DOI:
10.1039/D0QM00497A
(Research Article)
Mater. Chem. Front., 2021,
5, 355-367
A versatile synthetic approach to tunable dual-emissive Pdots with very small-size based on amphiphilic block copolymers for cell imaging†
Received
17th July 2020
, Accepted 2nd October 2020
First published on 6th October 2020
Abstract
Fluorescent polymer dots (Pdots) are a new type of efficient fluorophores with superior optical properties. However, their practical applications in bioimaging and biosensing are being hampered by their potential toxicity, poor water-solubility and the aggregation caused quenching effect. Herein, we report an effective strategy for the preparation of a series of new small-size aggregation-induced emission (AIE) active non-conjugated Pdots, which were self-assembled from three amphiphilic block copolymers, 4s-TPE-PCL-b-PNIPAM-Eu(III) (TPNE), 4s-TPE-PCL-b-PAA-Eu(III) (TPAE) and 4s-TPE-PCL-b-PVP-Eu(III) (TPVE). All of the blocks were synthesized by free-radical polymerization using a macroazoinitiator containing AIE fluorophore tetraphenylethene (TPE), poly(ε-caprolactone) (PCL) segment and azo group, respectively. The obtained TPNE, TPAE and TPVE Pdots had small diameters down to 5 nm and good monodispersity. The Pdots not only exhibited well-separated dual-wavelength emission by 430 and 615 nm upon excitation at 360 and 395 nm, but also displayed thermo-tunable and pH-dependent fluorescence properties, excellent photostability, good water-solubility, good biocompatibility, favorable photoreversibility and noncytotoxicity. More interestingly, the as-prepared three kinds of Pdots displayed reversibly distinct dual-color (blue-red) fluorescence in the targeted three cancer cells (HepG2, A549 and HeLa cells) by merely tuning the excitation wavelength, indicating their potential applications as sensor and multicolour imaging agents in cell biology and diagnostics.
Introduction
The rapid development of fluorescence imaging technology has become one of the most important tools for preclinical studies.1–3 Fluorescent organic nanoparticles are used as ideal contrast agents due to their biocompatibility, easy chemical modification, and high fluorescence quantum yield.4–7 Due to the high emissivity, excellent fluorescence brightness and low biotoxicity, polymer quantum dots (Pdots) have become a class of novel high-efficiency fluorescent sensors.8–12 In particular, stimuli-responsive Pdots have drawn much attention because of their advantages, such as excellent dispersity, fluorescence stability, biocompatibility in aqueous systems, and so on.13–15 These Pdots are able to change their physical structures and chemical properties reversibly or irreversibly responding to the external environment. Meanwhile, there are many reactive functional groups on the surface of Pdots, which can be attributed to integration with some fluorophores, drugs and targeting molecules through chemical or physical binding.16–18 However, the fluorescence of the traditional semiconducting Pdots would be quenched or weakened on the aggregation state due to the strong π–π intermolecular interaction. As a result, the notorious aggregation caused quenching (ACQ) effect severely limited the quality of applications in bioimaging.19–21 Fortunately, the discovery of some organic molecules with the aggregation induced emission (AIE) feature may help to effectively solve the fluorescence quenching of Pdots in the aggregation state.22–25 Moreover, AIE-active dyes have been intensively explored for application as excellent bioimaging agents owing to their outstanding optical features in the aggregated state as compared with traditional luminescent dyes during the last few decades. Therefore, AIE-active Pdots is a young and exciting research area to be explored for the development of excellent bioimaging agents.
In recent years, some semiconducting AIE-active Pdots have been reported as a novel type of ultra-bright fluorescence probe for sensing and imaging in biological systems.26–30 Meanwhile, a method was presented for the design of new highly fluorescent AIE-active Pdot systems by Yang-Hsiang Chan.31 However, these conjugated Pdots have poor water solubility and biocompatibility, which affects their applications in cell biology. In order to improve the biocompatibility of AIE-active Pdots, some researchers were committed to directly obtaining AIE Pdots by a complete self-assembly process after encapsulating the AIEgens into an amphiphilic polymer. For example, Bin Liu reported the first AIE-active nano-photosensitizer with near-infrared (NIR) emission and high 1O2 generation by encapsulating NIR AIEgens into amphiphilic copolymer DSPE-PEG-MAL.32 As a matter of fact, this kind of nonconjugated AIE Pdot has a number of advantages over semiconducting Pdot materials for biological applications. However, there is still a biological toxicity risk in view of the potential for AIEgen leakage during their application.33,34 Thus, it is highly desirable to develop a method for preparing AIE-active Pdots by chemical coupling of an AIE fluorophore to an amphiphilic block copolymer.
However, nonconjugated AIE-active Pdots formed by the self-assembly of amphiphilic polymer have been reported very rarely so far. Yan Zhang and co-authors reported a new AIE-active Pdot formed by the self-assembly of an AIE-conjugated block copolymer, which showed excellent stability and low toxicity in the application of cell imaging.35 But, the emission wavelengths of these nonconjugated AIE Pdots reported are mostly in the region of 400–550 nm. As a result, bioimaging with short-wavelength emission by using these Pdots has the defects of low penetration depth and spatial resolution. So, it is also required to develop red/near-infrared (NIR) AIE Pdots for high-quality bioimaging.
In our previous work, we prepared AIE-active Pdots by self-assembly of a 4-arm star homopolymer in aqueous medium and realized red/blue dual-alternating-color live cell imaging for the first time.36 However, the particle size of these Pdots was larger than 50 nm, which will greatly limit its application in specific labeling in vivo. Thus, in the present study, a novel AIE-based macro-azoinitiator (4s-TPE-PCL-AZO), various amphiphilic AIE-conjugated block copolymers and Eu(III)-based coordination complexes were successfully synthesized by ring-opening polymerization and free-radical polymerizations of different vinyl monomers. The nonconjugated AIE-active Pdots were subsequently prepared through the solution self-assembly and had small diameters down to 5 nm, which were too small in comparison with previous reported methods (Scheme 1). The biodegradable and biocompatible poly(ε-caprolactone) (PCL) was used as the hydrophobic segment, and the environmentally-sensitive Eu(III)-based coordination complexes of poly(N-isopropyl-acrylamide) (PNIPAM-Eu(III)), poly(acrylic acid) (PAA-Eu(III)), and polyvinylpyrrolidone (PVP-Eu(III)) were used as the hydrophilic segment, respectively. All of the Pdots exhibited excellent chemical and luminescence stability, which indicated no leakage of the AIE-active dye from the Pdots based on the stable chemical bonds between TPE and the copolymers. Meanwhile, the Pdots showed two well-separated emission peaks (blue at 430 nm and red at 615 nm) with a long wavelength interval, and possessed fluorescent-responsive properties. Moreover, these Pdots enable dual-alternating-color fluorescence for live cell imaging in three targeted cancer cells (HeLa, HepG2, A549 cells) by tuning of the excitation wavelength from 360 nm to 400 nm based on the low cytotoxicity, efficient cellular uptake and dual-alternating-color fluorescent emission of the Pdots. Therefore, this work could provide a practical and versatile approach to develop a kind of low-toxic, environmentally-sensitive, and fine-tuning emission AIE-active Pdots with extremely small size for fluorescent sensors and bioimaging probes.
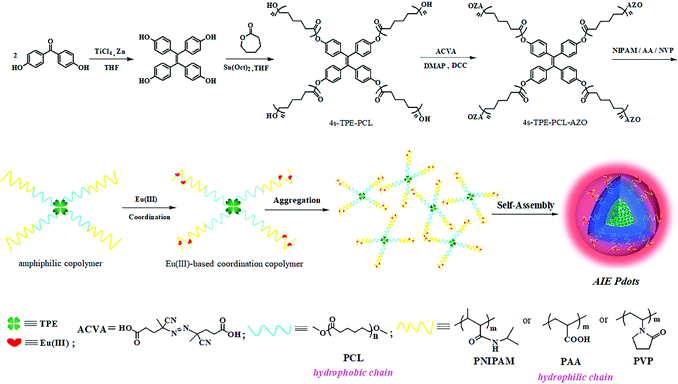 |
| Scheme 1 The synthetic route of TPNE/TPAE/TPVE Pdots and their schematic illustration of the self-assembly procedure. | |
Results and discussion
Design and synthesis of three amphiphilic block copolymers TPNE, TPAE and TPVE
Scheme 1 illustrates the synthetic routes to TPNE, TPAE and TPVE, and the detailed synthesis procedure and characterization are described in the ESI† (Fig. S1–S7). As shown in Scheme 1, three Eu(III)-based amphiphilic block copolymers 4s-TPE-PCL-b-PNIPAM-Eu(III) (TPNE), 4s-TPE-PCL-b-PAA-Eu(III) (TPAE) and 4s-TPE-PCL-b-PVP-Eu(III) (TPVE) having self-assembly ability were successfully prepared. Firstly, 1,1,2,2-tetrakis(4-hydroxyphenyl)-ethylene (TPE-4OH) was employed as an initiator for ring-opening polymerization of ε-caprolactone to prepare hydrophobic polymer 4s-TPE-PCL.37–39 A novel AIE-based macro-azoinitiator (4s-TPE-PCL-AZO) having an ability to initiate the radical polymerization of vinyl monomers was prepared via facile DCC-activated esterification of 4s-TPE-PCL with 4,4′-azobis (4-cyanovaleric acid) (ACVA) in mild conditions. The structure of 4s-TPE-PCL-AZO was identified by 1H NMR (Fig. S1, ESI†), FTIR (Fig. S2, ESI†), and GPC (Table 1), respectively. In Fig. S2 (ESI†), the appearances of a new peak of nitrile grouping (–C
N–) at 2100 cm−1 and a very strong peak of carbonyl (C
O) at 1730 cm−1 could be observed. In addition, an absorption peak at 835 cm−1 in the fingerprint region indicated the existence of 1,4-substituted benzene. The results supported the formation of macro-azoinitiator (4s-TPE-PCL-AZO). The number-average molecular weight (Mn) of 4s-TPE-PCL-AZO obtained by GPC was 9.5 × 103 and the polydispersity index (PDI) was 1.91 (Table 1).
Table 1 GPC data of 4s-TPE-PCL, 4s-TPE-PCL-AZO, TPN, TPA and TPV
Samples |
M
n (×104) |
M
w (×104) |
M
P (×104) |
M
z (×104) |
PDI (Mw/Mn) |
4s-TPE-PCL |
0.56 |
0.75 |
0.60 |
1.04 |
1.329 |
4s-TPE-PCL-AZO |
0.95 |
1.81 |
1.57 |
2.63 |
1.905 |
TPN |
4.62 |
8.20 |
2.58 |
26.0 |
1.774 |
TPA |
4.99 |
6.69 |
4.87 |
18.1 |
1.340 |
TPV |
7.37 |
9.24 |
5.71 |
19.0 |
1.254 |
Three different amphiphilic block copolymers 4s-TPE-PCL-b-PNIPAM (TPN), 4s-TPE-PCL-b-PAA (TPA) and 4s-TPE-PCL-b-PVP (TPV) subsequently were prepared by free-radical polymerization using 4s-TPE-PCL-AZO as a versatile initiator. TPN, TPA and TPV were identified by 1H NMR (Fig. S3–S5, ESI†), GPC (Table 1), and FTIR (Fig. 1). In the FTIR spectra of TPN, TPA and TPV, the peak at around 835 cm−1 in the fingerprint was ascribed to the characteristic absorption bands of 1,4-substituted benzene. Additionally, the FTIR spectra of TPN, TPA and TPV showed their characteristic absorption peaks of hydrophilic polymer segments, respectively. The methyl (–CH3) peaks at 2973 cm−1, and the peaks of the imino (–NH–) and carbonyl (C
O) groups at 3304 and 1649 cm−1 could be observed in the FTIR spectra of TPN. The FTIR spectra of TPA showed the peaks of the carboxyl groups at 3421 cm−1 (–OH) and 1720 cm−1 (C
O), respectively. TPV exhibited a carbonyl (C
O) peak at 1681 cm−1, C–N bond at 1285 cm−1 and methylene (–CH2–) peak at 2980 cm−1, respectively. These results indicated that three amphiphilic block copolymers were successfully synthesized. Moreover, the number-average molecular weights of TPN, TPA and TPV determined by GPC were 4.6 × 104, 5.0 × 104 and 7.4 × 104 with PDI of 1.77, 1.34 and 1.25, respectively (Table 1).
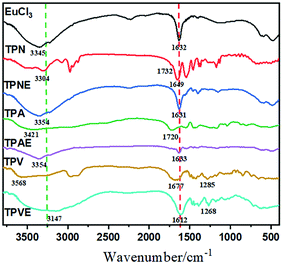 |
| Fig. 1 The IR spectra of EuCl3, copolymers and Eu(III)-based coordination copolymers. | |
Eu(III)-Based coordination copolymers TPNE, TPAE and TPVE were ultimately attained after Eu(III) ions were coordinated by O and N atoms in the side chains of the copolymers, respectively. Thus, copolymers TPNE, TPAE and TPVE had both the emission of the characteristic red europium fluorescence and the environmentally-sensitive properties of PNIPAM, PAA or PVP. The structures of TPNE, TPAE and TPVE were characterized by FTIR and X-ray photoelectron spectroscopy (XPS).
The IR spectrum of TPNE revealed the characteristic peaks of the hydrophobic fragment (4s-TPE-PCL) and hydrophilic polymer segments (PNIPAM) as shown in Fig. 1. However, compared with that of TPN, the stretching vibration of the acylamino group was significantly blue-shifted from 3304 to 3354 cm−1 and the peak of amide I was red-shifted from 1649 to 1631 cm−1 after TPN was coordinated with Eu(III), respectively. Meanwhile, the band of amide II at near 1540 cm−1 was greatly weakened and the bond at 1732 cm−1, which was ascribed to ester group absorption, disappeared in the IR spectrum of TPNE. The IR peak shift and intensity changes in Eu(III)-based coordination copolymer TPNE could be attributed to the strong complexation of Eu(III) with PNIPAM fragment of the copolymer with O and N donor groups, which resulted in the decreasing of the electron density and vibrational frequency of N–H and C
O.40,41 Similarly, compared with the IR spectrum of TPA, the peak of carboxyl groups of TPAE was also red-shifted from 1720 to 1633 cm−1, which was attributed to the strong complexation of Eu(III) with the PAA fragment of the copolymer with an O donor group. And, compared with the IR spectrum of TPV, the peak of carboxyl groups of TPVE was red-shifted from 1677 to 1612 cm−1 and the C–N bond peak of TPVE was red-shifted from 1285 to 1268 cm−1, which was attributed to the strong complexation of Eu(III) with the PVP fragment of the copolymer with O and N donor groups.
Moreover, XPS can be used to further confirm the structure of Eu(III)-based coordination copolymers by changes in the binding energy of the coordinate bond of O and N atoms in TPNE, TPAE and TPVE. The XPS spectra of three amphiphilic block copolymers and Eu(III)-based coordination copolymers were recorded as shown in Fig. 2. Meanwhile, the average binding energies of C1s, O1s, N1s, Eu3d and Eu4d are shown in Table 2. Comparison of the XPS spectra of TPN and TPNE, the N1s binding energy values for C–N bonds at 400.4 eV and the O1s binding energy values for C–O bonds at 532.1 eV in TPN increased by 0.9 and 1.1 eV in TPNE upon coordination with Eu(III) in Table 2, respectively. Furthermore, the new Eu3d peak at 1140.0 eV and Eu4d peak at 140.3 eV could be observed in the XPS spectrum of TPNE. The peak shifts and the appearance of Eu(III) peaks indicated that Eu(III) was coordinated by O and N atoms in TPN, which led to a decrease in the electron density of O1s and N1s atoms in the coordination copolymer.41 Similarly, it was also observed that the O1s binding energy values for C–O bonds at 534.4 eV in TPA increased by 1.4 eV. And the new Eu3d peak at 1141.0 eV and Eu4d peak at 138.6 eV appeared in the XPS spectrum of TPAE. The results indicated that Eu(III) was coordinated by O atoms of carboxyl group in the side chain of PAA. Meanwhile, the increasing of the N1s binding energy values (399.7 eV) and the O1s binding energy values (531.7 eV) in TPV was 1.1 and 1.2 eV in TPNE, respectively. Furthermore, a new Eu3d peak at 1135.8 eV and Eu4d peak at 133.5 eV appeared in the XPS spectrum of TPVE. The results indicated that Eu(III) was coordinated by O and N atoms in the side chain of PVP. Therefore, the combination of FTIR and XPS provided evidence of Eu(III) coordination to the O or N atoms in the side chains of copolymers TPN, TPA and TPV.
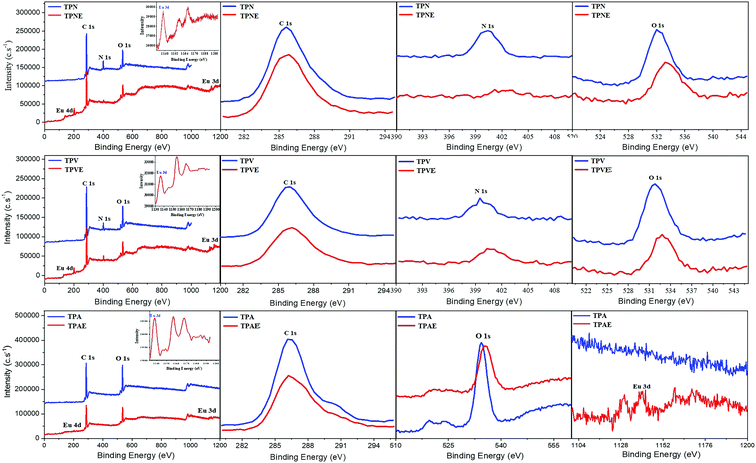 |
| Fig. 2 The XPS spectra of C1s, N1s, O1s and Eu3d of the copolymers. | |
Table 2 The binding energies of C(1s), O(1s), N(1s), Eu(3d) and Eu(4d) for the copolymers
Condition |
C(1s)/eV |
N(1s)/eV |
O(1s)/eV |
Eu(3d)/eV |
Eu(4d)/eV |
TPN |
285.5 |
400.4 |
532.1 |
— |
— |
TPNE |
285.5 |
401.3 |
533.2 |
1140.0 |
140.3 |
TPA |
286.3 |
— |
534.4 |
— |
— |
TPAE |
286.3 |
— |
535.8 |
1141.0 |
138.6 |
TPV |
286.0 |
399.7 |
531.7 |
— |
— |
TPVE |
286.1 |
400.8 |
532.9 |
1135.8 |
133.5 |
Besides, the elemental analyses of TPNE, TPAE and TPVE were performed using SEM-EDS (Fig. S6, ESI†) and EDX elemental mappings (Fig. S7, ESI†) in order to determine the content of major elements and elemental distributions. Obviously, Eu element appeared in the polymers after the Eu(III) was coordinated by oxygen and nitrogen atoms as shown in Fig. S6 (ESI†). Meanwhile, the elemental mappings showed that C, N, O, and Eu elements were homogeneously distributed throughout the sample, suggesting that Eu(III) was successfully modified on the polymers. Moreover, the percentages of the corresponding elements are listed in Table S1 (ESI†). The weight percentages of Eu element in TPNE, TPAE and TPVE were 11.7%, 9.4% and 15.3%, respectively. Then, the molar ratio of Eu to the coordinating unit in these polymers could be calculated according to the data of weight percentage of Eu element and the molecular weights of the polymers in Table 1. The molar ratios of Eu to the coordinating unit in TPNE, TPAE and TPVE were 0.39, 0.24 and 0.54, respectively.
Preparation and microstructure of three kinds of Pdots
Three kinds of AIE Pdots could be easily prepared by quickly adding H2O into the THF solution of TPNE, methanol solution of TPAE and THF solution of TPVE due to their amphiphilic properties. As shown in Scheme 1, these AIE Pdots formed by the self-assembly of amphiphilic 4-arm star block copolymers consisted of hydrophobic TPE and PCL as a core, and hydrophilic PNIPAM, PAA or PVP as a shell on the surface of the Pdots, which could create the biocompatible superhydrophilic nanostructured layer. Furthermore, Eu(III) could easily coordinate with a large number of functional amide groups or ester groups through oxygen and nitrogen atoms on the side-chains of PNIPAM, PAA or PVP. Therefore, there would be a benefit to allowing the application of hydrophobic AIEgens in a biological environment based on the advantages of soluble biocompatible Pdots by this method.
As shown in Fig. 3, all of the Pdots had regular spherical shape, good monodispersity and mean diameters in the range of 3–5 nm. The particle sizes of the TPNE, TPAE and TPVE Pdots were near 2.5 nm, 3.3 nm and 2.7 nm. All of the as-prepared Pdots by this method had small diameters down to 5 nm, which is smaller than previously reported methods.35,36
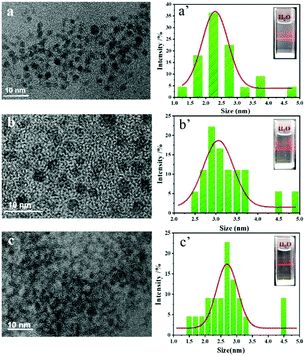 |
| Fig. 3 (a –c) TEM images of TPNE, TPAE and TPVE Pdots; (a′–c′) The particle size distribution histograms of TPNE, TPAE and TPVE Pdots. | |
Furthermore, it is important to establish the long-term structure, dimensional and fluorescence stabilities of Pdot materials. Compared with the weak physical adsorption in the non-conjugated Pdots by encapsulating AIE dyes into amphiphilic polymer, the dye-conjugated block copolymers could exhibit excellent chemical stability because of the strong covalent bonds between the AIEgens and copolymers. Firstly, three kinds of Pdots were chemically stable because there were no changes that could be found from the 1H NMR of the three block copolymers at the first day and the thirtieth days. Moreover, the three kinds of Pdots still exhibited regular sphere-like morphology and the mean diameters were around 5 nm with good dispersity, which ensured the dimensional stability of these Pdots (Fig. S8, ESI†). To examine the fluorescence stabilities of these Pdots, all of the TPNE, TPAE and TPVE Pdots were dialyzed against distilled water with membranes made of regenerated cellulose for 7 days. The fluorescence spectra of the three kinds of dialysates are shown in Fig. S9 (ESI†), respectively. Obviously, no fluorescence emission was found from these dialysates, which indicated that there was no dye leakage in these Pdots. So, all of the Pdots had good fluorescence stability.
Dual-emission spectroscopic characterization of the Pdots
Firstly, the effect of Eu(III) doping amount on the fluorescence of the Pdots in aqueous solution at a concentration of 20 mg mL−1 was examined. As Fig. 4a–c and Fig. S10 (ESI†) show, the doping amount of Eu(III) had a significant effect on the intensity ratio of blue and red light of the three Pdots. We observed that the blue emission of the Pdots at 430 nm gradually decreased and red emission at 615 nm increased with increasing Eu(III) concentration. Moreover, the increased fluorescence intensity ratio of I615/I430 (I615 and I430 were the fluorescence intensity of red emission and blue emission, respectively) could be observed by varying the excitation wavelength from 360 to 395 nm at a certain mass ratio of copolymers to Eu(III) (Fig. 4a–c). Obviously, the low ratio of I615/I430 at an excitation wavelength of 360 nm and the large ratio of I615/I430 at an excitation wavelength of 395 nm were expected to obtain the relatively pure blue light and red light. So, when the mass ratio of TPN to Eu(III) was 1
:
1, the as-prepared TPNE Pdots could simultaneously exhibit the optimum dual-color emission at two certain excitation wavelengths (360 nm and 395 nm). Similarly, the optimum mass ratio of TPA to Eu(III) was 1
:
2 for TPAE Pdots, and the optimum mass ratio of TPV to Eu(III) was 1
:
2 for TPVE Pdots.
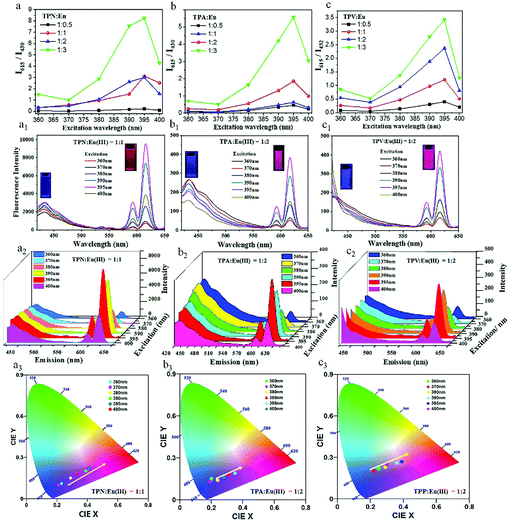 |
| Fig. 4 (a–c) The change trend of fluorescence intensity ratio of three Pdots in aqueous solution at 615 nm and 430 nm (or 432 nm) under the complex of copolymer and Eu(III) with different mass fractions (20 mg mL−1); (a1) the fluorescence spectra of TPNE in aqueous solution at different excitation wavelengths (the mass ratio of Eu to TPN is 1 : 1, 20 mg mL−1). (Inset: Photographs of TPNE in aqueous solution under irradiation of 365 nm and 395 nm ultraviolet (UV)); (b1) the fluorescence spectra of TPAE in aqueous solution at different excitation wavelengths (the mass ratio of Eu to TPA is 1 : 2, 20 mg mL−1). (Inset: Photographs of TPAE in aqueous solution under irradiation of 365 nm and 395 nm ultraviolet (UV)); (c1) the fluorescence spectra of TPVE in aqueous solution at different excitation wavelengths (the mass ratio of Eu to TPV is 1 : 2, 20 mg mL−1). (Inset: Photographs of TPVE in aqueous solution under irradiation of 365 nm and 395 nm ultraviolet (UV)); (a2–c2) the dual-emission fluorescence spectra of TPNE, TPAE and TPVE in aqueous solution at different excitations, respectively (20 mg mL−1); (a3–c3) the CIE chromaticity diagram of TPNE, TPAE and TPVE in aqueous solution at different excitations, respectively. | |
Subsequently, the photoluminescent properties of TPNE, TPAE and TPVE Pdots were investigated in water at room temperature in detail. The emission spectra of the three kinds of Pdots in aqueous solution at a concentration of 20 mg mL−1 are shown in Fig. 4a1–c1, respectively. It can be observed that all Pdots exhibited two strong luminescence bands centred at 430 and 615 nm, corresponding to the fluorescence of the blue-emitting TPE in the core of the Pdots and red-emitting Eu(III)-based coordination complexes (NIPAM-Eu(III), PAA-Eu(III) or PVP-Eu(III)) on the surface of these Pdots, respectively. Besides, when the excitation wavelength was in the range of 300 to 370 nm, the Pdots were mainly excited by blue fluorescence, and the Pdots mainly emitted red fluorescence in the range of 370–400 nm. In fact, the Pdots exhibited two red emission peaks centred around 590 and 615 nm, which were assigned to the 5D0 → 7F1 and 5D0 → 7F2 transitions of Eu(III) ions, respectively.42,43 Clearly, the most prominent red color emissions were the peaks at 615 nm by three Pdots between the two spectral peaks. Meanwhile, the low spectroscopic overlap between the emission of the donor (TPE) and absorption of the acceptor (Eu(III)-based coordination complexes) indicated the low FRET efficiency. Hence, the short- and long-wavelength fluorescence emission at 430 and 615 nm can be simultaneously observed. Furthermore, blue and red emissions were well separated by 185 nm, which was extremely beneficial for biosensing or bioimaging.
Interestingly, the fluorescence intensities of all Pdots could be finely tuned by switching the excitation wavelength. As shown in Fig. 4a2–c2, it was obvious that lower-energy emission at 430 nm was much higher than the higher-energy emission at 615 nm with 360 nm excitation. Subsequently, the red light intensity gradually increased and the blue light intensity decreased with the increase of excitation wavelength from 360 to 400 nm. Finally, the red emission peak was in a dominant position. In addition, as shown in the CIE chromaticity diagram in Fig. 4a3–c3, the different colors of light were produced on the standard swatches at different excitation wavelengths in the range of 360 to 400 nm. The colors of light were excessive from blue to red and could be adjusted according to the excitation wavelength.
Meanwhile, the fluorescence quantum yields (QY) of the Pdots for the blue and red emission were measured relative to quinine sulfate and rhodamine B in water, respectively. The QY for the blue and red emission are listed in Table S2 (ESI†). Obviously, the TPNE Pdots had higher QY for both blue (Φf,blue = 18.4%) and red (Φf,red = 45.5%) emission compared with those of TPAE (Φf,blue = 14.1%, Φf,red = 36.9%) and TPVE (Φf,blue = 15.5%, Φf,red = 42.8%) Pdots. The results indicated that PNIPAM was a very promising polymer matrix to prepare dye-modified biomaterials for sensing and imaging in biological systems. Moreover, every QY for red emission was nearly three times higher than that for blue emission, which could be attributed to the unique photophysical features of polymers doped with rare earths Eu(III) such as long luminescence lifetimes and high quantum yields. Besides, the average fluorescence lifetimes (τ) for the blue and red emission of the Pdots in water were also measured and listed in Table S2 (ESI†). Obviously, all of the Pdots had a relatively short fluorescence lifetime (τTPNE = 5.2 ns, τTPAE = 3.7 ns, τTPAE = 4.0 ns) for the blue emission and very long fluorescence lifetime (τTPNE = 1.7 ms, τTPAE = 0.7 ms, τTPAE = 1.4 ms) for the red emission. These favorable photophysical properties enabled the Pdots to act as ideal bioimaging agents.
Besides, the photo-stability is important for fluorescence probes in biological applications. In Fig. S11 (ESI†), it could be observed that both the short- and long-wavelength fluorescence intensities at 430 and 615 nm of TPNE, TPAE and TPVE Pdots in aqueous solutions slightly changed after 14 days, suggesting that all Pdots exhibited superior photostability. The could be ascribed to the effective polymer modification of dye TPE.
AIE behavior of the Pdots
Most of the traditional luminescent nano-sized particles suffer from a severe ACQ effect and their luminescence is quenched at high concentrations or in the aggregate state.44,45 However, AIE is a diametrically opposite phenomenon to ACQ. The AIE Pdot luminogens can effectively avoid the ACQ problem. To elucidate the AIE behavior of TPNE, TPAE and TPVE Pdots quantitatively, the experiments were arranged to investigate their fluorescence properties by adjusting the volume ratio of good solvents to poor solvents. After adding excess poor solvent, the polymer chains could aggregate in the solvent mixtures. Fluorescence spectra of the three Pdots in mixed solvents are shown in Fig. 5. Firstly, as shown in Fig. 5a, the fluorescence intensity of the TPNE Pdots emitted at 430 nm, corresponding to the fluorescence of the blue-emitting TPE, increased gradually in a nonlinear fashion with the increase of the fraction of poor solvent CH2Cl2 from 0% to 90%. And the fluorescence QY of TPNE for the fluorescence of the blue-emitting TPE was improved from 17.59% to 49.46% (Table S3, ESI†), displaying a typical AIE property. Meanwhile, a weak visible photoluminescence in pure THF and a strong blue light with 90% CH2Cl2 fraction could be observed by the naked eye under UV light in Fig. 5a1. The results obtained wereconsistent with the previous literature about TPE–polymer and could be best interpreted by the AIE feature of the TPE molecule in the core.37,38 When the Pdots were dissolved in a good solvent, the molecular chain was almost completely stretched, and the swinging space of the benzene ring on the TPE was relatively large. So, the vibration of the excited-state benzene ring in the Pdots dissipates part of the excitation energy in the form of thermal energy, resulting in lower utilization of fluorescence energy. With the increase of volume fraction of the poor solvent, the molecular spacing was reduced due to the aggregation, which hindered the vibration of the benzene ring of TPE. As a result, the fluorescence of the Pdots at 430 nm increased when the AIE molecule was limited to release energy in the form of thermal energy. So, the AIE-based polymer nanomaterial showed excellent fluorescence properties in the aggregation state and could ensure stronger fluorescence emission in the phagocytosis of cells, which is more conducive to broadening its application prospects in biology. However, there was no obvious fluorescence intensity and fluorescence QY (Φf,0% = 37.59%, Φf,90% = 37.87%, Table S3, ESI†) increase at 615 nm with the addition of CH2Cl2. Moreover, TPNE Pdots in the solid state also emitted bright blue light under UV light as shown in Fig. 5a2. All results indicated the excellent AIE feature of the TPNE Pdots at 430 nm.
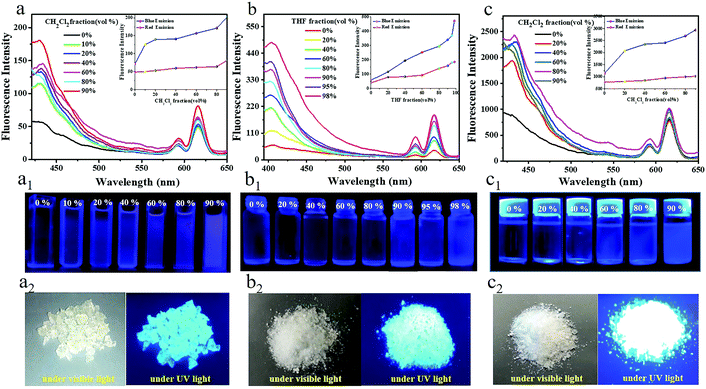 |
| Fig. 5 (a) Fluorescence spectra of TPNE Pdots with increasing fractions of CH2Cl2 in a CH2Cl2/THF mixed solvent (λex = 360 nm, 10 mg mL−1). (Inset: The relative change of blue emission at 430 nm and red emission at 615 nm against CH2Cl2 fraction, respectively.); (a1) the digital photographs of TPNE with different CH2Cl2 fraction in a CH2Cl2/THF mixed solvent under visible and UV light (365 nm). (a2) Photographic images of TPNE in the solid state under visible light and UV light; (b) fluorescence spectra of TPAE Pdots with increasing fractions of THF in THF/H2O mixed solvent (λex = 360 nm, 20 mg mL−1). (Inset: The relative change of blue emission at 430 nm and red emission at 615 nm against THF fraction, respectively.); (b1) the digital photographs of TPAE with different THF fraction in a THF/H2O mixed solvent under visible and UV light (365 nm). (b2) Photographic images of TPAE in the solid state under visible light and UV light. (c) Fluorescence spectra of TPVE Pdots with increasing fractions of CH2Cl2 in a CH2Cl2/THF mixed solvent (λex = 360 nm, 50 mg mL−1). (Inset: The relative change of blue emission at 430 nm and red emission at 615 nm against CH2Cl2 fraction, respectively.); (c1) the digital photographs of TPVE with different CH2Cl2 fractions in a CH2Cl2/THF mixed solvent under visible and UV light (365 nm). (c2) Photographic images of TPVE in the solid state under visible light and UV light. | |
Similarly, the fluorescence intensities and QY of the TPAE and TPVE Pdots at 430 nm also gradually increased with an increase in the volume fraction of the poor solvent THF or CH2Cl2 as displayed in Fig. 5(b, c) and (b1, c1) and Table S3 (ESI†). And, the red emissions and QY of the TPAE and TPVE Pdots at 615 nm did not apparently increase with the addition of poor solvent. Moreover, TPAE and TPVE Pdots in the solid state also showed bright blue luminescence under excitation of 365 nm UV light as shown in Fig. 5(b2, c2). Therefore, the results clearly indicated that all blue emission of the Pdots displayed a typical AIE property.
The temperature-sensitive property of the TPNE Pdots
PNIPAM is an interesting class of water-soluble temperature-sensitive hydrogels, which exhibits a coil to globule transition of the polymer chains with a lower critical solution temperature (LCST) of 32 °C.46–48 Because of the existence of a large number of PNIPAM chains as a shell on the surface of the TPNE Pdots, it was possible that the TPNE Pdots could exhibit thermo-sensitive properties. To investigate its temperature sensitivity, the optical transmittance change of TPNE was tested in aqueous solution at different temperatures in the range of 20–60 °C. As shown in Fig. 6a, the optical transmittance of the TPNE Pdots in aqueous solution at a concentration of 5 mg mL−1 decreased as the temperature increased and the lower critical solution temperature (LCST) was near 37 °C. Compared with pure PNIPAM, the TPNE Pdots exhibited a higher LCST, which was close to the normal body temperature of human beings. So, TPNE Pdots showed potential application in the field of stimuli-responsive biomaterials. Meanwhile, as shown in the insets in Fig. 6a, a TPNE Pdot aqueous solution was transparent at room temperature and became cloudy at a temperature higher than body temperature, which could be easily observed by the naked eye. Moreover, the clear aqueous solution of TPNE Pdots at 20 °C turned cloudy upon heating from 20 to 40 °C and further became transparent when cooling to 20 °C in Fig. 6b. More than 10 repeatable transparent/cloudy cycles under a temperature change could be determined, indicating the thermally reversible stimuli-responsive property of the TPNE Pdots.
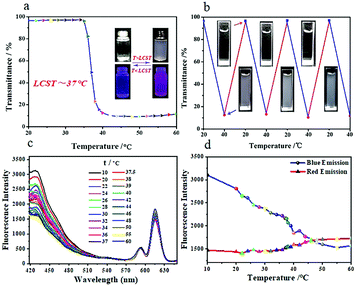 |
| Fig. 6 (a) Plot of transmittance of TPNE aqueous solution (5 mg mL−1) versus temperature in the range of 20–60 °C. (Inset: The digital photographs of TPNE aqueous solution at 20 °C (left) and 40 °C (right) under visible and UV light irradiation, respectively); (b) reversible phase transition diagram of TPNE aqueous solution at different temperatures; (c) fluorescent spectra of the TPNE Pdot aqueous solution (10 mg mL−1) with temperature increasing from 10 to 65 °C (λex = 360 nm); (d) the relative change of blue emission at 430 nm and red emission at 615 nm against temperature from 10 to 60 °C, respectively. | |
Besides, we examined whether and how the photoluminescent properties of the TPNE Pdots were affected by the phase transfer at different temperatures. The fluorescence intensity changes of TPNE Pdots in aqueous solution (10 mg mL−1) in the temperature range of 10–60 °C are shown in Fig. 6(c and d). Firstly, for the blue luminescence centered at 430 nm corresponding to the fluorescence of TPE, there are three different change trends in the temperature ranges of 10 to 60 °C in Fig. 6d, respectively. The blue emission peak initially decreased in intensity with increasing temperature from 10 to 39 °C, but then became suddenly increased near 40 °C. Subsequently, the fluorescence intensity decreased once from 42 to 60 °C. The acute temperature (39 °C) in the temperature-regulated fluorescence response of TPNE Pdot solution was close to its LCST. The results indicated that the fluorescence response of the TPNE Pdots originated from the phase transfer of TPNE. At a temperature as low as the LCST, the inter- and intra-molecular motions of TPE as a core in the Pdots were quickened with temperature increase, inducing a decrease of fluorescence intensity. Meanwhile, the degree of TPE aggregation was decreased due to the more stretched state of the polymer chains, originating from thermal induced chain conformational transformations. Therefore, the fluorescence intensity was found to decrease continuously with temperature increase from 10 to 39 °C. However, at temperatures around the LCST, the onset of the phase transition resulted in the aggregation formation of TPE molecules, which induced sudden fluorescence enhancement due to the AIE property of TPE. This result agreed with the work in our previous reports.38 Soon afterwards, the fluorescence intensity started to decrease once more at the higher temperature (40 °C) because the effect of quick molecular motions of TPE was stronger than its AIE effect. All results indicated that the temperature sensitivity of PNIPAM could impart the ability of temperature-responsive tunable fluorescence to the TPNE Pdots. However, the red emission intensity of Eu(III)-based coordination complexes (PNIPAM-Eu(III)) centred at 615 nm constantly and weakly increased with increasing temperature in the range of 10–60 °C, suggesting the stable red-emission at different temperatures. The above results implied not only that the inherent temperature sensitive property of PNIPAM was retained but also that one more function of thermo-tunable fluorescence was added to the TPNE Pdots.
The pH-sensitive property of the TPAE Pdots
In recent years, pH-sensitive amphiphilic block copolymers have shown potential applications in targeted drug delivery to tumor sites.49 Since PAA is a typical pH responsive weak polyelectrolyte polymer and can exhibit totally different conformation in acidic and basic environments, we investigated the influence of pH on the fluorescence of the TPAE Pdots. As shown in Fig. 7, the blue emission intensities of the TPAE Pdot aqueous solution at a concentration of 20 mg mL−1 initially increased from pH 1 to 8 and then decreased in the range of 8 to 14. It is well known that PAA undergoes contraction in acidic solution due to the protonation of –COOH on the PAA side chain, which leads to the decrease of the hydrophilicity of the polymer. So, the hydrophilic outer shell of the Pdots continuously shrank with increasing acidity and embedded the TPE luminophore doped inside the Pdots based on the existence of a large number of PAA chains as a shell on the surface of the TPAE Pdots. As a result, the limitation of TPE aggregation resulted in decreasing fluorescence intensity at low pH. In contrast, when the pH is gradually increased to a weakly basic condition, most of the –COOH are deprotonated and existed in the form of –COO−. The hydrophilic outer shell of the Pdots was in the swelling state due to the strong hydrogen bond between the deprotonated –COO− and water, which prevented the polymer chain outside from agglomerating. Therefore, the TPE luminophore was released and the fluorescence intensity was increased. However, strong electrostatic repulsion between the carboxylate groups (–COO−) in the outer shell of the Pdots prevented the TPE luminophores from approaching each other once more under a strongly alkaline environment, inducing a decrease of fluorescence intensity at pH 8–14. Differently, the change of red emission intensity of the Eu(III)-based coordination complexes (PAA-Eu(III)) centred at 615 nm was weak and stable in a wide range of pH. The above results also implied not only that the inherent pH sensitive property of PAA was retained but also that one more function of pH-tunable fluorescence was added to the TPAE Pdots. Furthermore, we know that the normal physiological environment of the human body has a pH of 7.4 and the pH of the tumor site is weakly acidic (∼6.8). It should be noted that the fluorescence intensity of the TPAE Pdots was pH dependent with the highest at pH 7–8. So, the TPAE Pdots were an ideal material used in the field of life sciences.
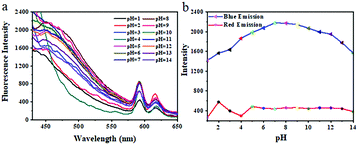 |
| Fig. 7 (a) Fluorescence spectra of TPAE aqueous solutions with different pH values; (b) the relative change of blue emission at 430 nm and red emission at 615 nm in different pH solutions, respectively. (λex = 360 nm, 20 mg mL−1). | |
The dual temperature/pH-sensitive properties of the TPVE Pdots
The smart AIE-active polymers have been widely used in biomedical applications such as biosensors and tissue engineering but the development of AIE-based multistimuli-responsive polymers still poses a limitation. Therefore, the design and development of new multi-responsive fluorescence materials based on AIE is necessary for biomedical applications.
PVP is widely used in biomedical fields due to its excellent chemical stability, solubility and low toxicity.50,51 Importantly, PVP is both temperature sensitive and pH sensitive.52 Similarly, it is also possible that TPVE Pdots could exhibit dual temperature/pH-sensitive properties based on the existence of a large number of PVP chains as a shell on the surface of the TPVE Pdots. We firstly tested the optical transmittance changes of the TPVE Pdot aqueous solution (5 mg mL−1) in the temperature range of 10–56 °C. As shown in Fig. 8a, its optical transmittance decreased as the temperature increased and the LCST was also near 32 °C. Meanwhile, as shown in the insets in Fig. 8a, the TPVE Pdot aqueous solution was transparent at room temperature and became cloudy at a temperature higher than LCST, which could be easily observed by the naked eye. Moreover, the clear aqueous solution of TPVE Pdots at 20 °C turned cloudy upon heating from 20 to 40 °C and further became transparent when cooling to 20 °C as shown in Fig. 8b. More than 5 repeatable transparent/cloudy cycles under a temperature change could be determined, indicating the thermally reversible stimuli-responsive property of the TPVE Pdots.
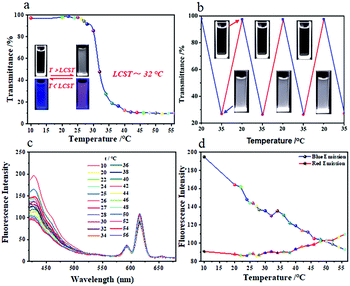 |
| Fig. 8 (a) Plot of transmittance of TPVE aqueous solution (5 mg mL−1) versus temperature in the range of 10–56 °C. (Inset: The digital photographs of TPVE aqueous solution at 20 °C (left) and 40 °C (right) under visible and UV light irradiation, respectively); (b) reversible phase transition diagram of TPVE aqueous solution at different temperatures; (c) fluorescent spectra of the TPVE Pdot aqueous solution (5 mg mL−1) with temperature increasing from 10 to 56 °C (λex = 360 nm); (d) the relative change of blue emission at 430 nm and red emission at 615 nm against temperatures from10 to 56 °C, respectively. | |
Furthermore, the fluorescence changes of the TPVE Pdot aqueous solution (5 mg mL−1) in the temperature range of 10–56 °C were also examined, and are shown in Fig. 8(c and d). The results showed that the fluorescence change at different temperatures was similar to that of TPNE Pdots. There were also three different change trends in the temperature range of 10 to 56 °C in Fig. 8d, respectively. The blue emission peak initially decreased in intensity with the temperature increase from 10 to 32 °C, but then became suddenly increased from 32 to 34 °C. Subsequently, the fluorescence intensity decreased from 34 to 56 °C once more. Similarly, the acute temperature (32 °C) in the temperature-regulated fluorescence response of the TPVE Pdot solution was consistent with its LCST, which indicated that the fluorescence response of the TPNE Pdots originated from the phase transfer of TPVE. Besides, the fluorescence intensity at 615 nm corresponding to the fluorescence of Eu(III)-based coordination complexes (PVP-Eu(III)) showed changes weakly with increasing temperature from 10 to 56 °C, indicating the stable red-emission at different temperatures. The results suggested not only that the inherent temperature sensitive property of PVP was retained but also that one more function of thermo-tunable fluorescence was added to the TPNE Pdots.
Then, we investigated the influence of pH on the fluorescence of TPVE Pdots in aqueous solution. As shown in Fig. 9, both the blue and red emission intensities in aqueous solution at a concentration of 20 mg mL−1 were pH dependent with the highest at pH 7. It is well known that the pH sensitive property of PVP is based on the protonation of tertiary amine groups on the PVP side chain under acidic conditions. The swelling of the polymer chains due to the protonation resulted in changes of fluorescence intensity in the pH range of 1 to 7. In addition, the hydrolysis of PVP was found to be easily occurring under basic conditions and poly(N-vinyl-γ-sodium aminobutyrate) (PVSA) is the product of its hydrolysis. The formation of PVSA largely enhanced the solubility of the TPVE Pdots, which led to the decreasing of the degree of TPE molecular aggregation. So, the limitation of TPE aggregation resulted in decreasing fluorescence intensity at pH 7–13. We know that the pH values in normal tissues are different from those in pathological tissues. Therefore, this interesting pH-tunable fluorescence response allowed its application in drug delivery.
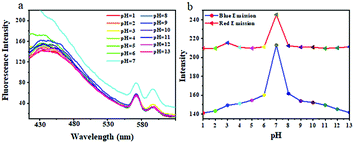 |
| Fig. 9 (a) Fluorescence spectra of TPVE in different pH solutions; (b) the relative change of blue emission at 435 nm and red emission at 615 nm in different pH solutions, respectively. (λex = 360 nm, 20 mg mL−1). | |
Cytotoxicity of the three kinds of Pdots
Water-soluble fluorescent polymer nanomaterials are expected to be excellent candidates for both in vitro and in vivo biological imaging of cells due to their small size, good stability and unique optical properties. In biomedical applications, the assessment of cytotoxicity is a necessary step in the evaluation of their biocompatibility. To investigate the cytotoxicity of TPNE, TPAE and TPVE Pdots, the effects of the three Pdots on HepG2, A549 and HeLa cell viability were tested by methylthiazolyldiphenyltetrazolium bromide (MTT) colorimetric assay in this study. As shown in Fig. 10, all of the cell viabilities of the three Pdots remained above 95% and no apparent cytotoxicity was observed after incubation for 48 h with different concentrations of Pdots (50 μg mL−1 to 400 μg mL−1), which indicated the noncytotoxicity of all AIE Pdots. Meanwhile, cytotoxicity data in Table S4 (ESI†) also showed that all three Pdots had relatively low or no cytotoxicities against HepG2, A549 and HeLa cells with IC50 (half-maximal inhibition) values higher than 630 μg mL−1, 500 μg mL−1 and 600 μg mL−1, respectively.
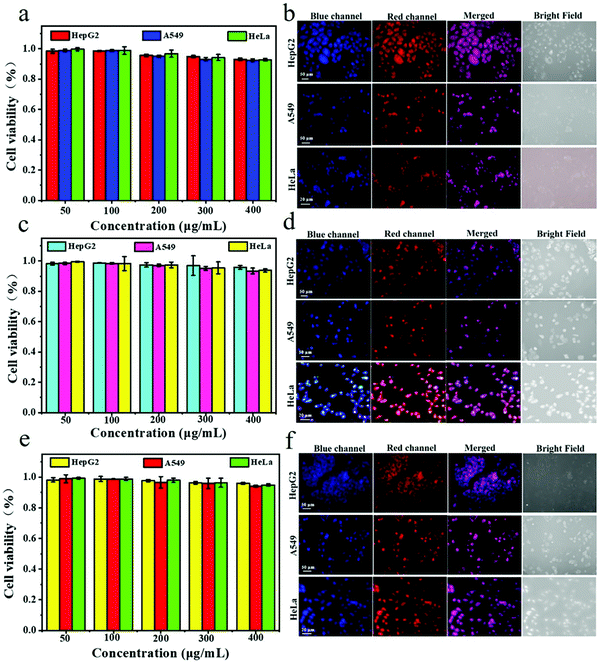 |
| Fig. 10 Dual-color co-localization images of HeLa, A549 and HepG2 cells. (a, c and e) Cytotoxic effects of TPNE, TPAE and TPVE Pdots against HeLa, A549 and HepG2 cells upon 48 h of incubation. Control: three cells in the absence of the three Pdots, respectively; (b, d and f) confocal laser scanning microscopy (CLSM) images of HeLa, A549 and HepG2 cells treated with TPNE, TPAE and TPVE Pdots at 37 °C for 24 h under excitation wavelength of 364 nm and 405 nm. The concentrations of Pdots incubated with cells was 100 μg mL−1. The scale bar represents 50 μm for A549 and HepG2 cells, and 20 μm for HeLa cells, respectively. | |
The dual-color living-cell imaging of three Pdots
Multicolor and photoswitchcable fluorescent polymer nanoparticles with small size, good photostability and biocompatibility are still scarce for bioimaging. TPNE, TPAE and TPVE Pdots emitted a blue fluorescence under 360 nm excitation and a red fluorescence under 395 nm excitation. Consequently, the three kinds of Pdots enabled alternating red-blue dual-channel images. We investigated the photoswitchable capability of the three Pdots in the living HepG2, A549 and HeLa cells by using confocal laser scanning microscopy (CLSM). Three cells were incubated with TPNE, TPAE and TPVE Pdots at a concentration of 100 μg mL−1 for 24 hours and their CLSM images are shown in Fig. 10b, d and f, respectively. By confocal microscopy, we found that all of the Pdots were effectively internalized into cells by endocytosis and mainly transported into the cytoplasm. All of the tumor cells showed strong fluorescence in the cytoplasm from three Pdots under UV light irradiation. Most importantly, bright fluorescence signals could be observed in the two channels (blue and red), which identified the ability of the probe to enter into living cells and maintained its dual-emission characteristic. The bright blue and red fluorescence signals in the cells derived from the emission of AIEgens TPE excited at 360 nm and Eu(III) complex excited at 395 nm in the Pdots, respectively. In addition, this fluorescence color switching was reversible in the cytoplasmic cell compartment under the irradiation of two different wavelength lights. Furthermore, the blue and red channel overlay resulted in purple color in areas where the true Pdot probes reside and the single-color areas, regardless of blue or red fluorescence, represent false positives. Therefore, it was possible to quickly achieve fine-tuning dual emission in a complex biological environment by simply adjusting the excited wavelength of the three Pdots. These results confirmed the potential usefulness of dual-color TPNE, TPAE and TPVE Pdots as smart fluorescent probes in biological systems.
Conclusions
In summary, a versatile approach was developed for the preparation of various stimuli-responsive dual-color Pdots with very small size through the solution self-assembly of amphiphilic AIE-conjugated block copolymers, which were synthesized by ring-opening and free-radical polymerization methods. All of the Pdots (TPNE, TPAE and TPVE Pdots) showed excellent water-solubility and had small diameters down to 5 nm, which is too small in comparison with previous reported methods. These Pdots exhibited well-separated dual emission with emission maxima at 430 and 615 nm, corresponding to the fluorescence of the AIE-active TPE in the core and Eu(III)-based coordination complexes on the surface, respectively. Moreover, the temperature-sensitive TPNE Pdots exhibited a lower LCST (∼37 °C) close to body temperature and thermo-tunable fluorescence properties. The pH-sensitive TPAE Pdots showed pH-dependent fluorescence with the highest at pH 7–8. And the temperature/pH dual stimuli-responsive TPVE Pdots exhibited thermo-tunable fluorescence similar to that of TPNE Pdots and pH-tunable fluorescence response with the highest at pH 7 similar to that of TPAE Pdots. Additionally, their non-cytotoxicity, AIE property and photostability provided further benefits in the field of biological imaging. Notably, all Pdots displayed reversibly distinct dual-color (blue-red) fluorescence in three targeted cancer cells (HepG2, A549 and HeLa cells) by merely tuning the excitation wavelength. Hence, this study provides a versatile approach for the preparation of novel environmentally-sensitive, photoswitchable dual-color and noncytotoxic extreme Pdots with very small particles (<5 nm), which are particularly suitable for applications in the field of fluorescence sensors and imaging in biomedicine.
Conflicts of interest
The authors declare no competing financial interests.
Acknowledgements
This project was supported by the National Natural Science Foundation of China (No. 21965032; 21761032; 51302222; 1363021), the Scientific Research Project of Higher Learning Institution in Gansu Province (No. 2018B-057) and the Key Laboratory of Ecological Environment Related Polymer Materials, Ministry of Education Open Fund (No. KF-18-05).
References
- S. K. Sun, H. F. Wang and X. P. Yan, Engineering Persistent Luminescence Nanoparticles for Biological Applications: From Biosensing/Bioimaging to Theranostics, Acc. Chem. Res., 2018, 51(5), 1131 CrossRef CAS.
- H. X. Yang, J. B. Zhuang, N. Li, Y. Li, S. Y. Zhu, J. X. Hao, J. Y. Xin and N. Zhao, Efficient near-infrared photosensitizer with aggregation-induced emission characteristics for mitochondria-targeted and image-guided photodynamic cancer therapy, Mater. Chem. Front., 2020, 4(7), 2064 RSC.
- Y. T. Gao, H. K. Zhang, Z. Y. He, F. Fang, C. Wang, K. H. Zeng, S. B. Gao, F. L. Meng, L. Luo and B. Z. Tang, Multicationic AIEgens for unimolecular photodynamic theranostics and two-photon fluorescence bioimaging, Mater. Chem. Front., 2020, 4(6), 1623 RSC.
- X. L. Guan, L. Wang, Z. F. Li, M. N. Liu, K. L. Wang, B. Lin, X. Q. Yang, S. J. Lai and Z. Q. Lei, Preparation of Multi-stimulus Responsive Polymer Nanospheres Based on AIE Effect and Its Cell Tracing Application, Acta Chim. Sin., 2019, 77(10), 1036 CrossRef CAS.
- H. Y. Liu, P. J. Wu, S. Y. Kuo, C. P. Chen, E. H. Chang, C. Y. Wu and Y. H. Chan, Quinoxaline-based Polymer Dots with Ultrabright Red to Near-infrared Fluorescence for In Vivo Biological Imaging, J. Am. Chem. Soc., 2015, 137(32), 10420 CrossRef CAS.
- C. S. Ke, C. C. Fang, J. Y. Yan, P. J. Tseng, J. R. Pyle, C. P. Chen, S. Y. Lin, J. X. Chen, X. J. Zhang and Y. H. Chan, Molecular Engineering and Design of Semiconducting Polymer Dots with Narrow-band, Near-infrared Emission for In vivo Biological Imaging, ACS Nano, 2017, 11(3), 3166 CrossRef CAS.
- B. R. Smith and S. S. Gambhir, Nanomaterials for In Vivo Imaging, Chem. Rev., 2017, 117(3), 901 CrossRef CAS.
- C. P. Chen, Y. C. Huang, S. Y. Liou, P. J. Wu, S. Y. Kuo and Y. H. Chan, Near-infrared Fluorescent Semiconducting Polymer Dots with High Brightness and Pronounced Effect of Positioning Alkyl Chains on the Comonomers, ACS Appl. Mater. Interfaces, 2014, 6(23), 21585 CrossRef CAS.
- L. Q. Xiong, Y. X. Guo, Y. M. Zhang and F. W. Cao, Highly luminescent and photostable near-infrared fluorescent polymer dots for long-term tumor cell tracking, J. Mater. Chem. B, 2016, 4(2), 202 RSC.
- H. Piwonski, W. Li, Y. Wang, T. Michinobu and S. Habuchi, Improved Fluorescence and Brightness of Near-Infrared and Shortwave Infrared Emitting Polymer Dots for Bioimaging Applications, ACS Appl. Mater. Interfaces, 2020, 2(2), 569 CAS.
- R. Gupta, W. J. Peveler, K. Lix and R. Algar, Comparison of Semiconducting Polymer Dots and Semiconductor Quantum Dots for Smartphone-Based Fluorescence Assays, Anal. Chem., 2019, 91(17), 10955 CrossRef CAS.
- Z. Zhang, X. F. Fang, Z. H. Liu, H. C. Liu, D. D. Chen, S. Q. He, J. Zheng, B. Yang, W. P. Qin, X. J. Zhang and C. F. Wu, Semiconducting Polymer Dots with Dual-Enhanced NIR-IIa Fluorescence for Through-Skull Mouse-Brain Imaging, Angew. Chem., Int. Ed., 2020, 59(9), 3691 CrossRef CAS.
- S. Mura, J. Nicolas and P. Couvreur, Stimuli-responsive nanocarriers for drug delivery, Nat. Mater., 2013, 12(11), 991 CrossRef CAS.
- X. L. Guan, Z. F. Li, L. Wang, M. N. Liu, K. L. Wang, X. Q. Yang, Y. L. Li, L. L. Hu, X. L. Zhao, S. J. Lai and Z. Q. Lei, Preparation of AIE Polymer Dots (Pdots) Based on Poly(N-vinyl-2-pyrrolidone)-Eu(III) Complex and Dual-color Live Cell Imaging, Acta Chim. Sin., 2019, 77(12), 1268 CrossRef CAS.
- C. H. Li and S. Y. Liu, Polymeric assemblies and nanoparticles with stimuli-responsive fluorescence emission characteristics, Chem. Commun., 2012, 48(27), 3262 RSC.
- B. Yang, Y. Li, X. Sun, X. R. Sun, X. L. Meng, P. Chen and N. Liu, A pH-responsive drug release system based on doxorubicin conjugated amphiphilic polymer coated quantum dots for tumor cell targeting and tracking, J. Chem. Technol. Biol., 2013, 88(12), 2169 CAS.
- Y. H. Chan, C. F. Wu, F. M. Ye, Y. H. Jin, P. B. Smith and D. T. Chiu, Development of Ultrabright Semiconducting Polymer Dots for Ratiometric pH Sensing, Anal. Chem., 2011, 83(4), 1448 CrossRef CAS.
- P. J. Wu, S. Y. Kuo, Y. C. Huang, C. P. Chen and Y. H. Chan, Polydiacetylene-Enclosed Near-Infrared Fluorescent Semiconducting Polymer Dots for Bioimaging and Sensing, Anal. Chem., 2014, 86(10), 4831 CrossRef CAS.
- L. Y. Zong, Y. J. Xie, C. Wang, J. R. Li, Q. Q. Li and Z. Li, From ACQ to AIE: the suppression of the strong π–π interaction of naphthalene diimide derivatives through the adjustment of their flexible chains, Chem. Commun., 2016, 52(77), 11496 RSC.
- H. B. Chen, X. F. Fang, Y. Jin, X. Hu, M. Yin, X. J. Men, N. Chen, C. H. Fan, D. T. Chiu, Y. Z. Wan and C. F. Wu, Semiconducting Polymer Nanocavities: Porogenic Synthesis, Tunable Host-Guest Interactions, and Enhanced Drug/siRNA Delivery, Small, 2018, 14(21), 1800239 CrossRef.
- Y. Rong, C. F. Wu, J. B. Yu, X. J. Zhang, F. M. Ye, M. Zeigler, M. E. Gallina, I. C. Zhang, Y. Wu, Y. H. Chan, W. Sun, K. Uvdal and D. T. Chiu, Multicolor Fluorescent Semiconducting Polymer Dots with Narrow Emissions and High Brightness, ACS Nano, 2013, 7(1), 376 CrossRef CAS.
- H. B. Wang and G. Y. Liu, Advances in Luminescent Materials with Aggregation-induced emission (AIE) Properties for Biomedical Applications, J. Mater. Chem. B, 2018, 6(24), 4029 RSC.
- K. Li, Z. S. Zhu, P. Q. Cai, R. R. Liu, N. Tomczak, D. Ding, J. Liu, W. Qin, Z. J. Zhao, Y. Hu, X. D. Chen, B. Z. Tang and B. Liu, Organic Dots with Aggregation-Induced Emission (AIE Dots) Characteristics for Dual-Color Cell Tracing, Chem. Mater., 2013, 25(21), 4181 CrossRef CAS.
- D. Ding, K. Li, B. Liu and B. Z. Tang, Bioprobes Based on AIE Fluorogens, Acc. Chem. Res., 2013, 46(11), 2441 CrossRef CAS.
- J. Qi, C. W. Sun, A. Zebibula, H. Q. Zhang, R. T. K. Kwok, X. Y. Zhao, W. Xi, J. W. Y. Lam, J. Qian and B. Z. Tang, Real-Time and High-Resolution Bioimaging with Bright Aggregation-Induced Emission Dots in Short-Wave Infrared Region, Adv. Mater., 2018, 30(12), 1706856 CrossRef.
- Z. Y. Wang, Y. Q. Feng, N. N. Wang, Y. X. Cheng, Y. W. Quan and H. X. Ju, Donor-Acceptor Conjugated Polymer Dots for Tunable Electrochemiluminescence Activated by Aggregation-Induced Emission-Active Moieties, J. Phys. Chem. Lett., 2018, 9(18), 5296 CrossRef CAS.
- Y. H. Chan, Y. H. Jin, C. F. Wu and D. T. Chiu, Copper(II) and iron(II) ion sensing with semiconducting polymer dots, Chem. Commun., 2011, 47(10), 2820 RSC.
- S. T. Chen, S. Cui, R. X. Du and M. Liu, Simultaneous near-infrared and green fluorescence from single conjugated polymer dots with aggregation-induced emission fluorogen for cell imaging, J. Mater. Chem. B, 2018, 6(47), 7871 RSC.
- Z. Zhang, D. D. Chen, Z. H. Liu, D. Wang, J. T. Guo, J. Zheng, W. P. Qin and C. F. Wu, Near-Infrared Polymer Dots with Aggregation-Induced Emission for Tumor Imaging, ACS Appl. Polym. Mater., 2020, 2(1), 74 CrossRef CAS.
- J. Yu, Y. Rong, C. T. Kuo, X. H. Zhou and D. T. Chiu, Recent Advances in the Development of Highly Luminescent Semiconducting Polymer Dots and Nanoparticles for Biological Imaging and Medicine, Anal. Chem., 2017, 89(1), 42 CrossRef CAS.
- W. K. Tsai, C. I. Wang, C. H. Liao, C. N. Yao, T. J. Kuo, M. H. Liu, C. P. Hsu, S. Y. Lin, C. Y. Wu, J. R. Pyle, J. X. Chen and Y. H. Chan, Molecular design of near-infrared fluorescent Pdots for tumor targeting: aggregation-induced emission versus anti-aggregation-caused quenching, Chem. Sci., 2019, 10(1), 198 RSC.
- W. B. Wu, D. Mao, F. Hu, S. D. Xu, C. Chen, C. J. Zhang, X. M. Cheng, Y. Y. Yuan, D. Ding, D. L. Kong and B. Liu, A Highly Efficient and Photostable Photosensitizer with Near-Infrared Aggregation-Induced Emission for Image-Guided Photodynamic Anticancer Therapy, Adv. Mater., 2017, 29(33), 1700548 CrossRef.
- Y. C. Chen, W. J. Zhang, Y. J. Cai, R. T. K. Kwok, Y. B. Hu, J. W. Y. Lam, X. G. Gu, Z. K. He, Z. Zhao, X. Y. Zheng, B. Chen, C. Gui and B. Z. Tang, AIEgens for dark through-bond energy transfer: design, synthesis, theoretical study and application in ratiometric Hg2+ sensing, Chem. Sci., 2017, 8(3), 2047 RSC.
- H. T. Feng, X. G. Gu, J. W. Y. Lam, Y. S. Zheng and B. Z. Tang, Design of multi-functional AIEgens: tunable emission, circularly polarized luminescence and self-assembly by dark through-bond energy transfer, J. Mater. Chem. C, 2018, 6(33), 8934 RSC.
- Y. Zhang, Y. J. Chen, X. Li, J. B. Zhang, J. L. Chen, B. Xu, X. Q. Fu and W. J. Tian, Folic acid-functionalized AIE Pdots based on amphiphilic PCL-b-PEG for targeted cell imaging, Polym. Chem., 2014, 5, 3824 RSC.
- X. L. Guan, B. C. Lu, Q. J. Jin, Z. F. Li, L. Wang, K. L. Wang, S. J. Lai and Z. Q. Lei, The AIE-Active Fluorescent Non-conjugated Polymer Dots for Dual-Alternating-Color Live Cell Imaging, Ind. Eng. Chem. Res., 2018, 57(44), 14889 CrossRef CAS.
- X. L. Guan, L. Meng, Q. J. Jin, B. C. Lu, Y. B. Chen, Z. F. Li, S. J. Lai and Z. Q. Lei, A New Thermo-, pH-, and CO2-Responsive Fluorescent 4-Arm Star Polymer with Aggregation-Induced Emission for Long-Term Cellular Tracing, Macromol. Mater. Eng., 2018, 303(6), 1700553 CrossRef.
- X. L. Guan, D. H. Zhang, L. Meng, Y. Zhang, T. M. Jia, Q. J. Jin, Q. B. Wei, D. D. Lu and H. C. Ma, Various Tetraphenylethene-Based AIEgens with Four Functional Polymer Arms: Versatile Synthetic Approach and Photophysical Properties, J. Ind. Eng. Chem., 2017, 56(3), 680 CrossRef CAS.
- X. L. Guan, D. H. Zhang, T. M. Jia, Y. Zhang, L. Meng, Q. J. Jin, H. C. Ma, D. D. Lu, S. J. Lai and Z. Q. Lei, Intriguingly Tuning Fluorescence of AIEgen Using Responsive Polyelectrolyte Microspheres, RSC Adv., 2016, 6(109), 107622 RSC.
- Y. Cai, M. Q. Chen, H. N. Ji, X. H. Huang and J. Shen, Synthesis and Spectroscopic Studies of Eu ∼(3+) Complex with Poly(N-vinyl acetamide), Acta. Polym. Sin., 2003, 288(4), 599 Search PubMed.
- G. H. Cui, D. H. Zhao, P. Lv, Z. G. Gao, S. Y. Chen, N. N. Qiu, T. Satoh, T. Kakuchi and Q. Duan, Synthesis and characterization of Eu(III)-based coordination complexes of modified D-glucosamine and poly(Nisopropylacrylamide), Opt. Mater., 2017, 72, 115 CrossRef CAS.
- Y. Wang and H. L. Jiang, Eu(III) modifies the properties of poly(N-isopropylacrylamide), Mater. Lett., 2007, 61(13), 2779 CrossRef CAS.
- G. R. C. Choppin and Z. M. Wang, Correlation between Ligand Coordination Number and the Shift of the 7F0–5D0 Transition Frequency in Europium(III) Complexes, Inorg. Chem., 1997, 36(2), 249 CrossRef CAS.
- R. Hu, D. H. Xin, A. J. Qin and B. Z. Tang, Polymers with Aggregation-induced Emission Characteristics, Acta. Polym. Sin., 2018, 2(2), 132 Search PubMed.
- J. B. Wang, S. Xia, J. H. Bi, Y. B. Zhang, M. X. Fang, R. L. Luck, Y. B. Zeng, T. H. Chen, H. M. Lee and H. Y. Liu, Near-infrared fluorescent probes based on TBET and FRET rhodamine acceptors with different pKa values for sensitive ratiometric visualization of pH changes in live cells, J. Mater. Chem. B, 2019, 7(2), 198 RSC.
- J. Adelsberger, A. Kulkarni, A. Jain, W. N. Wang, A. Bivigou, P. Busch, V. Pipich, O. Holderer, T. Hellweg, A. Laschewsky, P. Müller-Buschbaum and C. M. Papadakis, Thermoresponsive PS-b-PNIPAM-b-PS Micelles: Aggregation Behavior, Segmental Dynamics, and Thermal Response, Macromolecules, 2010, 43(5), 2490 CrossRef CAS.
- H. Grull and S. Langereis, Hyperthermia-triggered drug delivery from temperature-sensitive liposomes using MRI-guided high intensity focused ultrasound, J. Controlled Release, 2012, 161(2), 317 CrossRef.
- W. Wang, C. Q. Gao, Y. Q. Qu, Z. F. Song and W. Q. Zhang,
In Situ Synthesis of Thermoresponsive Polystyrene-b-poly(N-isopropylacrylamide)-b-polystyrene Nanospheres and Comparative Study of the Looped and Linear Poly(N-isopropylacrylamide)s, Macromolecules, 2016, 49(7), 2772 CrossRef CAS.
- H. H. Liu, K. Miao, G. D. Zhao, C. X. Li and Y. L. Zhao, Synthesis of an amphiphilic PEG-PCL-PSt-PLLA-PAA star quintopolymer and its self-assembly for pH-sensitive drug delivery, Polym. Chem., 2014, 5(8), 3071 RSC.
- Z. Q. Feng, Z. P. Wang, C. Y. Gao and J. C. Shen, Template Polymerization to Fabricate Hydrogen-Bonded Poly(acrylic acid)/Poly(vinylpyrrolidone) Hollow Microcapsules with a pH-Mediated Swelling-Deswelling Property, Chem. Mater., 2007, 19(19), 4648 CrossRef CAS.
- X. L. Liu, Y. J. Xu, Z. Q. Wu and H. Chen, Poly(N-vinylpyrrolidone)-Modified Surfaces for Biomedical Applications, Macromol. Biosci., 2013, 13(2), 147 CrossRef CAS.
- Y. Y. Li, H. Cheng, J. L. Zhu, L. Yuan, Y. Dai, S. X. Cheng, X. Z. Zhang and R. X. Zhuo, Temperature- and pH-Sensitive Multicolored Micellar Complexes, Adv. Mater., 2009, 21(23), 2402 CrossRef CAS.
Footnote |
† Electronic supplementary information (ESI) available: Detailed experimental procedures and additional figures. See DOI: 10.1039/d0qm00497a |
|
This journal is © the Partner Organisations 2021 |
Click here to see how this site uses Cookies. View our privacy policy here.