue repair with accelerated dissolution rates.3,4 Several other compositions have been developed modifying biological capabilities with transition metals and using glass science phenomena such as the mixed alkali effect and mixed former effect to tailor properties.5–7
In order to produce a wide array of BG devices, several processing methods have been developed. Melting route and sol–gel process are currently the only methods used to create BGs but there are many ways to process the “raw” glass materials obtained thereof. Thermal processing of BGs can aid in terms of the mechanical properties of final products and provide better control of the dissolution rate.8 Crystallization of a secondary biocompatible phase to create a glass-ceramic material or sintering of BG powder are both common thermal processing techniques.9–14 Additive manufacturing techniques to fabricate porous scaffolds as well as advanced deposition methods to create thin films have also been studied to further add to the diverse potential of BG medical devices.15–18
Ions released from BGs have been shown to play several roles in increasing glass bioactivity and potential in tissue engineering. The first role is promoting the precipitation of bone-like mineral phases such as hydroxyapatite and fluorapatite. The bone bonding ability of BGs relies on the ability to slowly dissolve and induce the formation of nanocrystalline apatite at the bone/implant interface.19 Regeneration of hard tissues is further stimulated through BG-driven genetic expression and stimulation of osteogenic markers and control of cell cycle regulators via the release of key therapeutic ions.20 Soft tissue growth is also stimulated through genetic expression with proteins such as vascularization epithelial growth factor (VEGF) and different forms of matrix metalloproteinase (MMO).21 The angiogenic nature of some BGs also helps reform broken blood vessels and promote neo-vascularization, further accelerating the soft tissue repair timeline.22,23 Several other studies have shown that including other elements in the BG matrix can aid in antibacterial effects, anti-inflammatory stimulation, antioxidant and other beneficial biological processes.24–26Fig. 1 provides a visualization of the periodic table highlighting the role of each element in bioactive glasses.2
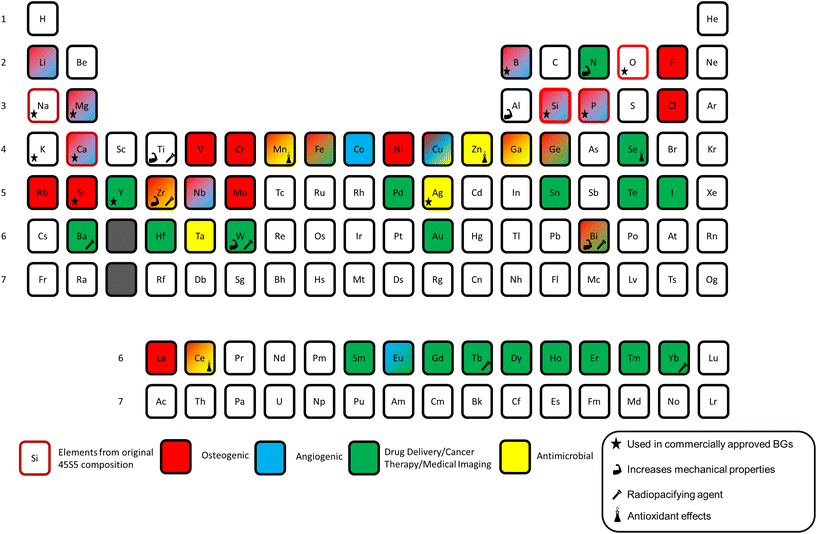 |
| Fig. 1 Periodic table of elements used in bioactive glasses with different therapeutic applications highlighted. Modified and reproduced from ref. 2 with permission from Elsevier, 2023. | |
Applications of BGs have dramatically grown over the last 15 years to include all aspects of medicine including both hard tissue and soft tissue regeneration, drug delivery, and cancer therapy.27,28 The ability to customize the composition and tailor the processing to target the specific needs of a medical device suits BGs well for most applications in tissue engineering.29 Increasing evidence is reported each year displaying the unique ability of BG-based devices to deliver key therapeutic ions while providing a bioresorbable platform for tissue engineering or as a drug delivery vehicle. The regeneration of hard tissue through BG-based devices has been shown effective in many forms such as glass monoliths, cements, putties, and 3D-printed scaffolds.30–35 Soft tissue applications have implemented “cotton candy-like” glass fibers, putties, and dozens of synthetic and natural polymer–matrix composites.36–40 Mesoporous BGs produced through sol–gel synthesis have provided a high surface area platform for high-capacity drug delivery.41–43 The applications of BGs are continually expanding as the level of interest in the field has grown exponentially as illustrated in Fig. 2.
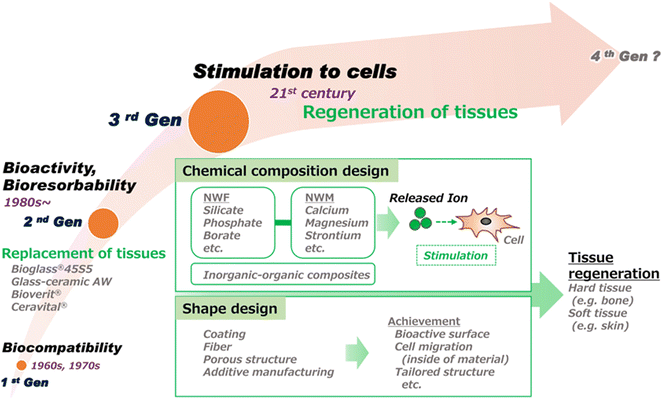 |
| Fig. 2 Progress in the development of bioactive glasses for clinical applications. This illustration highlights the advancement of research in the field from the discovery of BG biocompatibility to specific biological action due to release of inorganic ions.44 | |
Decades of research have explored the impact of bioactive glasses through in vitro and in vivo studies focusing on the glass structure, processing, and release of inorganic ions. The objective of this review is to guide the design of BG compositions by focusing on the different effects of ALAEs. Key properties, such as dissolution behavior, phase precipitation from solution, and mixed alkali effects, are briefly introduced in relation to their influence on glass structure. Additionally, the review emphasizes the influence of ALAEs on processing methods and related properties of bioactive glasses, providing valuable insights into how these ions affect the manufacturing and characteristics of these materials. This review places a primary focus on exploring the physiological consequences of leached alkali and alkaline earth ions, shedding light on their role in the human body and potential implications for medical applications. Understanding these effects is vital for the development and optimization of bioactive glasses for various biomedical purposes.
2. Role of ALAEs on structure and properties
The structure of bioactive glasses is critical to control its properties and processibility. Compositional design factors such as total network modifier content, elemental selection, and alkali to alkaline earth ratio, which primarily include Li2O, Na2O, and K2O, and MgO, CaO, SrO, and BaO, can affect structure and subsequently key properties such as apatite forming ability, dissolution kinetics, and cell biology. As depicted in Fig. 3, Na and Ca, as the primary representatives of ALAEs, have the capability to modify the glass network in silicate glass. This modification renders the glass suitable for a variety of applications in dental, bone/soft tissue engineering, and drug delivery. Researchers have diligently explored additional ALAEs to expand and provide further justification for the applications of BGs.
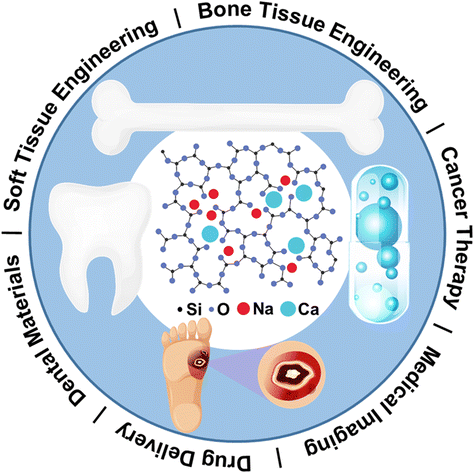 |
| Fig. 3 Sodium and calcium as primary representatives of ALAEs on glass network modification that render BGs for a variety of applications in dental, bone/soft tissue engineering, and drug delivery. From ref. 2 with permission from Elsevier, 2023. | |
2.1 Structure
Wallace et al.45 produced various melt-quenched bioactive glass formulations and investigated the influence of Na2O on the glass properties while keeping the glass network connectivity (NC) constant by systematically replacing CaO with Na2O. Linear decrease in the glass transition temperature and peak crystallization was detected as the content of Na2O increased. This was why the addition of Na2O caused glass network disruption because the glass network expanded with increasing Na2O content. Accordingly, decrement of glass transition temperature was observed with increasing Na2O content because this is an effect of glass network disruption.27 In fact, increasing the Na2O content in bioactive glasses as a replacement for CaO leads to the widening of the silicate network: in other words, the packing density decreases due to the substitution of one bivalent Ca2+ ion with two monovalent Na+ ions. As a macroscopic result, Farooq et al.46 showed that the glass density and hardness decrease because the glass network becomes less compact as Na2O increases.
Glass formation and stability are critical to understanding the durability and dissolution of a glass.47 In bioactive glass design, these are parameters of key interest because the primary mechanism for apatite forming ability and therapeutic ion release are through dissolution.48–50 The connectivity of a glass network and cross-linking density are used to understand solubility and surface reactivity of bioactive glasses.51 Furthermore, ALAEs with the same valency but different sizes can be substituted for each other resulting in the glass network to expand or compact.52 This has been shown to affect solubility and bioactivity of bioactive glass, both of which are important to increasing efficacy.52 The size of the cation can change the packing density of the glass which directly affects the dissolution behavior. An example of this would be replacing CaO with SrO will compact the silicate rings of the glass and slow dissolution.
Some ALAEs behave differently, such as MgO that acts as a network intermediate instead of a modifier in a highly disrupted silicate network.53 In bioactive glasses, a modifier to intermediate transition is expected to inhibit bioactivity because the dissolution and release of therapeutic ions is restricted compared to glasses which are less stable. However, for the case of MgO, it was observed that the likelihood for crystallization was also decreased when MgO acted as an intermediate.53 This would be a benefit for processing bioactive glasses. When new bioactive glasses are designed it is important to fully characterize the structure of the glass to ensure each component is acting as expected. The specific properties which are tailored by optimization of added ALAEs will be determined by the application and processing specifications.
2.2 Apatite forming ability
In vitro studies show BGs to form multiple phases including hydroxyapatite (HAp), hydroxy-carbonate apatite (HCA), amorphous calcium phosphate (ACP), fluorapatite, and chlorapatite.54,55 The phases that form are dependent on the glass composition, duration of exposure to a biologic environment, temperature, and pH. Understanding the factors which govern phase formation mechanisms and in vitro mineralization will guide the future of bioactive glass science. Apatite forming ability has been shown to depend on the level of polymerization of the glass network. Bioactive glasses with high network connectivity show decreased ion release rates which in turn slows apatite formation.56 A network connectivity of 2.4 has been established as the cut-off point for a BG to form apatite at its surface.51
Hydroxyapatite, Ca10(PO4)(OH)2, naturally requires free calcium and phosphate ions to be released at the surface of BGs. Both of these ions occur naturally in physiological fluids, the formation of HAp is highly compositional dependent.57 Although replacing calcium with other alkaline earth elements may not negatively affect dissolution rates, it can reduce the rate and amount of HAp formed.58,59 Magnesium has been well studied and described to delay the formation of an apatite layer.60,61 On the other hand, strontium has been found to assist in apatite formation through strontium-substituted apatite.62 Finally, alkali free BGs have also shown equal performance in apatite formation as alkali containing glasses. This suggests that free alkali elements in solution do not contribute to the formation of an apatite surface.63,64
2.3 Solubility
Solubility control of bioactive glasses is critical to improving bioactivity and performance. For bone formation, too much solubility can hinder the rate of HAp formation because the ions may be transported away from the healing site.65 In soft tissue healing applications, high solubility is important to ensuring no glass remains once the healing reaction is complete.66,67 Finding the right solubility rate for each application will help to optimize the effectiveness of bioactive glasses. Compositional dependence and processing dependence on the solubility of bioactive glasses is discussed further here.
Glass network disruption, through formation of non-bridging oxygens (NBOs), means higher reactivity of the glass in aqueous solution, for example, like biological fluids. As a matter of fact, the major added value of Na2O in melt-derived bioactive silicate glasses is related to surface reactivity and, hence, apatite-forming ability, which is key for bone-bonding. The bioactive role played by Na2O and other ALAEs in biomedical glasses is discussed elsewhere in this paper. As mentioned earlier here that although Na-containing bioactive glasses generally show good bioactivity and biocompatibility, high contents of Na2O can elicit a cytotoxic response.45 A similar disrupting effect on the glass network is associated to the introduction of MgO, leading to the creation of NBOs, decreasing the glass transition temperature and also – albeit indirectly – increasing the rate of bioactive glass dissolution,68 with an obvious impact on apatite-forming kinetics.
The solubility of glasses is directly tied to the structure of the glass which results from the manufacturing process and composition. A study on glasses containing SiO2, P2O5, CaO, and Na2O showed that the reactivity and bioactivity will decrease with increased density of the structure.69 The authors characterized the densification of glass by calculating the activation energy for silicon release in a series of glasses and found that 45S5 was very bioactive. Furthermore, an increase in silica and decrease in CaO and Na2O content respectively increased the activation energy, decreasing bioactivity. The sol–gel glasses in the study were found to be in the middle range of the melt-quench glasses studied.69 This indicates that the compositional effect on solubility is larger than the processing effect. However, it is not clear whether the observed decrease in solubility is primarily attributable to modifications in silicon, calcium, or sodium content. The mixed alkali effect (MAE), which means multiple alkali cations in the network can be observed to have non-linear property changes, should be further evaluated in these systems to understand the structure role.70 For example, Tylkowski and Brauer when substituted sodium ions for potassium or lithium ions in bioactive glass compositions observed that in compositions with a mixture of sodium and potassium, the crystallization temperature increased compared to 45S5. This means that the glass processing window was larger.71 Further evidence shows the MAE to decrease the probability of crystallization and promote glass stability.72 Therefore, the MAE should be considered for processing bioactive glasses containing more than one modifier.
When more than one alkali oxide is present in a glass composition and the MAE is observed, it is also common for structural changes to take place. For example, if sodium is replaced by lithium, a silicate network becomes more compact due to the variation in ionic radii between the interchanged ions.73 This is a good example of the structural characteristics of a glass network having direct influence on mechanical and thermal properties. Current bioactive glass literature focuses on sodium, lithium, and potassium.72–74 For application to bioactive glasses, it is important to tailor the combination of the alkali elements present to achieve optimal properties while limiting toxicity. Furthermore, understanding how the MAE may influence the solubility of the glass network is of current importance. By connecting the ion release rate and ion mobility to the MAE, a deeper understanding of diffusion in bioactive glass networks would emerge.75 If the solubility can be directly correlated to the mixed alkali effect, the MAE will be essential to understand the inclusion of multiple modifiers in bioactive glasses.
2.4 Alkali free bioactive glasses
Alkali free BGs have similar structures to alkali containing compositions as alkaline earth elements also act as network modifier. However, there are a variety of reasons researchers are exploring alkali free bioactive glasses. Improving cytotoxicity, decreasing the tendency for crystallization, and optimizing the dissolution rate are a few good examples.76–79 Limiting the alkali content can reduce cytotoxicity as elements like sodium rapidly increase the pH of the surrounding environment upon dissolution. Some glasses including alkali oxides have also been shown to absorb water through osmosis which limits their mechanical properties for certain applications like coatings on implants.76 These alkali free BGs have been studied primarily through sol–gel derived glasses as the compositional workspace is broadened through this method. The following sections of processing and physiology will discuss the potential advantages of alkali free BGs in greater detail. Alkali free BGs have delayed dissolution rates compared to alkali containing analogue compositions. Dissolution is driven by the ion exchange at the glass surface with hydroxyl groups causing the formation of silanol groups.80 Both static and dynamic dissolution studies show that alkali elements are the fastest to be released allowing for increased overall dissolution rates and formation of crystalline phases at the glass surface.81–83 Furthermore, the concentration and ratio of different alkaline earth elements in the glass matrix can further affect dissolution rate.84
3. Effect of ALAEs on processing and crystallization
3.1 Melt-derived glasses
While there is abundant literature on the biological effects elicited by ALAEs released from bioactive glasses, there is a much more limited amount of systematic studies specifically focusing on the role of these elements on sintering and processing. The reason is that processing of bioactive glasses per se is somewhat similar to that of common glasses with similar composition; hence, the consolidated knowledge of the latter group can be applied to the former.
3.1.1 Effects on viscosity.
In general, it is well known that modifiers – including ALAEs – disrupt the glass network and, therefore, are useful to allow glass production at lower temperatures because of their effect in decreasing the viscosity of the glass melt. In this regard, the viscosity-temperature relationship is key in determining the easiness of glass formation of any melt. Glass formation is promoted when crystallization is discouraged by the kinetic barrier to atomic arrangement, provided that (i) the viscosity is sufficiently high at the melting temperature of the crystalline phase that would form from the melt and/or (ii) the viscosity decreases very rapidly as temperature increases.85 Just to provide some quantifications, melting of silicate commercial glasses typically takes place at a viscosity below 10 Pa s and the viscosity at the working point (i.e., when a glass product is ready for being shaped) is about 103 Pa s. Modifiers generally tend to open up the 3D structural network of glass, thereby increasing the fluidity and decreasing the working temperature.85
3.1.2 Effects on thermal behavior, sintering and processing.
Bioactive glass products deriving from melts are typically obtained through three approaches: (i) direct casting of the melt into a mold (in this case a key role is played by the viscosity of the melt), (ii) fiber drawing (in this case a key role is played by glass transition temperature), and (iii) sintering of previously-prepared glass powders to fabricate, for example, porous scaffolds.
Therefore, glass stability against crystallization must be considered not only during the initial melting procedure (case (i)) or drawing (case (ii)) of the material, but also when glass-based products are obtained by applying secondary high-temperature treatments above Tg (case (iii)). It is well known that viscous flow sintering of glass particles is highly effective when the surface tension is high, the viscosity is low and crystallization does not take place.86,87 Lara et al.88 introduced a parameter, Sc, that quantifies the sinterability of glass, defined as:
where
Tx is the crystallization onset determined by DTA/DSC and
TMS is the temperature of maximum shrinkage determined from hot-stage microscopy (HSM) or dilatometer.
This parameter measures the competition between glass sintering and crystallization that may concurrently occur during heating (sinter-crystallization): the larger Sc, the more independent the kinetics of the two processes. A general rule can be proposed for the interpretation of Sc: if Sc < 0, only partial densification is achieved before crystallization begins (like in the case of 45S5 Bioglass®89); otherwise, if Sc ≥ 0, full densification occurs prior to crystallization (e.g. in bioactive glasses/glass-ceramics belonging to the CaO–MgO–SiO2–P2O5–Na2O–CaF2 system90). Therefore, higher values of Sc are related to higher final densities due to better sintering behavior and, ultimately, higher mechanical properties of the final product.
Another parameter describing glass stability is the difference between onset of crystallization and glass transition temperature (Tx − Tg). In this regard, the presence of MgO in the glass can produce a broadening of this range.91 In fact, it is known that resistance to crystallization is improved when CaO is partially substituted by MgO in SiO2–Na2O–CaO glasses.92 Verné et al.93 even revealed that replacement of CaO with MgO in a silicate SiO2–Na2O–K2O–MgO–CaO–P2O5 system, keeping all the other oxide amounts fixed, led to the inhibition of a crystalline phase.
The level of viscous flow during sintering is directly affected by the ALAEs content in the BGs. Nommeots-Nomm et al. studied the viscous flow sintering of BGs in a 3D-printed scaffold using synchrotron X-ray tomography to visualize the changes in inter-particle spacing (Fig. 4).
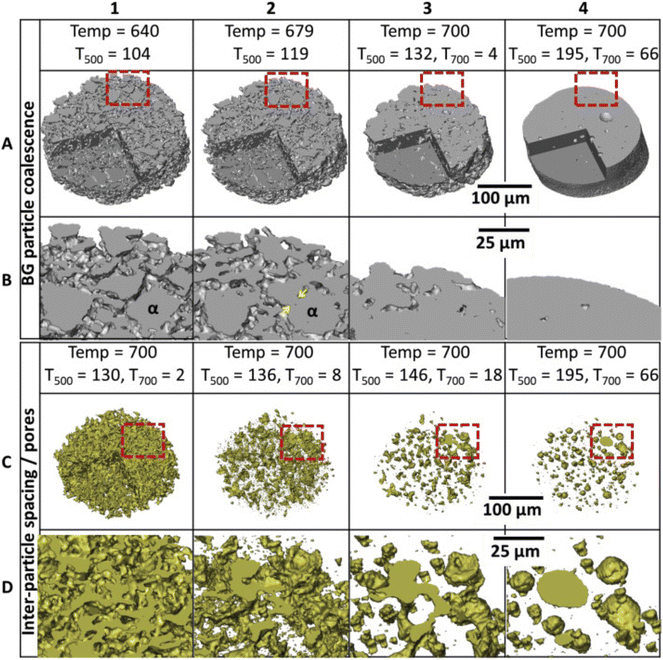 |
| Fig. 4 A 3D reconstructed volume of a small section of a scaffold strut showing the bioactive glass (BG) (A, B) and inter-particle spacing (pores) (C, D) phases sequentially with time and temperature. The lower images (C and D) are magnified areas of the red boxes in A and D. Yellow arrows indicate neck formation between particle α and one of its neighbors. From ref. 94 with permission from Elsevier, 2019. | |
To date, perhaps the most systematic, comprehensive and specific study on the influence of ALAEs on thermal processing of bioactive glasses was reported by Brink,95 who analyzed the thermal behavior of 40 silicate glasses in the Na2O–K2O–MgO–CaO–B2O3–P2O5–SiO2 system by primarily using hot-stage microscopy. The silica content in the glasses was in the range of 39 to 70 wt% and all glasses containing below 54 mol% SiO2 were shown to devitrify during viscosity measurements. Generally, glasses that devitrified contained more alkaline modifiers but less alkaline-earth modifiers than glasses with a large working range (or alternatively, a high Tx − Tg range). This study suggested that such temperature interval can be enlarged in bioactive glasses by decreasing the amount of alkaline modifiers (especially Na2O) and/or increasing the amount of alkaline-earth modifiers (especially MgO). These conclusions, coming from the analysis of a relatively large number of glass systems, are consistent with those arisen in the previously-cited reports.
It is worth underlining that, if the complexity of glass composition increases – which is the typical case of most bioactive glasses –, it is impossible to precisely recognize the effect of each single alkaline/alkaline-earth modifier on thermal behavior. On one hand, Mancuso et al.96 prepared a set of multicomponent bioactive silicate glasses containing various amounts and types of alkaline (Na2O, K2O)/alkaline-earth modifiers (CaO, MgO) and reported that they showed similar thermal profiles upon heating according to hot-stage microscopy, which were characterized by an increase in sample dimensions after the maximum shrinkage temperature and before the melting onset took place. These results are consistent with the findings reported by Baino et al.89 who found that a bioactive glass (CEL2) with composition 45SiO2–3P2O5–26CaO–7MgO–15Na2O–4K2O (mol%) exhibited a significant volumetric expansion after the first densification step. Hence, these studies suggest that the sintering profiles of multicomponent silicate glasses are relatively insensitive to the presence of different network modifiers in the glass structure. On the other hand, hot-stage microscopy analysis reported by Fiume et al.97 on a bioactive glass with similar composition to the previously-cited CEL2 (47.5SiO2–2.5P2O5–20CaO–10MgO–10Na2O–10K2O (mol%)) revealed an elbow-shaped sintering profile without any volumetric expansion before melting. This was also the same profile exhibited by the simpler 45S5 quaternary composition.98
Perhaps, a clearer, more conclusive and general picture about the role of modifiers on glass sintering could be obtained by systematically analyzing the existing data from the literature by machine learning/artificial intelligence approaches.
Modifiers also affect the thermal expansion coefficient (TEC) of glasses; when a glass coating has to be produced, the TEC of glass should match that of the substrate to prevent the glass pulling away from the base implant upon processing.17 The TEC of 45S5 Bioglass® (15 × 10−6 °C−1) is significantly higher than that of titanium alloys (about 9 × 10−6 °C−1) and alumina (about 8 × 10−6 °C−1), which are commonly used to fabricate orthopedic and dental implants: therefore, there has been the need for developing new glass formulations with a more suitable TEC for use as coating materials. In this regard, bioactive glasses belonging to the SiO2–CaO–MgO–Na2O–K2O–P2O5 system have been widely investigated to match the TEC of the Ti6Al4 V alloy and alumina.99,100 Partial replacement of Na2O with K2O and CaO with MgO was the most common strategy to design and adjust the TEC of the glass in a controlled way.101,102
Gonzalo-Juan et al.103 also investigated the role played by alkaline/alkaline-earth modifiers on the injectability of bioactive pastes produced using a melt-derived CaO–MgO–SiO2–Na2O–P2O5–CaF2 glass and two organic carriers (polyethylene glycol (PEG) and glycerol). While pure physical interactions were detected between PEG and the surface of the bioactive glass particles, chemical bonding was observed between glycerol and glass, enhancing the network cross-linking degree. Accordingly, the network of glycerol-glass paste was more condensed in comparison to that of PEG-glass and as-such glass because the fraction of Q2 sites significantly decreased while the fraction of Q3 units increased due to the covalent interaction between glass particles and the –OH groups of glycerol. Such chemical interactions between organic carrier and glass particles negatively affected the viscoelastic behavior of the pastes yielding to lower flowability of glycerol-glass composites as compared to the PEG-based counterpart.
3.1.3 Effects on crystallization and mechanical properties.
As already mentioned, many bioactive glass products are obtained by sintering of “greens”, which may be associated to concurrent devitrification leading to glass-ceramic implants. In general, crystallization can have benefits and disadvantages. The motivation behind developing bioactive glass-ceramics is typically dictated by the mechanical limitations of bioactive glasses.9 Kokubo et al.104 developed the bioactive glass-ceramic A/W Cerabone, which is obtained from the crystallization of a glass in the SiO2–P2O5–CaO–MgO–CaF2 system and contains fluorapatite and wollastonite crystals. This glass-ceramic is mechanically stronger than other bioactive glasses (e.g. 45S5 Bioglass®) due to the inherently higher mechanical properties of crystals compared to the amorphous matrix, is able to bond to bone in vivo and can be machined to match anatomical sites.104
The presence of specific crystalline phases can be deliberately induced by properly modulating glass composition (including the content of ALAEs) and/or applying subsequent heat treatment. Phase diagrams are useful to predict if a melt of given formulation will originate either a fully amorphous glass or a partially-crystalline solid (glass-ceramic). For example, a proper amount of CaO was introduced in the A/W Cerabone in order to obtain wollastonite crystals in the final product. On the other hand, devitrification may compromise the material bioactivity: in this regard, 45S5-derived glass-ceramic is less bioactive than the parent glass since crystallization of a calcium-sodium silicate phase lead to a decrease of apatite-forming ability because of a decrement in the overall glass-ceramic reactivity, which is mainly associated to the amorphous phase.105 Therefore, the achievement of a balance between bioactivity and mechanical strength must is a challenge.
The thermal properties of a glass determine the processing regime for bioactive glasses and glass-ceramics, while thermal properties are in turn dictated by glass composition. Controlled crystallization is key for tailoring the properties of glass-ceramics. Spontaneous crystallization, by contrast, caused by a pronounced tendency to devitrify during cooling of the melt, is undesirable because it prevents obtaining a bioactive glass in an amorphous state and makes it challenging – if not impossible – to control crystal phases, size and number via heat treatment. Bioactive glasses tend to crystallize easily during both cooling of the melt and heat treatment of a glass, and one of the main reasons is their low NC compared to conventional silicate glasses.5,106 While many bioactive glasses show surface crystallization, the famous 45S5 Bioglass® shows both surface and internal crystallization, which does not allow viscous flow to take place for both bulk and powder sample, thereby impeding full densification.107–109
The sinterability window of 45S5 Bioglass® is extremely narrow and, thus, it cannot be sintered without undergoing devitrification.110 This issue pushed scientists to develop glass compositions with appropriate dosage of modifiers and, thus, a larger sintering window allowing the fabrication of mechanically stronger product. For example, Fu et al.111 prepared foam-replicated 13–93 glass scaffolds having a compressive strength of 18 MPa, which was significantly higher than that of 45S5 Bioglass®-derived glass-ceramic scaffolds (0.2–0.4 MPa (ref. 105)) with analogous porosity (85 vol%) and 3D trabecular architecture.
Studies on Biosilicate112 showed that the glass-ceramic with 34% of crystalline volume exhibited much better mechanical properties than the parent glass, while the crystal size seemed to have a lower influence on mechanical performance. Fig. 5 highlights this change in flexural strength while also providing microstructural images of the glass-ceramics.112 The type of crystal phases can also have direct influence on the mechanical properties of a glass-ceramic. This is particularly noticeable in apatite-containing glass-ceramics such as the apatite-wollastonite, apatite-mica or apatite-mullite systems,113 where the function of the apatite phase was to provide bioactivity, while the other phase(s) provided mechanical strength. In the case of A/W Cerabone, wollastonite (CaSiO3) strongly improves the compressive strength (up to 1080 MPa), flexural strength (up to 215 MPa), Young's modulus (118 GPa) and fracture toughness (up to 2 MPa m1/2) in comparison to bioactive glass-ceramics without wollastonite crystals.
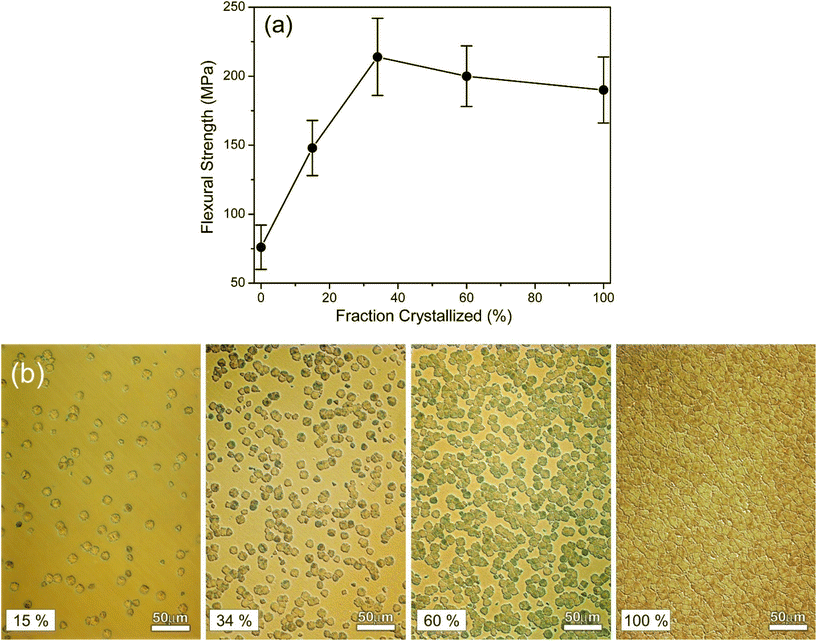 |
| Fig. 5 Effect of crystallization percentage on mechanical properties of a phospho-silicate combeite glass-ceramic. (a) Flexural strength vs. fraction crystallized during a multistage heat treatment schedule. (b) Optical microscope images showing microstructure examples of increasingly crystalized glass-ceramic samples. From ref. 112 with permission from Elsevier, 2001. | |
Machinability of glass-ceramics is also important in the production of orthopedic and dental implant. In the Bioverit glass-ceramics, machinability originates from the presence of mica crystals. While both Bioverit I and Bioverit II contain a mica crystalline phase, the mica crystals in the former show a morphology resembling flat flakes, while those in the latter have the shape of spherical lamellae, giving the crystals a cabbage-like appearance.114,115 As a result, Bioverit II can be machined more easily than Bioverit I.
Finally, alkali-free bioactive glass-ceramics have also been developed within the CaO–MgO–SiO2–P2O5–CaF2 glasses and glass-ceramics composition, in a diopside (CaMgSi2O6) – fluorapatite (Ca5(PO4)3F) – tricalcium phosphate (3CaO·P2O5) join. Favorable results by sintering the glasses to appropriate density, leading to good bending strength have been reported. By opting for alkali-free compositions, it is possible to avoid the undesirable effects of alkali ions on the sintering and crystallization behaviors of the glasses. Moreover, the choice of a chain silicate mineral like diopside, exhibiting an elongated and interlocking morphology, contributed to the improvement of mechanical properties.76,116,117
3.2 Gel-derived glasses
Sol–gel technique is attracting an increasing attention due to the lower temperature required for the process compared to melting as well as the possibility to synthesize glasses with different shapes (bulk, powders, coatings…) and tune the composition, porosity and surface area.118–120 In particular, the control and modulation of the composition of sol–gel glasses have been the subject of several works.118,121 In fact, the introduction of specific elements can confer peculiar properties to the material.24 However, several parameters, such as the type of used precursors, their amounts and ratio, have to be taken into consideration because they could affect the gel formation, the gelling time, or induce the precipitation of crystalline phases, which in turn can influence glass porosity and bioactivity. Among the elements present or introduced in the compositions of bioactive glasses obtained via sol–gel, some ALAEs have been reported to play a key role.
Calcium, for example, has always been present in bioactive sol–gel glass compositions, since the first SiO2–CaO binary or SiO2–CaO–P2O5 ternary systems122–124 because of its intrinsic role in the bioactivity mechanism. Ca is conventionally introduced into sol–gel bioactive glass compositions as Ca(NO3)2·4H2O. However, some works highlighted a non-homogeneous distribution of Ca, particularly in large monoliths.125,126 The cause of this inhomogeneity was attributed to the solubility of calcium nitrate in the pore liquor, which is a by-product of the condensation reaction during gelation and ageing steps. In fact, this liquid is eliminated during the shrinkage of the gel and evaporates during the drying phase. Therefore, calcium nitrate remains as a deposit on the external surfaces of the monolith and manages to enter and diffuse in a limited way only after the stabilization heat treatment.126,127
In order to improve Ca introduction in the glass network, several authors investigated different calcium sources, such as calcium chloride, calcium acetate, calcium hydroxide, calcium ethoxide or calcium methoxyethoxide (CME).126,128–131 highlighting advantages and disadvantages in sol–gel glass processing. For example, some authors showed that the use of calcium alkoxides causes a rapid gelation of the sol.131,132
Bossard et al.131 investigated in detail the mechanisms and precursors that allow the Ca incorporation into sol–gel silica-based bioactive glasses by means of low-temperature synthesis. They evaluated the Ca introduction in a bioactive glass composition (75SiO2–25CaO wt%) employing different precursors, including CaCl2, tricalcium dicitrate tetrahydrate, calcium acetate monohydrate, calcium ethoxide (CE – Ca(OC2H5)2), or calcium hydroxide (CH – Ca(OH)2). First of all, they estimated the influence of the pH on Ca incorporation, since previous studies have observed a good Ca introduction for samples using calcium alkoxides precursor (at pH > 2), whereas Ca was not incorporated employing CaCl2 or Ca(NO3)2 (at pH < 2).126 However, the obtained results evidenced that the pH alone does not explain the Ca introduction: even if the pH was raised to 5 in all the syntheses, Ca was not incorporated using CaCl2 or calcium citrate. The authors highlighted the significant importance of the basic property of the Ca precursor in the incorporation mechanisms. In fact, even if the pH of the sol was raised above the isoelectric point of the silicic acid, allowing the formation of SiO− and therefore the possible ionic-covalent bond with Ca2+ ions, the nature of the Ca2+ counterion – especially that of the neutral salts (e.g. Cl−) – prevents the formation of a SiO−–Ca2+ bond. Therefore, Ca incorporation at low temperatures is allowed when strongly basic Ca precursors are used.
Among alkali metals, the successful incorporation of Na in sol–gel bioactive glass composition was achieved only recently. Indeed, all the sol–gel bioactive compositions belonging to 49-86S family contain SiO2, CaO and P2O5.122 The issue of introducing Na2O into the sol–gel composition is due to the high hydrolytic reactivity of sodium alkoxide in water. A first attempt to introduce Na in a phosphate-based sol–gel bioresorbable glass composition was performed by Carta et al.,133 employing alkoxide precursors in an ethylene glycol solution and nitrogen atmosphere. Despite the good results obtained, this synthesis was discontinued, probably due to the technical difficulties and cost related to the highly-controlled environment required for the process.
Subsequently, other authors developed a new sol–gel-based protocol for the synthesis of Na2O-containing bioactive glass-ceramics in aqueous solution under ambient conditions using NaNO3 as Na precursor.134 The adopted process allowed obtaining the complete decomposition of NaNO3 for samples sintered at 1000 °C, with the consequent formation of Na2Ca2Si3O9 as a crystalline phase. NaNO3 was adopted as Na precursor in different works135,136 but some concerns recently arose about its use. In this regard, Chitra and co-authors136 compared the role of sodium nitrate and sodium hydroxide in the synthesis and properties of SiO2–P2O5–CaO–Na2O glass. Concerning the synthesis process, the authors evidenced a greater Na introduction when NaNO3 was employed as Na source and an increased tendency to crystallize. According to the authors, this result, together with that obtained from the evaluation of the bioactivity and biological testing, suggested that sodium nitrate was a preferable precursor to develop bioactive and stable bioactive glass via sol–gel.
As regards mesoporous sol–gel glasses, Kumar et al.137 in 2017 developed for the first time a mesostructured 45S5 bioactive glass by acid-assisted sol–gel method followed by evaporation-induced self-assembly process, using sodium acetate and calcium acetate hydrate as sodium oxide and calcium oxide sources, respectively. They obtained a fully amorphous glass with a mesoporous structure; however, the amount of alkali/alkaline earth elements was significantly lower (especially Na) than the theoretical composition.
Magnesium138–141 and potassium142,143 have also been introduced as nitrates in sol–gel compositions. However, no particular effects on the glass synthesis process were found in the analyzed studies, which mainly concerned the investigation of structure, bioactivity and biological behavior. For example, Fiume et al.141 synthesized a complex composition via sol–gel (SiO2–P2O5–CaO–MgO–Na2O–K2O) and highlighted that the introduction of several alkali/alkaline earth elements in the system, together with the need to perform a calcination treatment at high temperature to remove the nitrates used as precursors, produced the partial crystallization of the material. The formation of crystalline phases affected the specific surface area (SSA), which decreased by increasing the calcination temperature and was significantly lower than the SSA of simpler sol–gel compositions.
The formation of crystalline phases during heat treatments in sol–gel processes can affect both the SSA and the formation of an ordered mesoporosity. As previously noted, very complex compositions and the use of reagents, such as nitrates, which require high temperatures to be removed, often induce the formation of crystalline phases. However, some authors143 proposed a new sol–gel method to synthesize a 45S5-derived glass with high amount of CaO (45.6 mol%), partial/complete substitution of sodium oxide with potassium oxide, and crystallization temperature pushed to 900 °C. These authors observed that by varying the content of alkaline elements, and in particular by using the highest potassium amount, it was possible to avoid crystallization phenomena. This allowed producing amorphous materials even with a calcination temperature of 850 °C.
Recently, the introduction of lithium in sol–gel glasses has also been investigated144–147 due to its role in bone regeneration and osteochondral tissue. Different Li precursors were used, including lithium citrate, lithium chloride and lithium nitrate; however, the influence of lithium in the sol–gel process was little explored. Moghanian and co-authors evidenced a lower cross-link density and lower crystallization temperature for the Li-containing bioactive glass, and consequent higher stability of the glass structure. These results can be ascribed to the smaller ionic radius of lithium and the different coordination number in comparison with calcium. Maçon et al.145 investigated the role of lithium nitrate and lithium citrate as precursors in the synthesis of binary sol–gel glasses (90SiO2–10Li2O mol%) by adding the precursors in an acidic solution of hydrolyzed tetraethylorthosilicate (TEOS). They observed a different gelation time using the two precursors: the sol containing lithium citrate gelled in 1 h, while the sol containing lithium nitrate gelled in 3 days. These differences were ascribed to the pH increase (up to 5.3, above the isoelectric point of silicic acid) for the solution containing the lithium citrate (due to the release of citric acid) and the consequent change of the kinetics of the silica network condensation. The authors also underlined that that the use of lithium citrate allowed obtaining mesoporous glass containing lithium as a network modifier, due to the lower decomposition temperature of citrate; on the contrary, a dense glass-ceramic was obtained using lithium nitrate since the complete nitrate decomposition generated lithium metasilicate. As already observed for Ca, the use of nitrates involves high calcination temperatures which can induce the formation of crystalline phases.
Finally, several articles can also be found in the literature concerning Sr-doped sol–gel glasses.148–154 Usually, Sr substitutes Ca because they have the same valence and similar ionic radius; also, both elements should act as network modifiers. Sr is introduced in sol–gel glass composition again mostly as nitrate; however, some studies reported the use of Sr chloride as a precursor.153 The composition of the obtained glasses often differs from the theoretical one and has a lower Sr content.148 This is due – like for Ca-containing glasses – to the use of nitrates, which allow the Sr incorporation only by ion diffusion during the heat treatment; furthermore, some of the strontium nitrate is removed during washing steps performed before the heat treatment. The introduction of Sr has no effect on the morphology of the obtained glass (in the case of particles), but a decrease in the oxygen density and an increase in the size of the particles were observed due to a slightly larger ionic radius of Sr compared to Ca, which produces an expansion of the glass network.148,149
In another work, Taherkhani et al. investigated the effect of the substitution of Sr for Ca in a mesoporous sol–gel bioactive composition.154 They evidenced a tendency to crystallize with increasing Sr amounts and the consequent formation of Sr2SiO4. The performed BET analyses showed mesoporous texture with a high specific surface area; however, the influence of Sr and the resulting crystallization on the specific surface area was not investigated in detail.
Recently, other alkali and alkaline earth metals have been introduced in sol–gel bioactive glass compositions. For example, Ouyang and co-workers evaluated the effect of rubidium addition (0, 1, 3 and 5 at%) into a bioactive SiO2–CaO sol–gel glass.155 The incorporation of Rb did not affect the synthesis process as well as the glass size, shape and structure. However, its amount incorporated in the glass was lower than the theoretical one; the authors explained this difference highlighting that, also in this case, the metallic ions diffuse into the silicate matrix only after the heat treatment and the washing step performed before the treatment could remove the Rb precursor. Moreover, the larger radius of Rb as compared to Ca decreased its affinity with Si and the repulsion interaction between Ca and Rb ions (both positively charged) could further decrease its adsorption.
Majumdar et al. investigated the introduction of barium on 45S5 sol–gel glass nanoparticles (44.85SiO2–2.6P2O5–24.3Na2O–26.9CaO–1.35BaO mol%). Doping with Ba had no influence on the synthesis process: the obtained glass had a mesoporosity similar to 45S5 and a slightly lower specific surface area.156
3.3 Processing of composites and hybrids
The introduction of alkali or alkaline earth ions into glass compositions and their effect on the synthesis process can also affect the design of composite and hybrid materials. In particular, hybrid organic/inorganic systems consist of interpenetrating nanoscale networks of silica and biodegradable polymers and can be produced by introducing a polymer in the sol–gel process. The presence of alkali or alkaline earth elements in sol–gel glasses and, above all, the use of specific precursors of these elements can significantly influence the synthesis process of the hybrid material.
Ca, for example, has been introduced in the hybrids as calcium nitrate157 but, as previously discussed, high temperatures for removing nitrates must be reached, which are not compatible with the synthesis of hybrids. For this reason, a different Ca source is needed for hybrids.
In this context, different authors126,132 investigated the role of calcium precursors in the sol–gel synthesis of hybrids. The comparison was performed among calcium methoxyethoxide (CME), calcium chloride and calcium nitrate, investigating also the temperature at which Ca is incorporated into the 70S30C (70SiO2–30CaO mol%) network. These studies evidenced that, by using calcium nitrate, Ca enters the glass network only above 400 °C, while calcium was not incorporated at any temperature if calcium chloride was adopted. Instead, the use of CME permitted the incorporation of Ca at very low temperature (about 60 °C), which is useful for the synthesis of hybrids.126 In fact, CME as Ca precursor allowed a fast gelation in the mixed organic/inorganic mixing system without using hazardous catalysts, such as hydrofluoric acid, and a better Ca incorporation.132 Moreover, the Ca source strongly influenced the dissolution rate of hybrid system, since CME allows effective cross-linking of the polymer and a consequent steady release, while a sudden release was observed by adopting CaCl2 as a precursor. Also, Lao et al.158 have recently developed a simple process at room temperature for synthesizing bioactive glass/gelatin hybrid scaffolds. In this work, Ca(OEt)2 was used as the calcium source and the gelling of the hybrid solution was delayed to 2 hours using a water/TEOS molar ratio reduced by two under diluted conditions. These studies represented an advancement in the synthesis of organic–inorganic hybrid scaffolds at room temperature.
The problem of the heat treatment necessary to incorporate ions starting from nitrates into the sol–gel glasses does not arise if composite materials are produced. However, some studies have highlighted the effects of alkali/alkaline earth ions on the properties of the obtained composites. For example, in a recent study150 some authors evaluated the influence of Sr incorporation in sol–gel bioactive glasses in chitosan/alginate/strontium-doped glass composite scaffolds. They highlighted how the presence of Sr in the glass improved the mechanical properties of the scaffolds. In fact, it was observed that Sr, after substituting Ca, increased the number of interactions in the glass bonding network due to the higher ionic radius. On the other hand, no effects of the replacement of Ca with Sr were found in terms of degradation profile and scaffold swelling. On the contrary, Jalise et al.,159 evidenced an accelerated degradation rate for Sr-modified bioactive glass nanoparticle/gelatin scaffolds, and they ascribe this behavior to the larger ionic radius of Sr that favors the disorder of the glass lattice.
4. Physiological role of ALAEs
An understanding of the natural function of ALAEs in biological systems is critical in unlocking the breath of applications in bioactive glasses. Elements such as sodium, potassium, and calcium exist at high concentrations in the body, while lithium, strontium, and barium exist at very trace amounts. Table 1 provides the concentration of the many inorganic including some ALAEs and ions in the body. This following section discusses the physiological role of each alkali and alkaline earth element as well as reviews in vitro and in vivo studies showing the biological impact of BGs.
Table 1 The main elements that compose the human body molecules (including water). Na, K, Ca, Mg and P which are the main components of BGs are among these elements
Element |
Symbol |
Percent mass |
Percent atoms |
Oxygen |
O |
65.0 |
24.0 |
Carbon |
C |
18.5 |
12.0 |
Hydrogen |
H |
10 |
62.0 |
Nitrogen |
N |
3.2 |
1.1 |
Calcium |
Ca |
1.5 |
0.22 |
Phosphorus |
P |
1.0 |
0.22 |
Potassium |
K |
0.4 |
0.03 |
Sulfur |
S |
0.3 |
0.038 |
Sodium |
Na |
0.2 |
0.037 |
Chlorine |
Cl |
0.2 |
0.024 |
Magnesium |
Mg |
0.1 |
0.015 |
All others |
|
<0.1 |
<0.3 |
Compared to transition metals, ALAEs do not have as versatile physiological effects, but are almost always more biocompatible. As shown in Table 1, most of the ALAE elements are abundant in the body while the transition metals or rare earth elements are not. However, in order to achieve expression of certain proteins, exhibit strong antibacterial effects, or create reactive oxygen species, the addition of transition metals may be required. Table 2 provides a comparison of the ALAE elements with several other species most commonly studied in BGs.
Table 2 Comparison of alkali and alkaline earth elements to other relevant species when added to bioactive glasses
Class |
Species |
Positive interaction |
Negative interaction |
Ref. |
ALAEs |
Li |
- Increases osteoblast cell activity |
- Toxic in high concentrations |
145, 146, 160 and 161 |
- Increases angiogenesis through Wnt/β-catenin pathway and TGFβ |
|
Na |
- Encourages dissolution through NBO creating |
- Drastically increases pH during dissolution |
45 and 46 |
K |
- Encourages dissolution |
- Increases pH during dissolution |
162–165
|
- Improve glass forming ability and cause mixed alkali effect |
|
Mg |
- Highly cyto-compatible |
- Decreases solubility |
93, 116 and 166–168 |
- Increases cell attachment |
- Slows dissolution kinetics |
- Increases osteogenic differentiation |
- Decreases rate of calcium-phosphate layer precipitation |
- Increases expression of collagen type 1, alkaline phosphatase, and osteocalcin |
|
Ca |
- Stimulates expression of angiogenic genes: VEGF and bFGF |
|
10 and 169 |
- Encourages HAp layer precipitation |
|
- Regulates homeostasis; specifically factors II, VII, IX, X, and XI |
|
Sr |
- Improves osteogenesis |
|
156 and 170–181 |
- Inhibits osteoclastogenic action |
|
- Anti-bacterial |
|
Ba |
- Anti-inflammatory through reduction of LPS-induced elevation of interleukin-6 (IL-6) |
- Toxic in high concentrations |
156, 182 and 183 |
- Potential for magnetic hyperthermia cancer treatment applications |
|
Transition metals |
Ti |
- Minimal effect on pH during dissolution |
- Decreases dissolution rate of glass through strengthening glass network |
184–187
|
- Increases mechanical properties of glass |
- High TiO2 concentrations required for antibacterial activity |
- Increased ALP activity |
|
- Increases dentin remineralization in dental applications |
|
V |
- Luminescent on 450–800 nm range |
- Extensive studies on cytocompatibility still required |
188–192
|
- V5+ initiates early stages of wound healing |
- Oxidation state of dissolution product may have different effects |
- In vivo studies show significant increase in wound vascularization and bone mineralization |
- Only small compositions studied (up to 3 mol%) |
Mn |
- Stimulates expression of osteogenic genes: ALP, type 1 collagen, osteocalcin, bone morphogenetic proteins, and sICAM-1 |
- Concentrations limited to below 5 mol% (less than 0.1 mg ml−1) for long term biocompatibility |
193–199
|
- Magnetic properties with potential for magnetic hyperthermia |
|
- Antibacterial against several bacterial strains |
|
Fe |
- Magnetic properties with potential for magnetic hyperthermia |
- Cytocompatibility requires more study |
200–204
|
- Potential for Fenton reaction therapy |
|
- Improved HAp formation |
|
Co |
- Stimulates expression of angiogenic genes: VEGF, HIF-1α, and ALP |
- Cytotoxicity at moderate concentrations (above 10 ppm) |
205–210
|
- Increases ESM deposition |
- Can be carcinogenic and genotoxic through various pathways |
- Radiopaque |
- Hinders osteogenic actions |
Ni |
- Increase mechanical strength |
- Limited bioactive role |
211–213
|
|
- No effect on mineralization |
Cu |
- Stimulates expression of angiogenic genes: VEGF, HIF-1α, ALP. bFGF, and PDGF |
- Some studies show toxicity at low concentrations (1 μm mL−1) |
38, 201, 209 and 214–220 |
- Antibacterial against a wide variety of strains |
|
- Anti-inflammatory through over-expression of interleukins |
|
- Promising for applications in photothermal therapy |
|
Zn |
- Increases osteopontin expression to facilitate osteogenesis |
- Large pH changes upon dissolution (composition dependent) |
215 and 221–227 |
- Antibacterial against several bacterial strains |
|
- Encourages enamel remineralization |
|
- Antimicrobial |
|
Zr |
- Increases mechanical properties |
- Decreases dissolution rate of the glass |
228–231
|
- Radiopaque |
|
Ag |
- Antibacterial against several bacterial strains |
- Can show cytotoxic properties with concentrations varying from 1–20 mg/mL |
232–240
|
Au |
- Antibacterial against Gram positive and Gram negative bacteria |
- Studies limited to Au-nanoparticle composites |
241 and 242 |
- Does not hinder hydroxyapatite formation |
- Au nanoparticle concentrations studied are very low (0.15–0.2 mol%) |
Metalloids/non-metals |
B |
- Encourages precipitation of HAp |
- Inhibits the proliferation of bone marrow stromal cells if B concentration is >0.65 mM |
4, 78, 189 and 243–248 |
- Stimulates expression of angiogenic gene VEGF |
|
- Encourages increased secretion of ECM |
|
- Early stage research shows promise for axon regeneration |
|
Si |
- Primary component of most BGs |
|
249–253
|
- Stimulates expression of osteogenic genes: ALP, BSP, BMP, collagen type 1 |
|
- Stimulates expression of osteogenic genes VEGF, ECM |
|
P |
- Stimulates expression of osteogenic genes matrix Gla protein (MGP) for bone |
|
63, 63, 106, 245 and 254–257 |
- Stimulates expression of angiogenic genes: VEGF and FOXC2 |
|
- Enhances precipitation of HAp |
|
Ge |
- Encourages precipitation of Hap |
- Limited studies available with in vitro or in vivo specific results |
258–260
|
Te |
- Strong antibacterial effects against Gram positive and Gram negative strains |
- In depth in vitro and in vivo studies required to ensure biocompatibility and long term efficacy |
261 and 262 |
- Alkali-tellurite glasses able to form CaP layer at surface during dissolution |
|
Post-transition metals |
Al |
- Increases mechanical properties of glass |
- No major biological properties |
263–267
|
|
- Some compositions show cytotoxic effects |
Ga |
- Excellent antibacterial properties |
|
191 and 268–273 |
- Produces reactive oxygen species |
|
Bi |
- Radiopaque |
- Hinders HAp formation at higher concentrations |
274–277
|
- Photothermal effect for cancer therapy |
- Higher concentrations less effective than un-doped compositions in cell proliferations (<5 wt%) |
- Antibacterial effect |
|
- Antifungal properties |
|
Rare earth elements |
Ce |
- Antioxidant activity through reduced oxygen speies |
|
191, 269, 273 and 278–285 |
- Can be antibacterial at higher concentrations |
|
- Either no effect or positive effect on cell viability in most studies |
|
Eu |
- Strong luminescence under ultraviolet radiation |
|
286–288
|
- Stimulates expression of osteogenic genes: ALP, OCN, OPN and COL-I in BMSC cells |
|
- Stimulates expression of angiogenic genes: CD31, MMP9, VEGFR1/2 and PDGFRα/β of HUVEC cells |
|
Gd |
- Activates Wnt/β-catenin signaling pathway |
- Decreases dissolution kinetics of the glass |
287 and 289–291 |
- Stimulates expression of osteogenic genes: OCN and BSP |
- Slightly lower cell viability than glasses containing no rare earth elements |
Sm |
- Increases dissolution rate of glass |
- Biocompatibility as function of Sm concentration not thoroughly studied |
292–295
|
- Makes local pH during dissolution more stable |
|
- Strong luminescent properties in visible wavelengths |
|
4.1 Alkali Elements
4.1.1 Lithium.
4.1.1.1 Biological activity.
Lithium is the smallest of the alkali ions yielding the highest charge density and lowest coordination numbers for complex binding.296 Although there is no known natural biological role of lithium, this element has been implicated in a variety of biomedical processes ranging from pharmacological treatment of neuropsychiatric disease and cytopenia to regenerative medicine applications of angiogenesis and neuron regeneration.160,297
Oral lithium carbonate is approved for the treatment of bipolar disorder.298 The mechanism of action has still to be definitely elucidated; however, lithium was revealed to produce a myriad of both excitatory and inhibitory effects at the cellular level.299–302 The glycogen kinase synthase 3 beta (GSK-3B) pathway has been implicated with bipolar disorder and as a possible therapeutic target of lithium. It has been proposed that lithium inactivates apoptotic activities in the GSK-3B pathway serving as a neuroprotective agent. Other therapeutic targets investigated include mitochondrial proteins, glutamatergic neurons, and circadian rhythm proteins.300,301
The therapeutic potential of lithium does not end with neurosynaptic disorders, but this small alkali metal has been heavily investigated in the treatment for granulocytopenia.303 Granulocytes, such as neutrophils, basophils, and eosinophils can become depleted as a result of a variety of insults including strong chemotherapeutics.304 Lithium has been shown to boost the production of such cells.305–307
Lithium salts have been investigated in angiogenesis to determine a possible role in tissue engineering applications. Guo et al. investigated the effects of millimolar concentrations of lithium chloride on human brain endothelial cells and rat astrocytes. They illustrated that lithium ions, when compared with sodium ions, promoted higher phosphorylation of GSK-3B and increased expression of Vascular Endothelial Growth Factor (VEGF).308 VEGF is a key protein in angiogenesis, i.e. the growth of new blood vessels.309 Furthermore, lithium has been explored for a possible role in axonal regeneration. Lithium initiates all key cellular processes in axonal regeneration including inhibiting GSK-3, expression of c-JUN, expression of BCL-2, and induction of angiogenesis.308,310–312 The combined effects of these cellular processes have been illustrated in a variety of animal models.313,314
Another key aspect related to biological effects of lithium lies in the interplay between similar chemical species, mainly sodium and magnesium. Sodium is a monovalent alkali ion with the most similar size to lithium while magnesium, although being divalent, has a size comparable to lithium. It has been shown that lithium can compete with sodium and magnesium for various substrate binding sites and ion channels.299,300 For example, lithium can inhibit several magnesium-dependent proteins including G proteins, inositol monophosphates, and bisphosphate 3-prime-nucleotidase.299,315–317
Administration of lithium can carry a set of negative consequences. By mimicking alkali and alkali earth metals, lithium can cause damage to a variety of organ systems. Chiefly, lithium will utilize sodium transporters in the kidney to increase its own reabsorption. Therefore, in states of dehydration and increased expression of sodium transporters, excessive lithium will be reabsorbed creating higher serum levels of lithium that perpetuate lithium toxicity.318,319 Lithium is also known to induce nephropathy causing kidney failure; however, the mechanism of action has still to be clearly elucidated.320 Through competing with calcium, lithium can block calcium receptors in the parathyroid leading to hyperparathyroidism.321 Lastly, lithium can displace potassium in myocardial tissue, possibly leading to arrhythmia.322 Nonspecifically, lithium has been associated with psoriasis, hair loss, tremor, nausea, and diarrhea.298
4.1.1.2 BG evidence.
Khan et al. studied the in vivo biological properties of binary doping of lithium and strontium into boro-silicate BG composition (10.9Na2O–23.9CaO–1.5TiO2–1.4B2O3–1.7P2O5–56.3SiO2, by mol%).323 Lithium-containing BG (L-BG) was doped with 0.25 mol% Li2O and the binary doped strontium and lithium-containing BG (LS-BG) was doped with 0.3 mol% Li2O and 1 mol% SrO. The BG powders were isostatically cold-pressed in the form of implantable scaffolds. Preliminary bioactivity testing and in vitro characterization showed promising results for both doped samples. Crystallization of HAp was decreased through lithium doping in the bioactivity studies. However, both L-BG and LS-BG showed greater concentrations of bi-carbonates and bi-phosphates in the supernatant from static dissolution studies. This was argued to increase cell proliferation through the synthesis of extracellular matrix and collagen in vitro. All doped BGs showed accelerated cell proliferation rates as compared to the base glass composition with L-BG showing the highest performance. BG scaffolds were then implanted into sixteen New Zealand White rabbits. Chronological radiographs were taken immediately after implantation and once a month up to four months. Intermuscular injections of fluorochrome were administered prior to sacrificing in order to quantify new bone growth along with micro-computed tomography to assess bone-in growth into the scaffolds. L-BG showed increased bone growth as compared to the base glass with enhanced defect healing. LS-BG radiographs show narrowing of the bony defects and shrinking of the implant at one month post operation. Fig. 6 shows images of the radiographs taken monthly post operation for each of the implanted compositions. Histological evaluations after 4 months showed that doped samples had significantly increased levels of angiogenesis. Specifically, lithium-doped BG samples had higher proliferation of osteoblasts and angiogenic proliferation. Finally, the researchers concluded that lithium, even at small doping levels, had a profound effect on bone healing, better implant anchorage, and formation of new blood vessels. However, further studies are recommended to determine the exact mechanisms that Li+ has in the early stages of dissolution.
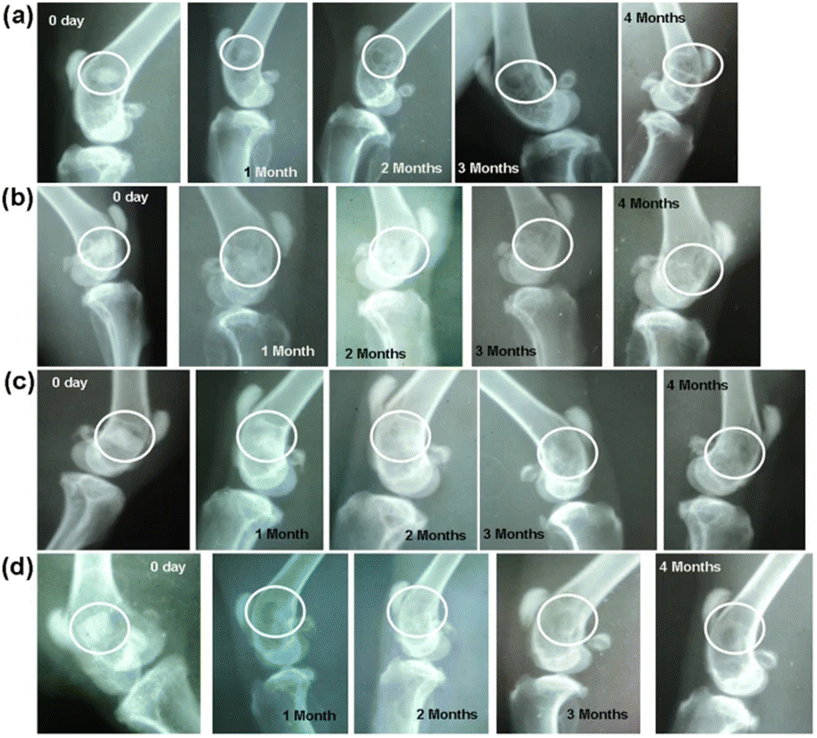 |
| Fig. 6 Radiographs taken after implantation and after 1, 2, 3 and 4 months post-operation. BG compositions implanted are (a) BG, (B) L-BG, (c) S-BG, and D (LS-BG). From Khan et al.323 with permission from Springer Nature, 2016. | |
A study published in 2017 specifically investigated the angiogenic nature of lithium-containing BGs.324 The 45S5 composition was doped with 5 mol% Li2O directly replacing Na2O. The effect of the dissolution products from both 45S5 and 45S5.5Li were evaluated in vitro through a series of tests including cell proliferation assay, cell migration assay, cell transmigration assay, enzyme linked immunosorbent assay, and determination of β-Catenin by Western Blot. Greater cell proliferation was shown with the dissolution products containing Li+, however both BG compositions showed increased performance due to proangiogenic ions such as silicon. Migration studies showed a significantly increased performance of lithium containing 45S5 with 28 ± 2% gap closure after 8 hours as compared to roughly 20% closure for 45S5. The tubulogenesis assay demonstrated Li+ role in angiogenesis with nearly twice the number of endothelial tubules formed after 8 hours. Western blot analysis confirmed that lithium-containing 45S5 expressed β-catenin, a canonical pathway aiding in the accelerated activation of transcription factors. Ultimately, lithium doping of BGs was determined to provide therapeutic effects with an emphasis on accelerating angiogenic factors.
Above report indicated that Li-incorporated 45S5 BG could directly stimulate HUVEC behavior in vitro by activating the canonical Wnt/-catenin pathway,28 however, the detailed intracellular mechanisms involved in Li-mediated angiogenesis and its stimulatory effect on in vivo blood formation were studied later by Liu et al.325 They tried to delve deeper into the mechanisms behind lithium-mediated angiogenesis and investigated the pro-angiogenic potential of 3D printed Li-incorporated glass-ceramic scaffolds (based on the 45S5 composition) using an in vivo ectopic angiogenesis model. The angiogenic nature of lithium-containing BGs was evaluated through the specific monitoring of cell derived exosomal miR-130a.325 A comprehensive in vitro study was used to deeply understand the performance and genetic activation of a lithium doped bioactive glass ceramic. In vitro experimentation shows lithium provides increased activation of Wnt/β-catenin, AKT, and NF-κB signaling pathways. Li+ dissolution also was shown to increase expression of proangiogenic factors secreted from bone marrow stromal cells. When incorporated into 3D printed scaffolds, Li-containing BGs were shown to accelerate vascularization in bone regeneration for large-sized defect repair.
4.1.2 Sodium.
4.1.2.1 Biological role.
Sodium, the most abundant alkali ion in extracellular fluids, has a multitude of physiological roles at various system levels.326 It controls blood volume and pressure in the circulator system, osmotic gradient, electrical activity, and nutrient absorption on the cellular level, and mediates protein function on the subcellular.327
Sodium exerts a massive influence on osmotic pressure in the vascular system. As the most plentiful ionic species in blood plasma, tight regulation of sodium is critical for control of blood volume and blood pressure.326–328 For example, activation of the Renin-Angiotensin-Aldosterone-System (RAAS) can induce reabsorption of sodium in the nephrons of the kidney. As a result, the increased osmotic pressure in the nephron pulls water back in the vascular space, thereby decreasing urinary excretion and maintaining blood volume.329
On the cellular level, sodium aids the creation and maintenance of cellular membrane electrical potentials. Membrane potential arises from the Nernst potential that balances the diffusion force from the concentration gradient across the ion semi-impermeable phospholipid membrane.330 To generate such concentration gradients, active transport through the sodium–potassium pump is required. Utilizing adenosine triphosphate (ATP) as an energy source, the cell membrane protein will take 3 sodium ions from the intracellular space to the extracellular space, against the concentration graduation. Simultaneously, 2 potassium ions will be taken from the extracellular space into the intracellular space. The net exchange will yield a charge differential between the internal and external side of the cell membrane, thereby creating a membrane potential.330,331 An illustration of this process is provided in Fig. 7 visualizing the ion movement across the membrane. The membrane potential is a key aspect of neuron excitability and has been found to play a role in other biological phenomena such as fertilization, kidney function, cell growth, and wound healing processes.332,333
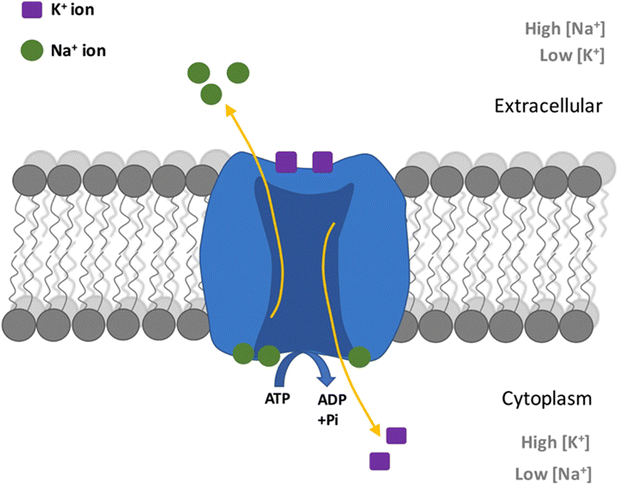 |
| Fig. 7 Diagram showing process in a Na+/K+ pump in neurons caused by action potential. From ref. 334 with permission from Springer, 2019. | |
Another function of sodium is in the nutrient absorption. Cotransport occurs when two separate species are required for passage through a cell membrane transporter. In the digestive tract, sodium is required for absorption of key nutrients such as glucose and amino acids.332,335 Furthermore, in cells throughout the body, sodium is required for not only nutrient uptake but also mineral transport. Sodium-dependent transport of chloride, calcium, and magnesium are all critical for maintaining homeostasis.335
Sodium forms a small, mobile ion that typically complexes with a coordination number of 6. The ion interactions with biomolecules have been shown to be critical for biological activity.296 For example, the protein thrombin was found to be most active in the presence of sodium chloride solutions when compared to lithium chloride, potassium chloride, and rubidium chloride.336 These findings imply a high level of specificity between the substrate protein and sodium ions. Although integrations of sodium ions and biomolecules exist and influence biology, it is important to note that such interactions are up to 4 orders of magnitude lower than those of divalent alkali earth ions.296,337
As illustrated above, sodium is essential for a large variety of physiological functions; however, too much sodium can be deleterious at the whole organism level. High amount of sodium in the blood (or hypernatremia) can lead to increase blood volumes causing high blood pressure. High blood pressure can cause heart failure or arterial damage in the heart, eyes, or kidney.338 Transiently, hypernatremia can cause intense thirst, lethargy, and decreases reflexes.339
4.1.2.2 BG evidence.
Sodium is a major component of many biomaterials formulations as well as most of experimental and commercial BG compositions. Sodium plays important roles in wound dressings such as sodium alginate and sodium carboxymethyl cellulose.340,341 However, the specific biological role of sodium in BG has not been studied extensively yet. 45S5 Bioglass® has been characterized comprehensively for its influence on cell proliferation and gene expression with no direct evaluation of the role of sodium ions.
Wallace et al. studied the biological response of BGs with varying sodium content to establish sodium role in gene expression and cell proliferation.45 The base BG composition used a general soda-lime phosphosilicate system (3SiO2–0.07P2O5–(3–x)CaO–xNa2O, by mol%) with constant network connectivity of 2.04 with varying Na2O content from 0–30 mol%. The glasses were pressed and sintered into disks at appropriate firing temperatures. Bioactivity testing showed that increased sodium content yielded rapid dissolution along with rapid pH increases. The result of the rapid pH increase inhibited cell proliferation as well as significantly lowered protein generation. Cell viability test and total protein assay suggested that the optimal concentration of sodium in the above-studied BG composition was roughly 7.5 mol% from a biological viewpoint. This study brought awareness to the potential cytotoxic effect of high sodium content and challenged the mainstream development of sodium-rich BG compositions, which were considered favorable from the viewpoints of processing (e.g., need for lower sintering temperature) and apatite-forming ability.
4.1.3 Potassium.
4.1.3.1 Biological role.
As a relatively small monovalent cation, potassium acts similarly to sodium; however there are distinct differences in their physiological roles. While sodium is maintained in high levels in the extracellular space, potassium is maintained in high levels in the intracellular space. The relative gradients help maintain neutral overall osmotic gradients between cells and extracellular fluid, thereby avoiding excessive influx and efflux of water into/from cells.332,337 These gradients are maintained by the ATP hydrolyzing sodium–potassium pump as mentioned previously. Potassium is also critical to electrochemical tissue properties and protein function.
One of the differences between the roles of sodium and potassium arises in their relative activity in excitability tissues. The resting membrane potential is created by the sodium–potassium pump, but specific ion channels can perturb that potential. When sodium channels open, sodium will flow down the concentration gradient and rush into cells causing the once negative resting potential to shoot up or depolarize, thus becoming slightly positive. This is the first step in an action potential, or the electrochemical manner in which neurons transmit signals. The next step is the closure of sodium channels and opening of potassium channels. Similar to sodium, the positively charged potassium ions will follow their concentration gradient; however, they will flow out of the cell causing the potential at the cell membrane interior to become negative once again or repolarize.333,342 Although being chemically similar in terms of relative mobility and charge, potassium and sodium have opposite effects on membrane potential. Similar activity can be seen in other excitable tissue such as cardiac muscle and pacemaker cells.343
Potassium ions can also form complexes with biological molecules just as sodium does. However, potassium ions are larger and thereby facilitate large coordination numbers spanning from 6 to 8.296 Due to these differences, potassium will interact with different substrates. For example, 70 kDa heat shock cognate protein is more active in solutions of potassium chloride when compared to sodium chloride.344
Excessive potassium can lead to hyperkalemia and impacting heart, skeletal muscle, and kidney function. High potassium content in the serum will disrupt normal cardiac electrical function leading to decreased cardiac output.345 In muscles, high extracellular potassium disrupts impulse conduction, thus leading to global muscle weakness.346 In the kidneys, high potassium content will lead to retention of hydronium ions, therefore lowering blood pH.347
4.1.3.2 BG evidence.
Several studies have been published investigating the replacement of Na2O with K2O in different BG systems.163,164 The main objective, as indicated in the preceding section, was to increase the glass stability of BGs by substituting Na with K and producing fiber. Mixed alkali effects have also been researched in order to improve mechanical properties and sinterability. However, there is a lack of systematic studies observing the biological impact of the incremental replacement of Na2O with K2O.
A mesoporous K-doped BG has been developed to determine the biomedical properties and drug delivery capabilities.142 This mesoporous BG was produced through sol–gel processing with the composition 49SiO2·20CaO·20Na2O·7K2O·4P2O5 (mol%). The sol–gel derived product showed an average particle size of 1 μm, an interconnected mesoporous network, and an average pore size of 21 nm. Due to the high surface area, the BG particles degraded rapidly in physiological fluid showing accelerated HAp formation. In vitro results demonstrated biocompatibility of the product with encouraging results from cell proliferation and cell cycle assays. As compared to 45S5 glass, the K-doped mesoporous BG showed increased osteoblastic activity by roughly 50% and slightly higher levels of cell proliferation.
Potassium-containing BGs have shown increased performance in dental applications due to the desensitization properties of K+.165 For example, Na2O was fully replaced with K2O, on a weight basis, in the BioMinF composition (36–40)SiO2–(28–30)CaO–(22–24)Na2O–(4–6)P2O5–(1.5–3)CaF2 to determine the improvement on dissolution rates, apatite forming ability, and the eventual suitability for dentin hypersensitivity treatment. Static dissolution studies showed nearly equivalent performance of both parent BioMinF and K-substituted BioMinF with similar dissolution rate, pH change, and precipitation of fluorapatite. Although both BG compositions have shown nearly identical performance for in vitro bioactivity, the K-containing BG was selected for future dentin sensitivity treatments. Several further studies have been published showing that potassium-based compositions provide therapeutic effects through blocking nerves in dentin tubules while also aiding in dental restoration.165,348
4.1.4 Rubidium.
4.1.4.1 Biological activity.
Rubidium has no known natural biological role. The properties of rubidium are similar to those of potassium and, as a result, both of them behave similarly in cells. Radioactive rubidium has been utilized as a potassium ion tracer in biological research.349 Similarly, radioactive rubidium has been used as a diagnostic contrast agent in positron emission tomography.349,350 High metabolic activity and, therefore, increased expression of sodium–potassium pumps cause the preferential uptake of the radioactive rubidium in cancers cell over normal tissues.331,350 Therapeutically, rubidium has been investigated in the fields of neuropsychiatric diseases as well as osteogenesis. Rubidium has been studied in preclinical and clinical trials as a possible treatment for depression.351–353 To date, no consensus has been achieved about the mechanism for anti-depressive effects. As for osteogenesis, rubidium chloride was shown to activate osteoblast activity and inhibit osteoclast activity. Specifically, rubidium ions target Jnk/p-38-mediated NF-κB activation.354 NF-κB encompasses a family of transcription factors. In the case of bone, it acts as key mediator in osteoclastogenesis, a critical part of healthy bone formation.355 Rubidium levels tend to collect in the body due to the elements long half-life of over 50 days.356
4.1.4.2 BG evidence.
Although rubidium is not abundant biologically, it has been applied for use in several biomaterial platforms. Rubidium has shown antibacterial properties and modulatory cell responses when used in biomaterials such as hydrogels, implant coatings, and other ceramic materials.357,358 When used as a dopant in BGs, rubidium shows biocompatibility through in vitro cytotoxicity screenings, as well as promising results from cell adhesion testing.155,359
Rubidium has been successfully added to bioactive glass microspheres for transient embolic medical devices.360 Glass composition BRS2 (70B2O3–28Rb2O–2SrO, by mol%) has been reported to be degradable and radiopaque making them suitable for transarterial embolization. The borate based composition shows degradation times of around 24 hours without circulating to unwanted locations in the body such as the brain or lung. Thus, these BGs may be suitable for cancer treatment through blocking blood flow to tumors or other abnormal areas.
4.1.5 Cesium.
Cesium, lying below rubidium in the periodic table, is fairly unremarkable in terms of biomedical applications. Radioactive cesium has been utilized in brachytherapy for treatment of cancers.361,362 Furthermore, cesium chloride was explored in high pH cancer therapy.363,364 However, the mechanism and efficacy have yet to be validated and cesium is associated with a variety of toxicities such as cardiac arrhythmia.363,365,366 No examples of cesium-containing BGs have been reported so far in the literature.
4.1.6 Francium.
Francium is the largest alkali element. Due to its nuclear instability, it has no known biological function. This highly radioactive element has no biomedical applications. No examples of francium-containing BGs have been reported so far in the literature.
4.2 Alkaline earth elements
4.2.1 Beryllium.
Beryllium, the smallest of the alkali earth elements, has no known biological role. Aside from device hardware, beryllium has no biomedical applications either. Occupational exposure to beryllium has been associated with a variety of maladies including allergic reactions as well as intestinal and lung disease.367
4.2.2 Magnesium.
4.2.2.1 Biological role.
Magnesium, the second smallest alkali earth ion, has a divalent ionic state with high charge density.368 The small divalent ion forms strong ligand complexes with typical coordination numbers of 2 or 4 in solution.296,369 As a result, magnesium can interact strongly with both nucleotides and proteins and influence a plethora of biological processes.370
The majority of intracellular magnesium is complexed with nucleotide triphosphates, chiefly ATP. Positively charged magnesium interacts strongly with negatively charged phosphate groups.371 This complex is critical for facilitating the hydrolysis of ATP to adenosine diphosphate (ADP). The energy released in this reaction is essential to many biological functions including muscle contraction, cellular signaling, active transport, and nucleotide synthesis among many others.372
Nuclei acids such as deoxyribose nuclei acid (DNA) and ribose nucleic acid (RNA) require magnesium cations to confer stability. Similar to ATP, negatively charged phosphate groups attract magnesium ions. In aqueous solutions, a magnesium ion maintains a shell of water molecules around it. As magnesium and its associated water shell approach nuclei acids, the water molecules will form hydrogen bonds. The hydrogen bonds reduce charge density on the DNA or RNA and stabilize optimal configurations.373 It is also possible for magnesium to directly interact with nuclei acids. For example, Mg can from a complex ligand bond with DNA and, instead of stabilizing DNA, it will cause a tight distortion and render the macromolecule unusable.374 Therefore, a balance of magnesium is critical for DNA and RNA function.
Many enzymes utilize magnesium as either a cofactor or an activator. For example, many enzymes involved in glucose metabolism require magnesium to catalyze reactions that leverage the transfer of high energy inorganic phosphate. For example, hexokinase catalyzes the first step of glycolysis by extracting a phosphate from ATP and attaching it onto a molecule of glucose.315,369,375 Similarly, key cell signaling enzymes, such as the insulin receptor, require magnesium to transduce endocrine signals into cellular responses. There are over 800 known enzymes that somehow use magnesium in the reactions where they are involved.368
The bulk of magnesium in the body can be found in bone. Magnesium can coat HAp crystals in the matrix of cortical bone as well as be doped into mineral component of bone. Magnesium ions control the solubility of calcium and potassium, therefore greatly influencing the size of HAp crystals and, as a result, their stability.368 Magnesium deficiency has been associated with osteoporosis and increased bone fractures due to large HAp crystals decreasing overall stiffness and strength of bone.376 On a cellular level, it was found that control of magnesium levels in bone tissue is critical for proper enzyme activity and protein expression associated with osteogenesis.377
Apart from the role in hard tissues, magnesium and calcium interactions influence cardiac physiology, too.368,370,375 Calcium plays a critical role in the cardiac action potential (details in section 4.2.1). However, magnesium can block L-type calcium through either direct inhibition of ion permeability or perturbation of voltage gate properties. Magnesium functions in a protective manner regulating myocardial contractions.378 Clinically, magnesium has been proven to be life-saving for the deadly cardiac arrhythmia Torsade's De Pointes.379 However, the mechanism has yet to be definitively determined.
Transmission of glutamate, an excitatory neurotransmitter, through N-methyl-D-aspartate (NMDA) receptor is regulated by magnesium ions.370 At normal resting membrane potential, Mg ions will block glutamate bound ion channels on the postsynaptic neuron. When the membrane potential rises, magnesium will release from NMDA receptors allowing for an influx of calcium and transmission of the neural signal.380 During states of hypomagnesemia, these neural pathways will be unregulated and hyperexcitability ensues.381
Excessively high levels of magnesium, though rare outside the context of kidney failure and excessive intake, can be deadly. Moderately high amounts of magnesium will yield an altered mental state, decreased reflexes, constipation, and headache.382 However, excessively high serum magnesium can lead to decreased breathing, high blood pressure, muscle paralysis, cardiac arrhythmia, and even coma.382,383
4.2.2.2 BG evidence.
Magnesium is perhaps the most commonly used element in BGs outside of those used in the original 45S5 composition (Si, Ca, Na, P). The incorporation of magnesium into BGs has been studied extensively for structural, processing, and biological impacts.166 As magnesium is among the most abundant metals in the body, it is natural to consider it in biomaterial applications. Magnesium has been shown to not only tailor the degradation rates of BGs, but also stimulate increased cell proliferation and antibacterial activity. Further discussion of magnesium in BGs is reported by Cacciotti.227
Moghanian et al. studied the in vitro effects of incremental calcium replacement with MgO on cell proliferation and antibacterial activity.384 The 58S compositional system was implemented using the formula 60SiO2–4P2O5–(36–x)CaO–xMgO (where x = 0, 1, 3, 5, 8, 10) on a molar basis. All glasses were synthesized using the sol–gel process. The results of the cell proliferation study revealed that 5 mol% MgO had the highest stimulation of cell proliferation. Concentrations over 5 mol% showed decreasing returns, which was thought to be due to higher pH values of the dissolution product solution. Mg-containing BGs were then tested for antibacterial properties against methicillin-resistant Staphylococcus aureus (MRSA) bacteria. Again, 58S glass containing 5 mol% MgO showed the most promising results with a bactericidal percentage of nearly 50% in just 24 hours.
A glass using the composition 45SiO2–3P2O5–26CaO–15Na2O–7MgO–4K2O mol% was then studied in vivo for osteogenic potential.385 This melt-derived glass was delivered in the form of foam-like 3D scaffolds. 20 Wistar rats received scaffolds in induced 6 mm cranial defects and were monitored for up to 12 weeks. Rats from each sample group were sacrificed at 4 and 12 weeks post-implantation for histology and immunohistochemistry evaluations. Compared to a standardized reference, the Mg-doped BG clearly demonstrated increased levels of bone regeneration after both time periods. Immunohistochemistry also demonstrated the ability of Mg-doped BGs to express osteocalcin and osteonectin after 12 weeks. This study concluded that magnesium is suitable and recommended for use in BGs applied to hard tissue regeneration.
Magnesium-containing bioactive glass-ceramics have been reviewed comprehensively for attractive biomedical applications.167 Several crystalline phases containing magnesium were listed including diopside, fluorophlogopite, potassium fluorrichterite, akermanite, merwinite, monticellite, whitlockite, and enstatite. Magnesium was also discussed to be used as a stabilization agent to control crystallization of high-calcium-content phases. Magnesium content in the discussed glass ceramic families were shown to negatively impact the apatite-forming ability. However, the in vitro and in vivo studies included in the review showed high biocompatibility with cells and animal models. Furthermore, good osteointegration of magnesium-doped implants was shown with rabbit models.
The combinatorial effects of Si4+, Ca2+ and Mg2+ ions released from BGs were studied for their effects on osteoblast osteocalcin expression and biomineralization.249 Silicate and calcium ions were found to up-regulate osteocalcin expression while magnesium was found to down-regulate it. Osteocalcin is discussed to be critical in early stage osteogenesis suggesting that magnesium may not be ideal for applications requiring fast results. However, delayed release of magnesium ions can be used to develop materials with controlled bone formation rates. Magnesium is also recognized to aid in the remodeling process which will strengthen the newly formed bone.
Finally, magnesium has been employed as a dopant in BG nanofiber membranes created via electrospinning.386 Anti-bacterial, anti-inflammatory, angiogenic, and wound healing capabilities of these fibers were evaluated. In vitro assessment of cytocompatibility showed strong cell proliferation and angiogenesis. Furthermore, cellular response from murine macrophages showed decreased cellular inflammation as a response to the dissolution products. Proliferation of both Gram-positive and Gram-negative bacterial strains were diminished by the BG fibers. Lastly, gene expression assays showed down-regulated pro-inflammatory factors, up-regulated anti-inflammatory factors, and up-regulated angiogenic factors. Overall, magnesium doped silicate based electrospun fibers show promising properties in the treatment of infected wounds as a wound dressing material.
4.2.3 Calcium.
4.2.3.1 Biological role.
Calcium is key to many biological processes. Unlike other electrolytes, free calcium ion concentrations are over 4 orders of magnitude higher in extracellular fluids than in the intracellular environment.387 The high level of control is critical to calcium cell signaling, cofactor action, neuronal and cardiac properties. Calcium not only functions in physiology but plays a structural role as a key element in bone.
Secondary messengers are molecules in cells than can disseminate a single extracellular signal throughout a cell.388 For example, an extracellular ligand can bind G-protein coupled receptors triggering the activation of intracellular g-proteins. The g-protein can activate a variety of targets such as phospholipase C. From the cell membrane, phospholipase C can trigger the release of 1,4,5-trisphosphate (IP3) into the cytoplasm. At this point, soluble IP3 can bind to IP3 receptors on the endoplasmic reticulum, leading to the release of many calcium ions into the cytoplasm ultimately spreading the initial signal throughout the entire cell.389 The calcium-dependent signal transduction is crucial to a myriad of processes including fertilization, proliferation, metabolism, and glandular secretion.389–391 A specialized version of calcium signaling exists for muscle contractions. As motor neurons excite muscle tissues, changes in membrane potential will trigger the intracellular sarcoplasmic reticulum to release calcium ions. As calcium ions bind troponin C on muscle fibers, a confirmational change occurs activating muscle fibers for contraction.392
Similar to magnesium, calcium plays the role of cofactor in many enzymatic activities. The small, highly charged ion interacts with negatively charged groups on both substrates and enzymes. Calcium cofactor abilities are strongly exemplified in the blood coagulation cascade. Triggered by a blood vessel injury, a series of serine protease are activated to ultimately produce a fibrin clot to cease bleeding.393,394 Calcium is required by factors XI, IX, X, VII, and II as well as tissue factor. Specifically, calcium functions as a cofactor for serine protease activity. Serine proteases facilitate the activation of clotting factors and therefore calcium is a strict requirement for the clotting cascade.395Fig. 8 provides an illustration of the coagulation process emphasizing each factor. Transglutaminases represent another class of enzymes requiring calcium396 for catalyzing the formation of a covalent bond between glutamine side groups and primary amines, transglutaminases are critical for formation of crosslinked structures that offer protection and stability for tissues including skin, nerves, lung, and bone.396–399
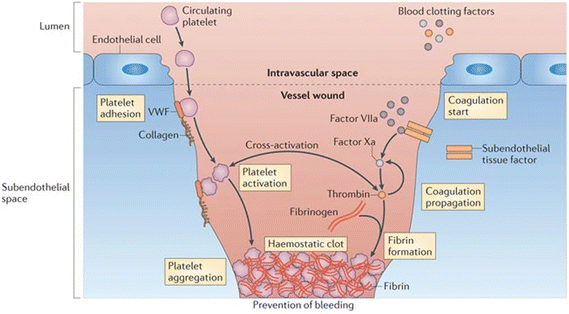 |
| Fig. 8 Visualization of hemostasis showing all processes involved in clot formation. From ref. 400 with permission from Springer Nature, 2012. | |
Calcium dynamics are essential to functioning to a variety of electrically excitable tissues. Cardiac pacemaker cells, such as those in the sinoatrial node of the heart, control the rate and rhythm of human heart beats. Hyperpolarization-activated cyclic nucleotide–gated (HCN) calcium channels allow for a slow, controlled depolarization of pacemaker cells. Once a threshold membrane potential is reached, L-type calcium channels open, leading to full depolarization and initiation of a heart beat signal.401 These calcium channels are common therapeutic targets for arrhythmia.402 Furthermore, calcium is responsible for the plateau phenomena seen in cardiomyocytes action potentials.403 This is key in facilitating complete contraction of the heart muscle.
In neurons, calcium is essential in signal transmission across synapses. As an electrochemical signal reaches the terminal aspect of a nerve, voltage gated calcium channels open. The rapid influx of calcium triggers the release of neurotransmitter into the synaptic cleft. Neurotransmitters then diffuse across the cleft and interact with the postsynaptic neuron to transmit the signal.404
Calcium dynamics are essential to functioning to a variety of electrically excitable tissues. Cardiac pacemaker cells, such as those in the sinoatrial node of the heart, control the rate and rhythm of human heart beats. Hyperpolarization-activated cyclic nucleotide-gated (HCN) calcium channels allow for a slow, controlled depolarization of pacemaker cells. Once a threshold membrane potential is reached, L-type calcium channels open, leading to full depolarization and initiation of a heart beat signal.401 These calcium channels are common therapeutic targets for arrhythmia.402 Furthermore, calcium is responsible for the plateau phenomena seen in cardiomyocytes action potentials.403 This is key in facilitating complete contraction of the heart muscle.
In neurons, calcium is essential in signal transmission across synapses. As an electrochemical signal reaches the terminal aspect of a nerve, voltage gated calcium channels open. The rapid influx of calcium triggers the release of neurotransmitter into the synaptic cleft. Neurotransmitters then diffuse across the cleft and interact with the postsynaptic neuron to transmit the signal.404
Calcium is a vital element playing various roles in biochemical/biological reactions but is also dramatically important in maintaining the structure of the skeletal system. In bone, calcium is nearly entirely present in calcium phosphate mineral phases. Hydroxyapatite is the major mineral phase of bone which provides strength for the skeletal system while also acting as a metabolic reservoir for cellular fluids.405 The skeletal system is dominated by this crystalline inorganic phase in cortical bone, showing 80%–90% calcification, which provides the majority of structural function.406 Trabecular bone provides metabolic roles with 15%–25% calcification.
4.2.3.2 BG evidence.
Calcium has been implemented in nearly every current BG composition due to its well-known structural role in both glass science and physiology. It has been used as a baseline in most studies replacing it incrementally with other modifiers to determine the biological implications. A study published in 2020 reverses the stereotypical approach and investigates the biological impacts of calcium content on a binary CaO–SiO2 system with CaO concentration up to 15%.407 Glasses were produced through a modified Stöber sol–gel process with spherical morphology and a monodispersed sized. In vitro bioactivity and in vitro cytotoxicity were used to evaluate the impact of calcium concentration. The effects of calcium content on bioactivity were relatively small over the investigated compositional range, suggesting that dissolution rates were not compositionally dependent at this range. Rate of HAp formation was directly related to the calcium content of the glass. Increased calcium content did not show any in vitro cytotoxicity.
Another study by Catauro et al. investigated the effect of a SiO2–CaO–P2O5 system on cell proliferation.408 A wider compositional range of BGs with molar Ca/P ratios of 3, 6, 9, 12 was prepared through sol–gel synthesis. In vitro testing included cell attachment, cell morphology observation, and cell proliferation. Increasing calcium concentration was found to have the largest effect on cell proliferation. Calcium content increased the biological performance of the compositional workspace suggesting that different ratios of Ca/P can be applied to multiple clinical applications.
Calcium has proven paramount in formation of new blood vessels through production of angiogenic growth factors. Simulation of the up-regulation and production of critical growth factors like vascularization growth factor (VEGF) or basic fibroblast growth factor (bFGF) among others.409,410 Several in vitro and in vivo trials illustrate the role of calcium and bioactive glasses as whole in stimulating angiogenesis in different tissue engineering environments.23 These effects are essential wound healing applications in order to accelerate the tissue healing process.
4.2.4 Strontium.
4.2.4.1 Biological role.
Strontium exhibits similar properties to calcium in biological systems. As a result, it is readily absorbed and has found clinical relevance in bone health. Strontium has also been investigated for their roles in dental health, radiotherapy, and sensory irritation suppression.411–414
Calcium sensing receptor (CaSR) can be found on the parathyroid gland function in calcium homeostasis as well as the renal tubules controlling urine calcium level.415 Due to chemical similarities, strontium ions can bind to the ligand binding domain of CaSR. Furthermore, CaSR is found on osteoblasts and osteoclasts, and thus allows strontium to influence bone metabolism.416 Specifically, strontium activates osteoblast activity and inhibits osteoclasts. Through NFATc1/Wnt, Runx2, and Cox-2 signaling strontium enhances the differentiations of preosteoblasts into mature osteoblasts.417,418 Furthermore, strontium activates ERK1/2, p38, and AKT to enhance bone formation.419,420 As for osteoclasts, strontium will block cellular maturations and induce apoptosis.421 Strontium has been investigated in the treatment for osteoporosis. Strontium ranelate has been studied in several clinical trials showing the ability to both limit fractures and increase bone mass.422–425
Similar to bone, strontium can play a protective effect on teeth. The presence of strontium ions in an acidic solution reduces enamel erosion. It is proposed that strontium forms a protective layer around the enamel.426 Additionally, strontium in drinking water and toothpaste has been shown to both stave dental caries as well as mineralize bone tissue.427 Interestingly, strontium has an additional benefit of treating dentin hypersensitivity.428
Radioactive strontium-89 has been leveraged for local radiotherapy. Due to chemical similarities to calcium, this therapeutic ion is preferentially taken up by malignancy in bones. Clinically, strontium-89 has been successful in treating painful bony metastasis for a variety of cancers.429–431
Strontium biological influence expands into sensory applications as well. Zhai et al. illustrated in humans that topical strontium nitrate can lower both the magnitude and duration of a histamine induced itching sensation.432 Several similar studies have corroborated these results.433–435 This effect is likely due to strontium blocking of calcium channels in sensory neurons.436
4.2.4.2 BG evidence.
The effects of strontium in hard tissue applications has been previously reviewed extensively.170 The biological impacts of strontium have been studied outside of BG field in biomaterial platforms such as strontium chloride solutions, strontium ranelate, and in other implantable bioceramics such as calcium orthophosphates.169,171,177 Strontium has been studied as a dopant in BGs in many different forms including powders, granules, fibers, scaffolds, and pastes while also being added to all families of BGs. Primary in vitro studies have shown that strontium improves osteogenesis induction through various mechanisms which include upregulation of critical genes, activation of signaling pathways, and regulating differentiation of stem cells.172–176,178 Moreover, strontium inhibits osteoclastogenic action as shown by Gentleman et al.179 Finally, strontium shows antibacterial properties through mechanisms that are not well understood yet. Dissolution products of strontium-containing BGs have been shown effective against certain strains of bacteria including Escherichia Coli (E. coli) and Porphyromonas gingivalis (P, gingivalis).180,437
Bone regeneration of strontium-containing BGs was studied in vivo by Esfahanizadeh et al.181 Critical size calvarial defects in 12 New Zealand White rabbits were treated with 45S5, magnesium-doped 45S5 (Mg-45S5), and strontium-doped 45S5 (Sr-45S5) glasses. The rabbits were allowed 4 and 8 weeks for tissue regeneration with half the populations being sacrificed at 4 weeks. Both Mg-45S5 and Sr-45S5 glasses showed increased new bone regeneration as compared to undoped 45S5 group. However, after 8 weeks the Sr-45S5 group showed significantly higher rates of new bone regeneration as compared to the other test groups.
4.2.5 Barium.
4.2.5.1 Biological role.
Barium has no known natural biological role; however, it finds great use as an X-ray contrast agent. This high-atomic-number alkali earth ion can absorb X-rays much better than human tissues. Administered orally or transrectally, barium sulfate can be used to probe maladies of the upper and lower digestive tract, respectively. Due to toxicity, no intravenous applications of barium exist.438
4.2.5.6 BG evidence.
Barium has been incorporated in BGs showing several therapeutic effects including antibacterial and anti-inflammatory properties. The radiopaque performance of barium has been well studied for applications involving medical imaging as it allows for easy differentiation between the implant and host tissue. Experimental evaluation on the effects of barium in vitro have included cell proliferation studies, hemolysis, and genetic stimulation assays to screen the Ba effects before continuing to in vivo studies.
Majumdar et al. comprehensively probed the biological effects of barium on the 45S5 system. 1.35 mol% BaO replaced Na2O and SiO2 and was produced through sol–gel synthesis.156 This composition was compared against a sol–gel derived 45S5 product. Both glasses showed comparable particle size and specific surface area through dynamic light scattering and nitrogen adsorption BET. A hemolysis assay proved that the barium-containing glass was biocompatible without disrupting red blood cells and causing hemoglobin to leave the cells. Comparable results were generated from a cell proliferation study showing that dissolution products from both glasses increased cell count from the original count. Wound healing assays again showed comparable results to 45S5 with the 62.36 ± 4.73% closure after 24 hours for the barium-containing glass. Increased performance of the barium-containing BG was seen in the genetic upregulation of anti-inflammation genes. As compared to 45S5, barium-containing BG had an increase in anti-inflammatory marker IL-10 of 19.91 pg mL−1. Inflammatory markers were decreased by the barium containing BG as compared to 45S5 for marker IL-6 by 55.87 pg mL−1 and for TNF-α they were decreased by 39.98 pg mL−1.
The same research group recently published an in vivo toxicological evaluation of barium-doped 45S5 BG in adult albino Wistar rats.182Fig. 9 highlights the experimental procedures and toxicological assays used in this study. Acute oral toxicity, subacute oral toxicity, organ coefficients, histological, and biochemical indices were used to critically evaluate the role of barium ions in bone and soft tissues. Oral doses of 300 and 2000 mg kg−1 of both barium-containing BG and Ba-free 45S5 were administered with no treatment-related mortality observed. Organ coefficient studies showed no statistical changes in organ coefficient of the brain, heart, kidney, lungs, liver, or spleen for both BG compositions. Furthermore, no changes in muscle coordination, spontaneous locomotion, or observed anxiety was shown in any of the treated rats. The study concluded that barium was safe and therapeutic in its use in BGs.
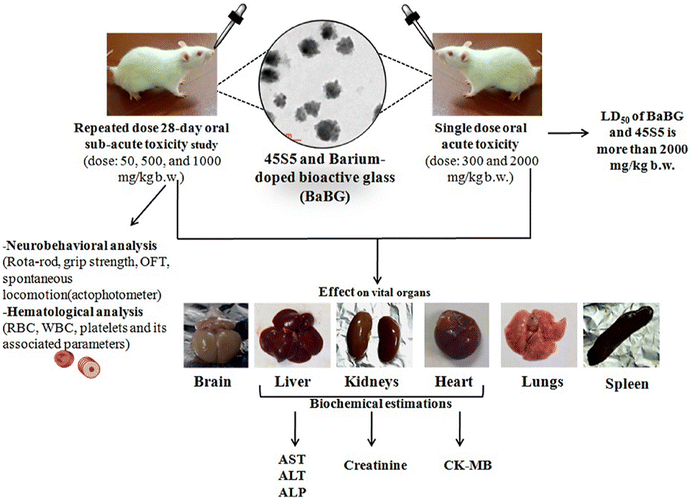 |
| Fig. 9 Illustration showing the procedures and assays used by Majumdar and Krishnamurthy. Wistar rats received single or repeated dosage of 45S5 or barium doped 45S5 to comprehensively determine acute and chronic toxicity in several organs. Two characterization techniques were used to analyze the brain, liver, kidneys, heart, lungs, and spleen of sacrificed rats. No organ toxicity was found in male or female rats. From ref. 182 with permission from Elsevier, 2022. | |
An in vivo study published in 2018 showed the potential for barium-containing BGs in the application of gastro-duodenal ulcers.183 1.3 mol% BaO was added to the 45S5 Bioglass® system and synthesized via melt quenching. The particle size of 45S5 and barium-containing BG were comparable at around 2 μm. BGs suspended in a 0.5% carboxy-methyl-cellulose mixture were administered orally to the rats. Several types of ulcers were studied including ethanol-induced, aspirin-induced, pylorus litigation-induced, acetic acid-induced, and cysteamine-induced. Dissolution products from barium-containing BGs aided in neutralizing gastric acid showing normal architecture, less hemorrhages, and less necrosis in several ulcer models. The barium-containing glasses also showed the creation of a physical protective barrier providing antiulcer effects.
4.2.6 Radium.
Radium, the heaviest of the alkali earth metals, has no known biological function and is severely limited in applications due to its radioactive nature. Similar to strontinum-89, radium-223 in its chloride salt form has been approved for radiation therapy in bony metastatic cancers.439
5. Conclusion and guidance for compositional design
ALAEs play a vital, multifunctional role in bioactive glasses that affect the glass structure, processing, and physiological impact. By modifying the glass structure, phase precipitation and dissolution rates can be tailored to a specific medical application. ALAEs play a direct role in certain processing methods that can enhance medical device performance in vitro and in vivo. Due to the complexity of the structure-property relationships and biological responses of bioactive glasses, many questions are raised on the topic of compositional design. Below, several questions regarding the design of bioactive glass compositions are raised and discussed thoroughly.
What is the objective of designing bioactive glasses in modern medicine surpassing the traditional notion of bone bonding ability?
• First, the desired application needs to be considered. Soft tissue or bone tissue engineering, antibacterial deficiency (e.g., osteomyelitis treatment), preventive dentistry, cancer treatment, etc. all need the proper adjustment of dissolution. Hard tissue applications, such as bone regeneration, do not require rapid dissolution rates. Rather, bone bonding ability, HAp formation, and some therapeutic effects are more important. For soft tissue wound healing, delivery therapeutic ions and production of growth factors are needed quickly to initiate the wound healing process. Complete dissolution is also required in order to ensure that no immune response or negative effects occur in the long-term timeframe.
What structural features govern dissolution rates and how ALAEs help?
• Network connectivity of the glass structure directly affects the dissolution of the BG composition. For example, the creation of NBOs related to introduction of NWMs improves the degradation of silicate-based glasses. On the contrary, for borate glasses, the addition of NWM creates a unique anomaly. The inclusion of the ALAEs shifts the structural units from the three coordinate BO3 to the BO4 unit. This enhances the connectivity of the glass and therefore increases its durability. The structural units of the glass need to be considered intently when designing a BG composition.
Can we control congruent vs. incongruent dissolution through compositional design and ALAEs addition?
• To start, congruent dissolution refers to a material that dissolves completely into the constituent ions rather than leaving a residual solid behind. This is vital when considering applications that require the BG particle to be removed before the healing process is complete. Congruent dissolution can be designed as a function of the composition. Certain compounds are less likely to dissolve in solution as they strengthen the glass network and durability. Potential examples of these compounds include Al2O3, ZrO2, and TiO2.
How can we optimize liquidus temperature through composition?
• Minimizing the liquidus temperature allows for optimization of processing costs as well as glass forming ability. Optimizing the ratio of ALAEs with NWFs can lower overall melting temperature while still maintaining the desired level of network connectivity. Designing compositions with lower processing temperatures will require much less energy to manufacture, driving costs down. Also, by minimizing the temperature difference between the melt temp and solid minimizes tendency to crystallize. Designer glasses around thermodynamic eutectics create lower temperature compositions with higher glass forming ability.
What role do ALAEs in the thermodynamics and kinetics of crystallization? Can they be used to influence or prevent crystallization?
• The thermodynamics of crystallization can be brought back to the fundamental thermodynamics of mixing. If Gibbs free energy of mixing is positive, the system will become separated. The temperature at which nucleation occurs is usually found somewhere between the glass transition temperature and the crystallization peak temperature, which can be determined through differential scanning calorimetry. By adding ALAEs to the glass network, these temperatures decrease, and sometimes the temperature difference between them does as well. The atomic radius also plays an important role here. As previously mentioned, larger ALAEs will create a denser ring structure. The kinetics of crystallization is heavily dependent on the viscosity of the glass composition. ALAEs play an important role in lowering the viscosity of the melt. In lower viscosity melts, it is easier for atoms to diffuse across the liquidus-nucleus barrier which grows the crystallized phase.
How do BGs containing ALAEs provide therapeutic effects instead of harmful reactions?
• BGs excel for local therapy such that high levels of therapeutic species are only experienced at the site of administration, while whole body levels remain below toxic limits. For example, lithium is known to be toxic in humans at serum levels in excess of 1.5 mM.440 However, in vitro studies suggest lithium levels in excess of 5 mM may be required for induction of desired therapeutic response.308 This precludes systemic administration from clinical relevance. The key with BGs is twofold: local delivery and controlled dissolution. Implanted lithium doped BG biomaterials have been utilized for the growth of soft and hard tissues alike, yet evidence of lithium induced toxicity was not noted in in vivo studies.323–325 This is due to the fact that high lithium levels are restricted to the area directly surrounding the implant. Lithium is slowly released from the implant and will initially diffuse through the local environment mediating its therapeutic action. The stray ions that do diffuse out of the treated area and into the blood stream are heavily diluted and therefore induce no adverse effects. The key is that the amount of lithium is sufficient for local treatment but not large enough that escaping ions induce systemic toxicity. This idea can be generalized to all ALAEs.
• Furthermore, the incorporation of ALAEs in bioactive glass compositions to elicit specific therapeutic effect could carry an additional advantage. The existing literature has demonstrated that many elements from the transition metal class can elicit potent therapeutic responses (e.g. pro-angiogenic effect by copper and cobalt, antibacterial properties by silver) but, on the other hand, they are also known to induce important toxic effects in the body at relatively low dosages. In order to overcome this drawback, using “safer” ALAEs at proper concentration can be considered as an alternative to transition metal incorporation in the attempt to achieve the same beneficial effect while avoiding toxic side effects.
Can machine learning/artificial intelligence be used to further the advancement of bioactive glasses especially more rational addition of ALAEs?
• Recently, there has been an effort to “decode the glass genome” in order to accelerate our understanding of the structure–property relationships in glass. With an emphasis on modeling, vast datasets can be generated on properties such as dissolution behavior, fracture toughness, viscosity, and glass forming ability, among many others. However, computation techniques cannot be relied upon for modeling the biological response between a biomaterial and a cellular interface. Combining the datasets created through modeling techniques and experimental tests can yield powerful information for designing the optimized BG composition. Machine learning has been employed to predict the properties of biomaterials (including glasses). Developing larger datasets will in turn generate more accurate results from machine learning models allowing for the creation of the next generation of bioactive glasses.
How does the introduction of lesser studied ALAE elements or even other therapeutic inorganic ions effect the commercialization process of novel bioactive glass compositions?
• Novel bioactive glass compositions, morphologies, and products have been approved for commercial use by different international regulatory agencies through different methods. In the United States, the 510k process allows an application to forego comprehensive clinical trials is deemed sufficiently similar to a product that is existent on the market.441 This allows similar compositions to the original 45S5 Bioglass® composition to be passed without the scrutiny of the many studies needed in clinical trials. This “sufficiently similar” rhetoric has been used for commonly studied compositions such as 45S5 or 53SP4. Compositions studied in academia containing more exotic elements that have not been introduced in the clinic may receive more curiosity and hesitation for regulatory agencies forcing a more demanding road to approval.
Conflicts of interest
There are no conflicts to declare.
References
- L. L. Hench, The story of Bioglass®, J. Mater. Sci.: Mater. Med., 2006, 17(11), 967–978 CrossRef CAS PubMed.
- A. Shearer, M. Montazerian and J. C. Mauro, Modern definition of bioactive glasses and glass-ceramics, J. Non-Cryst. Solids, 2023, 608, 122228 CrossRef CAS.
- X. Liu, M. N. Rahaman and D. E. Day,
In Vitro Degradation and Conversion of Melt-Derived Microfibrous Borate (13–93B3) Bioactive Glass Doped with Metal Ions, J. Am. Ceram. Soc., 2014, 97(11), 3501–3509 CrossRef CAS.
- X. Liu, M. N. Rahaman and D. E. Day, Conversion of melt-derived microfibrous borate (13–93B3) and silicate (45S5) bioactive glass in a simulated body fluid, J. Mater. Sci.: Mater. Med., 2013, 24, 583–595 CrossRef CAS PubMed.
- D. S. Brauer, Bioactive Glasses—Structure and Properties, Angew. Chem., Int. Ed., 2015, 54(14), 4160–4181 CrossRef CAS PubMed.
- M. Karlsson, M. Schuch, R. Christensen, P. Maass, S. W. Martin and S. Imberti,
et al., Structural Origin of the Mixed Glass Former Effect in Sodium Borophosphate Glasses Investigated with Neutron Diffraction and Reverse Monte Carlo Modeling, J. Phys. Chem. C, 2015, 119(49), 27275–27284 CrossRef CAS.
- M. Storek, R. Böhmer, S. W. Martin, D. Larink and H. Eckert, NMR and conductivity studies of the mixed glass former effect in lithium borophosphate glasses, J. Chem. Phys., 2012, 137(12), 124507 CrossRef PubMed.
- D. Bellucci, V. Cannillo and A. Sola, An overview of the effects of thermal processing on bioactive glasses, Sci. Sinter., 2010, 42(3), 307–320 CrossRef CAS.
- M. Montazerian and E. Dutra Zanotto, History and trends of bioactive glass-ceramics, J. Biomed. Mater. Res., Part A, 2016, 104(5), 1231–1249 CrossRef CAS PubMed.
- D. Bellucci, A. Sola and V. Cannillo, Hydroxyapatite and tricalcium phosphate composites with bioactive glass as second phase: State of the art and current applications, J. Biomed. Mater. Res., Part A, 2016, 104(4), 1030–1056 CrossRef CAS PubMed.
- L. Lefebvre, L. Gremillard, J. Chevalier, R. Zenati and D. Bernache-Assolant, Sintering behaviour of 45S5 bioactive glass, Acta Biomater., 2008, 4(6), 1894–1903 CrossRef CAS PubMed.
- M. Montazerian, A. Shearer and J. C. Mauro, Perspectives on the impact of crystallization in bioactive glasses and glass-ceramics, Int. J. Ceram. Eng. Sci., 2024, e10194 CrossRef CAS.
- A. Moeini, T. Hassanzadeh Chinijani, A. Malek Khachatourian, M. Vinicius Lia Fook, F. Baino and M. Montazerian, A critical review of bioactive glasses and glass–ceramics in cancer therapy, "Int. J. Appl. Glass Sci., 2023, 14(1), 69–87 CrossRef CAS.
- M. Montazerian, F. Baino, E. Fiume, C. Migneco, A. Alaghmandfard and O. Sedighi,
et al., Glass-ceramics in dentistry: Fundamentals, technologies, experimental techniques, applications, and open issues, Prog. Mater. Sci., 2023, 132, 101023 CrossRef CAS.
- B. C. Palivela, S. D. Bandari and R. S. Mamilla, Extrusion-based 3D printing of bioactive glass scaffolds-process parameters and mechanical properties: A review, Bioprinting, 2022, 27, e00219 CrossRef.
- R. Gmeiner, U. Deisinger, J. Schonherr, B. Lechner, R. Detsch, A. R. Boccaccini and J. Stampfl, Additive Manufacturing of Bioactive Glasses and Silicate Bioceramics, J. Ceram. Sci. Technol., 2015, 6(02), 75–86 Search PubMed.
- A. Sola, D. Bellucci, V. Cannillo and A. Cattini, Bioactive glass coatings: a review, Surf. Eng., 2011, 27(8), 560–572 CrossRef CAS.
- R. Sergi, D. Bellucci and V. Cannillo, A Comprehensive Review of Bioactive Glass Coatings: State of the Art, Challenges and Future Perspectives, Coatings, 2020, 10(8), 757 CrossRef CAS.
- L. L. Hench, R. J. Splinter, W. C. Allen and T. K. Greenlee, Bonding mechanisms at the interface of ceramic prosthetic
materials, J. Biomed. Mater. Res., 1971, 5(6), 117–141 CrossRef.
- A. Hoppe, N. S. Güldal and A. R. Boccaccini, A review of the biological response to ionic dissolution products from bioactive glasses and glass-ceramics, Biomaterials, 2011, 32(11), 2757–2774 CrossRef CAS PubMed.
- L. L. Hench, Genetic design of bioactive glass, J. Eur. Ceram. Soc., 2009, 29(7), 1257–1265 CrossRef CAS.
- S. Kargozar, F. Baino, S. Hamzehlou, R. G. Hill and M. Mozafari, Bioactive Glasses: Sprouting Angiogenesis in Tissue Engineering, Trends Biotechnol., 2018, 36(4), 430–444 CrossRef CAS PubMed.
- A. A. Gorustovich, J. A. Roether and A. R. Boccaccini, Effect of Bioactive Glasses on Angiogenesis: A Review of In Vitro and In Vivo Evidences, Tissue Eng., Part B, 2010, 16(2), 199–207 CrossRef CAS PubMed.
- U. Pantulap, M. Arango-Ospina and A. R. Boccaccini, Bioactive glasses incorporating less-common ions to improve biological and physical properties, J. Mater. Sci.: Mater. Med., 2021, 33(1), 3 CrossRef PubMed.
- K. Zheng, W. Niu, B. Lei and A. R. Boccaccini, Immunomodulatory bioactive glasses for tissue regeneration, Acta Biomater., 2021, 133, 168–186 CrossRef CAS PubMed.
- S. Gupta, S. Majumdar and S. Krishnamurthy, Bioactive glass: A multifunctional delivery system, J. Controlled Release, 2021, 335, 481–497 CrossRef CAS PubMed.
- I. Farooq, Z. Imran, U. Farooq, M. A. Leghari and H. Ali, Bioactive Glass: A Material for the Future, World J. Dent., 2012, 3, 199–201 CrossRef.
- M. Cannio, D. Bellucci, J. A. Roether, D. N. Boccaccini and V. Cannillo, Bioactive Glass Applications: A Literature Review of Human Clinical Trials, Materials, 2021, 14(18), 5440 CrossRef CAS PubMed.
-
A. Hoppe and A. R. Boccaccini, Biological Impact of Bioactive Glasses and Their Dissolution Products, in Biomaterials for Oral and Craniomaxillofacial Applications, 2015, vol. 17, pp. 22–32 Search PubMed.
- L. C. Gerhardt and A. R. Boccaccini, Bioactive Glass and Glass-Ceramic Scaffolds for Bone Tissue Engineering, Materials, 2010, 3(7), 3867–3910 CrossRef CAS PubMed.
- A. A. El-Rashidy, J. A. Roether, L. Harhaus, U. Kneser and A. R. Boccaccini, Regenerating bone with bioactive glass scaffolds: A review of in vivo studies in bone defect models, Acta Biomater., 2017, 62, 1–28 CrossRef CAS PubMed.
- J. R. Jones, Review of bioactive glass: From Hench to hybrids, Acta Biomater., 2013, 9(1), 4457–4486 CrossRef CAS PubMed.
- L. L. Hench, N. Roki and M. B. Fenn, Bioactive glasses: Importance of structure and properties in bone regeneration, J. Mol. Struct., 2014, 1073, 24–30 CrossRef CAS.
-
J. Will, L. C. Gerhardt and A. R. Boccaccini, Bioactive Glass-Based Scaffolds for Bone Tissue Engineering, in Tissue Engineering III: Cell - Surface Interactions for Tissue Culture, ed. C. Kasper, F. Witte and R. Pörtner, Advances in Biochemical Engineering Biotechnology, Springer, Berlin, Heidelberg, 2012. pp. 195–226. DOI:10.1007/10_2011_106.
- M. D. O’Donnell and R. G. Hill, Influence of strontium and the importance of glass chemistry and structure when designing bioactive glasses for bone regeneration, Acta Biomater., 2010, 6(7), 2382–2385 CrossRef PubMed.
- M. N. Rahaman, D. E. Day, B. Sonny Bal, Q. Fu, S. B. Jung and L. F. Bonewald,
et al., Bioactive glass in tissue engineering, Acta Biomater., 2011, 7(6), 2355–2373 CrossRef CAS PubMed.
- V. Miguez-Pacheco, L. L. Hench and A. R. Boccaccini, Bioactive glasses beyond bone and teeth: Emerging applications in contact with soft tissues, Acta Biomater., 2015, 13, 1–15 CrossRef CAS PubMed.
- S. Kargozar, S. Hamzehlou and F. Baino, Can bioactive glasses be useful to accelerate the healing of epithelial tissues?, Mater. Sci. Eng., C, 2019, 97, 1009–1020 CrossRef CAS PubMed.
- S. Kargozar, S. Hamzehlou and F. Baino, Potential of Bioactive Glasses for Cardiac and Pulmonary Tissue Engineering, Materials, 2017, 10(12), 1429 CrossRef PubMed.
- S. Kargozar, M. Mozafari, S. Hamzehlou and F. Baino, Using Bioactive Glasses in the Management of Burns, Front. Bioeng. Biotechnol., 2019, 7 DOI:10.3389/fbioe.2019.00062.
- C. Wu, J. Chang and Y. Xiao, Mesoporous bioactive glasses as drug delivery and bone tissue regeneration platforms, Ther. Delivery, 2011, 2(9), 1189–1198 CrossRef CAS PubMed.
- J. Hum and A. R. Boccaccini, Bioactive glasses as carriers for bioactive molecules and therapeutic drugs: a review, J. Mater. Sci.: Mater. Med., 2012, 23(10), 2317–2333 CrossRef CAS PubMed.
- C. Vichery and J. M. Nedelec, Bioactive Glass Nanoparticles: From Synthesis to Materials Design for Biomedical Applications, Materials, 2016, 9(4), 288 CrossRef PubMed.
- A. Obata, S. Lee and T. Kasuga, Bioactive glass materials for tissue regeneration, J. Ceram. Soc. Jpn., 2022, 130(8), 595–604 CrossRef CAS.
- K. E. Wallace, R. G. Hill, J. T. Pembroke, C. J. Brown and P. V. Hatton, Influence of sodium oxide content on bioactive glass properties, J. Mater. Sci.: Mater. Med., 1999, 10(12), 697–701 CrossRef CAS PubMed.
- I. Farooq, M. Tylkowski, S. Müller, T. Janicki, D. S. Brauer and R. G. Hill, Influence of sodium content on the properties of bioactive glasses for use in air abrasion, Biomed. Mater., 2013, 8(6), 065008 CrossRef CAS PubMed.
- J. Jiusti, D. R. Cassar and E. D. Zanotto, Which glass stability parameters can assess the glass–forming ability of oxide systems?, Int. J. Appl. Glass Sci., 2020, 11(4), 612–621 CrossRef CAS.
- T. Mehrabi, A. S. Mesgar and Z. Mohammadi, Bioactive Glasses: A Promising Therapeutic Ion Release Strategy for Enhancing Wound Healing, ACS Biomater. Sci. Eng., 2020, 6(10), 5399–5430 CrossRef CAS PubMed.
- M. Fuchs, E. Gentleman, S. Shahid, R. G. Hill and D. S. Brauer, Therapeutic Ion-Releasing Bioactive Glass Ionomer Cements with Improved Mechanical Strength and Radiopacity, Front. Mater., 2015, 2, 63 Search PubMed.
- C. Wu and J. Chang, Multifunctional mesoporous bioactive glasses for effective delivery of therapeutic ions and drug/growth factors, J. Controlled Release, 2014, 193, 282–295 CrossRef CAS PubMed.
- R. Hill, An alternative view of the degradation of bioglass, J. Mater. Sci. Lett., 1996, 15(13), 1122–1125 CrossRef CAS.
- D. S. Brauer, Bioactive Glasses-Structure and Properties, Angew. Chem., Int. Ed., 2015, 54(14), 4160–4181 CrossRef CAS PubMed.
- S. J. Watts, R. G. Hill, M. D. O'Donnell and R. V. Law, Influence of magnesia on the structure and properties of bioactive glasses, J. Non-Cryst. Solids, 2010, 356(9–10), 517–524 CrossRef CAS.
- L. L. Dai, F. Nudelman, C. H. Chu, E. C. M. Lo and M. L. Mei, The effects of strontium-doped bioactive glass and fluoride on hydroxyapatite crystallization, J. Dent., 2021, 105, 103581 CrossRef CAS PubMed.
- Y. Yu, Z. Bacsik and M. Edén, Contrasting In Vitro Apatite Growth from Bioactive Glass Surfaces with that of Spontaneous Precipitation, Materials, 2018, 11(9), 1690 CrossRef PubMed.
- M. Edén, The split network analysis for exploring composition–structure correlations in multi-component glasses: I. Rationalizing bioactivity-composition trends of bioglasses, J. Non-Cryst. Solids, 2011, 357(6), 1595–1602 CrossRef.
- G. Kaur, O. P. Pandey, K. Singh, D. Homa, B. Scott and G. Pickrell, A review of bioactive glasses: Their structure, properties, fabrication and apatite formation, J. Biomed. Mater. Res., Part A, 2014, 102(1), 254–274 CrossRef PubMed.
- M. Blochberger, L. Hupa and D. S. Brauer, Influence of zinc and magnesium substitution on ion release from Bioglass 45S5 at physiological and acidic pH, Biomed. Glasses, 2015, 1(1) DOI:10.1515/bglass-2015-0009.
- S. Charjri, S. Bouhazma, I. Adouar, S. Herradi, M. Khaldi and B. El Bali,
et al., Synthesis, characterization, and evaluation of bioactivity in the CaO-SiO2-P2O5-MgO system with different CaO/MgO Ratios, J. Phys.: Conf. Ser., 2019, 1292, 012013 CrossRef.
- M. R. T. Filgueiras, G. La Torre and L. L. Hench, Solution effects on the surface reactions of three bioactive glass compositions, J. Biomed. Mater. Res., 1993, 27(12), 1485–1493 CrossRef CAS PubMed.
- M. R. Filgueiras, G. La Torre and L. L. Hench, Solution effects on the surface reactions of a bioactive glass, J. Biomed. Mater. Res., 1993, 27(4), 445–453 CrossRef CAS PubMed.
- Y. C. Fredholm, N. Karpukhina, D. S. Brauer, J. R. Jones, R. V. Law and R. G. Hill, Influence of strontium for calcium substitution in bioactive glasses on degradation, ion release and apatite formation, J. R. Soc., Interface, 2011, 9(70), 880–889 CrossRef PubMed.
- M. Mneimne, R. G. Hill, A. J. Bushby and D. S. Brauer, High phosphate content significantly increases apatite formation of fluoride-containing bioactive glasses, Acta Biomater., 2011, 7(4), 1827–1834 CrossRef CAS PubMed.
- D. S. Brauer, N. Karpukhina, M. D. O'Donnell, R. V. Law and R. G. Hill, Fluoride-containing bioactive glasses: Effect of glass design and structure on degradation, pH and apatite formation in simulated body fluid, Acta Biomater., 2010, 6(8), 3275–3282 CrossRef CAS PubMed.
- S. M. Rabiee, N. Nazparvar, M. Azizian, D. Vashaee and L. Tayebi, Effect of ion substitution on properties of bioactive glasses: A review, Ceram. Int., 2015, 41(6), 7241–7251 CrossRef CAS.
- M. N. Rahaman, D. E. Day, B. Sonny Bal, Q. Fu, S. B. Jung and L. F. Bonewald,
et al., Bioactive glass in tissue engineering, Acta Biomater., 2011, 7(6), 2355–2373 CrossRef CAS PubMed.
- L. L. Hench and H. A. Paschall, Direct chemical bond of bioactive glass-ceramic materials to bone and muscle, J. Biomed. Mater. Res., 1973, 7(3), 25–42 CrossRef CAS PubMed.
- E. Dietrich, H. Oudadesse, A. Lucas-Girot and M. Mami,
In vitro bioactivity of melt-derived glass 46S6 doped with magnesium, J. Biomed. Mater. Res., Part A, 2009, 88(4), 1087–1096 CrossRef PubMed.
- D. Arcos, D. C. Greenspan and M. Vallet-Regí, A new quantitative method to evaluate the in vitro bioactivity of melt and sol-gel-derived silicate glasses: Bioactivity of Silicate Glasses, J. Biomed. Mater. Res., Part A, 2003, 65(3), 344–351 CrossRef CAS PubMed.
- I. Elgayar, A. E. Aliev, A. R. Boccaccini and R. G. Hill, Structural analysis of bioactive glasses, J. Non-Cryst. Solids, 2005, 351(2), 173–183 CrossRef CAS.
- M. Tylkowski and D. S. Brauer, Mixed alkali effects in Bioglass® 45S5, J. Non-Cryst. Solids, 2013, 376, 175–181 CrossRef CAS.
- M. C. Crovace, V. O. Soares, A. C. M. Rodrigues, O. Peitl, L. M. S. C. Raucci and P. T. de Oliveira,
et al., Understanding the mixed alkali effect on the sinterability and in vitro performance of bioactive glasses, J. Eur. Ceram. Soc., 2021, 41(7), 4391–4405 CrossRef CAS.
- D. S. Brauer, R. Brückner, M. Tylkowski and L. Hupa, Sodium-free mixed alkali bioactive glasses, Biomed. Glasses, 2016, 2(1) DOI:10.1515/bglass-2016-0012.
- M. Tylkowski and D. S. Brauer, Mixed alkali effects in Bioglass® 45S5, J. Non-Cryst. Solids, 2013, 376, 175–181 CrossRef CAS.
- A. Atila, Y. Ouldhnini, S. Ouaskit and A. Hasnaoui, Atomistic insights into the mixed-alkali effect in phosphosilicate glasses, Phys. Rev. B, 2022, 105(13), 134101 CrossRef CAS.
- A. Goel, S. Kapoor, R. R. Rajagopal, M. J. Pascual, H. W. Kim and J. M. F. Ferreira, Alkali-free bioactive glasses for bone tissue engineering: A preliminary
investigation, Acta Biomater., 2012, 8(1), 361–372 CrossRef CAS PubMed.
- A. F. Brito, B. Antunes, F. dos Santos, H. R. Fernandes and J. M. F. Ferreira, Osteogenic capacity of alkali-free bioactive glasses. In vitro studies: osteogenic capacity of alkali-free bioactive glasses, J. Biomed. Mater. Res., 2017, 105(8), 2360–2365 CrossRef CAS PubMed.
- S. Aqdim, M. Naji, A. Chakir and A. E. Bouari, Design, synthesis and structural properties of borate glasses: Towards an alkali-free bioactive glass, J. Non-Cryst. Solids, 2022, 597, 121876 CrossRef CAS.
- A. Gaddam, P. Gołębiewski, H. R. Fernandes, D. Pysz, A. S. Neto and R. Diduszko,
et al., Development of microfibers for bone regeneration based on alkali–free bioactive glasses doped with boron oxide, J. Am. Ceram. Soc., 2021, 104(9), 4492–4504 CrossRef CAS.
- L. L. Hench, Bioceramics: From Concept to Clinic, J. Am. Ceram. Soc., 1991, 74(7), 1487–1510 CrossRef CAS.
- M. Arango-Ospina, L. Hupa and A. R. Boccaccini, Bioactivity and dissolution behavior of boron-containing bioactive glasses under static and dynamic conditions in different media, Biomed. Glasses, 2019, 5(1), 124–139 Search PubMed.
- S. Fagerlund, P. Ek, L. Hupa and M. Hupa, Dissolution Kinetics of a Bioactive Glass by Continuous Measurement, J. Am. Ceram. Soc., 2012, 95(10), 3130–3137 CrossRef CAS.
- A. Stiller, M. Engblom, O. Karlström, M. Lindén and L. Hupa, Impact of fluid flow rate on the dissolution behavior of bioactive glass S53P4, J. Non-Cryst. Solids, 2023, 607, 122219 CrossRef CAS.
- L. Hupa, S. Fagerlund, J. Massera and L. Björkvik, Dissolution behavior of the bioactive glass S53P4 when sodium is replaced by potassium, and calcium with magnesium or strontium, J. Non-Cryst. Solids, 2016, 432, 41–46 CrossRef CAS.
-
J. E. Shelby, Introduction to Glass Science and Technology, The Royal Society of Chemistry, 1997. Available from: https://onlinelibrary.wiley.com/doi/abs/10.1002/ange.19971092243 Search PubMed.
- P. Panda and R. Raj, Sintering and Crystallization of Glass at Constant Heating Rates, J. Am. Ceram. Soc., 2005, 72, 1564–1566 CrossRef.
- M. Ferraris and E. Verné, Viscous phase sintering of particle-reinforced glass matrix composites, J. Eur. Ceram. Soc., 1996, 16(4), 421–427 CrossRef CAS.
- C. Lara, M. J. Pascual and A. Durán, Glass-forming ability, sinterability and thermal properties in the systems RO–BaO–SiO2 (R=Mg, Zn), J. Non-Cryst. Solids, 2004, 348, 149–155 CrossRef CAS.
- F. Baino, M. Ferraris, O. Bretcanu, E. Verné and C. Vitale-Brovarone, Optimization of composition, structure and mechanical strength of bioactive 3-D glass-ceramic scaffolds for bone substitution, J. Biomater. Appl., 2013, 27(7), 872–890 CrossRef CAS PubMed.
- I. Kansal, D. U. Tulyaganov, A. Goel, M. J. Pascual and J. M. F. Ferreira, Structural analysis and thermal behavior of diopside-fluorapatite-wollastonite-based glasses and glass-ceramics, Acta Biomater., 2010, 6(11), 4380–4388 CrossRef CAS PubMed.
-
P.W McMillan, Glass-ceramics, Non-metallic solids, Academic Press, London, 2nd edn, 1979, 285 p Search PubMed.
-
M. V. Artamonova, M. S. Aslanova and I. M. Buzhinsky, Chemical Technology of Glasses and Glass-Ceramics, Stroiizdat, 1983 Search PubMed.
- E. Verné, O. Bretcanu, C. Balagna, C. L. Bianchi, M. Cannas and S. Gatti,
et al., Early stage reactivity and in vitro behavior of silica-based bioactive glasses and glass-ceramics, J. Mater. Sci.: Mater. Med., 2009, 20(1), 75–87 CrossRef PubMed.
- A. Nommeots-Nomm, C. Ligorio, A. J. Bodey, B. Cai, J. R. Jones and P. D. Lee,
et al., Four-dimensional imaging and quantification of viscous flow sintering within a 3D printed bioactive glass scaffold using synchrotron X-ray tomography, Mater. Today Adv., 2019, 2, 100011 CrossRef.
- M. Brink, The influence of alkali and alkaline earths on the working range for bioactive glasses, J. Biomed. Mater. Res., 1997, 36(1), 109–117 CrossRef CAS PubMed.
- E. Mancuso, O. A. Bretcanu, M. Marshall, M. A. Birch, A. W. McCaskie and K. W. Dalgarno, Novel bioglasses for bone tissue repair and regeneration: Effect of glass design on sintering ability, ion release and biocompatibility, Mater. Des., 2017, 129, 239–248 CrossRef CAS PubMed.
- E. Fiume, G. Serino, C. Bignardi, E. Verné and F. Baino, Sintering Behavior of a Six-Oxide Silicate Bioactive Glass for Scaffold Manufacturing, Appl. Sci., 2020, 10(22), 8279 CrossRef CAS.
- O. Bretcanu, X. Chatzistavrou, K. Paraskevopoulos, R. Conradt, I. Thompson and A. R. Boccaccini, Sintering and crystallisation of 45S5 Bioglass® powder, J. Eur. Ceram. Soc., 2009, 29(16), 3299–3306 CrossRef CAS.
- S. Lopez-Esteban, C. F. Gutierrez-Gonzalez, L. Gremillard, E. Saiz and A. P. Tomsia, Interfaces in graded coatings on titanium-based implants, J. Biomed. Mater. Res., Part A, 2009, 88(4), 1010–1021 CrossRef CAS PubMed.
- F. Baino, M. Marshall, N. Kirk and C. Vitale-Brovarone, Design, selection and characterization of novel glasses and glass-ceramics for use in prosthetic applications, Ceram. Int., 2015, 42, 1482–1491 CrossRef.
- S. Lopez-Esteban, E. Saiz, S. Fujino, T. Oku, K. Suganuma and A. P. Tomsia, Bioactive glass coatings for orthopedic metallic implants, J. Eur. Ceram. Soc., 2003, 23(15), 2921–2930 CrossRef CAS.
- J. M. Gomez-Vega, E. Saiz, A. P. Tomsia, T. Oku, K. Suganuma and G. W. Marshall,
et al., Novel Bioactive Functionally Graded Coatings on Ti6Al4 V, Adv. Mater., 2000, 12(12), 894–898 CrossRef CAS.
- I. Gonzalo-Juan, D. U. Tulyaganov, C. Balan, R. Linser, J. M. F. Ferreira and R. Riedel,
et al., Tailoring the viscoelastic properties of injectable biocomposites: A spectroscopic assessment of the interactions between organic carriers and bioactive glass particles, Mater. Des., 2016, 97, 45–50 CrossRef CAS.
- T. Kokubo, Bioactive glass ceramics: properties and applications, Biomaterials, 1991, 12(2), 155–163 CrossRef CAS PubMed.
- Q. Z. Chen, I. D. Thompson and A. R. Boccaccini, 45S5 Bioglass®-derived glass–ceramic scaffolds for bone tissue engineering, Biomaterials, 2006, 27(11), 2414–2425 CrossRef CAS PubMed.
- R. G. Hill and D. S. Brauer, Predicting the bioactivity of glasses using the network connectivity or split network models, J. Non-Cryst. Solids, 2011, 357(24), 3884–3887 CrossRef CAS.
- J. Massera, S. Fagerlund, L. Hupa and M. Hupa, Crystallization Mechanism of the Bioactive Glasses, 45S5 and S53P4, J. Am. Ceram. Soc., 2012, 95(2), 607–613 CrossRef CAS.
- R. Golovchak, P. Thapar, A. Ingram, D. Savytskii and H. Jain, Influence of phase separation on the devitrification of 45S5 bioglass, Acta Biomater., 2014, 10(11), 4878–4886 CrossRef CAS PubMed.
- F. Baino and E. Fiume, Quantifying the effect of particle size on the crystallization of 45S5 bioactive glass, Mater. Lett., 2018, 224, 54–58 CrossRef CAS.
- A. R. Boccaccini, Q. Chen, L. Lefebvre, L. Gremillard and J. Chevalier, Sintering, crystallisation and biodegradation behaviour of Bioglass®-derived glass–ceramics, Faraday Discuss., 2007, 136, 27–44 RSC.
- Q. Fu, M. N. Rahaman, B. S. Bal, R. F. Brown and D. E. Day, Mechanical and in vitro performance of 13–93 bioactive glass scaffolds prepared by a polymer foam replication technique, Acta Biomater., 2008, 4(6), 1854–1864 CrossRef CAS PubMed.
- O. Peitl, E. D. Zanotto, F. C. Serbena and L. L. Hench, Compositional and microstructural design of highly bioactive P2O5–Na2O–CaO–SiO2 glass-ceramics, Acta Biomater., 2012, 8(1), 321–332 CrossRef CAS PubMed.
- T. Duminis, S. Shahid and R. G. Hill, Apatite Glass-Ceramics: A Review, Front. Mater., 2017, 3 DOI:10.3389/fmats.2016.00059.
- W. Vogel and W. Höland, The Development of Bioglass Ceramics for Medical Applications, Angew. Chem., Int. Ed. Engl., 1987, 26(6), 527–544 CrossRef.
- A. Gebhardt, T. Höche, G. Carl and I. I. Khodos, TEM study on the origin of cabbage-shaped mica crystal aggregates in machinable glass-ceramics, Acta Mater., 1999, 47(17), 4427–4434 CrossRef CAS.
- S. Kapoor, A. Goel, M. J. Pascual and J. M. F. Ferreira, Alkali-free bioactive diopside–tricalcium phosphate glass-ceramics for scaffold fabrication: Sintering and crystallization behaviours, J. Non-Cryst. Solids, 2016, 432, 81–89 CrossRef CAS.
- S. Kapoor, A. Goel, M. J. Pascual and J. M. F. Ferreira, Thermo-mechanical behaviour of alkali free bioactive glass-ceramics co-doped with strontium and zinc, J. Non-Cryst. Solids, 2013, 375, 74–82 CrossRef CAS.
- K. Deshmukh, T. Kovářík, T. Křenek, D. Docheva, T. Stich and J. Pola, Recent advances and future perspectives of sol–gel derived porous bioactive glasses: a review, RSC Adv., 2020, 10(56), 33782–33835 RSC.
- K. Zheng and A. R. Boccaccini, Sol-gel processing of bioactive glass nanoparticles: A review, Adv. Colloid Interface Sci., 2017, 249, 363–373 CrossRef CAS PubMed.
- F. Baino, E. Fiume, M. Miola and E. Verné, Bioactive sol-gel glasses: Processing, properties, and applications, Int. J. Appl. Ceram. Technol., 2018, 15(4), 841–860 CrossRef CAS.
- G. Kaur, G. Pickrell, N. Sriranganathan, V. Kumar and D. Homa, Review and the state of the art: Sol–gel and melt quenched bioactive glasses for tissue engineering, J. Biomed. Mater. Res., Part B, 2016, 104(6), 1248–1275 CrossRef CAS PubMed.
- L. L. Hench, Sol-gel materials for bioceramic applications, Curr. Opin. Solid State Mater. Sci., 1997, 2(5), 604–610 CrossRef CAS.
- R. Li, A. E. Clark and L. L. Hench, An investigation of bioactive glass powders by sol-gel processing, J. Appl. Biomater., 1991, 2(4), 231–239 CrossRef CAS PubMed.
- J. Zhong and D. C. Greenspan, Processing and properties of sol–gel bioactive glasses, J. Biomed. Mater. Res., 2000, 53(6), 694–701 CrossRef CAS PubMed.
- S. Lin, C. Ionescu, S. Baker, M. E. Smith and J. R. Jones, Characterisation of the inhomogeneity of sol-gel-derived SiO2-CaO bioactive glass and a strategy for its improvement, J. Sol-Gel Sci. Technol., 2010, 53(2), 255–262 CrossRef CAS.
- B. Yu, C. A. Turdean-Ionescu, R. A. Martin, R. J. Newport, J. V. Hanna and M. E. Smith,
et al., Effect of calcium source on structure and properties of sol-gel derived bioactive glasses, Langmuir, 2012, 28(50), 17465–17476 CrossRef CAS PubMed.
- S. Lin, C. Ionescu, K. J. Pike, M. E. Smith and J. R. Jones, Nanostructure evolution and calcium distribution in sol–gel derived bioactive glass, J. Mater. Chem., 2009, 19(9), 1276–1282 RSC.
- G. Poologasundarampillai, C. Ionescu, O. Tsigkou, M. Murugesan, R. G. Hill and M. M. Stevens,
et al., Synthesis of bioactive class II poly(γ-glutamic acid)/silica hybrids for bone regeneration, J. Mater. Chem., 2010, 20(40), 8952–8961 RSC.
- T. Miyazaki, C. Ohtsuki and M. Tanihara, Synthesis of bioactive organic-inorganic nanohybrid for bone repair through sol-gel processing, J. Nanosci. Nanotechnol., 2003, 3(6), 511–515 CrossRef CAS PubMed.
- M. M. Pereira, A. E. Clark and L. L. Hench, Calcium phosphate formation on sol-gel-derived bioactive glasses in vitro, J. Biomed. Mater. Res., 1994, 28(6), 693–698 CrossRef CAS PubMed.
- C. Bossard, H. Granel, É. Jallot, V. Montouillout, F. Fayon and J. Soulié,
et al., Mechanism of Calcium Incorporation Inside Sol-Gel Silicate Bioactive Glass and the Advantage of Using Ca(OH)2 over Other Calcium Sources, ACS Biomater. Sci. Eng., 2019, 5(11), 5906–5915 CrossRef CAS PubMed.
- G. Poologasundarampillai, B. Yu, O. Tsigkou, D. Wang, F. Romer and V. Bhakhri,
et al., Poly(γ-glutamic acid)/silica hybrids with calcium incorporated in the silica network by use of a calcium alkoxide precursor, Chemistry, 2014, 20(26), 8149–8160 CrossRef CAS PubMed.
- D. Carta, D. M. Pickup, J. C. Knowles, M. E. Smith and R. J. Newport, Sol–gel synthesis of the P2O5–CaO–Na2O–SiO2 system as a novel bioresorbable glass, J. Mater. Chem., 2005, 15(21), 2134–2140 RSC.
- Q. Z. Chen, Y. Li, L. Y. Jin, J. M. W. Quinn and P. A. Komesaroff, A new sol–gel process for producing Na2O-containing bioactive glass ceramics, Acta Biomater., 2010, 6(10), 4143–4153 CrossRef CAS PubMed.
- S. A. Syed Nuzul Fadzli, R. Shamsudin, S. R. Zainudin and F. Zainuddin, Preperation and Characterization of Macroporous Bioactive Glass Ceramic made via Sol-Gel Route and Powder Sintering Method, Sains Malays., 2018, 47(5), 1025–1031 CrossRef.
- S. Chitra and S. Balakumar, Insight, into the impingement of different sodium precursors on structural, biocompatible, and hemostatic properties of bioactive materials, Mater. Sci. Eng., C, 2021, 123, 111959 CrossRef PubMed.
- A. Kumar, S. Murugavel, A. Aditya and A. R. Boccaccini, Mesoporous 45S5 bioactive glass: synthesis, in vitro dissolution and biomineralization behavior, J. Mater. Chem. B, 2017, 5(44), 8786–8798 RSC.
- J. Soulié, J. M. Nedelec and E. Jallot, Influence of Mg doping on the early steps of physico-chemical reactivity of sol–gel derived bioactive glasses in biological medium, Phys. Chem. Chem. Phys., 2009, 11(44), 10473–10483 RSC.
- R. Bento, A. Gaddam and J. M. F. Ferreira, Sol–Gel Synthesis and Characterization of a Quaternary Bioglass for Bone Regeneration and Tissue Engineering, Materials, 2021, 14(16), 4515 CrossRef CAS PubMed.
- A. Balamurugan, G. Balossier, J. Michel, S. Kannan, H. Benhayoune and A. H. S. Rebelo,
et al., Sol gel derived SiO2-CaO-MgO-P2O5 bioglass system—Preparation and in vitro characterization, J. Biomed. Mater. Res., Part B, 2007, 83(2), 546–553 CrossRef CAS PubMed.
- E. Fiume, C. Migneco, E. Verné and F. Baino, Comparison between Bioactive Sol-Gel and Melt-Derived Glasses/Glass-Ceramics Based on the Multicomponent SiO2–P2O5–CaO–MgO–Na2O–K2O System, Materials, 2020, 13(3), 540 CrossRef CAS PubMed.
- M. Shoaib, A. Saeed, J. Akhtar, M. S. U. Rahman, A. Ullah and K. Jurkschat,
et al., Potassium-doped mesoporous bioactive glass: Synthesis, characterization and evaluation of biomedical properties, Mater. Sci. Eng., C, 2017, 75, 836–844 CrossRef CAS PubMed.
- D. Bellucci, A. Sola, R. Salvatori, A. Anesi, L. Chiarini and V. Cannillo, Sol-gel derived bioactive glasses with low tendency to crystallize: synthesis, post-sintering bioactivity and possible application for the production of porous scaffolds, Mater. Sci. Eng., C, 2014, 43, 573–586 CrossRef CAS PubMed.
- P. Han, C. Wu, J. Chang and Y. Xiao, The cementogenic differentiation of periodontal ligament cells via the activation of Wnt/β-catenin signalling pathway by Li+ ions released from bioactive scaffolds, Biomaterials, 2012, 33(27), 6370–6379 CrossRef CAS PubMed.
- A. L. B. Maçon, M. Jacquemin, S. J. Page, S. Li, S. Bertazzo and M. M. Stevens,
et al., Lithium-silicate sol–gel bioactive glass and the effect of lithium precursor on structure–property relationships, J. Sol-Gel Sci. Technol., 2017, 81(1), 84–94 CrossRef PubMed.
- A. Moghanian, S. Firoozi and M. Tahriri, Synthesis and in vitro studies of sol-gel derived lithium substituted 58S bioactive glass, Ceram. Int., 2017, 43(15), 12835–12843 CrossRef CAS.
- S. Li, A. L. B. Maçon, M. Jacquemin, M. M. Stevens and J. R. Jones, Sol-gel derived lithium-releasing glass for cartilage regeneration, J. Biomater. Appl., 2017, 32(1), 104–113 CrossRef CAS PubMed.
- L. Mosqueira, B. R. Barrioni, T. Martins, F. G. Melo, N. M. Ocarino and R. Serakides,
et al., Strontium-releasing sol–gel bioactive glass spheres and their ability to stimulate osteogenic differentiation in osteoporotic bone marrow mesenchymal stem cells, J. Mater. Res., 2021, 36(2), 459–474 CrossRef CAS.
- S. Hesaraki, M. Gholami, S. Vazehrad and S. Shahrabi, The effect of Sr concentration on bioactivity and biocompatibility of sol–gel derived glasses based on CaO–SrO–SiO2–P2O5 quaternary system, Mater. Sci. Eng., C, 2010, 30(3), 383–390 CrossRef CAS.
- H. Manoochehri, M. Ghorbani, M. Moosazadeh Moghaddam, M. R. Nourani, P. Makvandi and E. Sharifi, Strontium doped bioglass incorporated hydrogel-based scaffold for amplified bone tissue regeneration, Sci. Rep., 2022, 12(1), 10160 CrossRef CAS PubMed.
- J. Lao, E. Jallot and J. M. Nedelec, Strontium-Delivering Glasses with Enhanced Bioactivity: A New Biomaterial for Antiosteoporotic Applications?, Chem. Mater., 2008, 20, 4969–4973 CrossRef CAS.
- S. Amudha, J. R. Ramya, K. T. Arul, A. Deepika, P. Sathiamurthi and B. Mohana,
et al., Enhanced mechanical and biocompatible properties of strontium ions doped mesoporous bioactive glass, Composites, Part B, 2020, 196, 108099 CrossRef CAS.
- S. Fiorilli, G. Molino, C. Pontremoli, G. Iviglia, E. Torre and C. Cassinelli,
et al., The Incorporation of Strontium to Improve Bone-Regeneration Ability of Mesoporous Bioactive Glasses, Materials, 2018, 11(5), 678 CrossRef PubMed.
- S. Taherkhani and F. Moztarzadeh, Influence of strontium on the structure and biological properties of sol–gel-derived mesoporous bioactive glass (MBG) powder, J. Sol-Gel Sci. Technol., 2016, 78(3), 539–549 CrossRef CAS.
- S. Ouyang, K. Zheng, Q. Huang, Y. Liu and A. R. Boccaccini, Synthesis and characterization of rubidium-containing bioactive glass nanoparticles, Mater. Lett., 2020, 273, 127920 CrossRef CAS.
- S. Majumdar, S. K. Hira, H. Tripathi, A. S. Kumar, P. P. Manna and S. P. Singh,
et al., Synthesis and characterization of barium-doped bioactive glass with potential anti-inflammatory activity, Ceram. Int., 2021, 47(5), 7143–7158 CrossRef CAS.
- S. H. Rhee, Y. K. Lee, B. S. Lim, J. J. Yoo and H. J. Kim, Evaluation of a novel poly(epsilon-caprolactone)-organosiloxane hybrid material for the potential application as a bioactive and degradable bone substitute, Biomacromolecules, 2004, 5(4), 1575–1579 CrossRef CAS PubMed.
- J. Lao, X. Dieudonné, F. Fayon, V. Montouillout and E. Jallot, Bioactive glass–gelatin hybrids: building scaffolds with enhanced calcium incorporation and controlled porosity for bone regeneration, J. Mater. Chem. B, 2016, 4(14), 2486–2497 RSC.
- S. Z. Jalise, N. Baheiraei and F. Bagheri, The effects of strontium incorporation on a novel gelatin/bioactive glass bone graft: In vitro and in vivo characterization, Ceram. Int., 2018, 44(12), 14217–14227 CrossRef.
- D. P. Kuffler, Can lithium enhance the extent of axon regeneration and neurological recovery following peripheral nerve trauma?, Neural Regen. Res., 2021, 17(5), 948–952 CrossRef PubMed.
- A. M. El-Kady, M. M. Farag and A. M. I. El-Rashedi, Bioactive glass nanoparticles designed for multiple deliveries of lithium ions and drugs: Curative and restorative bone treatment, Eur. J. Pharm. Sci., 2016, 91, 243–250 CrossRef CAS PubMed.
- L. A. Acevedo, L. A. Campos, I. C. Dechandt, G. Alegria, R. L. Siqueira and E. D. Zanotto,
et al., Effect of bioactive glasses containing strontium and potassium on dentin permeability, J. Biomed. Mater. Res., Part B, 2022, 110(3), 517–526 CrossRef CAS PubMed.
- D. Bellucci, V. Cannillo, G. Ciardelli, P. Gentile and A. Sola, Potassium based bioactive glass for bone tissue engineering, Ceram. Int., 2010, 36(8), 2449–2453 CrossRef CAS.
- V. Cannillo and A. Sola, Potassium-Based Composition for a Bioactive Glass, Ceram. Int., 2009, 35, 3389–3393 CrossRef CAS.
- M. Tiskaya, D. Gillam, S. Shahid and R. Hill, A Potassium Based Fluorine Containing Bioactive Glass for Use as a Desensitizing Toothpaste, Molecules, 2021, 26(14), 4327 CrossRef CAS PubMed.
- M. Diba, F. Tapia, A. R. Boccaccini and L. A. Strobel, Magnesium-Containing Bioactive Glasses for Biomedical Applications, Int. J. Appl. Glass Sci., 2012, 3(3), 221–253 CrossRef CAS.
- M. Diba, O. M. Goudouri, F. Tapia and A. R. Boccaccini, Magnesium-containing bioactive polycrystalline silicate-based ceramics and glass-ceramics for biomedical applications, Curr. Opin. Solid State Mater. Sci., 2014, 18(3), 147–167 CrossRef CAS.
- V. G. Varanasi, J. B. Owyoung, E. Saiz, S. J. Marshall, G. W. Marshall and P. M. Loomer, The ionic products of bioactive glass particle dissolution enhance periodontal ligament fibroblast osteocalcin expression and enhance early mineralized tissue development, J. Biomed. Mater. Res., Part A, 2011, 98(2), 177–184 CrossRef PubMed.
- M. Bohner, Calcium orthophosphates in medicine: from ceramics to calcium phosphate cements, Injury, 2000, 31, D37–D47 CrossRef PubMed.
- S. Kargozar, M. Montazerian, E. Fiume and F. Baino, Multiple and Promising Applications of Strontium (Sr)-Containing Bioactive Glasses in Bone Tissue Engineering, Front. Bioeng. Biotechnol., 2019, 7, 161 CrossRef PubMed.
- S. C. Verberckmoes, M. E. De Broe and P. C. D'Haese, Dose-dependent effects of strontium on osteoblast function and mineralization, Kidney Int., 2003, 64(2), 534–543 CrossRef CAS PubMed.
- P. J. Marie, Strontium ranelate: New insights into its dual mode of action, Bone, 2007, 40(5, Supplement 1), S5–S8 CrossRef CAS.
- M. Huang, R. G. Hill and S. C. F. Rawlinson, Strontium (Sr) elicits odontogenic differentiation of human dental pulp stem cells (hDPSCs): A therapeutic role for Sr in dentine repair?, Acta Biomater., 2016, 38, 201–211 CrossRef CAS PubMed.
- A. Schindeler and D. G. Little, Ras-MAPK Signaling in Osteogenic Differentiation: Friend or Foe?, J. Bone Miner. Res., 2006, 21(9), 1331–1338 CrossRef PubMed.
- F. Yang, D. Yang, J. Tu, Q. Zheng, L. Cai and L. Wang, Strontium Enhances Osteogenic Differentiation of Mesenchymal Stem Cells and In Vivo Bone Formation by Activating Wnt/Catenin Signaling, Stem Cells, 2011, 29(6), 981–991 CrossRef CAS PubMed.
- Y. Chen, H. C. Whetstone, A. C. Lin, P. Nadesan, Q. Wei and R. Poon,
et al., Beta-Catenin Signaling Plays a Disparate Role in Different Phases of Fracture Repair: Implications for Therapy to Improve Bone Healing, PLoS Med., 2007, 4(7), e249 CrossRef PubMed.
- S. O'Donnell, A. Cranney, G. A. Wells, J. Adachi and J. Y. Reginster, Strontium ranelate for preventing and treating postmenopausal osteoporosis, Cochrane Database Syst. Rev., 2006 DOI:10.1002/14651858.CD005326.pub3.
- S. Peng, G. Zhou, K. D. K. Luk, K. M. C. Cheung, Z. Li and W. M. Lam,
et al., Strontium Promotes Osteogenic Differentiation of Mesenchymal Stem Cells Through the Ras/MAPK Signaling Pathway, Cell. Physiol. Biochem., 2009, 23(1–3), 165–174 CrossRef CAS PubMed.
- E. Gentleman, Y. Fredholm, G. Jell, N. Lotfibakhshaiesh, M. O'Donnell and R. Hill,
et al., The Effects of Strontium-Substituted Bioactive Glasses on Osteoblasts and Osteoclasts In Vitro, Biomaterials, 2010, 31, 3949–3956 CrossRef CAS PubMed.
- J. Liu, S. C. F. Rawlinson, R. G. Hill and F. Fortune, Strontium-substituted bioactive glasses in vitro osteogenic and antibacterial effects, Dent. Mater., 2016, 32(3), 412–422 CrossRef CAS PubMed.
- N. Esfahanizadeh, M. Montazeri, M. R. Nourani and M. Harandi, Use of bioactive glass doped with magnesium or strontium for bone regeneration: A rabbit critical-size calvarial defects study, Dent. Res. J., 2022, 19, 18 CrossRef PubMed.
- S. Majumdar and S. Krishnamurthy, In vivo toxicological evaluation of barium-doped bioactive glass in rats, Ceram. Int., 2022, 48(22), 33288–33305 CrossRef CAS.
- P. Paliwal, A. S. Kumar, H. Tripathi, S. P. Singh, S. C. U. Patne and S. Krishnamurthy, Pharmacological application of barium containing bioactive glass in gastro-duodenal ulcers, Mater. Sci. Eng., C, 2018, 92, 424–434 CrossRef CAS PubMed.
- J. Pawlik, M. Widziołek, K. Cholewa-Kowalska, M. Łączka and A. M. Osyczka, New sol-gel bioactive glass and titania composites with enhanced physico-chemical and biological properties, J. Biomed. Mater. Res., Part A, 2014, 102(7), 2383–2394 CrossRef PubMed.
- O. Rodriguez, W. Stone, E. H. Schemitsch, P. Zalzal, S. Waldman and M. Papini,
et al., Titanium addition influences antibacterial activity of bioactive glass coatings on metallic implants, Heliyon, 2017, 3(10), e00420 CrossRef PubMed.
- S. Smith, O. ElKashty, F. Tamimi, S. D. Tran and M. Cerruti, Titanium-Containing Silicate-Based Sol–Gel Bioactive Glass: Development, Characterization, and Applications, Langmuir, 2021, 37(49), 14243–14253 CrossRef CAS PubMed.
- R. Shafaghi, O. Rodriguez, S. Phull, E. H. Schemitsch, P. Zalzal and S. D. Waldman,
et al., Effect of TiO2 doping on degradation rate, microstructure and strength of borate bioactive glass scaffolds, Mater. Sci. Eng., C, 2020, 107, 110351 CrossRef CAS PubMed.
- A. M. Deliormanli, In vitro assessment of degradation and mineralisation of V2O5 substituted borate bioactive glass scaffolds, Mater. Technol., 2014, 29(6), 358–365 CrossRef CAS.
- A. Deliormanlı, Size-dependent degradation and bioactivity of borate bioactive glass, Ceram. Int., 2013, 39, 8087–8095 CrossRef.
- K. E. Moyer, A. A. Saba, R. M. Hauck and H. P. Ehrlich, Systemic vanadate ingestion modulates rat tendon repair, Exp. Mol. Pathol., 2003, 75(1), 80–88 CrossRef CAS PubMed.
- A. M. Deliormanlı, H. Seda Vatansever, H. Yesil and F. Özdal-Kurt, In vivo evaluation of cerium, gallium and vanadium-doped borate-based bioactive glass scaffolds using rat subcutaneous implantation model, Ceram. Int., 2016, 42(10), 11574–11583 CrossRef.
- J. Li, X. Li, J. Li, X. Pu, J. Wang and Z. Huang,
et al., Effects of incorporated vanadium and its chemical states on morphology and mesostructure of mesoporous bioactive glass particles, Microporous Mesoporous Mater., 2021, 319, 111061 CrossRef CAS.
- B. R. Barrioni, P. Naruphontjirakul, E. Norris, S. Li, N. L. Kelly and J. V. Hanna,
et al., Effects of manganese incorporation on the morphology, structure and cytotoxicity of spherical bioactive glass nanoparticles, J. Colloid Interface Sci., 2019, 547, 382–392 CrossRef CAS PubMed.
- P. Bragiel, P. Ficek, W. Prochwicz, I. Radkowska and N. Veeraiah, Are the phosphorus-rich NaO–CaO–BO–SiO–PO glasses bioactive and what is an influence of doping with manganese oxide?, Mater. Sci.-Pol., 2017, 35(4), 760–766 CrossRef CAS.
- B. R. Barrioni, E. Norris, S. Li, P. Naruphontjirakul, J. R. Jones and M. d. M. Pereira, Osteogenic potential of sol–gel bioactive glasses containing manganese, J. Mater. Sci.: Mater. Med., 2019, 30(7), 86 CrossRef PubMed.
- B. R. Barrioni, A. C. Oliveira, M. de Fátima Leite and M. de Magalhães Pereira, Sol–gel-derived manganese-releasing bioactive glass as a therapeutic approach for bone tissue engineering, J. Mater. Sci., 2017, 52(15), 8904–8927 CrossRef CAS.
- M. Curcio, A. De Stefanis, A. De Bonis, R. Teghil and J. V. Rau, Pulsed laser deposited bioactive RKKP-Mn glass-ceramic coatings on titanium, Surf. Coat. Technol., 2019, 357, 122–128 CrossRef CAS.
- M. Miola, C. V. Brovarone, G. Maina, F. Rossi, L. Bergandi and D. Ghigo,
et al., In vitro study of manganese-doped bioactive glasses for bone regeneration, Mater. Sci. Eng., C, 2014, 38, 107–118 CrossRef CAS PubMed.
- Q. Nawaz, M. A. U. Rehman, A. Burkovski, J. Schmidt, A. M. Beltrán and A. Shahid,
et al., Synthesis and characterization of manganese containing mesoporous bioactive glass nanoparticles for biomedical applications, J. Mater. Sci.: Mater. Med., 2018, 29(5), 64 CrossRef PubMed.
- A. El-Fiqi and H. W. Kim, Iron ions-releasing mesoporous bioactive glass ultrasmall nanoparticles designed as ferroptosis-based bone cancer nanotherapeutics: Ultrasonic-coupled sol–gel synthesis, properties and iron ions release, Mater. Lett., 2021, 294, 129759 CrossRef CAS.
- Y. Li, V. Ramesh, F. Bider, N. Bradshaw, C. Rehbock and A. R. Boccaccini,
et al., Co-doping of iron and copper ions in nanosized bioactive glass by reactive laser fragmentation in liquids, J. Biomed. Mater. Res., Part A, 2022, 110(9), 1537–1550 CrossRef CAS PubMed.
-
Bioactive Glass and Glass-Ceramics Containing Iron Oxide: Preparation and Properties, in Trends in Biomaterials, Jenny Stanford Publishing, 2016 Search PubMed.
- F. Kermani, A. Vojdani-Saghir, S. Mollazadeh Beidokhti, S. Nazarnezhad, Z. Mollaei and S. Hamzehlou,
et al., Iron (Fe)-doped mesoporous 45S5 bioactive glasses: Implications for cancer therapy, Transl. Oncol., 2022, 20, 101397 CrossRef PubMed.
- A. Yazdanpanah and F. Moztarzadeh, Synthesis and characterization of Barium-Iron containing magnetic bioactive glasses: The effect of magnetic component on structure and in vitro bioactivity, Colloids Surf., B, 2019, 176, 27–37 CrossRef CAS PubMed.
- F. Baino, M. Montazerian and E. Verné, Cobalt-Doped Bioactive Glasses for Biomedical Applications: A Review, Materials, 2023, 16(14), 4994 CrossRef CAS PubMed.
- S. Chen, M. Michálek, D. Galusková, M. Michálková, P. Švančárek and A. Talimian,
et al., Multi-targeted B and Co co-doped 45S5 bioactive glasses with angiogenic potential for bone regeneration, Mater. Sci. Eng., C, 2020, 112, 110909 CrossRef CAS PubMed.
- A. G. S. de Laia, T. M. Valverde, B. R. Barrioni, P. d. S. Cunha, A. M. de Goes and M. C. de Miranda,
et al., Cobalt-containing bioactive glass mimics vascular endothelial growth factor A and hypoxia inducible factor 1 function, J. Biomed. Mater. Res., Part A, 2021, 109(7), 1051–1064 CrossRef CAS PubMed.
- A. K. Solanki, F. V. Lali, H. Autefage, S. Agarwal, A. Nommeots-Nomm and A. D. Metcalfe,
et al., Bioactive glasses and electrospun composites that release cobalt to stimulate the HIF pathway for wound healing applications, Biomater. Res., 2021, 25(1), 1 CrossRef CAS PubMed.
- S. Jana, P. Datta, H. Das, S. Jaiswal, P. R. Ghosh and D. Lahiri,
et al., Copper and cobalt doped bioactive glass-fish dermal collagen electrospun mat triggers key events of diabetic wound healing in full-thickness skin defect model, J. Mech. Behav. Biomed. Mater., 2022, 134, 105414 CrossRef CAS PubMed.
- Z. Deng, B. Lin, Z. Jiang, W. Huang, J. Li and X. Zeng,
et al., Hypoxia-Mimicking Cobalt-Doped Borosilicate Bioactive Glass Scaffolds with Enhanced Angiogenic and Osteogenic Capacity for Bone Regeneration, Int. J. Biol. Sci., 2019, 15(6), 1113–1124 CrossRef CAS PubMed.
- V. K. Vyas, A. S. Kumar, A. Ali, S. Prasad, P. Srivastava and S. P. Mallick,
et al., Assessment of nickel oxide substituted bioactive glass-ceramic on in vitro bioactivity and mechanical properties, Bol. Soc. Esp. Ceram. Vidrio, 2016, 55(6), 228–238 CrossRef.
- V. K. Vyas, A. S. Kumar, S. P. Singh and R. Pyare, Effect of nickel oxide substitution on bioactivity and mechanical properties
of bioactive glass, Bull. Mater. Sci., 2016, 39(5), 1355–1361 CrossRef CAS.
- V. K. Vyas, A. S. Kumar, S. P. Singh and R. Pyare, Destructive and non-destructive behavior of nickel oxide doped bioactive glass and glass-ceramic, J. Aust. Ceram. Soc., 2017, 53(2), 939–951 CrossRef CAS.
- J. Han, N. Hassani Besheli, D. Deng, B. A. J. A. van Oirschot, S. C. G. Leeuwenburgh and F. Yang, Tailoring Copper-Doped Bioactive Glass/Chitosan Coatings with Angiogenic and Antibacterial Properties, Tissue Eng., Part C, 2022, 28(7), 314–324 CrossRef CAS PubMed.
- S. Huo, S. Liu, Q. Liu, E. Xie, L. Miao and X. Meng, Copper–Zinc-Doped Bilayer Bioactive Glasses Loaded Hydrogel with Spatiotemporal Immunomodulation Supports MRSA-Infected Wound Healing, Adv. Sci., 2023, 11(5) DOI:10.1002/advs.202302674.
- S. Kargozar, M. Mozafari, S. Ghodrat, E. Fiume and F. Baino, Copper-containing bioactive glasses and glass-ceramics: From tissue regeneration to cancer therapeutic strategies, Mater. Sci. Eng., C, 2021, 121, 111741 CrossRef CAS PubMed.
- G. Kohoolat, P. Alizadeh, F. Motesadi Zarandi and Y. Rezaeipour, A ternary composite hydrogel based on sodium alginate, carboxymethyl cellulose and copper-doped 58S bioactive glass promotes cutaneous wound healing in vitro and in vivo, Int. J. Biol. Macromol., 2024, 259, 129260 CrossRef CAS PubMed.
- L. Weng, S. K. Boda, M. J. Teusink, F. D. Shuler, X. Li and J. Xie, Binary Doping of Strontium and Copper Enhancing Osteogenesis and Angiogenesis of Bioactive Glass Nanofibers while Suppressing Osteoclast Activity, ACS Appl. Mater. Interfaces, 2017, 9(29), 24484–24496 CrossRef CAS PubMed.
- R. Lin, C. Deng, X. Li, Y. Liu, M. Zhang and C. Qin,
et al., Copper-incorporated bioactive glass-ceramics inducing anti-inflammatory phenotype and regeneration of cartilage/bone interface, Theranostics, 2019, 9(21), 6300–6313 CrossRef CAS PubMed.
- A. P. Kornblatt, V. G. Nicoletti and A. Travaglia, The neglected role of copper ions in wound healing, J. Inorg. Biochem., 2016, 161, 1–8 CrossRef CAS PubMed.
- V. Aina, G. Malavasi, A. Fiorio Pla, L. Munaron and C. Morterra, Zinc-containing bioactive glasses: Surface reactivity and behaviour towards endothelial cells, Acta Biomater., 2009, 5(4), 1211–1222 CrossRef CAS PubMed.
- V. Aina, A. Perardi, L. Bergandi, G. Malavasi, L. Menabue and C. Morterra,
et al., Cytotoxicity of zinc-containing bioactive glasses in contact with human osteoblasts, Chem.-Biol. Interact., 2007, 167(3), 207–218 CrossRef CAS PubMed.
- P. Balasubramanian, L. A. Strobel, U. Kneser and A. R. Boccaccini, Zinc-containing bioactive glasses for bone regeneration, dental and orthopedic applications, Biomed. Glasses, 2015, 1(1) DOI:10.1515/bglass-2015-0006.
- A. Oki, B. Parveen, S. Hossain, S. Adeniji and H. Donahue, Preparation and in vitro bioactivity of zinc containing sol-gel–derived bioglass materials, J. Biomed. Mater. Res., Part A, 2004, 69(2), 216–221 CrossRef PubMed.
- D. Kim, Y. S. Shim, S. Y. An and M. J. Lee, Role of Zinc-Doped Bioactive Glass Encapsulated with Microspherical Gelatin in Localized Supplementation for Tissue Regeneration: A Contemporary Review, Molecules, 2021, 26(7), 1823 CrossRef CAS PubMed.
- P. Thanasrisuebwong, J. R. Jones, S. Eiamboonsert, N. Ruangsawasdi, B. Jirajariyavej and P. Naruphontjirakul, Zinc-Containing Sol-Gel Glass Nanoparticles to Deliver Therapeutic Ions, Nanomaterials, 2022, 12(10), 1691 CrossRef CAS PubMed.
- I. Cacciotti, Bivalent cationic ions doped bioactive glasses: the influence of magnesium, zinc, strontium and copper on the physical and biological properties, J. Mater. Sci., 2017, 52(15), 8812–8831 CrossRef CAS.
- A. Moghanian, M. Zohourfazeli and M. H. M. Tajer, The effect of zirconium content on in vitro bioactivity, biological behavior and antibacterial activity of sol-gel derived 58S bioactive glass, J. Non-Cryst. Solids, 2020, 546, 120262 CrossRef CAS.
- M. Montazerian, B. E. Yekta, V. K. Marghussian, C. F. Bellani, R. L. Siqueira and E. D. Zanotto, Bioactivity and cell proliferation in radiopaque gel-derived CaO–P2O5–SiO2–ZrO2 glass and glass–ceramic powders, Mater. Sci. Eng., C, 2015, 55, 436–447 CrossRef CAS PubMed.
- A. Moghanian, M. Zohourfazeli, M. Haji Mahdi Tajer and A. K. Miri, Comprehensive in vitro studies of novel sol gel-derived Zr4+/Zn2+ co-substituted bioactive glass with enhanced biological properties for bone healing, J. Non-Cryst. Solids, 2021, 566, 120887 CrossRef CAS.
- M. Montazerian, G. V. S. Gonçalves, M. E. V. Barreto, E. P. N. Lima, G. R. C. Cerqueira and J. A. Sousa,
et al., Radiopaque Crystalline, Non-Crystalline and Nanostructured Bioceramics, Materials, 2022, 15(21), 7477 CrossRef CAS PubMed.
- H. Wang, S. Zhao, X. Cui, Y. Pan, W. Huang and S. Ye,
et al., Evaluation of three-dimensional silver-doped borate bioactive glass scaffolds for bone repair: Biodegradability, biocompatibility, and antibacterial activity, J. Mater. Res., 2015, 30(18), 2722–2735 CrossRef CAS.
- M. B. Taye, H. S. Ningsih and S. J. Shih, Antibacterial and In Vitro Bioactivity Studies of Silver-Doped, Cerium-Doped, and Silver–Cerium Co-Doped 80S Mesoporous Bioactive Glass Particles via Spray Pyrolysis, Appl. Sci., 2023, 13(23), 12637 CrossRef CAS.
-
M. Diba and A. R. Boccaccini, 9 - Silver-containing bioactive glasses for tissue engineering applications, in Precious Metals for Biomedical Applications, ed. N. Baltzer and T. Copponnex, Woodhead Publishing, 2014, pp. 177–211. Available from: https://www.sciencedirect.com/science/article/pii/B9780857094346500093 Search PubMed.
- R. Borges, J. S. S. Oliveira, A. P. Queiroz, T. Zambanini, A. M. Hanashiro and N. B. Lima,
et al., On the structure of Ag-containing sol-gel bioactive glasses: A surface crystal growth of metallic silver removes its network modifier role in the glass structure, Open Ceram., 2023, 16, 100449 CrossRef CAS.
- M. Lallukka, A. Houaoui, M. Miola, S. Miettinen, J. Massera and E. Verné, In vitro cytocompatibility of antibacterial silver and copper-doped bioactive glasses, Ceram. Int., 2023, 49(22, Part B), 36044–36055 CrossRef CAS.
- Z. R. Tóth, A. Feraru, D. Debreczeni, M. Todea, R. A. Popescu and T. Gyulavári,
et al., Influence of different silver species on the structure of bioactive silicate glasses, J. Non-Cryst. Solids, 2022, 583, 121498 CrossRef.
- N. Pajares, Y. Wagley, C. V. Maduka, D. W. Youngstrom, A. Yeger and S. F. Badylak,
et al., Silver-Doped Bioactive Glass Particles For In Vivo Bone Tissue Regeneration And Enhanced Methicillin-Resistant Staphilococcus Aureus (Mrsa) Inhibition, Mater. Sci. Eng., C, 2021, 120, 111693 CrossRef PubMed.
- S. Shirgill, G. Poologasundarampillai, S. Jabbari, J. Ward and S. A. Kuehne, Silver-doped bioactive glass fibres as a potential treatment for wound-associated bacterial biofilms, Biofilm, 2023, 5, 100115 CrossRef CAS PubMed.
- F. E. Ciraldo, L. Liverani, L. Gritsch, W. H. Goldmann and A. R. Boccaccini, Synthesis and Characterization of Silver-Doped Mesoporous Bioactive Glass and Its Applications in Conjunction with Electrospinning, Materials, 2018, 11(5), 692 CrossRef PubMed.
- K. Magyari, T. Nagy-Simon, A. Vulpoi, R. A. Popescu, E. Licarete and R. Stefan,
et al., Novel bioactive glass-AuNP composites for biomedical applications, Mater. Sci. Eng., C, 2017, 76, 752–759 CrossRef CAS PubMed.
- S. Grandi, V. Cassinelli, M. Bini, E. Saino, P. Mustarelli and C. R. Arciola,
et al., Bone reconstruction: Au nanocomposite bioglasses with antibacterial properties, Int. J. Artif. Organs, 2011, 34(9), 920–928 CrossRef CAS PubMed.
- G. L. da Silva, I. F. Rodrigues, S. S. S. Pereira, G. M. G. Fontoura, A. S. Reis and F. Pedrochi,
et al., Bioactive antibacterial borate glass and glass-ceramics, J. Non-Cryst. Solids, 2022, 595, 121829 CrossRef CAS.
-
S. B. Jung and D. E. Day, Wound Care Compositions Comprising Borate (B2O3) Glass-Based Particles, Columbia, MO, 9486554B2, 2016 Search PubMed.
- Phosphate and Borate Bioactive Glasses. 2022. Available from: https://pubs.rsc.org/en/content/ebook/978-1-83916-164-3.
- D. Ege, K. Zheng and A. R. Boccaccini, Borate Bioactive Glasses (BBG): Bone Regeneration, Wound Healing Applications, and Future Directions, ACS Appl. Bio Mater., 2022, 5(8), 3608–3622 CrossRef CAS PubMed.
-
S. Jung, Borate based bioactive glass scaffolds for hard and soft tissue engineering, Doctoral Dissertations, 2010. Available from: https://scholarsmine.mst.edu/doctoral_dissertations/2075 Search PubMed.
- H. Fu, Q. Fu, N. Zhou, W. Huang, M. N. Rahaman and D. Wang,
et al., In vitro evaluation of borate-based bioactive glass scaffolds prepared by a polymer foam replication method, Mater. Sci. Eng., C, 2009, 29(7), 2275–2281 CrossRef CAS.
- N. Saffarian Tousi, M. F. Velten, T. J. Bishop, K. K. Leong, N. S. Barkhordar and G. W. Marshall,
et al., Combinatorial effect of Si4+, Ca2+, and Mg2+ released from bioactive glasses on osteoblast osteocalcin expression and biomineralization, Mater. Sci. Eng., C, 2013, 33(5), 2757–2765 CrossRef CAS PubMed.
- J. Turner, A. Nandakumar, N. Anilbhai, A. R. Boccaccini, J. R. Jones and G. Jell, The effect of Si species released from bioactive glasses on cell behaviour: A quantitative review, Acta Biomater., 2023, 170, 39–52 CrossRef CAS PubMed.
- M. S. K. Mubina, S. Shailajha, R. Sankaranarayanan and S. T. Smily, Enriched biological and mechanical properties of boron doped SiO2-CaO-Na2O-P2O5 bioactive glass ceramics (BGC), J. Non-Cryst. Solids, 2021, 570, 121007 CrossRef CAS.
- N. Al-Harbi, H. Mohammed, Y. Al-Hadeethi, A. S. Bakry, A. Umar and M. A. Hussein,
et al., Silica-Based Bioactive Glasses and Their Applications in Hard Tissue Regeneration: A Review, Pharmaceuticals, 2021, 14(2), 75 CrossRef CAS PubMed.
- S. Ferraris, I. Corazzari, F. Turci, A. Cochis, L. Rimondini and E. Vernè, Antioxidant Activity of Silica-Based Bioactive Glasses, ACS Biomater. Sci. Eng., 2021, 7(6), 2309–2316 CrossRef CAS PubMed.
- K. Kajander, S. V. Sirkiä, P. K. Vallittu, T. J. Heino and J. A. Määttä, Bioactive glasses promote rapid pre-osteoblastic cell migration in contrast to hydroxyapatite, while carbonated apatite shows migration inhibiting properties, Sci. Rep., 2023, 13(1), 20587 CrossRef CAS PubMed.
- W. C. Lepry and S. N. Nazhat, A Review of Phosphate and Borate Sol–Gel Glasses for Biomedical Applications, Adv. NanoBiomed Res., 2021, 1(3), 2000055 CrossRef CAS.
- M. N. Capela, D. M. Tobaldi, C. Oliveira, A. Pereira, A. S. Duarte and M. P. Seabra,
et al., Bioactivity and antibacterial activity against E-coli of calcium-phosphate-based glasses: Effect of silver content and crystallinity, Ceram. Int., 2017, 43(16), 13800–13809 CrossRef CAS.
- L. Ma, R. K. Brow and M. E. Schlesinger, Dissolution behavior of Na2O–FeO–Fe2O3–P2O5 glasses, J. Non-Cryst. Solids, 2017, 463, 90–101 CrossRef CAS.
- B. A. Khader, O. Rodriguez and M. R. Towler, Incorporating Germanium Oxide into the Glass Phase of Novel Zinc/Magnesium-Based GPCs Designed for Bone Void Filling: Evaluating Their Physical and Mechanical Properties, J. Funct. Biomater., 2018, 9(3), 47 CrossRef CAS PubMed.
- S. Mokhtari, E. A. Krull, L. M. Sanders, A. Coughlan, N. P. Mellott and Y. Gong,
et al., Investigating the effect of germanium on the structure of SiO2-ZnO-CaO-SrO-P2O5 glasses and the subsequent influence on glass polyalkenoate cement formation, solubility and bioactivity, Mater. Sci. Eng., C, 2019, 103, 109843 CrossRef CAS PubMed.
- Y. B. Saddeek, S. A. M. Issa, E. E. A. Guclu, O. Kilicoglu, G. Susoy and H. O. Tekin, Alkaline phosphate glasses and synergistic impact of germanium oxide (GeO2) additive: Mechanical and nuclear radiation shielding behaviors, Ceram. Int., 2020, 46(10, Part B), 16781–16797 CrossRef CAS.
- M. Miola, J. Massera, A. Cochis, A. Kumar, L. Rimondini and E. Vernè, Tellurium: A new active element for innovative multifunctional bioactive glasses, Mater. Sci. Eng., C, 2021, 123, 111957 CrossRef CAS PubMed.
- G. El-Damrawi, H. Doweidar and H. Kamal, Characterization of New Categories of Bioactive Based Tellurite and Silicate Glasses, Silicon, 2017, 9(4), 503–509 CrossRef CAS.
- K. Dimitriadis, D. U. Tulyaganov and S. Agathopoulos, Development of novel alumina-containing bioactive glass-ceramics in the CaO-MgO-SiO2 system as candidates for dental implant applications, J. Eur. Ceram. Soc., 2021, 41(1), 929–940 CrossRef CAS.
- Y. Basaran Elalmis, B. Karakuzu Ikizler, S. Kilic Depren, S. Yucel and I. Aydin, Investigation of alumina doped 45S5 glass as a bioactive filler for experimental dental composites, Int. J. Appl. Glass Sci., 2021, 12(3), 313–327 CrossRef CAS.
- F. Baino, J. Minguella-Canela, F. Korkusuz, P. Korkusuz, B. Kankılıç and M.Á Montealegre,
et al., In Vitro Assessment of Bioactive Glass Coatings on Alumina/Zirconia Composite Implants for Potential Use in Prosthetic Applications, Int. J. Mol. Sci., 2019, 20(3), 722 CrossRef CAS PubMed.
- A. Zandi Karimi, E. Rezabeigi and R. A. L. Drew, Aluminum-free glass ionomer cements containing 45S5 Bioglass® and its bioglass-ceramic, J. Mater. Sci. Mater. Med., 2021, 32(7), 76 CrossRef CAS PubMed.
- H. Tripathi, A. S. Kumar and S. P. Singh, Preparation and characterization of Li2O–CaO–Al2O3–P2O5–SiO2 glasses as bioactive material, Bull. Mater. Sci., 2016, 39(2), 365–376 CrossRef CAS.
- F. Kurtuldu, N. Mutlu, A. R. Boccaccini and D. Galusek, Gallium containing bioactive materials: A review of anticancer, antibacterial, and osteogenic properties, Bioact. Mater., 2022, 17, 125–146 CAS.
- F. Kurtuldu, N. Mutlu, M. Michálek, K. Zheng, M. Masar and L. Liverani,
et al., Cerium and gallium containing mesoporous bioactive glass nanoparticles for bone regeneration: Bioactivity, biocompatibility and antibacterial activity, Mater. Sci. Eng., C, 2021, 124, 112050 CrossRef CAS PubMed.
- K. S. Rana, L. d. Souza, M. A. Isaacs, F. N. S. Raja, A. P. Morrell and R. A. Martin, Development and Characterization of Gallium-Doped Bioactive Glasses for Potential Bone Cancer Applications, ACS Biomater. Sci. Eng., 2017, 3(12), 3425–3432 CrossRef CAS PubMed.
- S. Pourshahrestani, E. Zeimaran, N. A. Kadri, N. Gargiulo, S. Samuel and S. V. Naveen,
et al., Gallium-containing mesoporous bioactive glass with potent hemostatic activity and antibacterial efficacy, J. Mater. Chem. B, 2015, 4(1), 71–86 RSC.
- L. Souza, F. V. Ferreira, J. H. Lopes, J. A. Camilli and R. A. Martin, Cancer Inhibition and In Vivo Osteointegration and Compatibility of Gallium-Doped Bioactive Glasses for Osteosarcoma Applications, ACS Appl. Mater. Interfaces, 2022, 14(40), 45156–45166 CrossRef CAS PubMed.
- S. Shruti, F. Andreatta, E. Furlani, E. Marin, S. Maschio and L. Fedrizzi, Cerium, gallium and zinc containing mesoporous bioactive glass coating deposited on titanium alloy, Appl. Surf. Sci., 2016, 378, 216–223 CrossRef CAS.
- L. Wang, N. J. Long, L. Li, Y. Lu, M. Li and J. Cao,
et al., Multi-functional bismuth-doped bioglasses: combining bioactivity and photothermal response for bone tumor treatment and tissue repair, Light: Sci. Appl., 2018, 7(1), 1 CrossRef PubMed.
- S. S. Prasad, I. Ratha, T. Adarsh, A. Anand, P. K. Sinha and P. Diwan,
et al., In vitro bioactivity and antibacterial properties of bismuth oxide modified bioactive glasses, J. Mater. Res., 2018, 33(2), 178–190 CrossRef.
- C. Khatua, S. Bodhak, B. Kundu and V. K. Balla, In vitro bioactivity and bone mineralization of bismuth ferrite reinforced bioactive glass composites, Materialia, 2018, 4, 361–366 CrossRef CAS.
- H. A. El Batal, E. M. Abou Hussein, N. A. El Alaily and F. M. EzzEldin, Effect of different 3d transition metal oxides on some physical properties of γ-Irradiated Bi2O3-B2O3 glasses: A comparative study, J. Non-Cryst. Solids, 2020, 528, 119733 CrossRef CAS.
- A. M. Deliormanlı, S. Oguzlar and K. Ertekin, Photoluminescence and decay characteristics of cerium, gallium and vanadium - containing borate-based bioactive glass powders for bioimaging applications, Ceram. Int., 2021, 47(3), 3797–3807 CrossRef.
- A. Zambon, G. Malavasi, A. Pallini, F. Fraulini and G. Lusvardi, Cerium Containing Bioactive Glasses: A Review, ACS Biomater. Sci. Eng., 2021, 7(9), 4388 CrossRef CAS PubMed.
- G. Lusvardi, F. Fraulini, S. D'Addato and A. Zambon, Loading with Biomolecules Modulates the Antioxidant Activity of Cerium-Doped Bioactive Glasses, ACS Biomater. Sci. Eng., 2022, 8(7), 2890–2898 CrossRef CAS PubMed.
- C. Leonelli, G. Lusvardi, G. Malavasi, L. Menabue and M. Tonelli, Synthesis and characterization of cerium-doped glasses and in vitro evaluation of bioactivity, J. Non-Cryst. Solids, 2003, 316(2), 198–216 CrossRef CAS.
- C. Leonelli, G. Lusvardi, L. Menabue and M. Tonelli, Preliminary Experiments of In Situ Atomic Force Microscopy Observation of Hydroxyapatite Formation on Bioactive Glass Surface, J. Am. Ceram. Soc., 2002, 85(2), 487–489 CrossRef CAS.
- G. Göller and I. Akin, Effect of CeO2 Addition on In Vitro Bioactivity Properties of K-Mica-Fluorapatite Based Glass Ceramics, Key Eng. Mater., 2008, 361–363, 261–264 Search PubMed.
- A. J. Salinas, S. Shruti, G. Malavasi, L. Menabue and M. Vallet-Regí, Substitutions of cerium, gallium and zinc in ordered mesoporous bioactive glasses, Acta Biomater., 2011, 7(9), 3452–3458 CrossRef CAS PubMed.
- M. Ershad, A. Ali, N. S. Mehta, R. K. Singh, S. K. Singh and R. Pyare, Mechanical and biological response of (CeO2 + La2O3)-substituted 45S5 bioactive glasses for biomedical application, J. Aust. Ceram. Soc., 2020, 56(4), 1243–1252 CrossRef.
- C. Wu, L. Xia, P. Han, L. Mao, J. Wang and D. Zhai,
et al., Europium-Containing Mesoporous Bioactive Glass Scaffolds for Stimulating in Vitro and in Vivo Osteogenesis, ACS Appl. Mater. Interfaces, 2016, 8(18), 11342–11354 CrossRef CAS PubMed.
- T. Zambanini, R. Borges, P. C. Faria, G. P. Delpino, I. S. Pereira and M. M. Marques,
et al., Dissolution, bioactivity behavior, and cytotoxicity of rare earth-containing bioactive glasses (RE = Gd, Yb), Int. J. Appl. Ceram. Technol., 2019, 16(5), 2028–2039 CrossRef CAS.
- J. Liao, R. Han, Y. Wu and Z. Qian, Review of a new bone tumor therapy strategy based on bifunctional biomaterials, Bone Res., 2021, 9(1), 1–13 CrossRef PubMed.
- F. Liao, X. Y. Peng, F. Yang, Q. F. Ke, Z. H. Zhu and Y. P. Guo, Gadolinium-doped mesoporous calcium silicate/chitosan scaffolds enhanced bone regeneration ability, Mater. Sci. Eng., C, 2019, 104, 109999 CrossRef PubMed.
- D. Y. Zhu, B. Lu, J. H. Yin, Q. F. Ke, H. Xu and C. Q. Zhang,
et al., Gadolinium-doped bioglass scaffolds promote osteogenic differentiation of hBMSC via the Akt/GSK3β pathway and facilitate bone repair in vivo, Int. J. Nanomed., 2019, 14, 1085–1100 CrossRef CAS PubMed.
- R. Borges, J. F. Schneider and J. Marchi, Structural characterization of bioactive glasses containing rare earth elements (Gd and/or Yb), J. Mater. Sci., 2019, 54(17), 11390–11399 CrossRef CAS.
- M. Ershad, V. K. Vyas, S. Prasad, A. Ali and R. Pyare, Effect of Sm2O3 substitution on mechanical and biological properties of 45S5 bioactive glass, J. Aust. Ceram. Soc., 2018, 54(4), 621–630 CrossRef CAS.
-
A. Baranowska, M. Kochanowicz, J. Żmojda, P. Miluski, A. Wajda and M. Leśniak, et al., Biological properties of rare-earth doped bioactive glass, in Optical Fibers and Their Applications 2020, SPIE, 2020, pp. 12–17. Available from: https://www.spiedigitallibrary.org/conference-proceedings-of-spie/11456/1145604/Biological-properties-of-rare-earth-doped-bioactive-glass/10.1117/12.2566347.full Search PubMed.
- Y. Zhang, X. Wang, Y. Su, D. Chen and W. Zhong, A doxorubicin delivery system: Samarium/mesoporous bioactive glass/alginate composite microspheres, Mater. Sci. Eng., C, 2016, 67, 205–213 CrossRef CAS PubMed.
-
A. Baranowska, J. R. Dąbrowski, M. Kochanowicz and J. Dorosz, Effect of biodegradation on spectroscopic properties of Sm3+ doped 45S5 bioglass, in Photonics Applications in Astronomy, Communications, Industry, and High-Energy Physics Experiments 2018, SPIE, 2018, pp. 942–947. Available from: https://www.spiedigitallibrary.org/conference-proceedings-of-spie/10808/1080833/Effect-of-biodegradation-on-spectroscopic-properties-of-Sm3-doped-45S5/10.1117/12.2500274.full Search PubMed.
- M. J. Page and E. Di Cera, Role of Na+ and K+ in Enzyme Function, Physiol. Rev., 2006, 86(4), 1049–1092 CrossRef CAS PubMed.
- J. K. Rybakowski, Lithium in neuropsychiatry: a 2010 update, World J. Biol. Psychiatry, 2011, 12(5), 340–348 CrossRef PubMed.
-
K. Chokhawala, S. Lee and A. Saadabadi, Lithium, in StatPearls, StatPearls Publishing, Treasure Island, FL, 2022. Available from: https://www.ncbi.nlm.nih.gov/books/NBK519062/ Search PubMed.
- E. Jakobsson, O. Argüello-Miranda, S. W. Chiu, Z. Fazal, J. Kruczek and S. Nunez-Corrales,
et al., Towards a Unified Understanding of Lithium Action in Basic Biology and its Significance for Applied Biology, J. Membr. Biol., 2017, 250(6), 587–604 CrossRef CAS PubMed.
- D. Szklarska and P. Rzymski, Is Lithium a Micronutrient? From Biological Activity and Epidemiological Observation to Food Fortification, Biol. Trace Elem. Res., 2019, 189(1), 18–27 CrossRef CAS PubMed.
- E. Won and Y. K. Kim, An Oldie but Goodie: Lithium in the Treatment of Bipolar Disorder through Neuroprotective and Neurotrophic Mechanisms, Int. J. Mol. Sci., 2017, 18(12), 2679 CrossRef PubMed.
- G. S. Malhi, M. Tanious, P. Das, C. M. Coulston and M. Berk, Potential mechanisms of action of lithium in bipolar disorder. Current understanding, CNS Drugs, 2013, 27(2), 135–153 CrossRef PubMed.
- Review of Lithium Effects on Brain and Blood - Wise Young, 2009. Available from: https://journals.sagepub.com/doi/10.3727/096368909X471251?url_ver=Z39.88-2003&rfr_id=ori:rid:crossref.org&rfr_dat=cr_pub%20%200pubmed.
- Granulocytopenia - PubMed. [cited 2023 Jan 2]. Available from: https://pubmed.ncbi.nlm.nih.gov/30193521/.
- Lithium carbonate therapy for granulocytopenia in a patient with myelofibrosis and septic arthritis - PubMed. [cited 2023 Jan 2]. Available from: https://pubmed.ncbi.nlm.nih.gov/515774/.
- Effects of short-term lithium treatment on peripheral blood lymphocytes and granulocytes in healthy volunteers - PubMed. [cited 2023 Jan 2]. Available from: https://pubmed.ncbi.nlm.nih.gov/6359361/.
- R. Pazdur and A. H. Rossof, Cytotoxic chemotherapy for cancer in Felty's syndrome: role of lithium carbonate, Blood, 1981, 58(3), 440–443 CrossRef CAS PubMed.
- S. Guo, K. Arai, M. F. Stins, D. M. Chuang and E. H. Lo, Lithium Upregulates Vascular Endothelial Growth Factor in Brain Endothelial Cells and Astrocytes, Stroke, 2009, 40(2), 652–655 CrossRef CAS PubMed.
- The vascular endothelial growth factor (VEGF) family: angiogenic factors in health and disease | Genome Biology | Full Text. [cited 2023 Jan 2]. Available from: https://genomebiology.biomedcentral.com/articles/10.1186/gb-2005-6-2-209.
- H. Dong, X. Zhang, X. Dai, S. Lu, B. Gui and W. Jin,
et al., Lithium ameliorates lipopolysaccharide-induced microglial activation via inhibition of toll-like receptor 4 expression
by activating the PI3K/Akt/FoxO1 pathway, J. Neuroinflammation, 2014, 11(1), 140 CrossRef PubMed.
- Axon-Schwann cell interactions regulate the expression of c-jun in Schwann cells - PubMed. [cited 2023 Jan 2]. Available from: https://pubmed.ncbi.nlm.nih.gov/8833086/.
- H. K. Manji, G. J. Moore and G. Chen, Lithium up-regulates the cytoprotective protein Bcl-2 in the CNS in vivo: a role for neurotrophic and neuroprotective effects in manic depressive illness, J. Clin. Psychol., 2000, 61(Suppl 9), 82–96 CAS.
- Transplantation of a Peripheral Nerve with Neural Stem Cells Plus Lithium Chloride Injection Promote the Recovery of Rat Spinal Cord Injury - PubMed. [cited 2023 Jan 2]. Available from: https://pubmed.ncbi.nlm.nih.gov/29756516/.
- H. Eldar-Finkelman and A. Martinez, GSK-3 Inhibitors: Preclinical and Clinical Focus on CNS, Front. Mol. Neurosci., 2011, 4, 32 CAS.
- N. J. Birch, Lithium And Magnesium-Dependent Enzymes, Lancet, 1974, 304(7886), 965–966 CrossRef PubMed.
- J. D. Meisel and D. H. Kim, Inhibition of Lithium-Sensitive Phosphatase BPNT-1 Causes Selective Neuronal Dysfunction in C. elegans, Curr. Biol., 2016, 26(14), 1922–1928 CrossRef CAS PubMed.
- T. Yoshikawa and S. Honma, Lithium lengthens circadian period of cultured brain slices in area specific manner, Behav. Brain Res., 2016, 314, 30–37 CrossRef CAS PubMed.
- A. M. Van Alphen, T. M. Bosch, R. W. Kupka and R. Hoekstra, Chronic kidney disease in lithium-treated patients, incidence and rate of decline, Int. J. Bipolar Disord., 2021, 9, 1 CrossRef CAS PubMed.
- B. K. Kishore and C. M. Ecelbarger, Lithium: a versatile tool for understanding renal physiology, Am. J. Physiol.: Renal Physiol., 2013, 304(9), F1139–F1149 CrossRef CAS PubMed.
- What we need to know about the effect of lithium on the kidney - PubMed. Available from: https://pubmed.ncbi.nlm.nih.gov/27122541/.
- S. Naramala, H. Dalal, S. Adapa, A. Hassan and V. M. Konala, Lithium-induced Hyperparathyroidism and Hypercalcemia, Cureus, 2019, 11(5) DOI:10.7759/2Fcureus.4590.
- N. Mehta and R. Vannozzi, Lithium-induced electrocardiographic changes: A complete review, Clin. Cardiol., 2017, 40(12), 1363–1367 CrossRef PubMed.
- P. K. Khan, A. Mahato, B. Kundu, S. K. Nandi, P. Mukherjee and S. Datta,
et al., Influence of single and binary doping of strontium and lithium on in vivo biological properties of bioactive glass scaffolds, Sci. Rep., 2016, 6(1), 32964 CrossRef CAS PubMed.
- L. A. Haro Durand, G. E. Vargas, R. Vera-Mesones, A. Baldi, M. P. Zago and M. A. Fanovich,
et al., In Vitro Human Umbilical Vein Endothelial Cells Response to Ionic Dissolution Products from Lithium-Containing 45S5 Bioactive Glass, Materials, 2017, 10(7), 740 CrossRef PubMed.
- L. Liu, Y. Liu, C. Feng, J. Chang, R. Fu and T. Wu,
et al., Lithium-containing biomaterials stimulate bone marrow stromal cell-derived exosomal miR-130a secretion to promote angiogenesis, Biomaterials, 2019, 192, 523–536 CrossRef CAS PubMed.
- A. Leaf, The Clinical and Physiologic Significance of the Serum Sodium Concentration, N. Engl. J. Med., 1962, 267(2), 77–83 CrossRef CAS PubMed.
- P. Strazzullo and C. Leclercq, Sodium1, Adv. Nutr., 2014, 5(2), 188–190 CrossRef PubMed.
- A. Grillo, L. Salvi, P. Coruzzi, P. Salvi and G. Parati, Sodium Intake and Hypertension, Nutrients, 2019, 11(9), 1970 CrossRef CAS PubMed.
-
J. H. Fountain and S. L. Lappin, Physiology, Renin Angiotensin System, in StatPearls, StatPearls Publishing, Treasure Island, FL, 2022. Available from: https://www.ncbi.nlm.nih.gov/books/NBK470410/ Search PubMed.
-
S. M. Chrysafides, S. J. Bordes and S. Sharma, Physiology, Resting Potential, in StatPearls, StatPearls Publishing, Treasure Island, FL, 2022. Available from: https://www.ncbi.nlm.nih.gov/books/NBK538338/ Search PubMed.
-
Y. Pirahanchi, R. Jessu and N. R. Aeddula, Physiology, Sodium Potassium Pump, in StatPearls, StatPearls Publishing, Treasure Island,
FL, 2022. Available from: https://www.ncbi.nlm.nih.gov/books/NBK537088/ Search PubMed.
- K. Jomova, M. Makova, S. Y. Alomar, S. H. Alwasel, E. Nepovimova and K. Kuca,
et al., Essential metals in health and disease, Chem.-Biol. Interact., 2022, 367, 110173 CrossRef CAS PubMed.
- L. Aguilar-Bryan and J. Bryan, Molecular biology of adenosine triphosphate-sensitive potassium channels, Endocr. Rev., 1999, 20, 101–135 CAS.
- A. S. Pivovarov, F. Calahorro and R. J. Walker, Na+/K+-pump and neurotransmitter membrane receptors, Invertebr. Neurosci., 2018, 19(1), 1 Search PubMed.
- K. B. Gagnon and E. Delpire, Sodium Transporters in Human Health and Disease, Front. Physiol., 2021, 11, 588664 CrossRef PubMed.
- S. Prasad, A. M. Cantwell, L. A. Bush, P. Shih, H. Xu and E. Di Cera, Residue Asp-189 controls both substrate binding and the monovalent cation specificity of thrombin, J. Biol. Chem., 2004, 279(11), 10103–10108 CrossRef CAS PubMed.
- M. Moustakas, The Role of Metal Ions in Biology, Biochemistry and Medicine, Materials, 2021, 14(3), 549 CrossRef CAS PubMed.
- A. N. Desai, High Blood Pressure, J. Am. Med. Assoc., 2020, 324(12), 1254–1255 CrossRef PubMed.
- L. Shahrin, M. J. Chisti, S. Huq, T. Nishath, M. D. Christy and A. Hannan,
et al., Clinical Manifestations of Hyponatremia and Hypernatremia in Under-Five Diarrheal Children in a Diarrhea Hospital, J. Trop. Pediatr., 2016, 62(3), 206–212 CrossRef PubMed.
- S. Thomas, Alginate dressings in surgery and wound management–Part 1, J. Wound Care, 2000, 9(2), 56–60 CrossRef CAS PubMed.
- V. Jones, J. E. Grey and K. G. Harding, Wound dressings, Br. Med. J., 2006, 332(7544), 777–780 CrossRef PubMed.
-
M. H. Grider, R. Jessu and R. Kabir, Physiology, Action Potential, in StatPearls, StatPearls Publishing, Treasure Island, FL, 2022. Available from: https://www.ncbi.nlm.nih.gov/books/NBK538143/ Search PubMed.
- Cardiac transmembrane ion channels and action potentials: cellular physiology and arrhythmogenic behavior | Physiological Reviews. Available from: https://journals.physiology.org/doi/full/10.1152/physrev.00024.2019.
- M. C. O'Brien and D. B. McKay, How potassium affects the activity of the molecular chaperone Hsc70. I. Potassium is required for optimal ATPase activity, J. Biol. Chem., 1995, 270(5), 2247–2250 CrossRef PubMed.
- R. D. Toto, Serum Potassium and Cardiovascular Outcomes: The Highs and the Lows, Clin. J. Am. Soc. Nephrol., 2017, 12(2), 220–221 CrossRef PubMed.
-
L. V. Simon, M. F. Hashmi and M. W. Farrell, Hyperkalemia, in StatPearls, StatPearls Publishing, Treasure Island, FL, 2022. Available from: https://www.ncbi.nlm.nih.gov/books/NBK470284/ Search PubMed.
- R. W. Hunter and M. A. Bailey, Hyperkalemia: pathophysiology, risk factors and consequences, Nephrol., Dial., Transplant., 2019, 34(Suppl 3iii), 2–11 CrossRef PubMed.
- M. A. Abbassy, A. S. Bakry, R. Hill and A. Habib Hassan, Fluoride bioactive glass paste improves bond durability and remineralizes tooth structure prior to adhesive restoration, Dent. Mater., 2021, 37(1), 71–80 CrossRef CAS PubMed.
-
V. V. Kupriyanov, Rubidium in Biological Systems and Medicine, in Encyclopedia of Metalloproteins, ed. R.H Kretsinger, V.N Uversky and E.A Permyakov, Springer, New York, NY, 2013, pp. 1851–1856. DOI:10.1007/978-1-4614-1533-6_331.
- J. F. Chatal, F. Rouzet, F. Haddad, C. Bourdeau, C. Mathieu and D. Le Guludec, Story of Rubidium-82 and Advantages for Myocardial Perfusion PET Imaging, Front. Med., 2015, 2, 65 Search PubMed.
- G. Placidi, A. Lenzi, F. Lazzerini, L. Dell'Osso, G. B. Cassano and H. S. Akiskal, Exploration of the clinical profile of rubidium chloride in depression: a systematic open trial, J. Clin. Psychopharmacol., 1988, 8(3), 184–188 CrossRef CAS PubMed.
- R. Torta, G. Ala, R. Borio, A. Cicolin, S. Costamagna and L. Fiori,
et al., Rubidium chloride in the treatment of major depression, Minerva Psichiatr., 1993, 34(2), 101–110 CAS.
- N. Kordjazy, A. Haj-Mirzaian, S. Amiri, S. Ostadhadi, M. Kordjazy and M. Sharifzadeh,
et al., Elevated level of nitric oxide mediates the anti-depressant effect of rubidium chloride in mice, Eur. J. Pharmacol., 2015, 762, 411–418 CrossRef CAS PubMed.
- Z. Ouyang, Q. Huang, B. Liu, H. Wu, T. Liu and Y. Liu, Rubidium Chloride Targets Jnk/p38-Mediated NF-κB Activation to Attenuate Osteoclastogenesis and Facilitate Osteoblastogenesis, Front. Pharmacol., 2019, 10, 584 CrossRef CAS PubMed.
- B. F. Boyce, Z. Yao and L. Xing, Functions of NF-κB in Bone, Ann. N. Y. Acad. Sci., 2010, 1192, 367–375 CrossRef CAS PubMed.
- R. R. Fieve and K. R. Jamison, Rubidium: overview and clinical perspectives, Mod. Probl. Pharmacopsychiatry, 1982, 18, 145–163 CAS.
- M. Chen, S. Wu, Y. Tan, R. Li, Y. Liu and Q. Huang, Rubidium-doped titanium surfaces with modulatory effects on MC3T3-E1 cell response and antibacterial capacity against Staphylococcus aureus, Biomed. Mater., 2019, 14(4), 045016 CrossRef CAS PubMed.
- Y. Liu, Y. Tan and J. Wu, Rubidium doped nano-hydroxyapatite with cytocompatibility and antibacterial, J. Asian Ceram. Soc., 2021, 9(1), 323–333 CrossRef.
- Y. N. Tan, W. J. Chen, W. Wei, Q. L. Huang and X. He, Rubidium-modified bioactive glass-ceramics with hydroxyapatite crystals for bone regeneration, Trans. Nonferrous Met. Soc. China, 2021, 31(2), 521–532 CrossRef CAS.
- J. Doucet, K. MacDonald, C. Lee, R. A. Hana, G. Soulez and D. Boyd, The feasibility of degradable glass microspheres as transient embolic medical devices, J. Biomater. Appl., 2021, 35(6), 615–632 CrossRef CAS PubMed.
- High-Energy Radiography (Cobalt 60 and Cesium 137) for Tumor Localization and Treatment Planning | Radiology. [cited 2023 Jan 7]. Available from: https://pubs.rsna.org/doi/10.1148/78.2.260.
- W. C. Chen, M. Lafreniere, C. Phuong, S. J. Liu, J. D. Baal, M. Lometti, O. Morin, B. Ziemer, H. N. Vasudevan, C. G. Lucas, S. L. Hervey-Jumper, P. V. Theodosopoulos, S. T. Magill, S. Fogh, J. L. Nakamura, L. Boreta, P. K Sneed, M. W. McDermott, D. R. Raleigh and S. E. Braunstein, Resection with intraoperative cesium-131 brachytherapy as salvage therapy for recurrent brain tumors, J. Neurosurg., 2022, 137(4), 924–930 CAS.
- S. Venturi, Cesium in Biology, Pancreatic Cancer, and Controversy in High and Low Radiation Exposure Damage—Scientific, Environmental, Geopolitical, and Economic Aspects, Int. J. Environ. Res. Public Health, 2021, 18(17), 8934 CrossRef CAS PubMed.
- A. K. Brewer, The high pH therapy for cancer tests on mice and humans, Pharmacol., Biochem. Behav., 1984, 21(Suppl 1), 1–5 CrossRef PubMed.
- P. Melnikov and L. Z. Zanoni, Clinical Effects of Cesium Intake, Biol. Trace Elem. Res., 2010, 135(1), 1–9 CrossRef CAS PubMed.
- D. Sessions, K. Heard and M. Kosnett, Fatal Cesium Chloride Toxicity After Alternative Cancer Treatment, J. Altern. Complementary Med., 2013, 19(12), 973–975 CrossRef PubMed.
- R. G. Cooper and A. P. Harrison, The uses and adverse effects of beryllium on health, Indian J. Occup. Environ. Med., 2009, 13(2), 65–76 CrossRef PubMed.
- D. Fiorentini, C. Cappadone, G. Farruggia and C. Prata, Magnesium: Biochemistry, Nutrition, Detection, and Social Impact of Diseases Linked to Its Deficiency, Nutrients, 2021, 13(4), 1136 CrossRef CAS PubMed.
-
J. Weston, Biochemistry of Magnesium, in PATAI'S Chemistry of Functional Groups, John Wiley & Sons, Ltd, 2009. Available from: https://onlinelibrary.wiley.com/doi/abs/10.1002/9780470682531.pat0407 Search PubMed.
- Magnesium in Man: Implications for Health and Disease | Physiological Reviews. [cited 2023 Jan 11]. Available from: https://journals.physiology.org/doi/full/10.1152/physrev.00012.2014?rfr_dat=cr_pub++0pubmed&url_ver=Z39.88-2003&rfr_id=ori%3Arid%3Acrossref.org.
-
J. Dunn and M. H. Grider, Physiology, Adenosine Triphosphate, in StatPearls, StatPearls Publishing, Treasure Island, FL, 2022. Available from: https://www.ncbi.nlm.nih.gov/books/NBK553175/ Search PubMed.
- B. S. Khakh and G. Burnstock, The Double Life of ATP, Sci. Am., 2009, 301(6), 84–92 CrossRef CAS PubMed.
- A. Hartwig, Role of magnesium in genomic stability, Mutat. Res., Fundam. Mol. Mech. Mutagen., 2001, 475(1), 113–121 CrossRef CAS PubMed.
- J. Anastassopoulou and T. Theophanides, Magnesium-DNA interactions and the possible relation of magnesium to carcinogenesis. Irradiation and free radicals, Crit. Rev. Oncol. Hematol., 2002, 42(1), 79–91 CrossRef CAS PubMed.
- J. W. Seo and T. J. Park, Magnesium Metabolism, Electrolytes Blood Pressure, 2008, 6(2), 86–95 CrossRef CAS PubMed.
- S. Castiglioni, A. Cazzaniga, W. Albisetti and J. A. M. Maier, Magnesium and Osteoporosis: Current State of Knowledge and Future Research Directions, Nutrients, 2013, 5(8), 3022–3033 CrossRef CAS PubMed.
- W. C. Lu, E. Pringa and L. Chou, Effect of magnesium on the osteogenesis of normal human osteoblasts, Magnes. Res., 2017, 30(2), 42–52 CAS.
- M. Wang, M. Tashiro and J. R. Berlin, Regulation of L-type calcium current by intracellular magnesium in rat cardiac myocytes, J. Physiol., 2004, 555(Pt 2), 383–396 CrossRef CAS PubMed.
-
B. Cohagan and D. Brandis, Torsade de Pointes, in StatPearls, StatPearls Publishing, Treasure Island, FL, 2022. Available from: https://www.ncbi.nlm.nih.gov/books/NBK459388/ Search PubMed.
- S. Zhu, R. A. Stein, C. Yoshioka, C. H. Lee, A. Goehring and H. S. Mchaourab,
et al., Mechanism of NMDA Receptor Inhibition and Activation, Cell, 2016, 165(3), 704–714 CrossRef CAS PubMed.
- P. C. T. Pham, P. A. T. Pham, S. V. Pham, P. T. T. Pham, P. M. T. Pham and P. T. T. Pham, Hypomagnesemia: a clinical perspective, Int. J. Nephrol. Renovasc. Dis., 2014, 7, 219–230 CrossRef PubMed.
-
M. Cascella and S. Vaqar, Hypermagnesemia, in StatPearls, StatPearls Publishing, Treasure Island, FL, 2022. Available from: https://www.ncbi.nlm.nih.gov/books/NBK549811/ Search PubMed.
- H. Yamaguchi, H. Shimada, K. Yoshita, Y. Tsubata, K. Ikarashi and T. Morioka,
et al., Severe hypermagnesemia induced by magnesium oxide ingestion: a case series, CEN Case Rep., 2018, 8(1), 31–37 CrossRef PubMed.
- A. Moghanian, A. Sedghi, A. Ghorbanoghli and E. Salari, The effect of magnesium content on in vitro bioactivity, biological behavior and antibacterial activity of sol–gel derived 58S bioactive glass, Ceram. Int., 2018, 44(8), 9422–9432 CrossRef CAS.
- S. Kargozar, P. B. Milan, M. Amoupour, F. Kermani, S. Gorgani and S. Nazarnezhad,
et al., Osteogenic Potential of Magnesium (Mg)-Doped Multicomponent Bioactive Glass: In Vitro and In Vivo Animal Studies, Materials, 2022, 15(1), 318 CrossRef CAS PubMed.
- M. Liu, X. Wang, J. Cui, H. Wang, B. Sun and J. Zhang,
et al., Electrospun flexible magnesium-doped silica bioactive glass nanofiber membranes with anti-inflammatory and pro-angiogenic effects for infected wounds, J. Mater. Chem. B, 2023, 11(2), 359–376 RSC.
- F. Bronner, Extracellular and Intracellular Regulation of Calcium Homeostasis, Sci. World J., 2001, 1, 919–925 CrossRef CAS PubMed.
- A. C. Newton, M. D. Bootman and J. D. Scott, Second Messengers, Cold Spring Harbor Perspect. Biol., 2016, 8(8), a005926 CrossRef PubMed.
- M. J. Berridge, The Inositol Trisphosphate/Calcium Signaling Pathway in Health and Disease, Physiol. Rev., 2016, 96(4), 1261–1296 CrossRef CAS PubMed.
- E. Kania, G. Roest, T. Vervliet, J. B. Parys and G. Bultynck, IP3 Receptor-Mediated Calcium Signaling and Its Role in Autophagy in Cancer, Front. Oncol., 2017, 7, 140 CrossRef PubMed.
- Z. Song, Y. Wang, F. Zhang, F. Yao and C. Sun, Calcium Signaling Pathways: Key Pathways in the Regulation of Obesity, Int. J. Mol. Sci., 2019, 20(11), 2768 CrossRef CAS PubMed.
- I. Y. Kuo and B. E. Ehrlich, Signaling in Muscle Contraction, Cold Spring Harbor Perspect. Biol., 2015, 7(2), a006023 CrossRef PubMed.
-
R. Chaudhry, S. M. Usama and H. M. Babiker, Physiology, Coagulation Pathways, in StatPearls, StatPearls Publishing, Treasure Island, FL, 2022. Available from: https://www.ncbi.nlm.nih.gov/books/NBK482253/ Search PubMed.
- S. Palta, R. Saroa and A. Palta, Overview of the coagulation system, Indian J. Anaesth., 2014, 58(5), 515–523 CrossRef CAS PubMed.
- R. Kumar, Physiology, Coagulation Cascade: Inherited Disorders, and the Molecular Phenomenon of Alterations in Hemostasis, J. Clin. Haematol., 2021, 2(2), 62–64 Search PubMed.
- B. Ahvazi and P. M. Steinert, A model for the reaction mechanism of the transglutaminase 3 enzyme, Exp. Mol. Med., 2003, 35(4), 228–242 CrossRef CAS PubMed.
- K. Hitomi, Transglutaminases in skin epidermis, Eur. J. Dermatol., 2005, 15(5), 313–319 CAS.
- R. L. Eckert, M. T. Kaartinen, M. Nurminskaya, A. M. Belkin, G. Colak and G. V. W. Johnson,
et al., Transglutaminase Regulation of Cell Function, Physiol. Rev., 2014, 94(2), 383–417 CrossRef CAS PubMed.
- D. Aeschlimann, D. Mosher and M. Paulsson, Tissue transglutaminase and factor XIII in cartilage and bone remodeling, Semin. Thromb. Hemost., 1996, 22(5), 437–443 CrossRef CAS PubMed.
- B. Engelmann and S. Massberg, Thrombosis as an intravascular effector of innate immunity, Nat. Rev. Immunol., 2013, 13(1), 34–45 CrossRef CAS PubMed.
- A. O. Grant, Cardiac Ion Channels, Circ.: Arrhythmia Electrophysiol., 2009, 2(2), 185–194 Search PubMed.
- K. Shah, S. Seeley, C. Schulz, J. Fisher and S. Gururaja Rao, Calcium Channels in the Heart: Disease States and Drugs, Cells, 2022, 11(6), 943 CrossRef CAS PubMed.
- C. J. Fearnley, H. L. Roderick and M. D. Bootman, Calcium Signaling in Cardiac Myocytes, Cold Spring Harbor Perspect. Biol., 2011, 3(11), a004242 Search PubMed.
- T. C. Südhof, Calcium Control of Neurotransmitter Release, Cold Spring Harbor Perspect. Biol., 2012, 4(1), a011353 Search PubMed.
- L. Vannucci, C. Fossi, S. Quattrini, L. Guasti, B. Pampaloni and G. Gronchi,
et al., Calcium Intake in Bone Health: A Focus on Calcium-Rich Mineral Waters, Nutrients, 2018, 10(12), 1930 CrossRef PubMed.
- F. W. Wehrli, Structural and functional assessment of trabecular and cortical bone by micro magnetic resonance imaging, J. Magn. Reson. Imaging, 2007, 25(2), 390–409 CrossRef PubMed.
- X. Kesse, C. Vichery, A. Jacobs, S. Descamps and J. M. Nedelec, Unravelling the Impact of Calcium Content on the Bioactivity of Sol–Gel-Derived Bioactive Glass Nanoparticles, ACS Appl. Bio Mater., 2020, 3(2), 1312–1320 CrossRef CAS PubMed.
- M. Catauro, F. Papale, L. Sapio and S. Naviglio, Biological influence of Ca/P ratio on calcium phosphate coatings by sol-gel processing, Mater. Sci. Eng., C, 2016, 65, 188–193 CrossRef CAS PubMed.
- S. Kargozar, F. Baino, S. Hamzehlou, R. G. Hill and M. Mozafari, Bioactive Glasses: Sprouting Angiogenesis in Tissue Engineering, Trends Biotechnol., 2018, 36(4), 430–444 CrossRef CAS PubMed.
- L. L. Hench, Genetic design of bioactive glass, J. Eur. Ceram. Soc., 2009, 29(7), 1257–1265 CrossRef CAS.
- P. M. Quilty, D. Kirk, J. J. Bolger, D. P. Dearnaley, V. J. Lewington and M. D. Masone,
et al., A comparison of the palliative effects of strontium-89 and external beam radiotherapy in metastatic prostate cancer, Radiother. Oncol., 1994, 31(1), 33–40 CrossRef CAS PubMed.
- G. S. Hahn, Strontium is a potent and selective inhibitor of sensory irritation, Dermatol. Surg., 1999, 25(9), 689–694 CrossRef CAS PubMed.
- L. E. Pelepenko, M. A. Marciano, T. M. Francati, G. Bombarda, T. Bessa Marconato Antunes and F. Sorrentino,
et al., Can strontium replace calcium in bioactive materials for dental applications?, J. Biomed. Mater. Res., Part A, 2022, 110(12), 1892–1911 CrossRef CAS PubMed.
- B. F. A. Karim and D. G. Gillam, The Efficacy of Strontium and Potassium Toothpastes in Treating Dentine Hypersensitivity: A Systematic Review, Int. J. Dent., 2013, 2013, e573258 Search PubMed.
- R. V. Thakker, Calcium-sensing receptor: Role in health and disease, Indian J. Endocrinol. Metab., 2012, 16(Suppl 2), S213–S216 CrossRef CAS PubMed.
- B. Kołodziejska, N. Stępień and J. Kolmas, The Influence of Strontium on Bone Tissue Metabolism and Its Application in Osteoporosis Treatment, Int. J. Mol. Sci., 2021, 22(12), 6564 CrossRef PubMed.
- Strontium signaling: molecular mechanisms and therapeutic implications in osteoporosis - PubMed. [cited 2023 Jan 31]. Available from: https://pubmed.ncbi.nlm.nih.gov/22820094/.
- Strontium ranelate promotes odonto-/osteogenic differentiation/mineralization of dental papillae cells in vitro and mineralized tissue formation of the dental pulp in vivo | Scientific Reports. [cited 2023 Jan 31]. Available from: https://www.nature.com/articles/s41598-018-27461-7.
- M. S. Rybchyn, M. Slater, A. D. Conigrave and R. S. Mason, An Akt-dependent increase in canonical Wnt signaling and a decrease in sclerostin protein levels are involved in strontium ranelate-induced osteogenic effects in human osteoblasts, J. Biol. Chem., 2011, 286(27), 23771–23779 CrossRef CAS PubMed.
- D. Bryk, W. Olejarz and D. Zapolska-Downar, [Mitogen-activated protein kinases in atherosclerosis], Postepy Hig. Med. Dosw., 2014, 68, 10–22 CrossRef PubMed.
- P. J. Marie, P. Ammann, G. Boivin and C. Rey, Mechanisms of Action and TherapeuticPotential of Strontium in Bone, Calcif. Tissue Int., 2001, 69(3), 121–129 CrossRef CAS PubMed.
- Strontium Ranelate Reduces the Risk of Nonvertebral Fractures in Postmenopausal Women with Osteoporosis: Treatment of Peripheral Osteoporosis (TROPOS) Study | The Journal of Clinical Endocrinology & Metabolism | Oxford Academic. [cited 2023 Jan 31]. Available from: https://academic.oup.com/jcem/article/90/5/2816/2836859.
- Strontium ranelate reduces the risk of vertebral fracture in young postmenopausal women with severe osteoporosis | Annals of the Rheumatic Diseases. [cited 2023 Jan 31]. Available from: https://ard.bmj.com/content/67/12/1736.short.
- The Effects of Strontium Ranelate on the Risk of Vertebral Fracture in Women with Postmenopausal Osteoporosis | NEJM. [cited 2023 Jan 31]. Available from: https://www.nejm.org/doi/full/10.1056/nejmoa022436.
- How strontium ranelate, via opposite effects on bone resorption and formation, prevents osteoporosis | SpringerLink. [cited 2023 Jan 31]. Available from: https://link.springer.com/article/10.1007/s00198-010-1369-0.
- Y. L. Wang, H. H. Chang, Y. C. Chiang, C. H. Lin and C. P. Lin, Strontium ion can significantly decrease enamel demineralization and prevent the enamel surface hardness loss in acidic environment, J. Formosan Med. Assoc., 2019, 118(1, Part 1), 39–49 CrossRef PubMed.
- Dental caries and strontium concentration in drinking water and surface enamel - PubMed. [cited 2023 Jan 31]. Available from: https://pubmed.ncbi.nlm.nih.gov/6576005/.
- B. F. A. Karim and D. G. Gillam, The Efficacy of Strontium and Potassium Toothpastes in Treating Dentine Hypersensitivity: A Systematic Review, Int. J. Dent., 2013, 2013, 573258 CAS.
- D. P. Dearnaley, R. J. Bayly, R. P. A'Hern, J. Gadd, M. M. Zivanovic and V. J. Lewington, Palliation of bone metastases in prostate cancer. Hemibody irradiation or strontium-89?, Clin. Oncol., 1992, 4(2), 101–107 CrossRef CAS PubMed.
- F. Giammarile, T. Mognetti and I. Resche, Bone pain palliation with strontium-89
in cancer patients with bone metastases, Q. J. Nucl. Med., 2001, 45(1), 78–83 CAS.
- R. G. Robinson, Strontium-89–precursor targeted therapy for pain relief of blastic metastatic disease, Cancer, 1993, 72(11 Suppl), 3433–3435 CrossRef CAS PubMed.
- H. Zhai, W. Hannon, G. S. Hahn, R. A. Harper, A. Pelosi and H. I. Maibach, Strontium nitrate decreased histamine-induced itch magnitude and duration in man, Dermatology, 2000, 200(3) DOI:10.1159/000018367.
- A Novel Topical Formulation Containing Strontium Chloride Significantly Reduces the Intensity and Duration of Cowhage-Induced Itch. [cited 2023 Jan 31]. Available from: https://www.medicaljournals.se/acta/content/abstract/10.2340/00015555-1564.
- Strontium, ketamine target troublesome itch | MDedge Internal Medicine. [cited 2023 Jan 31]. Available from: https://www.mdedge.com/internalmedicine/article/134997/dermatology/strontium-ketamine-target-troublesome-itch.
- Effect of Strontium Chloride on Experimental Bladder Inflammation in Rat. [cited 2023 Jan 31]. Available from: https://www.hindawi.com/journals/isrn/2014/369292/.
- A. D. P. Papoiu, R. Valdes-Rodriguez, L. A. Nattkemper, Y. H. Chan, G. S. Hahn and G. Yosipovitch, A novel topical formulation containing strontium chloride significantly reduces the intensity and duration of cowhage-induced itch, Acta Derm.-Venereol., 2013, 93(5), 520–526 CrossRef PubMed.
- J. Zhang, S. Zhao, Y. Zhu, Y. Huang, M. Zhu and C. Tao,
et al., Three-dimensional printing of strontium-containing mesoporous bioactive glass scaffolds for bone regeneration, Acta Biomater., 2014, 10(5), 2269–2281 CrossRef CAS PubMed.
- E. Dallas and P. L. W. Cham, Barium: Rationale for a New Oral Reference Dose, J. Toxicol. Environ. Health, Part B, 2001, 4(4), 395–429 Search PubMed.
- J. Norum, E. R. Traasdahl, A. Totth, C. Nieder and J. A. Olsen, Health Economics and Radium-223 (Xofigo®) in the Treatment of Metastatic Castration-Resistant Prostate Cancer (mCRPC): A Case History and a Systematic Review of the Literature, Glob. J. Health Sci., 2016, 8(4), 1–9 Search PubMed.
-
S. A. Hedya, A. Avula and H. D. Swoboda, Lithium Toxicity, in StatPearls, StatPearls Publishing, Treasure Island, FL, 2023. Available from: https://www.ncbi.nlm.nih.gov/books/NBK499992/ Search PubMed.
- A. Shearer, M. Montazerian, J. J. Sly, R. G. Hill and J. C. Mauro, Trends and perspectives on the commercialization of bioactive glasses, Acta Biomater., 2023, 160, 14–31 CrossRef CAS PubMed.
|
This journal is © The Royal Society of Chemistry 2024 |
Click here to see how this site uses Cookies. View our privacy policy here.