DOI:
10.1039/D2TB00297C
(Paper)
J. Mater. Chem. B, 2022,
10, 4031-4039
In situ growth of WO3/BiVO4 nanoflowers onto cellulose fibers to construct photoelectrochemical/colorimetric lab-on-paper devices for the ultrasensitive detection of AFP†
Received
8th February 2022
, Accepted 19th April 2022
First published on 20th April 2022
Abstract
In this work, novel dual-mode lab-on-paper devices based on in situ grown WO3/BiVO4 heterojunctions onto cellulose fibers, as signal amplification probes, were successfully fabricated by the integration of photoelectrochemical (PEC)/colorimetric analysis technologies into a paper sensing platform for the ultrasensitive detection of alpha-fetoprotein (AFP). Specifically, to achieve an impressive PEC performance of the lab-on-paper device, the WO3/BiVO4 heterojunction was in situ grown onto the surface of cellulose fibers assisted with Au nanoparticle (Au NP) functionalization for enhancing the conductivity of the working zone of the device. With the target concentration increased, more immune conjugates could be captured by the proposed paper photoelectrode, which could lead to a quantitative decrease in the photocurrent intensity, eventually realizing the accurate PEC signal readout. To meet the requirement of end-user application, a colorimetric signal readout system was designed for the lab-on-paper device based on the color reaction of 3,3′5,5′-tetramethylbenzidine (TMB) oxidized by WO3/BiVO4 nanoflowers in the presence of H2O2. Noticeably, it is the first time that the WO3/BiVO4 heterojunction is in situ grown onto cellulose fibers, which enhances the sensitivity in view of both their PEC activity and catalytic ability. By controlling the conversion process of hydrophobicity and hydrophilicity on the lab-on-paper device combined with diverse origami methods, the dual-mode PEC/colorimetric signal output for the ultrasensitive AFP detection was realized. Under optimal conditions, the proposed dual-mode lab-on-paper device could enable the sensitive PEC/colorimetric diagnosis of AFP in the linear range of 0.09–100 ng mL−1 and 5–100 ng mL−1 with the limit of detection of 0.03 and 1.47 ng mL−1, respectively.
Introduction
Alpha-fetoprotein (AFP), as one of the major components of proteins in human embryonic serum, is mainly generated from the yolk sac, germ cell, and liver organ.1,2 Generally, the concentrations of AFP in the serum of the newborn and healthy adults are about 10–50 μg mL−1 and 25 ng mL−1, respectively.3–5 Although the concentration of AFP is low in serum, its concentration variation in serum has been identified to be associated with several diseases such as yolk sac tumor, hepatocellular carcinoma (HCC), and certain gastric carcinomas.6–12 Therefore, it is well worth to exploit effective strategies with advantageous techniques for the ultrasensitive determination of AFP.
Till date, several approaches based on various techniques for detecting AFP have been reported, including electrochemical,13,14 electrochemiluminescence (ECL),15 fluorescence,16 and enzyme-linked immunosorbent assay (ELISA).17 However, these strategies could not avoid potential challenges such as time consumption, high detection cost, and unsuitability for rapid analysis. To address these concerns, the photoelectrochemical (PEC) technique that integrates photochemistry with electrochemistry has been extensively explored for bioassays in recent years18,19 due to its inherent merits such as high sensitivity, easy operation with simple instrumentation, and low background signal.20,21 Unlike the conventional electrochemical sensing system for which normally a specific bias has to be applied for producing readout signals, the PEC sensing system can sufficiently reduce the dependence on potential application for signal generation resulting from the enhanced redox property of photoelectric materials with efficient carrier separation ability. Besides, the PEC sensing system possess two separate source parts for signal excitation and detection, making it a potentially higher sensitivity sensing technique because of the reduced background noise.22–27 In the PEC sensing process, electron-hole pair separation and charge transfer occur on the photoactive material under light irradiation, resulting in photoelectric signal conversion, and further inducing a great change in the corresponding light response, which is normally decided by the properties of photoactive materials.20,22 Therefore, to achieve a highly sensitive PEC platform for detecting AFP, it is extremely important to explore photoactive materials that play a key role for its construction.28 Semiconductor materials are widely applied to construct novel photoactive materials due to their satisfied electronic states of the valence band and conduction band as well as suitable bandgaps.21 In particular, oxide semiconductor materials, as a typical semiconductor, have been explored for the development of photoactive materials over the past few years, such as TiO2,29–31 WO3,32,33 ZnO,34–37 and SnO2.38 Of these, WO3, as a low-cost metal oxide semiconductor, is an indirect bandgap photoactive material with favorable photoconductive behaviors, which can absorb a wide range of wavelengths including part of visible light based on its moderate bandgap (2.5–2.7 eV), and good electron transport properties on account of its appropriate band-edge position.39 For all that, however, WO3 alone as a photoanode material still overcomes the limitations such as poor charge separation, fast recombination of photo-generated electron-hole pairs, long hole diffusion length, and less utilization of solar irradiation due to the narrow photoresponse region (≤460 nm) in PEC applications.40,41 To address this problem, forming binary nanocomposites via hybridizing WO3 with proper bandgap semiconductors is one of the effective strategies to improve the PEC performance of WO3.42 BiVO4, as a typical semiconductor with a moderate band gap (∼2.4 eV), high stability against photocorrosion, and a high specific surface area of various well-defined morphologies, has been proven to be one of the most potential sensitizers for adjusting the bandgap of semiconductors, and applied for biomedical developments with its toxic free nature, low cost, and long-term chemical stability.43–47 In such case, WO3/BiVO4 nanocomposites could not only integrate the merits of WO3 with good electron transport rate and BiVO4 with broad light absorption range, but also effectively realize the separation of photogenerated carriers for further enhancing the photocurrent response.42 Besides, WO3/BiVO4 nanocomposites could catalyze 3,3′5,5′-tetramethylbenzidine (TMB) to be oxidized with H2O2 due to its peroxidase mimic activity, and achieve colorimetric detection applications.48
In recent years, the cellulose paper has attracted extensive attention due to its inherited merits of good biocompatibility, easy functionalization, and acceptable biodegradability in the fabrication of portable diagnostic devices.49–57 Thus far, some lab-on-paper devices such as paper-based colorimetric biosensors,58 paper-based electrochemical analytical devices,59,60 enzyme-based paper biosensors,61 and self-powered origami paper analytical devices based on various techniques have been developed.62 What is more, since the cellulose paper has a unique porous structure, rough surface properties, and a large surface-to-volume ratio, the majority nanomaterials and functional groups could be modified on the surface of cellulose fibers, meeting the requirement as a satisfied substrate to construct biosensing platforms.
Herein, we propose a dual-mode PEC/colorimetric lab-on-paper device based on the in situ growth of WO3/BiVO4 heterojunctions onto cellulose fibers for highly sensitive AFP detection on account of their enhanced PEC activity and catalytic ability. At first, to realize the PEC mode, the WO3/BiVO4 heterojunction was in situ grown onto the surface of cellulose fibers, where a layer of Au NPs was grown for improving the electron transport rate, by a hydrothermal method, as illustrated in Scheme 1A. Then, the AFP antibodies (Ab) were immobilized onto a WO3/BiVO4 heterojunction-functionalized Au NP-modified paper working electrode (Au-PWE) using chitosan (CS) and glutaraldehyde (GLD) to capture AFP targets. By introducing bovine serum albumin (BSA) to avoid any nonspecific interaction, the AFP can be specifically recognized and connected via specific recognition on the photoanode surface of the proposed device, eventually achieving the ultrasensitive detection of AFP as the photocurrent response decreased based on the effective H2O2 electron donor consumption and apparent steric hindrance. To reach the colorimetric detection of AFP, WO3/BiVO4 nanoflowers were involved in the chromogenic region by a delicate design of the lab-on-paper device and utilized to efficiently catalyze the oxidation of embedded TMB in the presence of H2O2, successfully realizing the semi-quantitative AFP target detection using a colorimetric signal readout. As a result, the dual-mode PEC/colorimetric lab-on-paper device based on the in situ growth of WO3/BiVO4 heterojunctions onto cellulose fibers for ultrasensitive AFP detection was finally constructed. Besides, the good feasibility of the as-prepared lab-on-paper device applied in real blood serum sample detection was investigated, indicating the great potential applications in the point-of-care testing of AFP and clinical disease diagnosis.
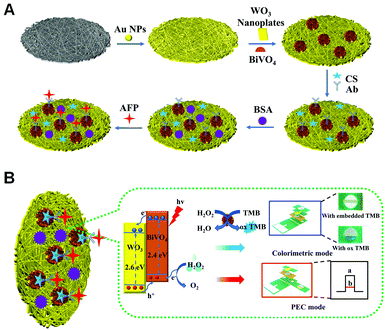 |
| Scheme 1 (A) Fabrication procedure of the lab-on-paper PEC sensing platform. (B) Schematic illustration of the PEC/colorimetric analytical principle. (Colorimetric mode: the folding state of the lab-on-paper device shown in blue rectangle and the colorimetric results before and after incubation of AFP; PEC mode: the folding state of the lab-on-paper device shown in red rectangle and the PEC signal before and after incubation of AFP.) | |
Experimental section
Materials and reagents
All aqueous solutions were prepared with deionized water (DI water, resistivity ≥18.25 MΩ cm−1), which was obtained from a Millipore water purification system. KCl, NaWO3 and NH4VO3 were purchased from Maclean Biochemical Technology Co., Ltd (Shanghai, China). Sodium hydroxide (NaOH) and Bi(NO3)3·5H2O, were provided by Aladdin (Shanghai, China). KH2PO4, Na2HPO4, (NH4)2C2O4 and urea were obtained from Tianjin Damao Chemical Reagent Company (Tianjin, China). Chloroauric acid (HAuCl4·4H2O) and hydrogen peroxide (H2O2) were ordered from Sangon Biological Engineering Technology and Services Co., Ltd (Shanghai, China). 3,3′,5,5′-Tetramethylbenzidine (TMB) and acetic acid were purchased from Shanghai Jizhi Biochemical Technology Co., Ltd (Shanghai, China). Bovine serum albumin (BSA) was obtained from Sigma-Aldrich Chemical Co. Phosphate-buffered saline (PBS, pH 7.4, 0.1 M) was prepared with Na2HPO4 (0.1 M), KH2PO4 (0.1 M), and KCl (0.1 M). Chitosan (CS) was purchased from Solarbio Co., Ltd (China). Glutaraldehyde (GLD; 50%) was purchased from Tianjin Chemical Plant Co., Ltd (Tianjin, China). Alpha-fetoprotein (AFP), fetoprotein antibody (anti-AFP), carcino-embryonic antigen (CEA) and prostate-specific antigen (PSA) were purchased from Shanghai Linc-Bio Science Co., Ltd (Shanghai, China). Human immunoglobulin G (H-IgG) was obtained from Shanghai Sangon Biotech Co., Ltd (China). All chemicals were used without further purification. Whatman chromatography paper #2 (58.0 cm × 68.0 cm) was received from GE Healthcare Worldwide (Shanghai, China), and it was cut into A4 size before use.
Apparatus and measurements
The photocurrent response and electrochemical impedance spectroscopy (EIS) were performed using a CHI 660D electrochemical workstation (Shanghai CH Instruments Co., China) with a strand three-electrode system consisting of the working, reference, and counter electrodes. Ultraviolet-visible (UV-vis) absorption spectra were recorded using a UV-2550 spectrophotometer (Shimadzu, Kyoto, Japan). Scanning electron microscopic (SEM) analyses were performed using a QUANTA FEG 250 thermal field emission SEM (FEI Co., USA) equipped with an Oxford X-MAX50 X-ray energy-dispersive spectrometer (EDS) (Oxford Co., UK). Transmission electron microscopic (TEM) images were acquired using a JEOL 4000 EX microscope. Infrared spectra were recorded using a Fourier transform infrared (FT-IR) Spectrum RX (PerkinElmer Spectoment). X-ray photoelectron spectroscopy (XPS) spectra were recorded using an ESCALAB MK II X-ray photoelectron spectrometer. X-ray diffraction (XRD) patterns were acquired using a Pa D8 advance diffractometer system equipped with a Cu Kα radiation source (Bruker Co., Germany). Raman spectrum was recorded using a Labram HR evolution spectrometer (Horiba jobin yvon Corporation, French). The EIS experiments were carried out in PBS (pH 7.4, 0.1 M) containing 5.0 mM [Fe(CN)6]3−/4− with 0.1 M KCl. Photocurrent response measurements were done in PBS (pH 6.5, 0.1 M) at room temperature with 200 μL of H2O2 (5 mM). During the PEC measurement procedure, a Xe lamp was used as the excitation light source, and the applied potential was 0.4 V.
Preparation of WO3 nanoflakes on the paper-based electrode
The preparation of a WO3 lab-on-paper device was similar to a previous protocol.33 Before the WO3 nanoflakes were modified to the paper electrode, a layer of Au nanoparticles was dropped to the paper work zone. Due to the high conductivity of Au nanoparticles, the WO3 nanoflakes are easy to grow on the work zone in situ. First, 0.5 g sodium tungsten (Na2WO3) was dissolved in 30 mL of deionized water under stirring at room temperature, and then 10 mL of 2.0 M HCl was slowly added into this solution. Subsequently, 0.4562 g of ammonium oxalate ((NH4)2C2O4) was added into 30 mL of deionized water under stirring. By mixing this solution under stirring for 30 minutes, a clear and transparent solution was obtained. The prepared precursor was transferred into a 100 mL Teflon-lined stainless autoclave. The lab-on-paper device was immersed and leaned against the wall of the Teflon vessel with the conducting side face down. After that, the Teflon-lined stainless autoclave was put into an oven at 160 °C for 1 h. Finally, the lab-on-paper device with WO3 nanoflakes was washed with deionized water and dried at 60 °C overnight.
Preparation of WO3/BiVO4 paper-based photoelectrodes
The synthesis method of BiVO4 is based on a procedure previously reported in the literature with minor modifications.63 Briefly, 4.3 mM NH4VO3 and 5.2 mM Bi(NO3)3·5H2O were dissolved in 40 mL deionized water under stirring and named solution A. Then, 20 mL deionized water was dissolved in 25 mM urea and named solution B. Solution B was dropped into solution A at a rate of 60 drops per minute after urea was completely dissolved. When a homogeneous orange solution was obtained, it was moved into a 100 mL Teflon-lined stainless autoclave with the conductive side of the WO3 nanoflake electrode facing down. After that, the Teflon-lined stainless autoclave was kept in an oven at 180 °C for 4 h. Finally, the WO3/BiVO4 paper-based electrode was washed thoroughly with deionized water and dried at 60 °C overnight.
Results and discussion
Mechanism of the constructed PEC/colorimetric lab-on-paper device
The working principle of the proposed lab-on-paper device based on in situ growth of the WO3/BiVO4 heterojunction onto cellulose fibers as a signal amplification probe for PEC/colorimetric sensing of AFP is illustrated in Scheme 1B. To achieve the advantageous PEC sensing mode, BiVO4 with good biocompatibility, which has a narrow band gap, was introduced to WO3 and formed the WO3/BiVO4 heterojunction, which is modified onto the 3D cellulose fibers of the working zone of the PEC detection tab in the lab-on-paper device, exhibiting the admirable PEC performance owing to the unique spatial structure of the functionalized working zone and their preferable morphologies, which could evidently harvest light energy by extending the absorption range, and ultimately improve the intensity of the produced photocurrent. Under irradiation of light, the photoinduced charge carriers of the semiconductor material could remain separated because of their intrinsic electrostatic field. In detail, the photo-induced electrons were transferred from the conduction band of the BiVO4 nanomaterial into WO3 nanosheets, while the holes generated in the valence band of WO3 were transferred into the BiVO4 nanomaterial, which could be consumed by H2O2 electron donors, further enlarging the initial PEC response by the improvement of the separation efficiency of electron-hole carriers. When the AFP targets were introduced by the specifically recognizing interaction with anti-AFP, which is immobilized onto the WO3/BiVO4 heterojunction, a significantly decreased photocurrent signal was produced with obvious steric effects, which are responsive to the concentration change of the target, finally achieving the quantitative PEC detection of AFP. Meanwhile, to realize the colorimetric mode detection of AFP, a colorimetric reaction was introduced by simply altering the device spatial configuration in the presence of WO3/BiVO4 nanoflowers, which could catalyze the TMB oxidation with existing H2O2. In this case, the TMB could lose electrons and transfer into its oxidation products, triggering the colorless solution to turn blue gradually. Accordingly, the proposed lab-on-paper device fabricated by integrating PEC and colorimetric strategies was effectively used for dual-mode ultrasensitive determination of AFP.
Construction and characterization of the dual-mode lab-on-paper device
A three-dimensional (3D) lab-on-paper device was fabricated to achieve PEC/colorimetric dual-mode AFP detection, and its assembly and operation illustration are exhibited in Fig. 1. (i) The designed lab-on-paper device possesses four parts including PEC detection tab, electrode tab (the yellow tab), washing tab, and colorimetric tab. In addition, the whole arrangement is shown in Fig. 1A. (ii) To obtain the PEC signal, the working zone of the PEC detection tab was modified with a layer of Au NPs (the modification process is explained in detail in ESI†) and the WO3/BiVO4 heterojunction was in situ grown onto cellulose fibers, as shown in Fig. 1B. (iii) As shown in Fig. 1C, the washing tab was folded downward, so that the channel of washing tab could touch the working zone of the detection tab. Therefore, the waste liquid could be collected by the waste pool to treat the liquid waste after each operation. Besides, after PEC detection, the rest liquid was also handled by this folding mode. (iv) In order to realize the dual-mode detection, different folding methods are illustrated in Fig. 1D and E. As illustrated in Fig. 1D, the electrode tab (with a counter and a reference electrode) was projected to right to cover the PEC detection tab. Then, the working zone (a circle of 10 mm in diameter) of the PEC detection tab was folded up to cover the electrode tab to form a three-electrode system. After adding 1.0 mL PBS (pH 6.5, 0.1 M) including 200 μL of H2O2 (5 mM) to the working zone of the detection tab (Fig. 1D), the amplification PEC signal was recorded using a CHI 660D electrochemical workstation. Finally, to achieve the colorimetric detection, the colorimetric tab was folded left, so that the embedded TMB could react with the injected H2O2 (5 mM, 250 μL) in the presence of WO3/BiVO4 nanoflower photoactive materials onto the working zone of the detection tab (Fig. 1E).
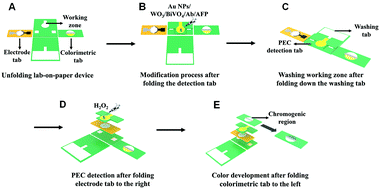 |
| Fig. 1 (A–E) Assembly and operation illustration of the lab-on-paper device. (A) Unfolding the lab-on-paper device. (B) Modification process after folding the detection tab. (C) Washing working zone after folding down the washing tab. (D) PEC detection after folding the electrode tab to the right. (E) Color development after folding the colorimetric tab to the left. | |
To make sure that the WO3/BiVO4 heterojunction was successfully grown onto the surface of cellulose fibers of the lab-on-paper device, the following technologies were applied, such as scanning electron microscopy (SEM), high-resolution transmission electron microscopy (HRTEM), energy-dispersive spectrum (EDS) elemental mapping, X-ray photoelectron spectroscopy (XPS) and X-ray diffraction (XRD). The SEM images in Fig. 2A and its inset image show different magnifications of a bare paper working electrode (PWE), and it can be seen that the bare paper consists of tangled cellulose fibers with porous networks, which could provide the potential platform with a large surface area for the modification of photoactive materials. To improve the conductivity and electron transfer ability of cellulose fibers, a layer of Au NPs was introduced to the surface of cellulose fiber. As shown in Fig. 2B, the uniform and continuous Au NP layer appeared onto the paper fiber surface. Fig. 2C and its inset present the SEM images of different magnifications of the WO3-functionalized Au-PWE (WO3/Au-PWE), which illustrate that the WO3 nanoplate was densely grown on the cellulose fiber surface, making it a larger specific surface area to load more BiVO4. The SEM images of WO3/BiVO4 heterojunctions are displayed in Fig. 2D and E, which clearly show nanoflower morphologies with a diameter of about 2.3 μm. The special nanoflower structure with a large specific surface area is beneficial for constructing the sensing platform. Fig. 2F shows the HRTEM image of the WO3/BiVO4 heterojunction and its inset a matched with the WO3(110) plane, 0.29 nm in size, and inset b agreed well with BiVO4(112), 0.2 nm in size. In addition, the elemental mapping under the SEM mode was applied to illustrate the presence of W, O, Bi, and V elements in a single WO3/BiVO4 nanoflower. The corresponding mapping SEM images are shown in Fig. 2G–K, which clearly show that the WO3/BiVO4 nanoflower was successfully synthesized. Besides, the energy-dispersive spectrum (EDS) mappings of different elements displayed in Fig. S5 (ESI†) clearly show the presence of W, O, Bi, and V elements, which demonstrated the successful synthesis of WO3/BiVO4 nanoflowers.
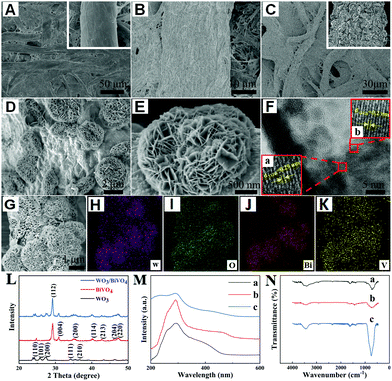 |
| Fig. 2 (A) SEM images of bare a PWE; inset: high-magnification image of bare PWE. (B) SEM image of Au-PWE. (C) WO3/Au-PWE; inset: high-magnification image of WO3/Au-PWE. (D and E) WO3/BiVO4 under different magnifications. (F) HRTEM image of the WO3/BiVO4 heterojunction (inset (a): the lattice of WO3; inset (b): the lattice of BiVO4). (G–K) Elemental mapping SEM mode of W, O, Bi, and V and the corresponding SEM image. (L) XRD patterns of WO3, BiVO4, and WO3/BiVO4. (M) UV-vis absorption spectrum of WO3 (curve a), BiVO4 (curve b), and WO3/BiVO4 (curve c). (N) FT-IR spectra of (a) WO3, (b) BiVO4, and (c) WO3/BiVO4. | |
XRD was performed to identify the crystal structure of the proposed WO3/BiVO4 heterojunction. It is seen from the XRD image, as exhibited in Fig. 2L, that there are a series of diffraction peaks at 24.3, 26.8, 28.2, 33.5, and 37.6°, which are assigned to the (110), (101), (200), (111), and (210) crystal planes of WO3, and the other diffraction peaks appear at 28.6, 30.9, 34.5, 39.7, 45.5, 47.0, and 49.6° which correspond to the (112), (004), (200), (114), (213), (204), and (220) crystal planes of BiVO4. The blue curve of the XRD patterns of WO3/BiVO4 nanoflowers was consistent with pure WO3 and BiVO4 XRD patterns, which clearly show that the WO3/BiVO4 heterojunction was successfully synthesized. To further verify the synthesized WO3/BiVO4 heterostructure, the ultraviolet-visible (UV-vis) absorption spectra and Fourier transform infrared (FT-IR) spectra were also adopted. As shown in Fig. 2M, the UV-vis absorption spectrum of WO3/BiVO4 (curve c) shows a wider absorption range than that of WO3 (curve a), indicating that the WO3/BiVO4 heterojunction enhanced the light harvesting ability. Consequently, the extended light absorption capacity of WO3/BiVO4 was conducive to amplify the photo-to-current conversion efficiency. The results from the Tauc plots (Fig. S6A, ESI†) also demonstrated the effect of heterojunction formation on the photoactive materials. Fig. 2N shows the IR spectra over the range of 500–4000 cm−1 at room temperature. The results indicate the different modes of vibrations of molecules with their associated energies in the infrared region. The representative absorption peak at 948 cm−1 observed in the WO3 (curve a) was the fundamental vibration, which was assigned to W–O. What is more, curve b represents BiVO4, and the peaks at 730 and 834 cm−1 in curve b belong to the asymmetric stretching and symmetric vibration of VO43− tetrahedron. Accordingly, the WO3/BiVO4 heterojunction synthesis was successfully realized (curve c).44,64 In addition, an elemental analysis of XPS technique was also carried out to confirm the successful preparation of the WO3/BiVO4 heterojunction. As exhibited in Fig. 3, the chemical states of W, O, Bi, and V elements in WO3/BiVO4 were investigated by XPS analysis. It can be seen that all the peak positions of WO3/BiVO4 in the XPS spectra were demarcated with C 1s at 281.9 eV. As shown in Fig. S8 (ESI†), the full survey spectrum results prove the existence of W, O, Bi, and V elements. The main XPS elemental spectra of W, O, Bi, and V elements are demonstrated in Fig. 3A–D. Fig. 3A shows two strength peaks at 32.4 and 34.4 eV, which are ascribed to the high oxidation state of W in WO3.65 The peak in Fig. 3B at 527.02 eV belongs to the lattice oxygen. Fig. 3C demonstrates two peaks of 156.18 and 161.47 eV, which could be attributed to Bi 4f7/2 and Bi 4f5/2 in the spin–orbit splitting of Bi3+. The peak of V 2p in Fig. 3D belongs to V5+ in BiVO4.66 All of the above-mentioned results illustrated the successful preparation of the WO3/BiVO4 heterojunction.
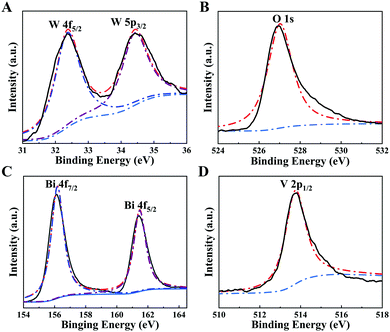 |
| Fig. 3 XPS spectrum of the WO3/BiVO4 heterojunction: (A) W 4f. (B) O 1s. (C) Bi 4f. (D) V 2p. | |
PEC and electrochemical characterization of the fabricated lab-on-paper device
To investigate the photoresponse performance of the as-prepared device, the transient photocurrent investigation was conducted by chronoamperometry under chopped light illumination. As exhibited in Fig. 4A, the photoresponse of the proposed device with various modification processes was measured at an applied bias potential of 0.4 V under light irradiation with on-off cycles. Compared with the bare BiVO4 electrode (curve a), a stronger photocurrent of WO3/BiVO4 (curve b) was achieved under illumination, evidentially suggesting the improvement of separation efficiency of electron–hole carriers by introduction of BiVO4 into WO3 to form WO3/BiVO4 heterojunctions. After modifying with CS, Ab, BSA and AFP onto a WO3/BiVO4 heterojunction-functionalized cellulose paper electrode, a descending trend of photocurrent intensity of the obtained photoelectrode appears with WO3/BiVO4/CS (curve c) > WO3/BiVO4/CS/Ab (curve d) > WO3/BiVO4/CS/Ab/BSA (curve e) > WO3/BiVO4/CS/Ab/BSA/AFP (curve f) because the relative insulative proteins could inhibit sacrificial agent diffusion to the electrode surface and further decrease the photocurrent intensity.8 Meanwhile, the successively decreased photocurrent intensity indicated the successful immobilization of these proteins onto the photoelectrode. For further investigating the assembly process of the proposed photoelectrode and its interface electron transfer activity, electrochemical impedance spectroscopy (EIS) was performed. Fig. 4B illustrates the electrochemical impedance Nyquist plot of the various modified process of the proposed photoelectrode with [Fe (CN)6]3−/4− for the redox probe. In addition, the Nyquist plot included a semicircle sector and a linear sector, where the diameter of the semicircle could directly express the interface electron transfer resistance (Ret) of the photoelectrode.46 It can be seen that Ret of WO3/BiVO4 (curve b) was smaller than WO3 (curve a) due to the quick electron transfer of the WO3/BiVO4 sensing interface, whereas when CS was introduced into the surface of the electrode, the Ret value of WO3/BiVO4/CS increased significantly (curve c) owing to the obstructing effect of the modified biomolecules for the ferricyanide diffusion to the surface of electrode. In addition, upon incubation of anti-AFP on the electrode surface, the Ret value increased more (curve d) because of the poor electron transfer ability. Finally, after BSA was added to the surface of the electrode to block the active sites, the value of Ret continued to increase (curve e), resulting from the poor conductivity of organic molecules as well as impeding the [Fe(CN)6]3−/4− transfer to the photoelectrode surface. Accordingly, the obtained EIS results of each modification step indicated the successful construction of the lab-on-paper device.
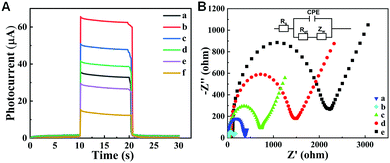 |
| Fig. 4 (A) Photocurrent responses of the modified electrode in PBS (0.1 M, pH 6.6) containing 5 mM H2O2 at 0.4 V: (a) BiVO4, (b) WO3/BiVO4, (c) WO3/BiVO4/CS, (d) WO3/BiVO4/CS/Ab, (e) WO3/BiVO4/CS/Ab/BSA, and (f) WO3/BiVO4/CS/Ab/BSA/AFP (cAFP = 0.1 ng mL−1). (B) Electrochemical impedance spectroscopy (EIS) for each modification process in PBS (0.1 M, pH 7.4) containing 5.0 mM [Fe(CN)6]3−/4− and 0.1 M KCl: (a) WO3, (b) WO3/BiVO4, (c) WO3/BiVO4/CS, (d) WO3/BiVO4/CS/Ab, and (e) WO3/BiVO4/CS/Ab/BSA. | |
Analytical performance of the lab-on-paper device for PEC/colorimetric of AFP immunosensing
To obtain the optimum PEC signal of the prepared sensing platform, experimental conditions including applied potential, the pH of electrolyte, the synthesis time of photoactive materials, and the incubation time of AFP were carefully investigated. (The details and the obtained results are illustrated in ESI† and Fig. S9, respectively.) Under the optimal experimental conditions, the analytical performance of the as-prepared PEC immunosensor was investigated by recording the photocurrents to different concentrations of AFP. As the AFP concentration increased from 0.1 to 100 ng mL−1, the photocurrent decreased accordingly because the more incubated AFP could inhibit more H2O2 in contact with the photoelectrode surface (Fig. 5A). As shown in Fig. 5B, the calibration plots indicated that the PEC signal decrement was proportional to the AFP concentrations logarithmically in the 0.09–100 ng mL−1 range. The linear equation is as follows: I = −3.32
log
cAFP + 9.70 (R2 = 0.997), and the limit of detection (LOD) is 0.03 ng mL−1 with a signal-to-noise (S/N) ratio of 3. Compared with previous reported works (Table 1: comparison of several immunosensors of AFP detection and Table S1 (ESI†): comparison with other PEC-based AFP biosensors), the proposed PEC immunosensor presented a wide linear range and low LOD, which could be attributed to the good property of the synthesized photoelectrode material and low background noise of PEC technical assay. What is more, the corresponding colorimetric detection could be realized by folding the colorimetric tab of the fabricated device. After introduction of H2O2 into the colorimetric tab, the enhanced catalytic activity of WO3/BiVO4 could catalyze TMB, which was simply pre-embedded at the detection zone of the colorimetric tab of the prepared lab-on-paper device to produce ox-TMB with a blue product. In this way, AFP could be detected by the color intensity of the colorimetric tab, realizing the colorimetric signal readout for AFP target semi-quantitative detection. The real images of colorimetric detection and the calibration curve can be seen in Fig. 5C. The corresponding linear equation is Y = −22.686
log
cAFP + 44.967 (R2 = 0.997) and the colorimetric LOD is 1.47 ng mL−1 with a linear range of 5–100 ng mL−1. Besides, the color changes from dark blue to cyan in colorimetric sensing with the increase in AFP concentration (as shown in inset a). Besides, in order to investigate the selectivity of the as-prepared sensing platform, the photocurrent signal of the lab-on-paper device was recorded when the device was incubated with different targets, AFP, CEA, PSA, and H-IgG, under the same experimental conditions (colorimetric experiments of selectivity experiments are shown in Fig. S10, ESI†). As shown in Fig. 5D, the photocurrent intensities of CEA, PSA, and H-IgG are similar to that of the blank experiment, and far more than that of the AFP sample, indicating the good selectivity of the as-prepared lab-on-paper device for detecting AFP with the signal-off PEC strategy. Fig. 5E shows the stability of the photocurrent responses of the PEC sensing platform. The photocurrent signals were recorded as the excitation light was turned on and off for 10 s about 10 times. Record 200 s later, the photocurrent response in Fig. 5E illustrates that the photocurrent can still reach about 95% compared with its initial photocurrent value, illustrating the good long-term stability of the prepared lab-on-paper device. In addition, the relative standard deviation (RSD) was less than 2%, certifying the good stability of the fabricated biosensor. Besides, to investigate the effects of different days of storage on the proposed biosensor, the photocurrent of the proposed biosensor stored at 4 °C was measured every 5 days after incubation with 0.1 ng mL−1 AFP (Fig. 5F). After 25 days, the current response generated by the proposed biosensor could still reach 94% of the initial signal, which indicated that the proposed paper chip has credible stability.
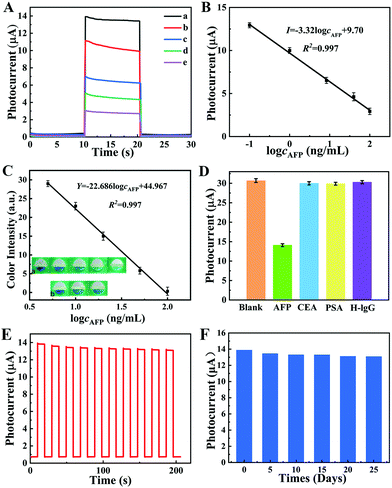 |
| Fig. 5 (A) Photocurrent responses of different concentrations of AFP at 0.1, 1, 10, 50, and 100 ng mL−1 (a–e). (B) Corresponding calibration curve. (C) Calibration curve of color intensity versus the logarithm of AFP (inset a: images of the corresponding chromogenic reactions; inset b: colorimetric sensing results of clinical serum with cAFP = 10 ng mL−1). (D) Selectivity of the proposed PEC assay of AFP (cAFP = 0.1 ng mL−1). (E) Stability of the photocurrent within 200 s (cAFP = 0.1 ng mL−1). (F) Stability of the proposed biosensor for different periods of storage: 0 days, 5 days, 10 days, 15 days, 20 days, and 25 days (cAFP = 0.1 ng mL−1). The error bar represents the standard deviation of five repeated tests. All photocurrent measurements were carried out in 0.1 M PBS (pH 6.6) containing 5 mM H2O2. The applied potential was 0.4 V. | |
Table 1 Comparison of several immunosensors of AFP detection
Method |
Detection range (ng mL−1) |
LOD |
Ref. |
ELISA |
0.1–100 |
1 ng mL−1 |
67
|
ECL |
0.5–20 |
0.1 ng mL−1 |
68
|
Electrochemical |
102–105 |
50 ng mL−1 |
69
|
Chemiluminescence |
10–150 |
7.2 pg mL−1 |
70
|
PEC |
0.09–100 |
0.03 ng mL−1 |
This work |
Application in human serum sample
To verify the practical application of the as-prepared dual-mode lab-on-paper device, clinical serum samples were detected. Briefly, real human serum samples were collected from the whole blood by centrifugation (10
000 rpm, 8 min). The supernatant was diluted and different concentrations of AFP were added. The analytical performance of serum sample-mixed AFP was investigated, and the findings (the average of three parallel experimental results) are shown in Table 2. It can be seen that the RSD and the recovery rate were from 1.17% to 5.6% and from 94.7% to 101.4%, respectively. The above-mentioned results indicated that the build device could accomplish clinical applications of AFP in human serum samples (ESI† shows more details). At the same time, colorimetric experiments in clinical serum were also investigated (colorimetric sensing results of clinical serum are illustrated in Fig. 5C), illustrating that the as-prepared lab-on-paper device could be utilized as a potential candidate for the clinical AFP detection.
Table 2 Determination of different concentrations of AFP incorporated into human serum by the proposed lab-on-paper device
Serum sample |
Added,a M |
Found, M |
Recovery,b % |
RSD, % |
The different concentration of AFP standards were added into the initial human serum samples, respectively.
The recovery was calculated by the value (the found concentration subtracted that of PEC immunoassay) relative to the added AFP level.
|
1 |
1 × 10−9 |
9.47 × 10−10 |
94.7 |
5.6 |
2 |
5 × 10−9 |
5.07 × 10−9 |
101.4 |
1.38 |
3 |
1 × 10−8 |
9.87 × 10−9 |
98.7 |
1.17 |
4 |
5 × 10−8 |
4.85 × 10−8 |
97.0 |
3.09 |
5 |
1 × 10−7 |
9.76 × 10−8 |
97.6 |
2.46 |
Conclusions
In summary, a dual-mode PEC/colorimetric lab-on-paper device has been successfully fabricated based on in situ growth of WO3/BiVO4 heterojunctions onto cellulose fibers for the ultrasensitive detection of AFP. The as-prepared WO3/BiVO4 nanoflower was well characterized by various techniques including SEM, EDS, HRTEM, XRD, UV-vis, FT-IR spectroscopy, and XPS. On account of the admirable PEC response and enhanced catalytic activity of WO3/BiVO4 nanoflower in situ growth onto the 3D cellulose fibers of the designed lab-on-paper device, the highly reliable PEC signal and colorimetric signal readout system for ultrasensitive AFP detection and semi-quantitative AFP detection has been successfully obtained. Besides, by the smart layout of the lab-on-paper device for conversion treatment between hydrophobicity and hydrophilicity, the washing issue in the operational process of the lab-on-paper device was easily solved by manually folding the washing tab of the device. We believe that the provided approach could provide an extensive strategy with features of simple and convenient operation processes for the ultrasensitive dual-mode determination of different kinds of biomarkers based on the lab-on-paper device.
Author contributions
Xu Li: investigation, methodology, writing-original draft. Kang Cui: conceptualization, supervision, writing-reviewing and editing. Mingzhen Xiu: investigation, methodology, data curation. Chenxi Zhou: investigation, formal analysis. Li Li: investigation, formal analysis. Jing Zhang: formal analysis, visualization. Shiji Hao: investigation, formal analysis. Lina Zhang: editing, resources. Shenguang Ge: conceptualization, investigation. Yizhong Huang: investigation, resources, funding acquisition. Jinghua Yu: funding acquisition, project administration.
Conflicts of interest
The authors declare no competing financial interests.
Acknowledgements
This work was financially supported by the Science and Technology Projects of University of Jinan (XKY2002), Natural Science Foundation of Shandong Province (ZR2020MB057), Research Start-up Funds of DGUT (211135067), Guangdong Basic and Applied Basic Research Foundation (2019A1515110187), Taishan Scholars Program, Case-by-Case Project for Top Outstanding Talents of Jinan, and the Excellent Youth Innovation Team in Universities of Shandong (2019KJC016).
References
- T. B. Tomasi, Jr., Annu. Rev. Med., 1977, 28, 453–465 CrossRef.
- T. Yamashita, M. Forgues, W. Wang, J. W. Kim, Q. Ye, H. Jia, A. Budhu, K. A. Zanetti, Y. Chen, L. X. Qin, Z. Y. Tang and X. W. Wang, Cancer Res., 2008, 68, 1451–1461 CrossRef CAS PubMed.
- X. Huo, P. Liu, J. Zhu, X. Liu and H. Ju, Biosens. Bioelectron., 2016, 85, 698–706 CrossRef CAS PubMed.
- J. Lin, C. He, L. Zhang and S. Zhang, Anal. Biochem., 2009, 384, 130–135 CrossRef CAS PubMed.
- Z. Song, R. Yuan, Y. Chai, Y. Zhuo, W. Jiang, H. Su, X. Che and J. Li, Chem. Commun., 2010, 46, 6750–6752 RSC.
- H. Ikeda, Y. Sato, N. Yoneda, K. Harada, M. Sasaki, S. Kitamura, Y. Sudo, A. Ooi and Y. Nakanuma, Hum. Pathol., 2012, 43, 1955–1963 CrossRef CAS PubMed.
- X. Li, X. Pan, J. Lu, Y. Zhou and J. Gong, Biosens. Bioelectron., 2020, 158, 112158 CrossRef CAS PubMed.
- J. Chen and G. C. Zhao, Biosens. Bioelectron., 2017, 98, 155–160 CrossRef CAS PubMed.
- D. Pan, K. Chen, Q. Zhou, J. Zhao, H. Xue, Y. Zhang and Y. Shen, Biosens. Bioelectron., 2019, 131, 178–184 CrossRef CAS PubMed.
- Q. Zhou, H. Xue, Y. Zhang, Y. Lv, H. Li, S. Liu, Y. Shen and Y. Zhang, ACS Sens., 2018, 3, 1385–1391 CrossRef CAS PubMed.
- K. H. Kim, S. Y. Lee, D. G. Kim, S. Y. Lee, J. Y. Kim and J. S. Yoo, Anal. Chem., 2020, 92, 12588–12595 CrossRef CAS PubMed.
- R. T. Ma, X. B. Zhao, X. Y. Sun, J. Liu, W. Ha and Y. P. Shi, J. Mater. Chem. B, 2019, 7, 6187–6194 RSC.
- S. Zhao, N. Liu, W. Wang, Z. Xu, Y. Wu and X. Luo, Biosens. Bioelectron., 2021, 190, 113466 CrossRef CAS PubMed.
- T. Feng, X. Qiao, H. Wang, Z. Sun, Y. Qi and C. Hong, J. Mater. Chem. B, 2016, 4, 990–996 RSC.
- X. Zhu, Q. Zhai, W. Gu, J. Li and E. Wang, Anal. Chem., 2017, 89, 12108–12114 CrossRef CAS PubMed.
- J. Zhang, S. Wang, K. Liu, Y. Wei, X. Wang and Y. Duan, Anal. Chem., 2015, 87, 2959–2965 CrossRef CAS PubMed.
- X. Bi and Z. Liu, Anal. Chem., 2014, 86, 959–966 CrossRef CAS PubMed.
- X. Li, Z. Zheng, X. Liu, S. Zhao and S. Liu, Biosens. Bioelectron., 2015, 64, 1–5 CrossRef PubMed.
- Q. Zhou, G. Li, K. Chen, H. Yang, M. Yang, Y. Zhang, Y. Wan, Y. Shen and Y. Zhang, Anal. Chem., 2020, 92, 983–990 CrossRef CAS PubMed.
- W. Yang, X. Wang, W. Hao, Q. Wu, J. Peng, J. Tu and Y. Cao, J. Mater. Chem. B, 2020, 8, 2363–2370 RSC.
- P. P. Li, Y. Cao, C. J. Mao, B. K. Jin and J. J. Zhu, Anal. Chem., 2019, 91, 1563–1570 CrossRef CAS PubMed.
- N. Hao, J. Lu, M. Chi, M. Xiong, Y. Zhang, R. Hua and K. Wang, J. Mater. Chem. B, 2019, 7, 1133–1141 RSC.
- J. Shu and D. Tang, Anal. Chem., 2020, 92, 363–377 CrossRef CAS PubMed.
- J. Shu and D. Tang, Chem. – Asian J., 2017, 12, 2780–2789 CrossRef CAS PubMed.
- Y. Gao, Y. Zeng, X. Liu and D. Tang, Anal. Chem., 2022, 94, 4859–4865 CrossRef CAS PubMed.
- A. Victorious, S. Saha, R. Pandey and L. Soleymani, Angew. Chem., Int. Ed., 2021, 60, 7316–7322 CrossRef CAS PubMed.
- X. Xiang, Y. Wang, Y. Zhang, R. Yuan and S. Wei, Chem. Commun., 2021, 57, 12480–12483 RSC.
- Z. Li, H. Yang, M. Hu, L. Zhang, S. Ge, K. Cui and J. Yu, ACS Appl. Mater. Interfaces, 2020, 12, 17177–17184 CrossRef CAS PubMed.
- F. Li, S. Wang, H. Yin, Y. Chen, Y. Zhou, J. Huang and S. Ai, ACS Sens., 2020, 5, 1092–1101 CrossRef CAS PubMed.
- M. Hao, P. Miao, Y. Wang, W. Wang, S. Ge, X. Yu, X. X. Hu, B. Ding, J. Zhang and M. Yan, Anal. Chem., 2021, 93, 11251–11258 CrossRef CAS PubMed.
- J. Xue, C. Gao, L. Zhang, K. Cui, W. He and J. Yu, J. Mater. Chem. B, 2018, 6, 4697–4703 RSC.
- B. R. Lee, M. G. Lee, H. Park, T. H. Lee, S. A. Lee, S. S. M. Bhat, C. Kim, S. Lee and H. W. Jang, ACS Appl. Mater. Interfaces, 2019, 11, 20004–20012 CrossRef CAS PubMed.
- J. Wang, Y. Pan, L. Jiang, M. Liu, F. Liu, M. Jia, J. Li and Y. Lai, ACS Appl. Mater. Interfaces, 2019, 11, 37541–37549 CrossRef CAS PubMed.
- S. Zhang, L. Feng, P. Li, L. Zhang, X. Chen, S. Chu, Y. Gao, S. Xie, J. Jiang and H. Wang, J. Mater. Chem. B, 2021, 9, 5869–5876 RSC.
- G. Sun, P. Wang, P. Zhu, L. Ge, S. Ge, M. Yan, X. Song and J. Yu, J. Mater. Chem. B, 2014, 2, 4811–4817 RSC.
- X. Zhang, H. Wang, J. Zhang, J. Li, Z. Ma, J. Li, B. Leng, P. Niu and B. Liu, Nano Energy, 2020, 77, 105119 CrossRef CAS.
- S. Lv, K. Zhang, L. Zhu and D. Tang, Anal. Chem., 2020, 92, 1470–1476 CrossRef CAS PubMed.
- D. Fan, C. Bao, X. Liu, D. Wu, Y. Zhang, H. Wang, B. Du and Q. Wei, J. Mater. Chem. B, 2018, 6, 7634–7642 RSC.
- S. H. Baeck, K. S. Choi, T. F. Jaramillo, G. D. Stucky and E. W. McFarland, Adv. Mater., 2003, 15, 1269–1273 CrossRef CAS.
- S. S. Kalanur, Y. J. Hwang, S. Y. Chae and O. S. Joo, J. Mater. Chem. A, 2013, 1, 3479–3488 RSC.
- Y. Wang, W. Tian, L. Chen, F. Cao, J. Guo and L. Li, ACS Appl. Mater. Interfaces, 2017, 9, 40235–40243 CrossRef CAS PubMed.
- Y. Pihosh, I. Turkevych, K. Mawatari, T. Asai, T. Hisatomi, J. Uemura, M. Tosa, K. Shimamura, J. Kubota, K. Domen and T. Kitamori, Small, 2014, 10, 3692–3699 CrossRef CAS.
- P. Zhang and X. W. D. Lou, Adv. Mater., 2019, 31, e1900281 CrossRef PubMed.
- J. H. Kim and J. S. Lee, Adv. Mater., 2019, 31, e1806938 CrossRef PubMed.
- H. Zhang, W. Zhou, Y. Yang and C. Cheng, Small, 2017, 13, 1603840 CrossRef PubMed.
- S. Z. Lv, K. Y. Zhang, Y. Y. Zeng and D. P. Tang, Anal. Chem., 2018, 90, 7086–7093 CrossRef CAS PubMed.
- J. Shu, Z. Qiu, Z. Lin, G. Cai, H. Yang and D. Tang, Anal. Chem., 2016, 88, 12539–12546 CrossRef CAS PubMed.
- S. Xu, B. Dai, W. Zhao, L. Jiang and H. Huang, Anal. Chem. Acta, 2020, 1120, 1–10 CrossRef CAS.
- S. Ge, L. Zhang, Y. Zhang, F. Lan, M. Yan and J. Yu, Nanoscale, 2017, 9, 4366–4382 RSC.
- C. Li, Y. Liu, X. Zhou and Y. Wang, J. Mater. Chem. B, 2020, 8, 3582–3589 RSC.
- K. Cui, C. Zhou, B. Zhang, L. Zhang, Y. Liu, S. Hao, X. Tang, Y. Huang and J. Yu, ACS Appl. Mater. Interfaces, 2021, 13, 33937–33947 CrossRef CAS PubMed.
- Y. Zhang, J. Xu, S. Zhou, L. Zhu, X. Lv, J. Zhang, L. Zhang, P. Zhu and J. Yu, Anal. Chem., 2020, 92, 3874–3881 CrossRef CAS.
- H. Wang, C. Zhou, X. Sun, Y. Jian, Q. Kong, K. Cui, S. Ge and J. Yu, Biosens. Bioelectron., 2018, 117, 651–658 CrossRef CAS.
- R. Liu, E. M. McConnell, J. Li and Y. Li, J. Mater. Chem. B, 2020, 8, 3213–3230 RSC.
- Y. Soda, D. Citterio and E. Bakker, ACS Sens., 2019, 4, 670–677 CrossRef CAS PubMed.
- J. Sun, L. Li, S. Ge, P. Zhao, P. Zhu, M. Wang and J. Yu, ACS Appl. Mater. Interfaces, 2021, 13, 3645–3652 CrossRef CAS.
- R. Ota, K. Yamada, K. Suzuki and D. Citterio, Analyst, 2018, 143, 643–653 RSC.
- X. Yin, L. Liang, P. Zhao, F. Lan, L. Zhang, S. Ge and J. Yu, J. Mater. Chem. B, 2018, 6, 5795–5801 RSC.
- F. Liu, S. Ge, J. Yu, M. Yan and X. Song, Chem. Commun., 2014, 50, 10315–10318 RSC.
- C. Zhou, K. Cui, Y. Liu, S. Hao, L. Zhang, S. Ge and J. Yu, Anal. Chem., 2021, 93, 5459–5467 CrossRef CAS PubMed.
- F. Mustafa and S. Andreescu, ACS Sens., 2020, 5, 4092–4100 CrossRef CAS PubMed.
- Y. Liu, K. Cui, Q. Kong, L. Zhang, S. Ge and J. Yu, Biosens. Bioelectron., 2020, 148, 111839 CrossRef CAS PubMed.
- Q. Han, L. Li, W. Gao, Y. Shen, L. Wang, Y. Zhang, X. Wang, Q. Shen, Y. Xiong, Y. Zhou and Z. Zou, ACS Appl. Mater. Interfaces, 2021, 13, 15092–15100 CrossRef CAS PubMed.
- W. Lai, Z. Chen, S. Ye, Y. Xu, G. Xie, C. Kuang, Y. Li, L. Zheng and L. Wei, J. Hazard. Mater., 2021, 408, 124621 CrossRef CAS PubMed.
- Q. Pan, H. Zhang, Y. Yang and C. Cheng, Small, 2019, 15, e1900924 CrossRef PubMed.
- Y. Qiu, W. Liu, W. Chen, W. Chen, G. Zhou, P. C. Hsu, R. Zhang, Z. Liang, S. Fan, Y. Zhang and Y. Cui, Sci. Adv., 2016, 2, e1501764 CrossRef.
- P. Preechakasedkit, W. Siangproh, N. Khongchareonporn, N. Ngamrojanavanich and O. Chailapakul, Biosens. Bioelectron., 2018, 102, 27–32 CrossRef CAS PubMed.
- X. L. Liang, N. Bao, X. Luo and S. N. Ding, Biosens. Bioelectron., 2018, 117, 145–152 CrossRef CAS PubMed.
- G. Li, S. Li, Z. Wang, Y. Xue, C. Dong, J. Zeng, Y. Huang, J. Liang and Z. Zhou, Anal. Biochem., 2018, 547, 37–44 CrossRef CAS PubMed.
- J. Liu, J. Zhao, S. Li, L. Zhang, Y. Huang and S. Zhao, Talanta, 2017, 165, 107–111 CrossRef CAS PubMed.
|
This journal is © The Royal Society of Chemistry 2022 |
Click here to see how this site uses Cookies. View our privacy policy here.