DOI:
10.1039/D1NA00293G
(Review Article)
Nanoscale Adv., 2021,
3, 5722-5744
Functionalized carbon nanotubes: synthesis, properties and applications in water purification, drug delivery, and material and biomedical sciences
Received
21st April 2021
, Accepted 8th August 2021
First published on 9th August 2021
Abstract
Carbon nanotubes (CNTs) are considered as one of the ideal materials due to their high surface area, high aspect ratio, and impressive material properties, such as mechanical strength, and thermal and electrical conductivity, for the manufacture of next generation composite materials. In spite of the mentioned attractive features, they tend to agglomerate due to their inherent chemical structure which limits their application. Surface modification is required to overcome the agglomeration and increase their dispersability leading to enhanced interactions of the functionalized CNTs with matrix materials/polymer matrices. Recent developments concerning reliable methods for the functionalization of carbon nanotubes offer an additional thrust towards extending their application areas. By chemical functionalization, organic functional groups are generated/attached to the surfaces as well as the tip of CNTs which opens up the possibilities for tailoring the properties of nanotubes and extending their application areas. Different research efforts have been devoted towards both covalent and non-covalent functionalization for different applications. Functionalized CNTs have been used successfully for the development of high quality nanocomposites, finding wide application as chemical and biological sensors, in optoelectronics and catalysis. Non covalently functionalized carbon nanotubes have been used as a substrate for the immobilization of a large variety of biomolecules to impart specific recognition properties for the development of miniaturized biosensors as well as designing of novel bioactive nanomaterials. Functionalized CNTs have also been demonstrated as one of the promising nanomaterials for the decontamination of water due to their high adsorption capacity and specificity for various contaminants. Specifically modified CNTs have been utilized for bone tissue engineering and as a novel and versatile drug delivery vehicle. This review article discusses in short the synthesis, properties and applications of CNTs. This includes the need for functionalization of CNTs, methods and types of functionalization, and properties of functionalized CNTs and their applications especially with respect to material and biomedical sciences, water purification, and drug delivery systems.
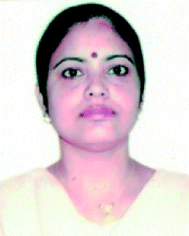 Rama Dubey | Rama Dubey obtained her PhD from Kanpur University in 2000. She was deputed to Penn State University and Arkansas University, USA in the year 2002 and 2007. Currently she is heading a team of scientists at the Defence Research Laboratory, DRDO, Tezpur, India. Her research areas are engineering and conducting polymers, smart technologies in the field of water purification, fuel and lubricants and microwave absorption. |
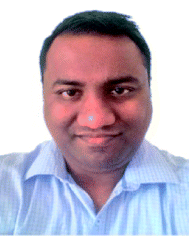 Dhiraj Dutta | Dhiraj Dutta graduated in 2005 from Tezpur Central University. He is a PhD student at NIT Nagaland. He joined DRDO as a scientist in 2008. His primary interests are development of technical materials for specific applications including aerograde materials with extremely low density. |
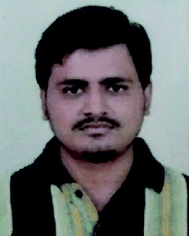 Arpan Sarkar | Arpan Sarkar completed his BSc with Chemistry honors from Calcutta University, Kolkata, West Bengal, India in 2009. Since, 2016 he has been working at the Defence Research Laboratory, Tezpur, Assam, India. He extensively surveyed the soil and water of the Eastern Himalaya region for several anthropogenic and geogenic factors and their impact on the environment. He is now involved in developing several water purification techniques. |
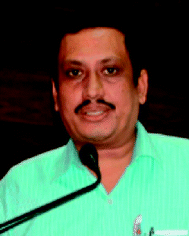 Pronobesh Chattopadhyay | Pronobesh Chattopadhyay, PhD, FNASc, is currently working as Scientist and Head, Pharmaceutical Technology Division, Defence Research Laboratory, Tezpur, Assam. He has 10 patents and more than 135 publications in reputed journals, 10 monographs and 4 book chapters. |
1. Introduction
Since their discovery by Iijima1 in 1991, carbon nanotubes (CNTs) have attracted considerable attention in applied research. There are mainly two types of carbon nanotubes: (i) single walled nanotubes (SWCNTs) and (ii) multiwalled nanotubes (MWCNTs). Both the types have rolled-up graphene sheets forming nanotube cylinders. The wall of nanotubes is made up of carbon hexagons and the tubular curved ends have some carbon pentagons. The salient features of both the types of carbon nanotubes are given in Table 1.
Table 1 Salient features of SWCNTs and MWCNTs
S. no. |
SWCNTs |
MWCNTs |
1 |
Single layer of graphene having a diameter of 0.4 to 3.0 nm |
Multiple layer (2–50) of graphene having a diameter 1.4 to 100 nm (15–55 nm preferred) |
2 |
Catalyst is required for synthesis |
Can be produced without a catalyst |
3 |
Bulk synthesis difficult due to requirement of proper control over growth and atmospheric conditions |
Bulk synthesis is easy |
4 |
Purity is poor |
Purity is high |
5 |
Functionalization sometimes results in defect generation |
Chances of defect generation during functionalization are comparatively less |
6 |
Characterization and evaluation is easy |
It is a very complex structure |
7 |
Easily twistable and pliable |
Can't be easily twisted |
1.1 Synthesis of carbon nanotubes
Several methods have been reported for the synthesis of CNTs, but the most common methods are arc-discharge,2–7 laser ablation8,9 and chemical vapor deposition (CVD).10–16 A comparative description of these three methods is given in Table 2. In addition to these, a few less frequently used approaches for synthesizing nanotubes are the gas phase catalytic process (HiPCO),17 flame synthesis,18 core shell polymer microsphere method,19 aerosol precursor method,20 arc water process,21 low temperature route,22 plasma method,23 fluidized bed method24 and nebulized spray process.25
Table 2 Comparison of main synthesis methods of carbon nanotubes
Method/requirements |
CVD |
Laser ablation |
Arc discharge |
Raw materials & availability |
Fossil based & botanical hydrocarbon, abundantly available |
Graphite, difficult to get |
Pure graphite, difficult to get |
Nature of process & description |
Continuous, substrate placed in an oven, and heated to high temperature followed by slow addition of carbon bearing gas such as methane. Gas decomposes to liberate carbon atoms which combine to form nanotubes |
Batch intense laser pulse used to blast graphite to generate carbon gas to form CNTs, and different conditions were tried to get optimum which produces a sufficient amount of SWCNTs |
Batch two graphite rods placed a few millimeters apart and connected to a power supply. At 100 amps carbon vaporizes and forms hot plasma |
Process condition |
High temperatures within 500 to 1000 °C at atmospheric pressure |
Argon or nitrogen gas at 500 Torr |
Low-pressure inert gas (helium) |
Production cost |
Low |
High |
High |
Post treatment |
Not required |
Required |
Required |
Yield |
High 20–100% |
Moderate 70% max |
Good 30–90% max |
SWCNT |
Long tubes with diameters ranging from 0.6 to 4 nm |
Long bundles of tubes with length 5–20 microns, and individual diameter from 1–2 nm |
Short tubes having diameters in range 0.6–1.4 nm |
MWCNT |
Long tubes with the diameter in the range of 10 to 240 nm |
MWNT synthesis is possible but it is too expensive |
Short tubes with inner diameter 1–3 nm and the outer diameter approximately 10 nm |
Purity |
High |
High |
High |
Production rate |
High |
Low |
Low |
Energy requirement |
Moderate |
High |
High |
Reactor design |
Easy |
Difficult |
Difficult |
Advantages |
Easy to scale up, simple process, long length SWCNTs with a controllable diameter and good purity |
Good quality SWNTs with high yield and narrow distribution compared to arc discharge |
Less expensive with open air synthesis. Can easily produce SWNT & MWNTs. SWNT with few structural defects & MWNTs even without a catalyst |
Disadvantages |
Synthesized CNTs are usually with defects |
Costly technique since it requires expensive lasers and high-power, limited to labscale |
Extensive purification required since nanotubes are short, tangled with random sizes and directions |
References |
10–16
|
8 and 9 |
2–7
|
1.2 Impressive properties of carbon nanotubes
CNTs have many magnificent properties that have motivated researchers of many disciplines to conduct intensive studies. Impressive properties in various aspects i.e. mechanical, electrical, thermal, chemical and biological are presented briefly in this section. Besides them, the small size of nanotubes which leads to many unique advantages will also be discussed.
1.2.1 Mechanical properties.
CNTs possess high stiffness and axial strength as a result of carbon–carbon sp2 bonding.26 They are the stiffest known fiber, with a measured Young's modulus of 1.4 TPa.27 Their elongation to failure is 20–30%, which combined with the stiffness projects a tensile strength above 100 GPa, the highest known so far. For example the Young's modulus of high strength steel is around 200 GPa, and its tensile strength is 1–2 GPa.28
1.2.2 Electrical properties.
The unique electrical properties of CNTs are derived from their 1D characteristics and the peculiar electronic structure of graphite. They possess extremely low electrical resistance. In addition, they show the highest current density of any known material measured, as high as 109 A cm−2.29,30 They have shown to be superconducting even at low temperatures. These unique electrical properties are utilized for fabricating field emission displays (FEDs) as well as constructing electronic computer circuits entirely out of carbon nanotubes.
1.2.3 Thermal, chemical and biological properties.
Before CNTs, diamond was known to be the best thermal conductor. CNTs have now shown to have a thermal conductivity at least twice that of diamond.31 They are chemically inert, especially when no defects are present. This makes them chemically stable and biologically compatible. Besides being chemically stable, they have a large surface to volume ratio and hence are becoming an interesting material for hydrogen storage. In terms of biological applications, SWCNTs have been used as drug delivery vehicles to bring chemicals into cells.32
1.2.4 Small size.
The biggest advantage of CNTs is their small diameter due to which they have been attached onto normal AFM tips in order to improve their resolving capability. The small size of SWCNTs makes it easier to study chemical bindings between only one pair of receptor/target. Another application utilizing the small size of SWCNTs is as electron emitters. This kind of emitter can be used to extract electrons to generate light, either visible (TV) or X-ray (portable and high resolution X-ray machine).33
1.3 Applications of CNTs
Among the plethora of applications for CNTs, polymer composites, field effect transistors, field emission displays and hydrogen storage appear to be the most promising areas. For example, CNTs have been considered to be novel components for molecular electronics and for integration into conventional circuits.34 CNT polymer composites are expected to be tougher and more scratch resistant than other materials.35 Recently CNTs have been used as field emission electron sources.36 This technology finds applications in the construction of flat panel displays and X-rays to microwave generators.37 Finally, storage of hydrogen on CNTs represents a great development in the field of fuel cells and a milestone towards energy clean systems.38,39
1.4 Disadvantages of CNTs
In spite of excellent properties and widespread applications, the lack of solubility in aqueous and organic media has been a major technological barrier for CNTs. This is due to their stable structure having pure carbon element. In addition, they have very high long range van der Waals forces of attraction due to which they have a tendency to aggregate together and it becomes very difficult to disperse them.40,41 Thus the poor processability due to their insoluble and intractable nature has hindered their use in many applications. To overcome this problem of poor processability and in order to take full advantage of the properties of CNTs, functionalization and solubilization of CNTs have received much recent attention. This helps to extend their application areas immensely.
1.5 Functionalization of CNTs and offered advantages
Functionalization is the generation of functional groups on the surfaces of CNTs. These functional groups help in decreasing the long range van der Waals forces of attraction and increase the CNTs-matrix/solvent interaction and give rise to a homogeneous dispersion or result in solubilization of CNTs.42,43 The effect of functionalization of CNTs on properties is given in Table 3. Thus functionalization enhances the reactivity, improves the solubility and provides an avenue for further chemical modification of CNTs such as ion adsorption, metal deposition, grafting reactions etc. In addition the functional groups can also serve as anchor groups for joining two moieties and further derivatization by chemical reactions with other functional groups. Taking the advantages offered by functionalization of CNTs into account, a lot of modeling studies have been carried out to predict the properties of functionalized CNTs as well as their impact on other biomolecules.44–46
Table 3 Effect of CNTs functionalization on properties
Functionalization method |
Property enhancement |
References |
Fluorination |
Enhanced solubility in organic solvents |
56 and 57 |
1,3-Dipolar cycloaddition |
Enhanced solubility in aqueous & organic solvents |
62 and 63 |
Aryl diazonium salt reduction |
Enhanced solubility in organic solvents |
64 and 65 |
Reactive species functionalization |
Further derivative functionalization |
66–68
|
Electrophilic addition |
Further derivative functionalization |
69
|
Metal-containing molecular complex formation |
Enhanced solubility & stability in organic solvents |
70 and 71 |
Carboxylation |
Further derivatization, use as molecular linker to interconnect nanotubes |
74–76
|
Nanoparticles deposition |
Soluble in water & able to trap water soluble metal ions |
88–99
|
Surfactant functionalization |
Enhanced dispersion |
102–113
|
Polymer wrapping |
Reversibly solubilized in water stable dispersion in water and organic solvents |
115–121
|
Polymer absorption |
Enhanced anchoring ability for proteins and small biomolecules, enhanced dispersion in organic solvents |
122–124
|
Polymer encapsulation |
Enhance dispersion of CNTs in a wide variety of polar and non polar solvents and polymer matrices |
125–129
|
Metal deposition |
Metal nanotube supported metal nanowire structures |
130–134
|
In this review, we have focused on different functionalization approaches for the synthesis of functionalized CNTs (f-CNTs), which are the primary prerequisite for application of CNTs in any area. Both covalent and non-covalent approaches for functionalization have been discussed in detail. The advantages and applications of f-CNTs have also been discussed with special emphasis on their application in the area of material and biomedical sciences, water purification, and drug delivery systems. Although different reviews on functionalization of CNTs and their application in the field of biomedical science, drug delivery, water treatment etc. have been published in the literature,47–55 there is a need for a comprehensive account encompassing different application areas together which has been incorporated in this review. The goal is to offer a brief and valuable description to the reader about the key functionalization strategies developed in this field along with their potential applications in the areas of human concern. At the end, future recommendations for this exciting research area have also been provided.
2. Methods of functionalization
There are many well reported methods of functionalization as shown in Scheme 1. Broadly they can be classified as either exohedral or endohedral, depending on whether the modification is being done on the outside or inside walls of the CNTs. The exohedral functionalization is further subdivided into two categories: (a) covalent functionalization and (b) non-covalent functionalization. Table 4 shows the advantages and disadvantages of various functionalization methods which are discussed below.
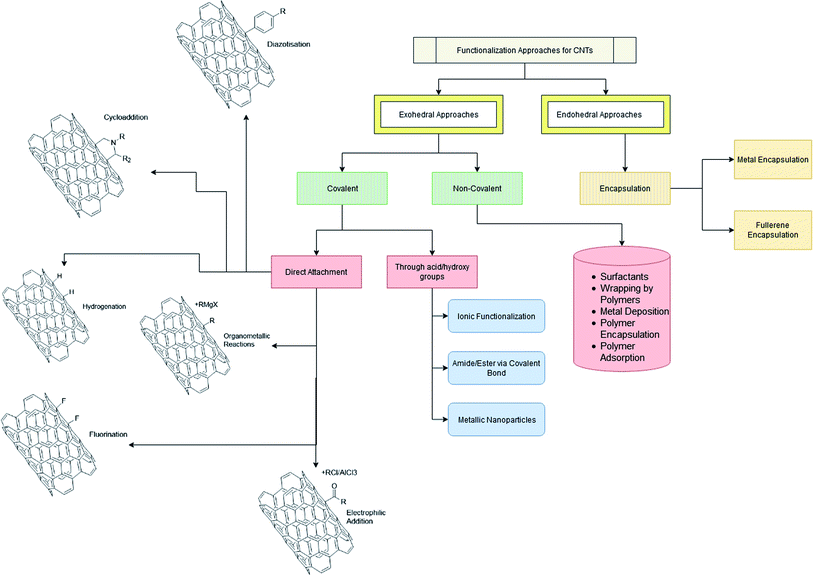 |
| Scheme 1 Functionalization approaches for CNTs. | |
Table 4 Advantages and disadvantages of various methods of CNT functionalization
Methods for CNT functionalization |
Advantages |
Disadvantages |
References |
Exohedral |
• Does not disrupt the main structure of CNTs |
• Properties are specific to the nature of functional groups attached |
|
• Properties of both CNTs and functional group achieved |
Covalent |
• Covalent bonds act as substitution sites for functionalization |
• Change of hybridization from sp2 to sp3 and loss of the p-conjugation system on the graphene layer |
56–99
|
• Secondary substitution reactions possible |
• This results in quenching of the tube emission |
• Functionalization leads to enhanced solubility in water and organic solvents |
• Sometimes CNTs get oxidatively consumed in the process |
• Hydrophilic groups introduced onto the CNT surface render them more biocompatible and biodegradable |
|
Non covalent |
• Possibility of adsorbing various groups on CNT surface without disturbing the π system of the graphene sheets |
• Stability of dispersions also depends on the nature and concentration of surfactants |
100–134
|
• Hydrophobic part of the adsorbed molecules attaches with nanotube sidewalls through van der Waals, π–π, CH–π and other interactions, whereas aqueous solubility is provided by the hydrophilic part of the molecules |
• Polymer wrapping over CNT surface is solvent dependent |
• Aggregation prevented by coulombic repulsion forces between modified CNTs |
|
• Polymer encapsulated CNTs could be well dispersed in polymer matrices and solvents |
|
• NTs serve as an electrodeposition template and subsequently as a wire to electrically connect the deposited Au, Pt and Pd nanoparticles resulting in metal nanotube supported metal nanowire structures |
• Non covalent strategy to attach carboxylic functional groups through p–p stacking interactions, thereby creating stable aqueous dispersions and limiting cytotoxicity |
Endohedral |
• Buckytubes constitute smart carrier systems which may be filled with tailored materials to address specific demands |
• This strategy requires CNTs to be opened, usually under aggressive conditions, which may lead to damaging of the outer wall or surface functionalization |
135–157
|
• CNTs can be filled with anticancer antimalarial drugs |
• Sensitive biological compounds with a low melting point or high decomposition rate can be easily dissolved and introduced into the CNTs, which would otherwise be impossible through a physical route |
• Filling yield tends to be rather low (scarcely above 20%) and dramatically reduced with a decrease in CNT diameter, which makes the task rather difficult in the case of SWNTs and DWNTs |
• MWNTs allow for prolonged release of the encapsulated drug, thereby increasing its anticancer efficacy |
2.1 Exohedral functionalization
2.1.1 Covalent functionalization.
Covalent functionalization can roughly be divided into two categories, i.e. direct attachment of functional groups to the graphitic surface such as fluorination, hydrogenation, 1,3 dipolar addition etc. or the use of nanotube bound carboxylic or hydroxyl functional groups for further derivatization (amidation or esterification) and chemical reactions as discussed below.
2.1.1.1 Covalent functionalization by direct attachment of functional groups.
(i) Fluorination.
Fluorination of CNTs was developed by Margrave and coworkers.56 Fluorination is generally carried out by treatment with molecular fluorine at temperatures between 150 and 600 °C. Depending on the reaction temperature, different degrees of fluorination can be accomplished. This method gives heavily functionalized CNTs with increased solubility in organic solvents such as alcohols.57 The covalent bond formation with fluorine has been confirmed by IR spectroscopy, through the C–F valence vibration at 1220–1250 cm−1. Fluorination drastically changes the electronic properties of CNTs making them insulators when fluorination was carried out above 250 °C. However, treatment of such derivatized nanotubes with hydrazine results in defunctionalization, such that intact CNTs can be recovered. In addition, F atoms could be displaced by various nucleophiles such as organolithium and Grignard reagents to give nanotubes functionalized with organic moieties,58 leading to much better dissolution properties.59
(ii) Hydrogenation.
Pekker and coworkers reported the hydrogenation of CNTs via birch reduction in ammonia.60 Later Han et al. used a gas phase technique for CNT functionalization with atomic hydrogen.61 This technique proved to be useful due to its clean and low temperature process in comparison to wet chemical methods which result in the change of physical and chemical properties of CNTs. This approach used a glow discharge to generate the necessary precursors and is suitable for attaching a wide variety of chemical groups to CNTs. The functionalization was carried out with atomic hydrogen. FTIR spectroscopy showed the presence of bands at 1370 and 1457 cm−1 that are due to the bending mode of C–H vibrations in SWCNTs based on functionalization with a H atom.
(iii) 1,3-Dipolar cycloadditions.
Prato and coworkers reported 1,3-dipolar cycloaddition62,63 of azomethine ylides (generated by condensation of substituted α-amino acids and an aldehyde as shown in Fig. 1) for functionalization of both single walled (SW) as well as MWCNTs. This type of functionalization leads to a very high level of solubility of the resulting products in water as well as organic solvents such as chloroform, acetone, methanol etc.
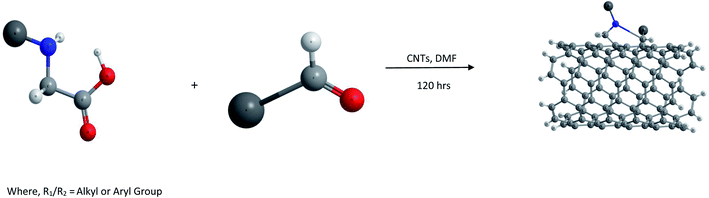 |
| Fig. 1 Cycloaddition reactions of CNTs, where R1/R2 = alkyl or aryl group. | |
The main advantage of this reaction is the easy attachment of pyrrolidine rings substituted with chemical functionalities to the side walls of the CNTs which leads to the construction of novel materials with diverse applications. Thus functionalized aldehydes can lead to 2-substituted pyrrolidine moieties located on the side walls of CNTs, while modified α-amino acids could also lead to numerous diverse functionalized materials.
(iv) Functionalization by reduction of aryl diazonium salts.
Aryl diazonium salts are known to react with olefins resulting in arylation of aromatic compounds. Hence SWCNTs have also been functionalized with aryl moieties by the reduction of a variety of substituted aryl diazonium salts either electrochemically64 or under normal room temperature conditions65 as shown in Fig. 2. It was found that CNTs derivatized with a 4-tert-butylbenzene moiety possess much improved solubility in organic solvents. However the functionalizing moieties can be removed by heating in an argon atmosphere.
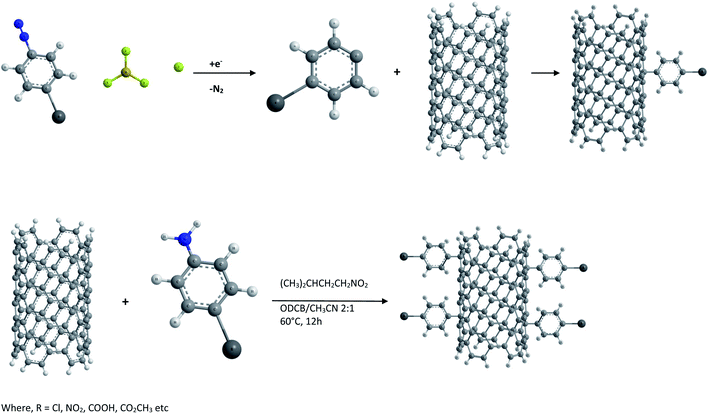 |
| Fig. 2 Arylation of CNTs via reduction of diazonium salts, where R = Cl, NO2, COOH, CO2CH3etc. | |
As described by J. M. Tour et al.64,65 both direct treatment of SWCNTs with aryl diazonium tetrafluoroborate salts in solution and in situ generation of diazonium with an alkyl nitrite are effective means of functionalization. In some cases direct treatment with preformed diazonium salts is observed to be effective at moderate or even room temperature. However the in situ generation of diazonium species is advantageous since it avoids the necessity of isolating and storing unstable and light sensitive aryl diazonium salts.
(v) Reactive species functionalization.
This type of functionalization was reported by M. Holzinger et al.66,67 and is achieved by directly reacting CNTs with highly reactive groups such as nitrenes, carbenes, radicals etc.68 as shown in Fig. 3 and 4. For example the thermal reaction of alkyl azidoformiates leads to N2 extrusion and alkoxycarbonylaziridino derivatized CNTs, which are soluble in organic solvents such as DMSO.
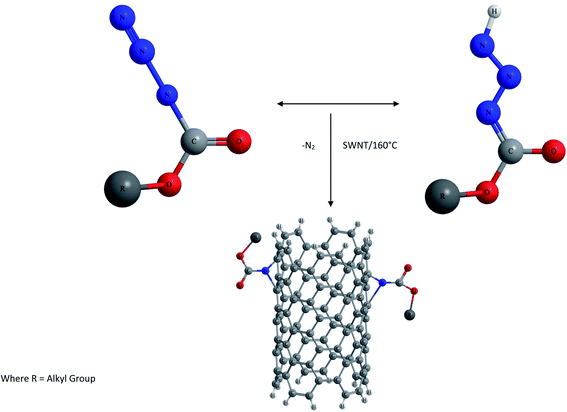 |
| Fig. 3 Functionalization of CNTs with nitrenes, where R = alkyl group. | |
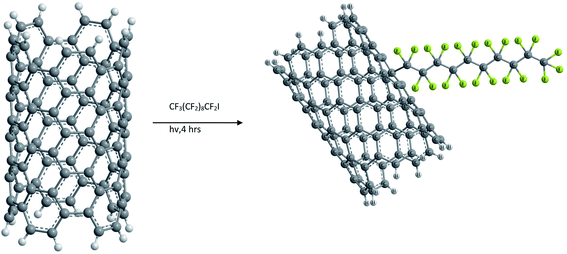 |
| Fig. 4 Functionalization of CNTs with radicals. | |
Stable solutions of functionalized SWCNTs are also formed from the addition of nucleophilic carbenes. Deprotonation of the dipyridyl imidazolium system leads to the generation of dipyridyl imidazolidene resulting in carbine addition. They react with the electrophilic π system of CNTS to give zwitterionic 1
:
1 adducts rather than cyclopropane systems because of the special stability of the resultant aromatic 14 π perimeter. Because of its high nucleophilicity, side wall functionalization of SWCNTs takes place by a nucleophilic polyaddition reaction. Thus, in this case no cyclopropanation occurs; rather each added group is bound through just one covalent bond to the tube and is positively charged due to the transfer of one negative charge per addend to the nanotube framework. The high solubility of carbine adducts is due to mutual electrostatic repulsion of the tubes.
Functionalization of SWCNTs with radicals was also carried out by photoinduced addition of perfluorinated alkyl radicals as shown in Fig. 4. This was conducted by irradiating a solution of heptadecafluorooctyl iodide with a mercury lamp. However, the fluoroalkyl substituted NTs did not show any improvement in solubility compared to raw SWCNTs.
(vi) Electrophilic addition.
An electrophilic addition to SWCNTs (prepared by a gas phase catalytic method) was reported by N. Tagmatarchis et al.69 Upon a mechanochemical reaction in the presence of a Lewis acid, such as aluminum trichloride (AlCl3), a molecule of chloroform was found to be added to the side walls of CNTs. It was also possible to exchange the chlorine atoms with hydroxyl groups, which in a second step were esterified to yield corresponding esters as shown in Fig. 5.
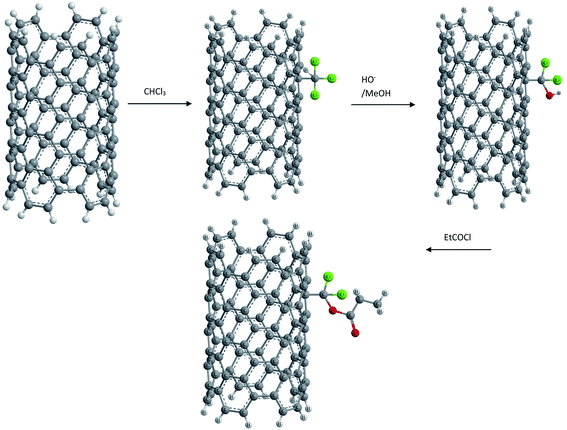 |
| Fig. 5 Electrophilic addition reaction of CNTs, where R = alkyl/aryl group. | |
This functionalization of SWCNTs not only resulted in a soluble functionalized material but also showed that specifically derivatized CNTs bearing free functional groups on their skeleton can serve as suitable synthons for the multistep synthesis of CNT based materials.
(vii) Functionalization of CNTs with a metal-containing molecular complex.
Both raw and oxidized CNTs have been functionalized with Vaskas compound trans-IrCl(CO)(PPh3)2 to form a covalent CNT-metal complex by coordination through the increased number of oxygen atoms, and formed a hexacoordinate structure around the Ir atom70 respectively. The complexes of oxidized nanotubes with Vaska's compound were soluble and stable in organic solutions compared to raw and oxidized tubes. Moreover the tubes could be easily recovered from solution. The novelty of this derivatization is that the nanotube can be considered as a primary ligand, as a functional moiety like any other, with respect to the central metal atom, Ir. In effect, Ir was found to coordinate with the nanotubes in several distinctive bonding arrangements, depending on the oxidation state of the nanotubes. Thus the electronic structure of nanotubes is preserved due to functionalization through coordinate attachment. A similar type of functionalization has also been observed with Wilkinson's complex, RhCl(PPh3)3.71 Hence, this functionalization opens up the area of metallo-organic chemistry suggesting potential application in catalysis and molecular electronics.
2.1.1.2 Covalent functionalization by derivatization of functional groups.
(I) Carboxylation and further derivatization.
The purification of CNTs involves the removal of metal particles or amorphous carbon from the crude material by oxidative methods in strong acidic media.72,73 Under such harsh conditions, the end caps of the nanotubes get opened and become acidic (–COOH) and other (–OH) functionalities suitable for further derivatization are formed at the tips as well as on the side walls.74–76 The tip functionalized CNTs can form a closed ring shape, or even function as single molecular sensors or probes, whereas surface functionalized CNTs can be used as a molecular linker to interconnect nanotubes77 as shown in Fig. 6.
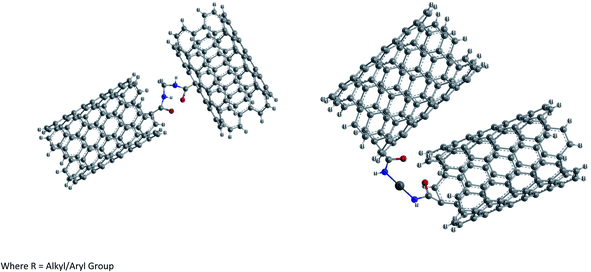 |
| Fig. 6 Linking of CNTs via surface and tip functionalized groups. | |
For carrying out the functionalization of CNTs by oxidation, potassium permanganate has been used as the oxidant in the presence of sulfuric acid. It has also been used along with a phase transfer catalyst.78 Other oxidants used to functionalize CNTs are concentrated nitric acid, concentrated sulfuric acid, a mixture of concentrated sulfuric and nitric acid, superacid HF/BF3, aqua regia, aqueous MO4 (M = Os, Ru), OsO4–NaIO4, H2O2, K2Cr2O7etc.79,80 However some of these reagents open the tips of the tubes, causing damage to the tubular structure, or even introducing contaminants.
(1) Amidation and esterification of –COOH groups via covalent linkages.
Generally activation of carboxyl moieties with thionyl chloride and subsequent reaction with amines are preferred as shown in Fig. 7, or alternatively, direct condensation of oxidized NTs with amines is also carried out.81
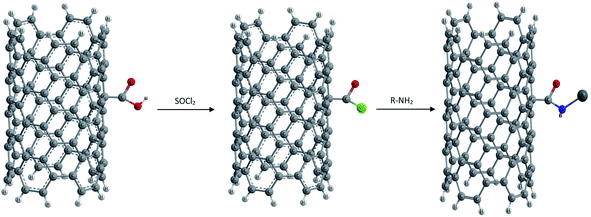 |
| Fig. 7 Amidation of COOH functionalized CNTs. | |
Niyogi and co-workers first reported the use of acid groups for attaching long alkyl chains to SWCNTs via amide linkages. Thus long chain alkylamines were condensed with carboxylic groups present on the surface of CNTs.82 The amido functional NTs were found to be soluble in solvents such as tetrahydrofuran or dichlorobenzene. Sun and coworkers showed that the esterification of carboxylic acids results in functionalization as well as solubilization of nanotubes of any length.83,84 Thus the amidation and esterification reactions of oxidized NTs have been reported which finally result in soluble functionalized CNTs.85,86 An advantage with ester linkages is that they can be facilely defunctionalized via acid or base catalyzed hydrolysis, allowing the recovery of carbon nanotubes from the soluble samples.
(2) Ionic functionalization of –COOH groups.
Non-covalent functionalization of –COOH groups present on the surface of pure SWCNTs has been carried out by reacting with octadecylamine.87 The acid base reaction leads to the formation of SWCNT-carboxylate zwitterions as shown in Fig. 8. These zwitterions are soluble in THF and 1,2-dichlorobenzene and partially in dichloromethane, chlorobenzene etc. This ionic functionalization approach shows advantages such as a high yield (∼70%) of soluble NTs compared to that obtained in covalent functionalization (∼30%).
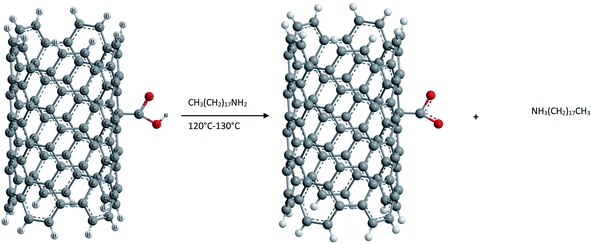 |
| Fig. 8 Ionic functionalization of COOH functionalized CNTs. | |
Further the presence of zwitterions improves the solubility of purified CNTs significantly and the acid base reaction represents the simplest possible route to soluble SWCNTs and can be readily scaled up at low cost. Unlike the covalent amide bond, the cation (+NH3(CH2)17CH3) in the ionic bond of the (SWCNT-COO−)+NH3-(CH2)17CH3 of soluble SWCNTs can be readily exchanged for other organic and inorganic cations. Thus it is possible to adjust the solubility properties of SWCNTs and to use soluble SWCNT (s-SWCNTs) as versatile building blocks for advanced SWCNT-based materials via supramolecular chemistry. Furthermore, such an ionic feature allows electrostatic interactions between SWCNTs and biological molecules and can serve as the basis for developing biocompatible SWCNTs.
(II) Attachment of metallic nanoparticles.
Carboxylic and hydroxyl functionalized carbon nanotubes have also been used for nanoparticle deposition by reducing metal precursors in ethylene glycol solutions.88–90 Several researchers have reported deposition of Pt, Ru and alloy PtRu nanoparticles by this method.91,92 Nanoparticles of 1–10 nm with metal loading less than 10 wt% have been obtained. Deposition of Pt nanoparticles has also been reported on sulfonic acid functionalized CNTs in which the sulfonic acid sites act as molecular sites for Pt ion adsorption, which get subsequently reduced to yield small Pt nanoparticles.93 Au has been deposited on CNTs by using a large variety of functional groups. For example Au nanoparticles were successfully self-assembled on the surface of MWCNT functionalized with mercaptobenzene moieties.94 On the other hand, Muhammad et al. reported deposition of Au nanoparticles on ethylenediamine functionalized SWCNTs.95 It was found that the Au NPs acted as an electron acceptor to the amine functionalized SWCNTs. Jiang et al. also attached Au NPs to nitrogen doped MWCNTs with a H2SO4–HNO3 chemical treatment.96 Ellis et al. demonstrated novel anchoring of gold nanoclusters onto CNTs via hydrophobic interactions between octanethiol capped nanoclusters and acetone activated CNT surfaces.97 According to Hwang et al. copper has also been uniformly deposited on functionalized MWCNTs by chemical methods.98 Recently, a novel type of Pd coated MWCNT has been reported by Adeli et al.99 They polymerized citric acid on the surface of functionalized (–COOH) MWCNTs resulting in MWCNT–graft-poly(citric acid) (MWCNT–g-PCA) hybrid materials. These materials were not only soluble in water but were also able to trap water soluble metal ions. Reduction of trapped metal ions in the polymeric shell of MWCNT–g-PCA hybrid materials by reducing agents such as sodium borohydride led encapsulated metal nanoparticles on the surface of the MWCNTs.
2.1.2 Noncovalent functionalization.
Non covalent treatment is particularly attractive because of the possibility of adsorption of various groups on the CNT surface without disturbing the π system of the graphene sheets.100 Non-covalent approaches are based on interactions of the hydrophobic part of the adsorbed molecules with nanotube sidewalls through van der Waals, π–π, CH–π and other interactions, and aqueous solubility is provided by the hydrophilic part of the molecules.101 The charging of the nanotube surface due to adsorbed ionic molecules additionally prevents nanotube aggregation by the coulombic repulsion forces between modified CNTs. In the last few years, the noncovalent treatment of CNTs with surfactants and polymers has been widely used in the preparation of both aqueous and organic solutions to obtain a high weight fraction of individually dispersed nanotubes.
2.1.2.1 Surfactant functionalization.
Surfactants generally act as “solubilizers” and disperse CNTs via physical adsorption. Generally the hydrophobic part of surfactants is oriented towards the surface of CNTs, whereas the polar moiety interacts with solvent molecules in the outer region as shown in Fig. 9. Both ionic and non-ionic surfactants such as sodium dodecyl sulphate (SDS), sodium dodecyl benzene sulfonate (SDBS), cetyltrimethyl ammonium bromide (CTAB), Brij, Tween, Triton X, and a siloxane polyether copolymer (PSPEO) have been used for dispersion of CNTs in an aqueous medium.102–106 It is believed that the nanotubes are in the hydrophobic interiors of the corresponding micelles, which results in stable dispersion. However, the nature and concentration of the surfactant plays a very important role in obtaining stable dispersions.
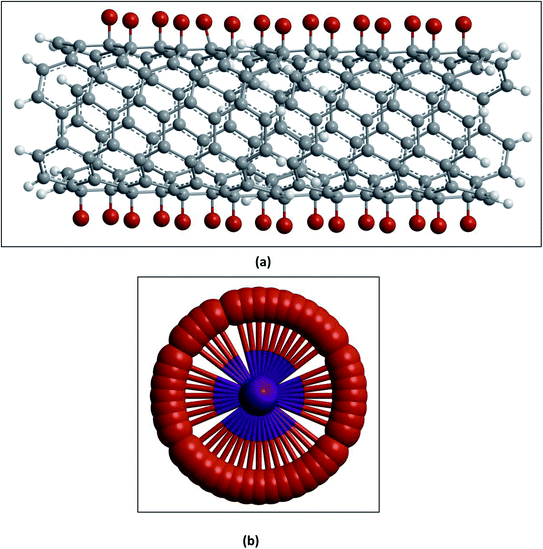 |
| Fig. 9 Surfactant functionalization of CNTs (a) and a surfactant micelle (b). | |
Dispersion/solubilization is generally achieved by placing CNTs in a solution of surfactant and sonication in an ultrasonic bath. During sonication, the provided mechanical energy overcomes the van der Waals interactions in the CNT bundles and leads to CNT exfoliation, and at the same time the surfactant molecules are adsorbed onto the surface of the CNT walls.107 The colloidal stability of the dispersion of the CNTs with adsorbed molecules on their surface is guaranteed by electrostatic and/or steric repulsion.108–111 Y. Shi et al.112 reported the preparation of individually dispersed SWCNTs by sonication in a micelle of SDS. Gao et al.113 obtained a homogeneous dispersion of MWCNTs at the optimum composition of 0.5 wt% MWCNTs and 2 wt% SDS relative to water. The interaction between CNTs and SDS through the hydrophobic segment causes a steric repulsion and higher negative surface charge, which improves the dispersion of CNTs. Thus the charge of ionic surfactants leads to electrostatic repulsion between the surfactant molecules, and this stabilizes the nanotube colloids, whereas the non-ionic surfactants stabilize the nanotubes in solution primarily by steric interactions. Compared to traditional alkyl chain surfactants, silicone polymer surfactants possess better properties such as super wettability and extremely low surface tension.114 PSPEO, a new amphiphilic macromolecule surfactant, consists of hydrophobic –Si–O–Si– main chains and hydrophilic pendant parts of polyethylene oxide, with a comb-polymer molecular structure. MWCNTs can be easily solubilized in water by non-covalent functionalization with PSPEO.102
2.1.2.2 Polymer wrapping.
Amphiphilic polymers are also used to solubilize CNTs. The main advantage of using polymers instead of small molecular surfactants is that polymers reduce the entropic penalty of micelle formation. It has been reported that some conjugated polymers have significantly higher energy of interaction with nanotubes than small molecules. O'Connell et al.115 reported that SWCNTs can be reversibly solubilized in water in the g L−1 concentration range by noncovalent association with a variety of linear polymers such as polyvinyl pyrrolidone (PVP) and polystyrene sulfonate (PSS).
PVP, a polymer with a hydrophobic alkyl backbone and hydrophilic pendant groups, gets coiled around the nanotube in a manner depicted in Fig. 10 such that its backbone is in contact with the nanotube surface and pyrrolidone groups are exposed to water.116 But the CNTs were found to unwrap on changing the solvent system. S. H. Tan et al.117 reported successful functionalization of MWCNTs with water soluble poly(2-ethyl-2-oxazoline) (PEOX) through the polymer wrapping process. The functionalized MWCNTs gave excellent and stable dispersion in water as well as other organic solvents such as ethanol and N,N-dimethylformamide (DMF). Compared to PVP, polynucleotides are arranged in an opposite manner on CNTs due to their hydrophilic sugar phosphate backbone and relatively hydrophobic aromatic nucleotide bases present as pendants. They form various helical wrappings around the nanotube so that aromatic nucleotide bases are in close contact with the SWCNTs.118 Wrapping of polymers based on conjugated poly(m-phenylenevinylene) (PmPV) systems is also very important as it allows the formation of very effective van der Waals interactions between the nanotubes and the conjugated backbone.119,120 Tang B. Z. et al.121 synthesized poly(phenyl acetylene) wrapped carbon nanotubes (PPAc-CNTs) which were soluble in organic solvents such as tetrahydrofuran, toluene, chloroform and 1,4-dioxane by in situ polymerization. Biological molecules such as peptides and proteins have also been reported to interact with the sidewalls of SWCNTs and make them more soluble in solvents.
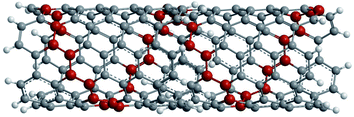 |
| Fig. 10 Functionalization of CNTs via polymer wrapping. | |
2.1.2.3 Polymer absorption.
Pyrenes, a group with a large aromatic system, have demonstrated very high affinity for the nanotube surface and are hence considered an efficient moiety for noncovalent functionalization,122 as shown in Fig. 11. In fact the interaction of the pyrene aromatic system with nanotubes is so effective that functionalized pyrenes have been used for anchoring proteins and small biomolecules. For example R. J. Chen et al. developed a method for the immobilization of proteins and small biomolecules into succinimidyl pyrenebutyrate modified SWCNTs.123 In addition, pyrenes bearing hydrophilic groups such as ammonium ions, carboxylates and block copolymer components give rise to tubes with aqueous solubility. Anthracenes are another class of polyaromatic molecules forming specific π–π interactions with nanotubes. However, anthracenes can be replaced by pyrenes which show stronger interactions with nanotubes. Heterocyclic polyaromatic molecules such as porphyrins and phthalocyanines can also form effective interactions with nanotubes. Nakashima et al. found that porphyrin has the ability to disperse SWCNTs for long periods in organic solvents.124
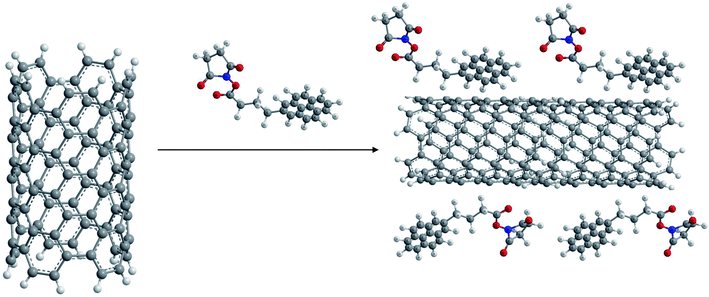 |
| Fig. 11 Functionalization of CNTs by substituted pyrenes. | |
2.1.2.4 Polymer encapsulation.
Encapsulating CNTs with polymers is used as an advantageous alternative approach to realize surface modification. Being a non covalent process, it offers the advantage of maintaining the inherent electronic properties of CNTs. For example, polymer encapsulated MWCNTs have been successfully prepared by Wang et al. through ultrasonically initiated in situ emulsion polymerizations of n-butyl acrylate (BA) and methyl methacrylate (MMA) in the presence of MWCNTs.125 The polymer encapsulated CNTs showed strong interactions between the polymer and MWCNT and they could be well dispersed in polymer matrices such as Nylon 6. In addition to encapsulation of MWCNTs, SWCNTs were also encased within crosslinked/hyperbranched amphiphilic copolymer micelles.126,127 The results showed that the dispersion performance of the obtained micelle encapsulated CNTs in water was greatly improved compared to that of pure CNTs. Stupp et al. reported encapsulation of both single and multiwall carbon nanotubes by self assembling peptide amphiphiles.128 CNTs have also been encapsulated inside polyamide microcapsules for the development of remotely triggerable microcapsules.129 Such encapsulations enhance the dispersion of CNTs significantly in a wide variety of polar and non-polar solvents and polymer matrices.
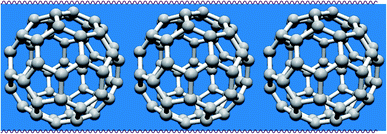 |
| Fig. 12 Fullerene encapsulation inside CNTs. | |
2.1.2.5 Metal deposition.
In addition to deposition of various metals on functionalized CNTs, different types of metals have successfully been deposited on unfunctionalized CNTs also. Different strategies involved are electrochemical deposition, electroless deposition with and without the aid of reducing agents and physical/chemical deposition on CNTs without surface activation. Among these, electroless deposition is of particular interest because of its simplicity and feasibility for large scale production of nanotube–nanoparticle hybrids. The electroless deposition of a large variety of metal nanoparticles including Cu and Ag has been reported on both SWCNTs and MWCNTs in the absence of any reducing agents.130 Dai and coworkers reported the spontaneous nucleation of bare Pt and Au particles on SWCNT side walls.131 Sometimes NTs were found to be oxidatively consumed in the process. Lemay et al. demonstrated electrodeposition of Au, Pt and Pd nanoparticles on the sidewalls of SWCNTs under direct potential control where the nanotube acts as a nonsacrificial template for the deposited clusters.132 Here the nanotube shows a dual function: initially as an electrodeposition template and subsequently as a wire to electrically connect the deposited Au, Pt and Pd nanoparticles. Spontaneous deposition of Pt and Au NPs on the sidewalls of single and multiwall CNTs was also reported by Wu et al.133 The studies showed that metal ions were first adsorbed on the sidewalls of CNTs and then were reduced by ethanol. Zhang et al. reported continuous and quasi-continuous coating of various metals such as Ti, Ni and Pd on suspended SWCNTs by electron beam evaporation134 resulting in metal nanotube supported metal nanowire structures.
2.2 Endohedral functionalization
Endohedral functionalization is of particular interest since the interior of carbon nanotubes is considered as a nanometer sized test tube which provides a highly unexplored, ultrapure nanospace where new reactions may be observed which do not take place outside the tubes.135 The interior surface of CNTs also exhibits a stronger binding energy for adsorbate molecules compared to the planar carbon surface, as expected for a molecule in close proximity to the curved internal surface of the tube.136,137 In addition, the inner cavity of CNTs offers space for the storage as well as protection of guest molecules.
2.2.1 Metal encapsulation.
At present, CNT encapsulated metallic materials have aroused great interest around the world due to the fact that the protective carbon layers prevent rapid oxidation of bare metal nanoparticles. Generally, encapsulation can be carried out by two methods either during CNT synthesis itself that is an in situ process or by wet chemical methods of opening and filling the nanotubes. Both processes have been widely studied. Various techniques have been developed for synthesizing in situ metal filled CNTs, such as the standard carbon arc technique, modified arc deposition or catalytic pyrolysis. Nanoparticles of nearly 15 metals (including Fe, Co, Ni, Ti, Cu and a few rare earth metals and metal carbides) have been successfully encapsulated in CNTs by including the metals in the carbon arc commonly used to synthesize the nanotubes.138–140 Several groups have studied the filling of CNTs by wet chemical methods.74,141 Small quantities of low melting metals such as lead, zinc, selenium and molten silver nitrate have also been incorporated into nanotubes through capillary action after the closed ends of nanotubes were partially opened chemically.142 To date, the uptake and the endohedral chemistry of metals and metal salts have been intensively investigated. For example, gold and platinum nanothreads have been created in the capillaries of the tubes, by treating the SWCNTs with the corresponding perchlorometallic acids at high temperatures. In some cases, the resulting nanothreads have been found to be single crystals.
2.2.2 Fullerene encapsulation.
The incorporation of fullerenes such as C60 and C70 (ref. 143–150) or metallofullerenes such as Sm@C82 (ref. 151–153) is an especially impressive example of the endohedral chemistry of SWCNTs (Fig. 12). This incorporation is executed at defect sites localized at the ends or in the sidewalls. The encapsulated fullerenes tend to form chains that are coupled by van der Waals forces. Such arrays are sometimes called ™bucky peapods, which are considered a very interesting and curious material among novel types of nanotube composites.
Peapods were first revealed by Luzzi et al.154 and then were synthesized in high yield by Burteaux et al.155 Upon annealing, the encapsulated fullerenes coalesce in the interior of the SWCNTs, which results in new, concentric, endohedral tubes, with a diameter of 0.7 nm.
2.2.3 Miscellaneous.
In addition to metal and fullerenes, other types of materials such as halogen elements have also been encapsulated inside CNTs.156,157
3. Important properties of functionalized CNTs
A variety of oligomeric and polymeric compounds have been used in the functionalization of CNTs due to their solubility in common organic solvents and water.158–160 This functionalization breaks nanotube bundles, which is essential to the solubility. For example the carboxylic groups of f-CNTs could be ionically bound to metal ions, or get covalently linked through different organic groups. Increased solubility due to exfoliation of the nanotube bundles is the most important, common, and frequently reported property of functionalized CNTs. The amide and ester functionalities dramatically improve their solubility in organic solvents. Similar solubility properties were also obtained for amine zwitterion functionalized SWCNTs. In addition, polymer wrapped CNTs are also among the most soluble samples. SWCNTs and MWCNTs functionalized with highly soluble PPEI-EI, PVA-VA and PEO polymers as well as with PEO-derived dendron moieties are soluble not only in organic solvents but also in water. Glucosamine and protein conjugates with CNTs are highly soluble in water. Attaching transition metal complexes to nanotubes increases their solubility in dimethylformamide, dimethylsulphoxide, tetrahydrofuran, methanol, chloroform, toluene etc. Strong luminescence was reported for CNTs chemically bound to PPEI-EI, PVA-VA polymers, and PEO derived dendron moieties. Luminescence quantum yields were found to be substantial, 11% for MWCNT-PPEI-EI in chloroform at 400 nm excitation.
4. Applications of functionalized CNTs
Functionalized CNTs find wide application in different areas of materials science, biomedical science, water purification and drug delivery systems as depicted in Table 5. The details are discussed below:
Table 5 Application of functionalized CNTs
f-CNT compound (chemical structure) |
Application area |
Advantages offered |
References |
Polyethylene/polystyrene/PMMA-covalently functionalized SWCNTs |
Advanced polymeric nanocomposites |
Enhanced dispersion of CNTs in polymer matrices |
43 and 161–165 |
Increased interfacial bonding |
Polymer encapsulated CNTs–Nylon 6 nanocomposite |
Advanced polymeric nanocomposites |
Improved interfacial adhesion between polymer encapsulated CNTs and Nylon 6 |
125
|
Metal NP supported CNTs |
Catalysis |
Improved catalytic activity and selectivity |
168–173
|
CNTs functionalized with Vaskas compound |
Catalysis and molecular electronics |
Enhanced solubility with solution durability |
70
|
CdSe QDs loaded multiamino f-CNTs & magnetic Fe3O4 NPs for nano sensors, optoelectronics |
Nano sensors, optoelectronics, catalysis etc. |
Optical property of QDs and magnetic properties of Fe3O4 nanoparticles were maintained |
174
|
Metal coated and metal filled CNTs |
Stealth technology |
Enhanced EM absorbing properties |
175 and 176 |
SWCNTs/PmPV |
Photovoltaic devices |
Enhanced optoelectronic properties |
119 and 120 |
Immobilization of DNA, proteins, oligonucleotides etc. |
Miniaturized novel biosensors |
Coupling of NTs electronic properties with recognition properties of immobilized biosystems |
177–181
|
Chemically functionalized CNTs |
Substrate for neuronal growth |
Controlled neuronal processes by charge manipulation of f-CNTs |
182
|
Glucose oxidase attached CNTs |
Single-molecule biosensors |
Controlled enzymatic activity |
183
|
Covalently linked nanotube peptide conjugates |
New bioactive nanomaterials |
Enhanced in vivo antibody response |
185 and 186 |
Peptide wrapped SWCNTs |
Designing & identification of peptide sequences |
Identification using phage display |
187
|
f-SWCNTs-ethylene oxide surfactants, 4-hydroxynonenal-MWCNTs |
Biomolecular recognition |
Detection of antibodies binding |
188
|
Adsorption of specific antibody |
CNTs and telomeric DNA fragment containing noncanonical G-quadruplex and i-motif forms |
Molecular dynamics simulation studies |
Encoding of CNTs with tubular nucleic acids for information storage |
189–191
|
Functionalized and cross linked CNTs |
Water purification |
Highly efficient and targeted removal of chemical and biological contaminants |
192, 193, 205, 207, 208, 210 and 211 |
Metal oxide coated CNTs |
Electrocatalytic water treatment |
Treatment by electrocatalytic process |
194 and 195 |
Magnetic CNTs, f-CNTs |
Separation of hydrocarbon and oil from water |
Enhanced separation capacity |
196–199
|
Rim functionalized CNTs, zwitterion functionalized CNTs/polyamide nanocomposite membranes, electrochemically modified CNTs |
Water desalination |
Enhanced performance |
200–203
|
Amine functionalized multi walled carbon nanotubes (MWCNTs)-polyimide membranes |
Organic solvent nanofiltration |
Enhanced flux |
204
|
PEG-CNTs |
Battery wastewater |
Specific removal of chromium and zinc |
206
|
Functionalized CNTs |
Drug delivery |
Biocompatibility and targeted drug delivery |
212, 213, 215–217 and 221 |
Polymeric nanohybrids and f-CNTs |
Drug delivery |
Targeted drug delivery for cancer therapy |
214
|
COOH functionalized SWCNTs |
Drug delivery |
Targeted delivery of cladribine and flutamide anticancer drug for in a gas phase and water solution |
218 and 219 |
PEG-SWCNTs |
Drug delivery |
Targeted drug delivery for cancer |
220
|
4.1 Materials science
In the area of materials science, f-CNTs are being widely used for the development of high quality nanocomposites. The use of f-CNTs for fabricating advanced polymeric composites43,161–163 offers significant advantages over the use of non functionalized CNTs, by increasing the dispersion of carbon nanotubes in polymeric matrices. The functional moiety increases the interfacial bonding, thereby, lessening fiber pullout during mechanical stress or entanglement in contrast to unfunctionalized CNTs where dispersion and interfacial bonding or entanglement is minimal. For example covalently functionalized SWCNTs have been used as fillers at >1 wt% concentration in polymeric hosts such as PMMA, PS, etc. For PMMA, tensile modulus increased by more than a factor of 2 with only 0.1 wt% f-SWCNTs added and for polystyrene f-SWCNT nanocomposites a substantial increase in viscosity and elasticity of the system was observed at low shear rates even at 1 wt% loading compared to 3 wt% pristine CNT loading which remained like a liquid.164,165 It was found that polymer encapsulated CNTs could be well dispersed in a Nylon 6 matrix compared to unmodified CNTs, due to improved interface adhesion between the polymer encapsulated CNTs and Nylon 6. It was found that yield strength improved by 30% and Youngs modulus by 35% at only 1 wt% content of polymer encapsulated CNTs.125 Thus the solubilization of CNTs via chemical functionalization is an effective approach to achieve a homogeneous dispersion of CNTs in polymer matrices for high quality nanocomposites.
Functionalized CNTs also possess wide application in catalysis by acting as support materials. V. O. Nyamori et al. reviewed various surface functionalization techniques for improving CNT properties for use as a catalyst support, and also available methods for catalyst nanoparticles loading on f-CNTs.166 B. Ye et al.167 reported oxygen functionalized CNTs for selective catalytic reduction of NOx with NH3. Oxygen functional groups were generated on the surface of CNTs by acid treatment, which acted as anchoring sites for nanoparticles of different metals. The synthesized functional materials exhibited an enhanced NOx removal efficiency of over 90% at 350–380 °C. More studies have been carried out in order to demonstrate that metal nanoparticles supported on carbon nanotubes provide much improved catalytic activity.168–172 Q. Wang et al. have173 reported that Pt nanoparticles deposited on Nafion® functionalized CNTs show much higher catalytic activity for the oxygen reduction reaction. The synthesized material also showed a slower decomposition rate in an electrochemically accelerated durability test making it a potential electrocatalyst for fuel cell applications.
In addition to metal coated CNTs, metal filled CNTs also have shown promising applications in heterogeneous catalysis. Gao et al. reported a simple strategy for loading multiamino f-CNTs with CdSe QDs and magnetic Fe3O4 nanoparticles via electrostatic attractions. It was observed that both the optical properties of QDs and magnetic properties of Fe3O4 nanoparticles were maintained and the resulting products showed potential applications in nano sensors, optoelectronics, catalysis and other fields.174 In addition to catalysis, metal coated and metal filled CNTs find wide application in stealth technology due to their EM absorbing properties.175,176
4.2 Biomedical science
Immobilization of biomolecules on CNTs for coupling the electronic properties of NTs with the specific recognition properties of the immobilized biosystems has motivated researchers to use CNTs as a new type of miniaturized biosensor materials.177,178 For example metallothionein proteins were trapped inside and placed on to the outer surfaces of open ended MWCNTs.179,180 Streptavidin was also found to adsorb on MWCNTs presumably via hydrophobic interactions between the nanotubes and hydrophobic domains of the proteins. DNA molecules adsorbed on MWCNTs via non specific interactions were also observed.178–180 In addition to MWCNTs, SWCNTs have also been noncovalently functionalized, and various biological molecules have subsequently been immobilized onto f-SWCNTs with a high degree of control and specificity.181 Haddon et al.182 reported the use of chemically modified CNTs as a substrate for growth of neurons. It was observed that by manipulating the charge carried by f-CNTs the outgrowth and branching pattern of neuronal processes could be controlled. Dekker et al.183 reported that redox enzyme glucose oxidase when attached to CNTs preserved their enzymatic activity. Also, the enzyme-coated tube was found to act as a pH sensor with large and reversible changes in conductance upon changes in pH.
Different types of biomolecular interactions with CNTs sidewalls have been explored extensively. For example water soluble IPEG-SWCNT samples have been used as a starting material in the exchange reaction with bovine serum albumin (BSA) protein under ambient conditions for the preparation of SWCNTs–BSA conjugates. IPEG-SWCNTs is also soluble in organic solvents such as chloroform, but the SWCNTs–BSA conjugate is only soluble in water. Thus the solubility change acts as an indicator for ester to amide transformation in the transformation reaction. The TEM results have confirmed that the conjugate sample contains well dispersed SWCNTs.
Functionalized carbon nanotubes have also been reported for targeting of Amphotericin B to cells.184 The association of CNTs with peptides is expected to be useful in biosensor applications and in the development of new bioactive nanomaterials. For example Pantarotto et al.185 demonstrated an enhanced in vivo antibody response from covalently linked nanotube peptide conjugates and Wang et al.186 reported identification of peptide sequences having specific affinities for CNTs by using a phage display. Zorbas et al.187 designed an amphiphilic peptide sequence which folds into an α-helix on nanotube sidewalls. For sensing Shim et al.188 functionalized substrate grown SWCNTs with ethylene oxide based surfactants. Subsequently these non-ionic surfactants were associated covalently with proteins to electrically detect the binding of antibodies. MWCNTs have been functionalized with 4-hydroxynonenal (4-HNE) to induce adsorption of 4-HNE antibody. This effect is interesting for the development of biosensors, because the electronic properties of the tubes can be combined with the recognition properties of the immobilized biosystems. In addition to this, molecular dynamics simulation studies have been carried out for systems composed of CNTs and Telomeric DNA fragment containing noncanonical G-quadruplex and i-Motif forms.189,190 Y. Zhang et al. also reported the encoding of CNTs with tubular nucleic acids for information storage, as DNA interacts with CNTs in a sequence specific manner resulting in different conformations including helix, i-motif, and G-quadruplex.191
4.3 Water purification
Research on safe effective and economic materials that can eliminate current and future contaminations from water is a scientific and technological issue of primary importance to the scientific community and scientists all over the world are deeply involved in this research. Among many materials developed for the said purpose CNTs especially functionalized carbon nanotubes have been widely researched for the said application. K. M. Lee et al.192 reviewed the adsorptive potential of f-CNTs for removal of water pollutants. M. Barrejón et al. studied the adsorptive removal of water contaminants by using chemically crosslinked SWCNTs.193 They reported that the cross-linked CNT adsorbents exhibited selective sorption capability along with good recyclability for removal of oils and organics from contaminated water. Jame et al.194 reviewed the use of electrochemically active CNTs filters as an efficient technology for water and wastewater treatment targeting chemical and biological contaminants. The advantage of using electrochemically active CNTs filters is that they provide additional electrooxidation of the adsorbed contaminants thus increasing the effectiveness of treatment. The working principles, impacting factors and latest developments of electrochemically active CNT filters along with challenges and future perspectives have been discussed. S. Y. Yang et al.195 studied metal oxide modified CNTs filters for electrocatalytic water treatment especially for the removal of phenol. It was observed that the performance of modified CNT performance was approximately twice when compared to that with bare CNT filters. In the wide spectrum of water contaminants magnetic CNTs (MCNTs) have been used by Wang et al.196 for the removal of oil droplets from contaminated water. The authors have designed, fabricated and experimentally evaluated superparamagnetic iron oxide nanoparticle (SPIONs) decorated CNTs for oil–water separation. They have shown that the synthesized material removes oil through a two step mechanism, where in the first step MCNTs get dispersed at the oil–water interface and in the second step drag the oil droplets with them out of water by a magnet. They have also mentioned that 80% of the separation capacity could be restored by a 10 min wash by using 1 ml ethanol for every 6 mg of MCNTs. J. Liu et al.197 used functionalized multiwalled carbon nanotubes for separation of emulsified oil from oily wastewater. According to a recent article by N. Al-Jammal198 functionalized carbon nanotubes have been used for hydrocarbon removal from water. As per a recent report by T. A. Abdullah et al.199 f-CNTs have been successfully used for oil spill clean up from water. Functionalized CNTs have also been used for desalination. Y. Hong et al. reported the use of rim functionalized carbon nanotubes for water desalination.200 In a membrane form, zwitterion functionalized carbon nanotube/polyamide nanocomposite membranes have been used for water desalination.201 Z. Z. Chowdhury et al.202 reviewed the physio-chemical aspect along with the working mechanism of electrochemically modified CNT membranes for desalination and water purification. Das et al.203 reported the synthesis and evaluation of functionalized carbon nanotube based polymer composite nanofiltration membranes for desalination. Farahani204et al. also reported the study of amine functionalized multi walled carbon nanotube (MWCNTs) based nanofiltration membranes for desalination due to their high adsorption capacity. I. G. Wenten et al.205 reviewed the progress of CNT functionalization applied to membrane filtration with respect to membrane properties and their performance. W. A. Hamzat et al.206 studied the performance of purified carbon nanotubes (P-CNTs) and polyethylene glycol carbon nanotubes (PEG-CNTs) to remove specifically chromium (Cr) and zinc (Zn) from battery wastewater by a batch adsorption process as a function of contact time, adsorbent dose and temperature. The results revealed that the optimum contact time and adsorbent dosage to remove Cr and Zn by P-CNTs and PEG-CNTs were 90 and 50 min and 0.3 g respectively. The study demonstrated that PEG-CNTs performed better compared to P-CNTs. E. M. Elsehly et al.207 reported the use of functionalized carbon nanotubes for fabrication of filters to remove chromium from aqueous solutions. Removal of heavy metals from water by using multistage functionalized multiwall carbon nanotubes has been reported by D. Budimirović et al.208 In a recent report by M. M. Aslam et al.209 the preparation and application of f-CNTs for water and wastewater treatment have been reviewed. It was observed that, functionalized CNTs have proven to be a promising nanomaterial for the decontamination of water particularly due to their high adsorption capacity. A. A. Atiyah et al.210 reported excellent antibacterial activity of silver nanoparticle deposited MWCNTs against two pathogenic bacteria, Pseudomonas aeruginosa and Staphylococcus aureus. Recently S. M. Al-Hakami et al.211 reported the use of microwave irradiated 1-octadecanol (C18) functionalized CNTs for removal of Escherichia coli (E. coli) from water. They observed that microwave irradiated unmodified CNTs removed only up to 98% of bacteria from water, while CNTs-C18 were able to remove up to 100% of bacteria under similar conditions.
4.4 Drug delivery system
Functionalized nanotubes have emerged as a novel and versatile drug delivery vehicles due to their high drug loading efficiency along with an ultrahigh surface area.212 S. Sharma et al. studied the effect of functionalization on the drug delivery potential of CNTs where the results showed extended resistance time with a sustained release profile for drug loaded surface engineered MWCNTs.213 S. Prakash et al. reviewed the use of f-CNTs as drug delivery vehicles in relation to cancer therapy.214 C. L. Lay et al. also studied the use of f-CNTs for anticancer drug delivery. They reported that the most widely adopted species for functionalization is poly(ethylene glycol), which enhances the biocompatibility and dispersability of CNTs in aqueous solution. Treatment efficacy of f-CNTs loaded with anticancer drugs, such as doxorubicin, and paclitaxel was studied both by in vitro and in vivo techniques. The results demonstrated that f-CNTs were promising for development of unique delivery systems for anticancer drugs.215 J. M. Tan et al.216 reviewed the biocompatibility of f-CNTs in designing drug delivery systems, where drug release from CNTs was achieved by utilizing various stimulants such as magnetic or electric field and changes in pH. They have discussed various parameters responsible for achieving higher therapeutic efficiency of targeted drug delivery. Recently, S. Paliwal et al.,217 reviewed f-CNTs as a nano carrier drug delivery system.
Molecular modeling studies have also been performed to gain a mechanistic understanding of surface functionalization of carbon nanotubes as a nanocarrier with cladribine anticancer drug.218 Studies have been conducted by means of density functional theory and molecular dynamics simulation calculations to investigate the drug delivery performance of the functionalized SWCNTs with a carboxylic acid group for flutamide anticancer drug in the gas phase as well as water solution.219 A. A. Bhirde et al.220 studied the distribution and clearance of polyethylene glycol (PEG)-SWCNTs as drug delivery vehicles for the anticancer drug cisplatin, where tumor growth inhibition was studied in mice. The studies revealed that control SWCNTs were lodged in lung tissues as large aggregates compared to PEG-SWCNTs, which showed little or no accumulation. J. Chen et al.32 studied the use of f-SWCNTs as a vehicle for tumor-targeted drug delivery. The results showed high potency of material towards specific cancer cell lines. In a recent review, J. Jampilek et al.221 summarized the latest designed nanosystems based on functionalized graphene and carbon nanotubes mainly for anticancer therapy.
5. Future recommendations
Functionalization of CNTs has allowed the drawbacks associated with pristine CNTs222,223 to be overcome as well as tuning their properties for specific applications.224–230 Further, the availability of a wide variety of functionalization approaches discussed in this article gives an opportunity for judicious selection of a particular method resulting in required functionality and performance. Not only have functional groups been added to the surface and tips of CNTs, but these unique nanomaterials have been used as a carrier vehicle loaded with a variety of biological molecules and drugs for their targeted delivery to any organ of the human body. As the application of f-CNTs in different areas is increasing, hazards due to their exposure to both humans and the environment are also increasing. Although a lot of studies have been carried out from time to time drawing the attention of the scientific community towards unexplored drawbacks and risks associated with this material,205,231–237 still deeper understanding of their harm to the environment and human body is urgently required.
In this respect a variety of novel functional groups are being reported by different researchers for tackling the biocompatibility issue. Such groups may be incorporated to functionalize carbon nanotubes in order to enhance their biocompatibility. A massive amount of work has been done on the functional properties of f-CNTs, which beyond doubt enhances the applicability of this material. However, an additional volume of work is yet to be undertaken for making this material bio-friendly and environment friendly. To decrease the toxicity concern of f-CNTs, computational tools can also be employed such as Autodock, Gaussian, etc. These tools along with their experimental validation may render this material a well accepted functional material.
6. Conclusion
CNTs have attracted attention of the scientific community due to their unique properties and wide application in the various areas of science and technology. But they also have some disadvantages preventing them being ideal materials for proposed applications. The primary one is the cost. Conventionally they are synthesized by different methods especially by arc discharge, laser vaporization and CVD. But now various modifications of these technique and also new techniques have emerged, which lower the cost considerably. On the other hand raw carbon nanotubes contain impurities such as metal nanoparticles and amorphous carbon which are to be removed by treatment with strong acids and oxidizing agents. The purified CNTs are inert and tend to agglomerate due to strong van der Waals forces and hence the dispersion and the reinforcing effect get suppressed. To overcome the aggregation and to increase dispersion, functionalization is needed. Different covalent and non covalent exohedral and endohedral functionalization techniques are used to improve their functional properties. The functionalized CNTs provide possibility of further chemical derivatization and thus have a greater versatility for applications of CNTs in different areas such as materials science, biomedical science and water purification. Functionalized carbon nanotubes have been widely studied and successfully used as adsorbents for removal of toxic contaminants such as chromium, arsenic, zinc, etc. along with liquid contaminants such as oils and organic solvents. They have been used for fabrication of ultra and nano filtration membranes for desalination application. Functionalized carbon nanotubes have also been used for water disinfection by removal of pathogenic microorganisms from water. Functionalized CNTs are also useful for delivery of drugs in solid tumors, and cancer and a plethora of other biomedical applications.
Conflicts of interest
There are no conflicts to declare.
Acknowledgements
The authors wish to express their gratitude to the Director Dr Sanjai Kumar Dwivedi, Defence Research Laboratory for giving permission to publish this review article.
References
- S. Iijima, Nature, 1991, 354, 56–58 CrossRef CAS.
- B. T. Yermagambet, M. K. Kazankapova, Z. M. Kassenova and A. T. Nauryzbayeva, Number, 2020, 5, 126–133 Search PubMed.
- K. K. Singh, S. K. Chaudhary, R. Venugopal and A. Gaurav, Proc. Inst. Mech. Eng., Part N, 2017, 231, 141–151 CAS.
- K. Anazawa, K. Shimotani, C. Manabe, H. Watanabe and M. Shimizu, Appl. Phys. Lett., 2002, 81, 739–741 CrossRef CAS.
- Z. Shi, Y. Lian, F. H. Liao, X. Zhou, Z. Gu, Y. Zhang, S. Iijima, H. Li, K. T. Yue and S. L. Zhang, J. Phys. Chem. Solids, 2000, 61, 1031–1036 CrossRef CAS.
- C. Journet, W. K. Maser, P. Bernier, A. Loiseau, M. Lamy de la Chapelle, S. Lefrant, P. Deniard, R. Lee and J. E. Fischer, Nature, 1997, 388, 756–758 CrossRef CAS.
- X. Sun, W. Bao, Y. Lv, J. Deng and X. Wang, Mater. Lett., 2007, 61, 3956–3958 CrossRef CAS.
- S. Arepalli, J. Nanosci. Nanotechnol., 2004, 4, 317–325 CrossRef CAS PubMed.
-
M. S. Hasnain and A. K. Nayak, in SpringerBriefs in Applied Sciences and Technology, Springer Verlag, 2019, pp. 17–20 Search PubMed.
- B. Verma, H. Sewani and C. Balomajumder, Environ. Sci. Pollut. Res., 2020, 27, 14007–14018 CrossRef CAS PubMed.
- C. J. Lee, J. H. Park and J. Park, Chem. Phys. Lett., 2000, 323, 560–565 CrossRef CAS.
- W. Z. Li, D. Z. Wang, S. X. Yang, J. G. Wen and Z. F. Ren, Chem. Phys. Lett., 2001, 335, 141–149 CrossRef CAS.
- Y. F. Zhang, Y. H. Tang, Y. Zhang, C. S. Lee, I. Bello and S. T. Lee, Chem. Phys. Lett., 2000, 330, 48–52 CrossRef CAS.
- M. Su, B. Zheng and J. Liu, Chem. Phys. Lett., 2000, 322, 321–326 CrossRef CAS.
- O. Noury, T. Stoöckli, M. Croci and A. Chaâtelain Jean-Marc Bonard, Chem. Phys. Lett., 2001, 346, 349–355 CrossRef CAS.
- R. S. Rajaura, I. Singhal, K. N. Sharma and S. Srivastava, Rev. Sci. Instrum., 2019, 90, 123903 CrossRef PubMed.
- P. Nikolaev, M. J. Bronikowski, R. K. Bradley, F. Rohmund, D. T. Colbert, K. A. Smith and R. E. Smalley, Chem. Phys. Lett., 1999, 313, 91–97 CrossRef CAS.
- R. L. Vander Wal, G. M. Berger and L. J. Hall, J. Phys. Chem. B, 2002, 106, 3564–3567 CrossRef CAS.
- D. Huličová, K. Hosoi, S. -I. Kuroda, H. Abe and A. Oya, Adv. Mater., 2002, 14, 452–455 CrossRef.
- K. Edgar and J. L. Spencer, Curr. Appl. Phys., 2004, 4, 121–124 CrossRef.
- R. C. Z. Lofrano, J. M. Rosolen and L. A. Montoro, Carbon, 2005, 43, 200 CrossRef.
- M. Shao, D. Wang, G. Yu, B. Hu, W. Yu and Y. Qian, Carbon, 2004, 42, 183–185 CrossRef CAS.
- M. wei Li, Z. Hu, X. zhang Wang, Q. Wu, Y. Chen and Y. L. Tian, Diam. Relat. Mater., 2004, 13, 111–115 CrossRef.
- Y. L. Li, I. A. Kinloch, M. S. P. Shaffer, J. Geng, B. Johnson and A. H. Windle, Chem. Phys. Lett., 2004, 384, 98–102 CrossRef CAS.
- S. R. C. Vivekchand, L. M. Cele, F. L. Deepak, A. R. Raju and A. Govindaraj, Chem. Phys. Lett., 2004, 386, 313–318 CrossRef CAS.
- D. H. Robertson, D. W. Brenner and J. W. Mintmire, Phys. Rev. B: Condens. Matter Mater. Phys., 1992, 45, 12592–12595 CrossRef PubMed.
- M. F. Yu, B. S. Files, S. Arepalli and R. S. Ruoff, Phys. Rev. Lett., 2000, 84, 5552–5555 CrossRef CAS PubMed.
- B. I. Yakobson, C. J. Brabec and J. Bernholc, Phys. Rev. Lett., 1996, 76, 2511–2514 CrossRef CAS PubMed.
- S. J. Tans, A. R. M. Verschueren and C. Dekker, Nature, 1998, 393, 49–52 CrossRef CAS.
- B. Q. Wei, R. Vajtai and P. M. Ajayan, Appl. Phys. Lett., 2001, 79, 1172–1174 CrossRef CAS.
- J. Hone, M. C. Llaguno, M. J. Biercuk, A. T. Johnson, B. Batlogg, Z. Benes and J. E. Fischer, Appl. Phys. Mater. Sci. Process, 2002, 74, 339–343 CrossRef CAS.
- J. Chen, S. Chen, X. Zhao, L. V. Kuznetsova, S. S. Wong and I. Ojima, J. Am. Chem. Soc., 2008, 130, 16778–16785 CrossRef CAS PubMed.
- F. Ito, K. Konuma and A. Okamoto, J. Appl. Phys., 2001, 89, 8141–8145 CrossRef CAS.
- P. Avouris, Acc. Chem. Res., 2002, 35, 1026–1034 CrossRef CAS PubMed.
- B. Safadi, R. Andrews and E. A. Grulke, J. Appl. Polym. Sci., 2002, 84, 2660–2669 CrossRef CAS.
- S. Fan, M. G. Chapline, N. R. Franklin, T. W. Tombler, A. M. Cassell and H. Dai, Science, 1999, 283, 512–514 CrossRef CAS PubMed.
- Q. H. Wang, A. A. Setlur, J. M. Lauerhaas, J. Y. Dai, E. W. Seelig and R. P. H. Chang, Appl. Phys. Lett., 1998, 72, 2912–2913 CrossRef CAS.
- A. C. Dillon, K. M. Jones, T. A. Bekkedahl, C. H. Kiang, D. S. Bethune and M. J. Heben, Nature, 1997, 386, 377–379 CrossRef CAS.
-
S. Mudassir Ali, K. Venkateswara Rao, J. V. Ramana Rao, V. Himabindu, M. Venkateswara Rao and V. Viditha, in Proceedings of the International Conference on Nanoscience, Engineering and Technology, ICONSET, 2011, pp. 1–4 Search PubMed.
- L. Moreira, R. Fulchiron, G. Seytre, P. Dubois and P. Cassagnau, Macromolecules, 2010, 43, 1467–1472 CrossRef CAS.
- Q. Chen, C. Saltiel, S. Manickavasagam, L. S. Schadler, R. W. Siegel and H. Yang, J. Colloid Interface Sci., 2004, 280, 91–97 CrossRef CAS PubMed.
- C. A. Dyke and J. M. Tour, Chem.–Eur. J., 2004, 10, 812–817 CrossRef CAS PubMed.
- J. Zhu, J. D. Kim, H. Peng, J. L. Margrave, V. N. Khabashesku and E. V. Barrera, Nano Lett., 2003, 3, 1107–1113 CrossRef CAS.
- Q. S. Yang, B. Q. Li, X. Q. He and Y. W. Mai, Adv. Condens. Matter Phys., 2014, 2014, 1–8 Search PubMed.
- M. Noordadi, F. Mehrnejad, R. H. Sajedi, M. Jafari and B. Ranjbar, PLoS One, 2018, 13, e0198519 CrossRef PubMed.
- P. Wolski, K. Nieszporek and T. Panczyk, Phys. Chem. Chem. Phys., 2017, 19, 9300–9312 RSC.
- S. Merum, J. B. Veluru and R. Seeram, Mater. Sci. Eng., B, 2017, 223, 43–63 CrossRef CAS.
- B. V. Basheer, J. J. George, S. Siengchin and J. Parameswaranpillai, Nano-Struct. Nano-Objects, 2020, 22, 100429 CrossRef CAS.
- R. Alshehri, A. M. Ilyas, A. Hasan, A. Arnaout, F. Ahmed and A. Memic, J. Med. Chem., 2016, 59, 8149–8167 CrossRef CAS PubMed.
-
S. Beg, M. Rahman, A. Jain, S. Saini, M. S. Hasnain, S. Swain, S. Imam, I. Kazmi and S. Akhter, in Fullerens, Graphenes and Nanotubes, Elsevier, 2018, pp. 105–133 Search PubMed.
- C. Pennetta, G. Floresta, A. C. E. Graziano, V. Cardile, L. Rubino, M. Galimberti, A. Rescifina and V. Barbera, Nanomaterials, 2020, 10, 1073 CrossRef CAS PubMed.
- A. A. Farghali, H. A. Abdel Tawab, S. A. Abdel Moaty and R. Khaled, J. Nanostructure Chem., 2017, 7, 101–111 CrossRef CAS.
- S. Ali, S. A. U. Rehman, H.-Y. Luan, M. U. Farid and H. Huang, Sci. Total Environ., 2019, 646, 1126–1139 CrossRef CAS PubMed.
- A. J. Haider, T. R. Marzoog, I. H. Hadi and Z. N. Jameel, J. Environ. Manage., 2018, 246, 030013 Search PubMed.
- L. Ma, X. Dong, M. Chen, L. Zhu, C. Wang, F. Yang and Y. Dong, Membranes, 2017, 7, 16 CrossRef PubMed.
- E. T. Mickelson, C. B. Huffman, A. G. Rinzler, R. E. Smalley, R. H. Hauge and J. L. Margrave, Chem. Phys. Lett., 1998, 296, 188–194 CrossRef CAS.
- E. T. Mickelson, I. W. Chiang, J. L. Zimmerman, P. J. Boul, J. Lozano, J. Liu, R. E. Smalley, R. H. Hauge and J. L. Margrave, J. Phys. Chem. B, 1999, 103, 4318–4322 CrossRef CAS.
- V. N. Khabashesku, W. E. Billups and J. L. Margrave, Acc. Chem. Res., 2002, 35, 1087–1095 CrossRef CAS PubMed.
- P. J. Boul, J. Liu, E. T. Mickelson, C. B. Huffman, L. M. Ericson, I. W. Chiang, K. A. Smith, D. T. Colbert, R. H. Hauge, J. L. Margrave and R. E. Smalley, Chem. Phys. Lett., 1999, 310, 367–372 CrossRef CAS.
- S. Pekker, J. P. Salvetat, E. Jakab, J. M. Bonard and L. Forró, J. Phys. Chem. B, 2001, 105, 7938–7943 CrossRef CAS.
- B. N. Khare, M. Meyyappan, A. M. Cassell, C. V. Nguyen and J. Han, Nano Lett., 2002, 2, 73–77 CrossRef CAS.
- N. Tagmatarchis and M. Prato, J. Mater. Chem., 2004, 14, 437 RSC.
- V. Georgakilas, K. Kordatos, M. Prato, D. M. Guldi, M. Holzinger and A. Hirsch, J. Am. Chem. Soc., 2002, 124, 760–761 CrossRef CAS PubMed.
- J. L. Bahr, J. Yang, D. V. Kosynkin, M. J. Bronikowski, R. E. Smalley and J. M. Tour, J. Am. Chem. Soc., 2001, 123, 6536–6542 CrossRef CAS PubMed.
- J. L. Bahr and J. M. Tour, Chem. Mater., 2001, 13, 3823–3824 CrossRef CAS.
- F. J. M. Holzinger, O. Vostrowsky, A. Hirsch, M. Kappes and R. Weiss, Angew. Chem., Int. Ed., 2001, 40, 4002–4005 CrossRef PubMed.
- M. Holzinger, J. Abraham, P. Whelan, R. Graupner, L. Ley, F. Hennrich, M. Kappes and A. Hirsch, J. Am. Chem. Soc., 2003, 125, 8566–8580 CrossRef CAS PubMed.
- Y. Ying, R. K. Saini, F. Liang, A. K. Sadana and W. E. Billups, Org. Lett., 2003, 5, 1471–1473 CrossRef CAS PubMed.
- N. Tagmatarchis, V. Georgakilas, M. Prato and H. Shinohara, Chem. Commun., 2002, 2, 2010–2011 RSC.
- S. Banerjee and S. S. Wong, Nano Lett., 2002, 2, 49–53 CrossRef CAS.
- S. Banerjee and S. S. Wong, J. Am. Chem. Soc., 2002, 124, 8940–8948 CrossRef CAS PubMed.
- P. X. Hou, S. Bai, Q. H. Yang, C. Liu and H. M. Cheng, Carbon, 2002, 40, 81–85 CrossRef CAS.
- H. Hiura, T. W. Ebbesen and K. Tanigaki, Adv. Mater., 1995, 7, 275–276 CrossRef CAS.
- S. C. Tsang, Y. K. Chen, P. J. F. Harris and M. L. H. Green, Nature, 1994, 372, 159–162 CrossRef CAS.
- R. M. Lago, S. C. Tsang, K. L. Lu, Y. K. Chen and M. L. H. Green, J. Chem. Soc., Chem. Commun., 1995, 1355–1356 RSC.
- K. C. Hwang, J. Chem. Soc., Chem. Commun., 1995, 173–174 RSC.
- S. R. P. W. Chiu, G. S. Duesberg and U. D. Weglikowska, Appl. Phys. Lett., 2002, 80, 3811–3813 CrossRef.
- N. Zhang, J. Xie and V. K. Varadan, Smart Mater. Struct., 2002, 11, 962 CrossRef CAS.
- V. Datsyuk, M. Kalyva, K. Papagelis, J. Parthenios, D. Tasis, A. Siokou, I. Kallitsis and C. Galiotis, Carbon, 2008, 46, 833–840 CrossRef CAS.
- S. Liang, G. Li and R. Tian, J. Mater. Sci., 2016, 51, 3513–3524 CrossRef CAS.
- M. A. Hamon, J. Chen, H. Hu, Y. Chen, M. E. Itkis, A. M. Rao, P. C. Eklund and R. C. Haddon, Adv. Mater., 1999, 11, 834–840 CrossRef CAS.
- S. Niyogi, M. A. Hamon, H. Hu, B. Zhao, P. Bhowmik, R. Sen, M. E. Itkis and R. C. Haddon, Acc. Chem. Res., 2002, 35, 1105–1113 CrossRef CAS PubMed.
- Y. P. Sun, W. Huang, Y. Lin, K. Fu, A. Kitaygorodskiy, L. A. Riddle, Y. J. Yu and D. L. Carroll, Chem. Mater., 2001, 13, 2864–2869 CrossRef CAS.
- K. Fu, W. Huang, Y. Lin, L. A. Riddle, D. L. Carroll and Y. P. Sun, Nano Lett., 2001, 1, 439–441 CrossRef CAS.
- Y. P. Sun, K. Fu, Y. Lin and W. Huang, Acc. Chem. Res., 2002, 35, 1096–1104 CrossRef CAS PubMed.
- F. Pompeo and D. E. Resasco, Nano Lett., 2002, 2, 369–373 CrossRef CAS.
- J. Chen, A. M. Rao, S. Lyuksyutov, M. E. Itkis, M. A. Hamon, H. Hu, R. W. Cohn, P. C. Eklund, D. T. Colbert, R. E. Smalley and R. C. Haddon, J. Phys. Chem. B, 2001, 105, 2525–2528 CrossRef CAS.
- M. F. Variava, T. L. Church, A. T. Harris and A. I. Minett, J. Mater. Chem. A, 2013, 1, 8509 RSC.
- V. Lordi, N. Yao and J. Wei, Chem. Mater., 2001, 13, 733–737 CrossRef CAS.
- R. Yu, L. Chen, Q. Liu, J. Lin, K. L. Tan, S. C. Ng, H. S. O. Chan, G. Q. Xu and T. S. A. Hor, Chem. Mater., 1998, 10, 718–722 CrossRef CAS.
- W. Zhang, J. Chen, G. F. Swiegers, Z.-F. Ma and G. G. Wallace, Nanoscale, 2010, 2, 282–286 RSC.
- L. Li and Y. Xing, J. Phys. Chem. C, 2007, 111, 2803–2808 CrossRef CAS.
- M. M. Waje, X. Wang, W. Li and Y. Yan, Nanotechnology, 2005, 16, S395–S400 CrossRef PubMed.
- J. Shi, Z. Wang and H. L. Li, J. Nanoparticle Res., 2006, 8, 743–747 CrossRef CAS.
- A. Muhammad, N. A. Yusof, R. Hajian and J. Abdullah, J. Mater. Res., 2016, 31, 2897–2905 CrossRef CAS.
- K. Jiang, A. Eitan, L. S. Schadler, P. M. Ajayan, R. W. Siegel, N. Grobert, M. Mayne, M. Reyes-Reyes, H. Terrones and M. Terrones, Nano Lett., 2003, 3, 275–277 CrossRef CAS.
- A. V. Ellis, K. Vijayamohanan, R. Goswami, N. Chakrapani, L. S. Ramanathan, P. M. Ajayan and G. Ramanath, Nano Lett., 2003, 3, 279–282 CrossRef CAS.
- G. L. Hwang, K. C. Hwang, Y. T. Shieh and S. J. Lin, Chem. Mater., 2003, 15, 1353–1357 CrossRef CAS.
- N. Sarlak, M. Adeli, M. Karimi, M. Bordbare and M. A. Farahmandnejad, J. Mol. Liq., 2013, 180, 39–44 CrossRef CAS.
- Y. Zhou, Y. Fang and R. Ramasamy, Sensors, 2019, 19, 392 CrossRef PubMed.
- N. Kocharova, T. Ääritalo, J. Leiro, J. Kankare and J. Lukkari, Langmuir, 2007, 23, 3363–3371 CrossRef CAS PubMed.
- C. Hu, H. Liao, F. Li, J. Xiang, W. Li, S. Duo and M. Li, Mater. Lett., 2008, 62(17–18), 2585–2588 CrossRef CAS.
- B. R. Priya and H. J. Byrne, J. Phys. Chem. C, 2008, 112, 332–337 CrossRef CAS.
- B. White, S. Banerjee, S. O'Brien, N. J. Turro and I. P. Herman, J. Phys. Chem. C, 2007, 111, 13684–13690 CrossRef CAS.
- T. J. McDonald, C. Engtrakul, M. Jones, G. Rumbles and M. J. Heben, J. Phys. Chem. B, 2006, 110, 25339–25346 CrossRef CAS PubMed.
- D. A. Britz and A. N. Khlobystov, Chem. Soc. Rev., 2006, 35, 637–659 RSC.
- H. Cui, X. Yan, M. Monasterio and F. Xing, Nanomaterials, 2017, 7, 262 CrossRef PubMed.
- M. F. Islam, E. Rojas, D. M. Bergey, A. T. Johnson and A. G. Yodh, Nano Lett., 2003, 3, 269–273 CrossRef CAS.
- R. Shvartzman-Cohen, Y. Levi-Kalisman, E. Nativ-Roth and R. Yerushalmi-Rozen, Langmuir, 2004, 20, 6085–6088 CrossRef CAS PubMed.
- O. V. Kharissova, B. I. Kharisov and E. G. de Casas Ortiz, RSC Adv., 2013, 3, 24812 RSC.
- J. Rausch, R.-C. Zhuang and E. Mäder, Compos. Appl. Sci. Manuf., 2010, 41, 1038–1046 CrossRef.
- Y. Shi, L. Ren, D. Li, H. Gao and B. Yang, J. Surf. Eng. Mater. Adv. Technol., 2013, 03, 6–12 Search PubMed.
- L. Jiang, L. Gao and J. Sun, J. Colloid Interface Sci., 2003, 260, 89–94 CrossRef CAS PubMed.
- D. Yin, P. Luo, J. Zhang, X. Yao, R. Wang, L. Wang and S. Wang, Appl. Sci., 2019, 9, 497 CrossRef CAS.
- M. J. O'Connell, P. Boul, L. M. Ericson, C. Huffman, Y. Wang, E. Haroz, C. Kuper, J. Tour, K. D. Ausman and R. E. Smalley, Chem. Phys. Lett., 2001, 342, 265–271 CrossRef.
- T. Fujigaya and N. Nakashima, Sci. Technol. Adv. Mater., 2015, 16, 024802 CrossRef PubMed.
- S. H. Tan, J. C. Goak, N. Lee, J.-Y. Kim and S. C. Hong, Macromol. Symp., 2007, 249–250, 270–275 CrossRef.
- M. Zheng, A. Jagota, E. D. Semke, B. A. Diner, R. S. McLean, S. R. Lustig, R. E. Richardson and N. G. Tassi, Nat. Mater., 2003, 2, 338–342 CrossRef CAS PubMed.
- D. W. Steuerman, A. Star, R. Narizzano, H. Choi, R. S. Ries, C. Nicolini, J. F. Stoddart and J. R. Heath, J. Phys. Chem. B, 2002, 106, 3124–3130 CrossRef CAS.
- A. Star, Y. Liu, K. Grant, L. Ridvan, J. F. Stoddart, D. W. Steuerman, M. R. Diehl, A. Boukai and J. R. Heath, Macromolecules, 2003, 36, 553–560 CrossRef CAS.
- B. Z. Tang and H. Xu, Macromolecules, 1999, 32, 2569–2576 CrossRef CAS.
- K. A. S. Fernando, Y. Lin, W. Wang, S. Kumar, B. Zhou, S. Y. Xie, L. S. T. Cureton and Y. P. Sun, J. Am. Chem. Soc., 2004, 126, 10234–10235 CrossRef CAS PubMed.
- R. J. Chen, Y. Zhang, D. Wang and H. Dai, J. Am. Chem. Soc., 2001, 123, 3838–3839 CrossRef CAS PubMed.
- H. Murakami, T. Nomura and N. Nakashima, Chem. Phys. Lett., 2003, 378, 481–485 CrossRef CAS.
- H. Xia, Q. Wang and G. Qiu, Chem. Mater., 2003, 15, 3879–3886 CrossRef CAS.
- Y. Kang and T. A. Taton, J. Am. Chem. Soc., 2003, 125, 5650–5651 CrossRef CAS PubMed.
- W. Zhou, S. Lv and W. Shi, Eur. Polym. J., 2008, 44, 587–601 CrossRef CAS.
- M. S. Arnold, M. O. Guler, M. C. Hersam and S. I. Stupp, Langmuir, 2005, 21, 4705–4709 CrossRef CAS PubMed.
- S. J. Pastine, D. Okawa, A. Zettl and J. M. J. Fréchet, J. Am. Chem. Soc., 2009, 131, 13586–13587 CrossRef CAS PubMed.
- L. Qu and L. Dai, J. Am. Chem. Soc., 2005, 127, 10806–10807 CrossRef CAS PubMed.
- H. C. Choi, M. Shim, S. Bangsaruntip and H. Dai, J. Am. Chem. Soc., 2002, 124, 9058–9059 CrossRef CAS PubMed.
- B. M. Quinn, C. Dekker and S. G. Lemay, J. Am. Chem. Soc., 2005, 127, 6146–6147 CrossRef CAS PubMed.
- P. J. Li, Y. Chai, X. L. Zhou, Q. F. Zhang and J. L. Wu, Fullerenes, Nanotub. Carbon Nanostruct., 2005, 13, 377–383 CrossRef CAS.
- Y. Zhang, N. W. Franklin, R. J. Chen and H. Dai, Chem. Phys. Lett., 2000, 331, 35–41 CrossRef CAS.
- K. Pichler, H. Kuzmany, H. Kataura and Y. Achiba, Phys. Rev. Lett., 2001, 87, 267401–267404 CrossRef PubMed.
- M. W. Maddox and K. E. Gubbins, J. Chem. Phys., 1997, 107, 9659–9667 CrossRef CAS.
- G. Stan and M. W. Cole, Surf. Sci., 1998, 395, 280–291 CrossRef CAS.
- X. Huang, H. Yu, H. Tan, J. Zhu, W. Zhang, C. Wang, J. Zhang, Y. Wang, Y. Lv, Z. Zeng, D. Liu, J. Ding, Q. Zhang, M. Srinivasan, P. M. Ajayan, H. H. Hng and Q. Yan, Adv. Funct. Mater., 2014, 24, 6516–6523 CrossRef CAS.
- T. Cui, X. Pan, J. Dong, S. Miao, D. Miao and X. Bao, Nano Res., 2018, 11, 3132–3144 CrossRef CAS.
- C. Guerret-Piécourt, Y. Le Bouar, A. Lolseau and H. Pascard, Nature, 1994, 372, 761–765 CrossRef.
- H. H. P. M. Ajayan, T. W. Ebbesen, T. Ichihashi, S. Iijima and K. Tanigaki, Nature, 1993, 362, 522–525 CrossRef.
- E. Dujardin, T. W. Ebbesen, H. Hiura and K. Tanigaki, Science, 1994, 265, 1850–1852 CrossRef CAS PubMed.
- B. W. Smith, M. Monthioux and D. E. Luzzi, Chem. Phys. Lett., 1999, 315, 31–36 CrossRef CAS.
- B. W. Smith and D. E. Luzzi, Chem. Phys. Lett., 2000, 321, 169–174 CrossRef CAS.
- G. H. Jeong, T. Hirata, R. Hatakeyama, K. Tohji and K. Motomiya, Carbon, 2002, 40, 2247–2253 CrossRef CAS.
- H. Kataura, Y. Maniwa, T. Kodama, K. Kikuchi, K. Hirahara, K. Suenaga, S. Iijima, S. Suzuki, Y. Achiba and W. Krätschmer, Synth. Met., 2001, 121, 1195–1196 CrossRef CAS.
- F. d B. Mota, E. F. Almeida Júnior and C. M. C. de Castilho, Braz. J. Phys., 2008, 38, 70–73 CrossRef.
- D. E. Luzzi and B. W. Smith, Carbon, 2000, 38, 1751–1756 CrossRef CAS.
- J. Sloan, R. E. Dunin-Borkowski, J. L. Hutchison, K. S. Coleman, V. Clifford Williams, J. B. Claridge, A. P. E. York, C. Xu, S. R. Bailey, G. Brown, S. Friedrichs and M. L. H. Green, Chem. Phys. Lett., 2000, 316(3–4), 191–198 CrossRef CAS.
- S. Bandow, M. Takizawa, K. Hirahara, M. Yudasaka and S. Iijima, Chem. Phys. Lett., 2001, 337, 48–54 CrossRef CAS.
- T. Okazaki, K. Suenaga, K. Hirahara, S. Bandow, S. Iijima and H. Shinohara, Phys. B Condens. Matter, 2002, 323, 97–99 CrossRef CAS.
- C. S. Allen, Y. Ito, A. W. Robertson, H. Shinohara and J. H. Warner, ACS Nano, 2011, 5, 10084–10089 CrossRef CAS PubMed.
- K. Hirahara, K. Suenaga, S. Bandow, H. Kato, T. Okazaki, H. Shinohara and S. Iijima, Phys. Rev. Lett., 2000, 85, 5384–5387 CrossRef CAS PubMed.
-
D. E. Luzzi and B. W. Smith, in Science and Application of Nanotubes, Kluwer Academic Publishers, 2005, pp. 67–76 Search PubMed.
- B. Burteaux, A. Claye, B. W. Smith, M. Monthioux, D. E. Luzzi and J. E. Fischer, Chem. Phys. Lett., 1999, 310, 21–24 CrossRef CAS.
- L. Grigorian, K. A. Williams, S. Fang, G. U. Sumanasekera, A. L. Loper, E. C. Dickey, S. J. Pennycook and P. C. Eklund, Phys. Rev. Lett., 1998, 80, 5560–5563 CrossRef CAS.
- X. Fan, E. C. Dickey, P. C. Eklund, K. A. Williams, L. Grigorian, R. Buczko, S. T. Pantelides and S. J. Pennycook, Phys. Rev. Lett., 2000, 84, 4621–4624 CrossRef CAS PubMed.
- A. M. Díez-Pascual, Macromol, 2021, 1, 64–83 Search PubMed.
- S. T. R. Naqvi, T. Rasheed, D. Hussain, M. Najam ul Haq, S. Majeed, S. shafi, N. Ahmed and R. Nawaz, J. Mol. Liq., 2020, 297, 111919 CrossRef CAS.
- R. Soleyman, S. Hirbod and M. Adeli, Biomater. Sci., 2015, 3, 695–711 RSC.
-
T. P. Dyachkova, D. V. Tarov, E. A. Burakova, E. N. Tugolukov, A. N. Blokhin, E. V. Galunin, L. V. Rosenblum and D. E. Kobzev, in IOP Conference Series: Materials Science and Engineering, IOP Publishing Ltd, 2019, vol. 693, p. 012011 Search PubMed.
- M. L. Shofner, V. N. Khabashesku and E. V. Barrera, Chem. Mater., 2006, 18, 906–913 CrossRef CAS.
- S. W. Kim, T. Kim, Y. S. Kim, H. S. Choi, H. J. Lim, S. J. Yang and C. R. Park, Carbon, 2012, 50, 3–33 CrossRef CAS.
- C. A. Mitchell, J. L. Bahr, S. Arepalli, J. M. Tour and R. Krishnamoorti, Macromolecules, 2002, 35, 8825–8830 CrossRef CAS.
- H. J. Barraza, F. Pompeo, E. A. O’Rear and D. E. Resasco, Nano Lett., 2002, 2(8), 797–802 CrossRef CAS.
- A. H. Labulo, B. S. Martincigh, B. Omondi and V. O. Nyamori, J. Mater. Sci., 2017, 52, 9225–9248 CrossRef CAS.
- B. Ye, S. I. Kim, M. Lee, M. Ezazi, H. D. Kim, G. Kwon and D. H. Lee, RSC Adv., 2020, 10, 16700–16708 RSC.
- M. Melchionna, S. Marchesan, M. Prato and P. Fornasiero, Catalysis Science and Technology, 2013,(1–3), 1–16 Search PubMed.
- J. P. Tessonnier, L. Pesant, G. Ehret, M. J. Ledoux and C. Pham-Huu, Appl. Catal. Gen., 2005, 288, 203–210 CrossRef CAS.
- J. John, E. Gravel, I. N. N. Namboothiri and E. Doris, Nanotechnol. Rev., 2012, 1, 515–539 CAS.
- N. Coustel, B. Coq, V. Brotons, P. S. Kumbhar, R. Dutartre, P. Geneste, J. M. Planeix, P. Bernier and P. M. Ajayan, J. Am. Chem. Soc., 1994, 116, 7935–7936 CrossRef.
- B. Wu, Y. Kuang, X. Zhang and J. Chen, Nano Today, 2011, 6, 75–90 CrossRef CAS.
- Q. Wang, N. Dai, J. Zheng and J. P. Zheng, J. Electroanal. Chem., 2019, 854, 113508 CrossRef CAS.
- W. Li, C. Gao, H. Qian, J. Ren and D. Yan, J. Mater. Chem., 2006, 16, 1852–1859 RSC.
- H. Lin, H. Zhu, H. Guo and L. Yu, Mater. Lett., 2007, 61, 3547–3550 CrossRef CAS.
- D. W. X. Gui, W. Ye, J. Wei, K. Wang, R. Lv, H. Zhu, F. Kang and J. Gu, J. Phys. D, 2009, 42, 75002–75005 CrossRef.
- Z. Guo, P. J. Sadler and S. C. Tsang, Adv. Mater., 1998, 10, 701–703 CrossRef CAS.
- F. Balavoine, P. Schultz, C. Richard, V. Mallouh, T. W. Ebbesen and C. Mioskowski, Angew. Chem., Int. Ed., 1999, 38, 1912–1915 CrossRef CAS PubMed.
- S. C. Tsang, Z. Guo, Y. K. Chen, M. L. H. Green, H. A. O. Hill, T. W. Hambley and P. J. Sadler, Angew. Chem., Int. Ed., 1997, 36, 2198–2200 CrossRef CAS.
- H. Maruyama and Y. Nakayama, Appl. Phys. Express, 2008, 1, 1240011–1240013 Search PubMed.
- R. J. Chen, Y. Zhang, D. Wang and H. Dai, J. Am. Chem. Soc., 2001, 123, 3838–3839 CrossRef CAS PubMed.
- H. Hu, Y. Ni, V. Montana, R. C. Haddon and V. Parpura, Nano Lett., 2004, 4, 507–511 CrossRef CAS PubMed.
- K. Besteman, J. O. Lee, F. G. M. Wiertz, H. A. Heering and C. Dekker, Nano Lett., 2003, 3, 727–730 CrossRef CAS.
- W. Wu, S. Wieckowski, G. Pastorin, M. Benincasa, C. Klumpp, J.-P. Briand, R. Gennaro, M. Prato and A. Bianco, Angew. Chem., Int. Ed., 2005, 44, 6358–6362 CrossRef CAS PubMed.
- D. Pantarotto, C. D. Partidos, R. Graff, J. Hoebeke, J. P. Briand, M. Prato and A. Bianco, J. Am. Chem. Soc., 2003, 125, 6160–6164 CrossRef CAS PubMed.
- S. Wang, E. S. Humphreys, S. Y. Chung, D. F. Delduco, S. R. Lustig, H. Wang, K. N. Parker, N. W. Rizzo, S. Subramoney, Y. M. Chiang and A. Jagota, Nat. Mater., 2003, 2, 196–200 CrossRef CAS PubMed.
- V. Zorbas, A. Ortiz-Acevedo, A. B. Dalton, M. M. Yoshida, G. R. Dieckmann, R. K. Draper, R. H. Baughman, M. Jose-Yacaman and I. H. Musselman, J. Am. Chem. Soc., 2004, 126, 7222–7227 CrossRef CAS PubMed.
- M. Shim, N. W. S. Kam, R. J. Chen, Y. Li and H. Dai, Nano Lett., 2002, 2, 285–288 CrossRef CAS.
- T. Panczyk, P. Wojton and P. Wolski, Int. J. Mol. Sci., 2020, 21(6), 1925 CrossRef CAS PubMed.
- P. Wolski, P. Wojton, K. Nieszporek and T. Panczyk, J. Phys. Chem. B, 2019, 123, 10343–10353 CrossRef CAS PubMed.
- Y. Zhang, F. Li, M. Li, X. Mao, X. Jing, X. Liu, Q. Li, J. Li, L. Wang, C. Fan and X. Zuo, J. Am. Chem. Soc., 2019, 141, 17861–17866 CrossRef CAS PubMed.
- K. M. Lee, C. P. P. Wong, T. L. Tan and C. W. Lai, Mater. Sci. Eng., B, 2018, 236–237, 61–69 CAS.
- M. Barrejón, Z. Syrgiannis, M. Burian, S. Bosi, T. Montini, P. Fornasiero, H. Amenitsch and M. Prato, ACS Appl. Mater. Interfaces, 2019, 11, 12920–12930 CrossRef PubMed.
- S. A. Jame and Z. Zhou, Nanotechnol. Rev., 2016, 5, 41–50 CAS.
- S. Y. Yang, C. D. Vecitis and H. Park, Environ. Sci. Pollut. Res., 2019, 26, 1036–1043 CrossRef CAS PubMed.
- H. Wang, K. Y. Lin, B. Jing, G. Krylova, G. E. Sigmon, P. McGinn, Y. Zhu and C. Na, Water Res., 2013, 47, 4198–4205 CrossRef CAS PubMed.
- J. Liu, X. Li, W. Jia, M. Ding, Y. Zhang and S. Ren, J. Dispersion Sci. Technol., 2016, 37, 1294–1302 CrossRef CAS.
- N. Al-Jammal, T. A. Abdullah, T. Juzsakova, B. Zsirka, I. Cretescu, V. Vágvölgyi, V. Sebestyén, C. Le Phuoc, R. T. Rasheed and E. Domokos, J. Environ. Chem. Eng., 2020, 8, 103570 CrossRef CAS.
- T. A. Abdullah, T. Juzsakova, S. A. Hafad, R. T. Rasheed, N. Al-Jammal, M. A. Mallah, A. D. Salman, P. C. Le, E. Domokos and M. Aldulaimi, Clean Technol. Environ. Policy, 2021, 1, 3 Search PubMed.
- Y. Hong, J. Zhang, C. Zhu, X. C. Zeng and J. S. Francisco, J. Mater. Chem. A, 2019, 7, 3583–3591 RSC.
- W. F. Chan, H. Y. Chen, A. Surapathi, M. G. Taylor, X. Shao, E. Marand and J. K. Johnson, ACS Nano, 2013, 7, 5308–5319 CrossRef CAS PubMed.
- Z. Z. Chowdhury, S. Sagadevan, R. Bin Johan, S. T. Shah, A. Adebesi, S. I. Md and R. F. Rafique, Mater. Res. Express, 2018, 5, 102001 CrossRef.
- R. Das, M. E. Ali, S. B. A. Hamid, S. Ramakrishna and Z. Z. Chowdhury, Desalination, 2014, 336, 97–109 CrossRef CAS.
- M. H. Davood, A. Farahani, D. Hua and T. S. Chung, J. Memb. Sci., 2018, 548, 319–331 CrossRef.
- M. Sianipar, S. H. Kim, Khoiruddin, F. Iskandar and I. G. Wenten, RSC Adv., 2017, 7, 51175–51198 RSC.
- W. A. Hamzat, A. S. Abdulkareem, M. T. Bankole, J. O. Tijani, A. S. Kovo and O. K. Abubakre, J. Environ. Sci. Health, Part A: Toxic/Hazard. Subst. Environ. Eng., 2019, 54, 827–839 CrossRef CAS PubMed.
- E. M. Elsehly, N. G. Chechenin, A. V. Makunin, H. A. Motaweh and E. G. Leksina, Water Sci. Technol., 2017, 75, 1564–1571 CrossRef CAS PubMed.
- D. Budimirović, Z. S. Veličković, Z. Bajić, D. L. Milošević, J. B. Nikolić, S. Drmanić and A. D. Marinković, J. Serb. Chem. Soc., 2017, 82, 1175–1191 CrossRef.
- M. M. A. Aslam, H. W. Kuo, W. Den, M. Usman, M. Sultan and H. Ashraf, Sustain, 2021, 13, 5717 CrossRef.
- A. A. Atiyah, A. J. Haider and R. M. Dhahi, IET Nanobiotechnol., 2019, 13, 597–601 CrossRef PubMed.
- S. M. Al-Hakami, A. B. Khalil, T. Laoui and M. A. Atieh, Bioinorg. Chem. Appl., 2013, 2013, 458943 Search PubMed.
- M. Karimi, N. Solati, M. Amiri, H. Mirshekari, E. Mohamed, M. Taheri, M. Hashemkhani, A. Saeidi, M. A. Estiar, P. Kiani, A. Ghasemi, S. M. M. Basri, A. R. Aref and M. R. Hamblin, Expert Opin. Drug Deliv., 2015, 12, 1071–1087 CrossRef CAS PubMed.
- S. Sharma, N. K. Mehra, K. Jain and N. K. Jain, Artif. Cells, Nanomed. Biotechnol., 2016, 44, 1851–1860 CrossRef CAS PubMed.
- S. Prakash, M. Malhotra, W. Shao, C. Tomaro-Duchesneau and S. Abbasi, Adv. Drug Deliv. Rev., 2011, 63, 1340–1351 CrossRef CAS PubMed.
- C. L. Lay, J. Liu and Y. Liu, Expet Rev. Med. Dev., 2011, 8, 561–566 CrossRef CAS PubMed.
- J. M. Tan, P. Arulselvan, S. Fakurazi, H. Ithnin and M. Z. Hussein, J. Nanomater., 2014, 2014, 917024 Search PubMed.
- S. Paliwal, K. Pandey, S. Pawar, H. Joshi and N. Bisht, Indian J. Pharm. Sci., 2020, 82, 766–772 CAS.
- M. Lotfi, A. Morsali and M. R. Bozorgmehr, Appl. Surf. Sci., 2018, 462, 720–729 CrossRef CAS.
- M. Kamel, H. Raissi, A. Morsali and M. Shahabi, Appl. Surf. Sci., 2018, 434, 492–503 CrossRef CAS.
- A. A. Bhirde, S. Patel, A. A. Sousa, V. Patel, A. A. Molinolo, Y. Ji, R. D. Leapman, J. S. Gutkind and J. F. Rusling, Nanomedicine, 2010, 5, 1535–1546 CrossRef CAS PubMed.
- J. Jampilek and K. Kralova, Materials, 2021, 14, 1–39 CrossRef PubMed.
- A. Bianco, K. Kostarelos and M. Prato, Chem. Commun., 2011, 47, 10182–10188 RSC.
- R. Das, S. B. Abd Hamid, M. E. Ali, M. S. M. Annuar, E. M. B. Samsudin and S. Bagheri, Sci. Adv. Mater., 2015, 7, 2726–2737 CrossRef CAS.
- P. C. Ma, N. A. Siddiqui, G. Marom and J. K. Kim, Compos. Appl. Sci. Manuf., 2010, 41, 1345–1367 CrossRef.
- N. J. Singhai and S. Ramteke, Curr. Nanosci., 2020, 16, 170–186 CrossRef CAS.
- Y. Hwang, S. H. Park and J. W. Lee, Polymers, 2017, 9, 13 CrossRef PubMed.
- Y. Zhang, Y. Bai and B. Yan, Drug Discov. Today, 2010, 15, 428–435 CrossRef CAS PubMed.
- A. V. Liopo, M. P. Stewart, J. Hudson, J. M. Tour and T. C. Pappas, J. Nanosci. Nanotechnol., 2006, 6, 1365–1374 CrossRef CAS PubMed.
- G. Pastorin, Pharm. Res., 2009, 26, 746–769 CrossRef CAS PubMed.
- C. Ménard-Moyon, E. Venturelli, C. Fabbro, C. Samorì, T. Da Ros, K. Kostarelos, M. Prato and A. Bianco, Expet Opin. Drug Discov., 2010, 5, 691–707 CrossRef PubMed.
- N. D. Koromilas, G. C. Lainioti, C. Gialeli, D. Barbouri, K. B. Kouravelou, N. K. Karamanos, G. A. Voyiatzis and J. K. Kallitsis, PLoS One, 2014, 9, 107029 CrossRef PubMed.
- M. Allegri, D. K. Perivoliotis, M. G. Bianchi, M. Chiu, A. Pagliaro, M. A. Koklioti, A. F. A. Trompeta, E. Bergamaschi, O. Bussolati and C. A. Charitidis, Toxicol. Rep., 2016, 3, 230–243 CrossRef CAS PubMed.
- D. F. Rodrigues, D. P. Jaisi and M. Elimelech, Environ. Sci. Technol., 2013, 47, 625–633 CrossRef CAS PubMed.
- E. Mohammadi, M. Zeinali, M. Mohammadi-Sardoo, M. Iranpour, B. Behnam and A. Mandegary, Hum. Exp. Toxicol., 2020, 39, 1147–1167 CrossRef CAS PubMed.
- C. L. Ursini, D. Cavallo, A. M. Fresegna, A. Ciervo, R. Maiello, S. Casciardi, F. Tombolini, G. Buresti and S. Iavicoli, J. Nanomater., 2012, 2012, 815979 Search PubMed.
- T. Coccini, E. Roda, D. A. Sarigiannis, P. Mustarelli, E. Quartarone, A. Profumo and L. Manzo, Toxicology, 2010, 269, 41–53 CrossRef CAS PubMed.
- G. Song, X. Guo, X. Zong, L. Du, J. Zhao, C. Lai and X. Jin, Dent. Mater. J., 2019, 38, 127–135 CrossRef CAS PubMed.
|
This journal is © The Royal Society of Chemistry 2021 |
Click here to see how this site uses Cookies. View our privacy policy here.