DOI:
10.1039/C2RA22351A
(Review Article)
RSC Adv., 2013,
3, 4473-4491
Recent advances in electrochemical glucose biosensors: a review
Received 29th September 2012, Accepted 6th December 2012
First published on 7th December 2012
Abstract
Glucose detection is of great significance in biomedical applications. Principles, methods and recent developments in electrochemical glucose sensors are reviewed here. Special attention is given to the discussion on some problems and bottlenecks in areas of nonenzymatic and enzymatic (glucose oxidase-based) amperometric glucose sensing.
1. Introduction
Glucose detection is of great importance in a variety of fields ranging from biomedical applications to ecological approaches.1 In clinical medicine, diabetes mellitus is one of the leading causes of death and disability in the world. This metabolic disorder results from insulin deficiency and hyperglycemia is reflected by blood glucose concentrations higher than the normal range of about 3.9–6.2 (empty stomach) or 3.9–7.8 (2 h after food) mM. The quantitative monitoring of blood glucose is of great clinical importance, which can greatly reduce the risks of diabetes mellitus-induced heart disease, kidney failure, or blindness.2,3 About 9,000 peer-reviewed articles relevant to glucose sensors have been recorded in the ISI web of knowledge. As shown in Fig. 1, the number of glucose sensor-relevant articles has maintained an increasing trend over the last 10 years. Electrochemical and optical methods have been extensively developed to monitor glucose. Obviously, photons are measured in optical methods, while electrons are measured in electrochemistry. Hence, the wireless characteristic of optical methods makes them very convenient for bioimaging and in vivo biosensing. Many optical methods, such as absorptiometry (and reflectometry), fluorescence, and surface plasmon resonance (SPR), are highly effective for glucose sensing, the readers may read relevant papers and reviews for details.4–11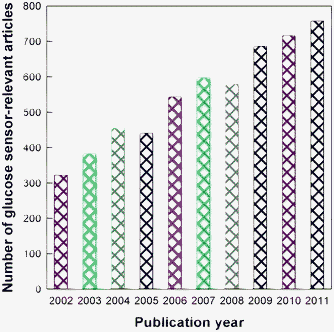 |
| Fig. 1 The numbers of glucose sensor-relevant articles published in the last 10 years (data collected from ISI web of knowledge). | |
Electrochemical methods, especially amperometric methods, have been widely utilized in glucose sensing. The nonenzymatic sensing and the enzymatic biosensing of glucose are two main categories of glucose sensing which have been widely investigated and utilized. Amperometric nonenzymatic glucose sensors based on the direct electrochemical oxidation of glucose have triggered great interest and have been widely exploited. The greatest advantage of amperometric nonenzymatic glucose sensing over enzymatic biosensing is that the former has addressed the problem of insufficient long-term stability, which is the most common and serious problem for enzymatic glucose sensors originating from the intrinsic nature of enzymes. A lot of metal materials, such as noble metals (e.g. Au and Pt) and their composites, have been widely exploited as electrode materials in nonenzymatic glucose sensing owing to their high electrocatalytic ability, high sensitivity and good selectivity to the electro-oxidation of glucose.12–20 The history of nonenzymatic glucose sensing can be traced back to the direct electro-oxidation of glucose to gluconic acid in a sulfuric acid solution at a lead anode reported by Walther Loeb in 1909. Studies of direct electro-oxidation21 and electroreduction22 of glucose in alkaline (pH > 11) and acidic (pH < 1) solutions continue to date.1 Nevertheless, the adsorption of oxidation intermediates of glucose (e.g. CO) or active species in the solution (e.g. Cl−) may notably block the electrode activity for direct electro-oxidation of glucose, which is one of the most serious problems for nonenzymatic glucose sensors.23,24 In addition, the amperometric nonenzymatic glucose sensors usually offer analytical selectivity inferior to the amperometric enzymatic glucose sensors because it is not easy for these electrocatalytic materials to be as specific as enzymes to catalyze the glucose oxidation.
Glucose oxidase (GOx)-based amperometric enzyme electrodes play a key role in the move to simple, easy-to-use blood glucose monitoring, since GOx is relatively low in price as well as having high bioactivity and stability.25 The entire field of enzyme electrodes can trace its origin back to the enzyme electrode fabricated by Clark and Lyons in 1962. Clark's original patent of amperometric enzyme electrode (Clark, L., Jr., U.S. Patent 33,539,455, 1970) covers the conversion of electroinactive substrates to electroactive products with the utilization of enzymes. Biosensors taking enzymes as their molecular recognition components are endowed with many advantages, such as high selectivity and sensitivity. With the rapid development of biology, chemistry, physics, medicine, and electronic technology over the years, enzyme-based biosensing is becoming one of the most active research areas in analytical chemistry due to its incomparable advantages over other techniques. Biosensors have been extensively studied since the work of Clark, and today glucose biosensors account for about 85% of the entire biosensor market in the world, due mainly to the notable biomedical significance of the rapid and convenient assay of blood glucose.26 The huge market size of glucose biosensors makes diabetes a model disease for developing new biosensing concepts. Important reviews on electrochemical glucose biosensing have been published recently. For instance, Wang has reviewed the principles of operation, history, recent developments, and current status of electrochemical glucose biosensors.26 Heller et al. have reviewed the electrochemical glucose sensors and their applications in diabetes management.1
Glucose dehydrogenase (GDH) is another kind of enzyme used in glucose biosensing and is also utilized in fabricating commercial test strips for blood glucose.27–30 GDH-based amperometric biosensors are advantageous in being able to be operated at lower detection potentials than the first-generation GOx-based sensors, and their performance is not influenced by the oxygen level in the analyte solution.31 Pyrroloquinoline quinone (PQQ)-dependent GDH (PQQGDH) and β-nicotinamide adenine dinucleotide (NAD)-dependent GDH are the two main types of GDH that have been applied in biosensors. PQQGDH is no longer looked upon as a candidate for physiological glucose determination because of the requirement of suitable detergents for solubilization and purification for membrane-bound PQQGDH, as well as the low selectivity and poor thermal stability of water-soluble PQQGDH.30 For NAD-dependent GDH-based amperometric biosensors, the need for the addition of the soluble NAD cofactor complicates the analysis system to some extent.25,31 Moreover, the electrochemistry of both the oxidized form (NAD+) and the reduced form (NADH) of NAD cofactor suffer from their irreversible characteristic, and the direct oxidation of NADH at unmodified electrodes requires a high overpotential owing to its sluggish electron-transfer kinetics. In addition, the common requirement of artificial electron acceptors for electrochemical measurements also greatly restricts the wide application of GDH.32 All of these drawbacks result in less popularity of GDH compared with GOx utilized in glucose biosensing.33
Isoenzyme 2 of hexokinase functions in sugar sensing and glucose repression in Saccharomyces cerevisiae.34 Hexokinase-based glucose biosensing has been recognized as a reference method for blood glucose determination because of its ultrahigh specificity. Hexokinase is highly recommended to be used in automated analyzer and emergency tests and the relevant kinetic mechanisms have been extensively examined by researchers.34–36 But hexokinase was not so widely used in the research of glucose biosensing as GOx, probably because of its relatively higher price, lower stability, and the need for ATP in its enzymatic reaction.
A number of studies have also been carried out to find a less invasive means to monitor blood glucose levels.37–39 Mid-infrared emission spectroscopy,40 the metabolic heat conformation method,41 and a GlucoWatch design based on electro-osmotic flow of subcutaneous fluid to the surface of the skin and detection of glucose with an enzyme electrode,42 have been exploited to monitor blood glucose non-invasively or minimally invasively. It has also been suggested that tear fluid can serve as a substitute for blood and non-invasive glucose monitoring has the potential to revolutionize diagnosis of diabetes.43,44 The development in non-invasive glucose monitoring has been reviewed in 2008 and it is expected to make greater progress in the future.39
Many reviews have been published on glucose sensing, but some important aspects on this topic have not been fully understood or not attracted enough attention. The great scientific and clinical importance of glucose sensors and the fast development of core and peripheral technologies also require continuous updating in their research statuses. This review mainly discusses the principles of electrochemical glucose sensors, including nonenzymatic glucose determination and GOx-based enzymatic glucose biosensing, their recent developments and current status, the major strategies for enhancing their performance, as well as the key challenges and opportunities in their further development and applications. Given the long history and broad field of electrochemical glucose biosensors, the authors apologize for the inevitable oversights of some important contributions.
2. Nonenzymatic electrochemical glucose sensors
The nonenzymatic sensing of glucose based on the direct electrochemistry of glucose (oxidation or reduction) is a rapid and cost-effective approach. Noble metals such as Pt15,17,45 and Au46,47 and their composites14,48,49 were usually chosen to develop nonenzymatic sensors in early research studies. Recently, Park et al. and Toghill et al. reviewed electrochemical nonenzymatic glucose sensing.50,51Three major problems exist in the direct oxidation of glucose on a conventional noble metal electrode: (1) the sensitivity of glucose sensing is restricted by the relatively sluggish kinetics of glucose electro-oxidation on conventional electrodes; (2) the activity of noble metal electrodes is often impaired by the irreversibly adsorbed oxidation intermediates of glucose and the adsorbed chloride ions (in our opinion, a permselective film against anionic Cl− will help here); and (3) the selectivity of nonenzymatic glucose sensors is relatively poor, since some other sugars and some endogenous interfering species can also be oxidized in the potential range of glucose oxidation.18,48,52 A high real surface area (with high roughness factor) of an electrode is strongly expected to aid highly sensitive and selective electro-oxidation of glucose because the adsorption of glucose on the electrode surface is a prerequisite step, and the electro-oxidation of the interfering electroactive species of ascorbic acid, uric acid, and p-acetamedophenol is independent of the electrode roughness because it is diffusion-controlled. Nowadays, many attempts have been made to develop various nanomaterials with distinguished characteristics to provide new opportunities for fabricating novel nonenzymatic glucose sensors. But the adsorption of chloride is very significant on the rough electrode surface. Alkaline conditions were demonstrated to be effective in eliminating the effect of chloride because of the pre-occupation of the OH group on the electrode surface.53
As is widely accepted, the catalytic process of nonenzymatic glucose oxidation includes the process of hemiacetalic hydrogen atom abstraction occurring simultaneously with the adsorption of the organic species, which is considered as the rate determining step in the catalytic process of glucose electro-oxidation. An “incipient hydrous oxide adatom mediator” (IHOAM) model was proposed by Burke and the hydroxide premonolayer formation was demonstrated to be one of the key points for the electrocatalytic process of glucose.54 The importance of the “active” hydroxide anions in the vicinity of the electrode surface produced by the dissociation of water (shown in eqn (1)) to the electro-oxidation of glucose and many other organic molecules is well known.45,51 The oxidative adsorbed hydroxide radical expressed as MOHads is formed by the chemisorption of hydroxide anions to the reductive metal adsorption site (expressed as M as shown in eqn (2)) and is believed to be the catalytic component of electrocatalysts for glucose.
It is believed that the chemisorbed MOHads takes part in the slow step of glucose oxidation. As is seen in the above equations, the increase in the OH− concentration definitely promotes the formation of MOHads.49 So nonenzymatic glucose sensing is a pH-dependent reaction and an alkaline environment is beneficial to it, which is the reason why a higher sensitivity of nonenzymatic glucose sensing is commonly observed in a higher pH environment.
Various nanomaterials, such as palladium nanoparticles supported on functional carbon nanotubes,55 Ti/TiO2 nanotube array/Ni composites,56 boron-doped diamond nanorods,18 electrospun palladium (IV)-doped copper oxide composites nanofibers,57 three-dimensionally ordered macroporous platinum templates,49 polycrystalline Pt electrodes,45 nanoporous Au,58 Cu nanoclusters/multiwalled carbon nanotubes (MWCNTs) composites,59 and highly dispersed Ni nanoparticles embedded in a graphite-like carbon film electrode,60 were developed as excellent electrocatalysts for nonenzymatic glucose sensing in alkaline media. Porous tubular palladium nanostructures was also applied to nonenzymatic glucose sensing in 0.10 M pH 8.1 PBS.61 It was also reported that the nonenzymatic electro-oxidation of glucose is greatly enhanced at Ni and Cu compared with Pt and Au electrodes as a result of their electrocatalytic effect mediated by surface-bound Ni2+/Ni3+ and Cu2+/Cu3+ redox couples.62,63 All the above nanomaterials exhibit sensitive and selective responses to glucose electro-oxidation in alkaline solutions, in which the existence of abundant OH− can largely promote the formation of MOHads and greatly reduce the adsorption of Cl−. As can be seen, various Pt, Au, Cu, Ni, Pd, Ti, TiO2, and carbon-based nanomaterials can be utilized for nonenzymatic glucose sensing in alkaline media because of the stability and excellent catalysis of these materials or their oxides/hydroxides under high pH conditions.
However, the alkaline environment may cause surface degradation of the electrocatalysts and thus limit their lifetime. In addition, the efficiency of nonenzymatic glucose sensors is usually characterized in neutral physiological media but the physiological concentration of Cl− can greatly suppress glucose adsorption in neutral media, and the physiological pH also significantly affects the electrocatalytic activity of the metal electrode to the oxidation of glucose. Various other nanomaterials have also been reported to be effective in detecting glucose in neutral media. For example, highly ordered Pt nanotube array electrodes were demonstrated to be sensitive, selective, and stable enough for nonenzymatic glucose sensoring.64 Park et al. reported enzyme-free glucose sensing using mesoporous Pt,17 and the mesoporous surface endowed the sensor with an excellent anti-poison ability, which retained sufficient sensitivity in the presence of excessive chloride ions (0.10 M KCl). The nanoporous PtPb networks14 synthesized by Wang et al. showed high sensitivity, high selectivity and excellent resistance towards poisoning by Cl−. It was also reported by Sun et al. that glucose can be selectively electro-oxidized on Pt–Pb alloy (Pt2Pb) electrodes at remarkably more negative potentials compared with pure Pt surfaces, and more stable and larger responses can be obtained on Pt2Pb because of its high surface roughness factor and particular nanostructure.20 Gao et al. developed a facile one-step ultrasonication-assisted electrochemical method to synthesize nanocomposites of graphene and PtNi alloy nanoparticles and demonstrated their use for highly selective and sensitive nonenzymatic glucose detection.48 Platinum, copper sulfide, and tin oxide nanoparticles-carbon nanotubes (CNTs) hybrid nanostructures exhibited excellent sensitivity, selectivity, and stability in nonenzymatic glucose sensing.15 Palladium-single-walled carbon nanotube hybrid nanostructures showed good electrocatalytic activity toward the oxidation of glucose in neutral media even in the presence of a high concentration of chloride ions.16 Copper micropuzzles, which are high-quality Cu microplates that undergo in situ large-scale assembly into puzzle-like patterns, were synthesized with the assistance of glucose and applied as a nonenzymatic glucose sensor exhibiting a good sensitivity and a wide range of detection concentrations for glucose at −0.67 V in pH 7.40 phosphate buffer solution (PBS).65 Xie et al. reported the preparation of Au-film electrodes in a glucose-containing Au-electroplating aqueous bath for a high-performance nonenzymatic glucose sensor and glucose/O2 fuel cell.66 Relatively active metals (e.g. Cu) can be electrochemically dissolved unless potentiostated at very low potentials, thus their applications in nonenzymatic glucose sensing at high potentials are limited in neutral media. Au nanomaterials are widely exploited as artificial enzymes because of their excellent stability and excellent catalytic effects. Gold nanoparticles (AuNPs) prepared via citrate reduction exhibit intrinsic GOx-like activity.67 Small Au nanoclusters (3.6 nm) were found to have intrinsic GOx-like catalytic activity, which can catalyze the oxidation of glucose with the cosubstrate O2 similar to that of the natural enzyme of GOx, with the production of gluconate and hydrogen peroxide.68 Luo et al. also reported the self-catalyzed, self-limiting growth of GOx-mimicking AuNPs and found that the H2O2 generated from AuNPs-catalyzed glucose oxidation can induce the AuNPs' seeded growth in the presence of HAuCl4.69 The artificial enzymes here catalyze glucose oxidation in an oxygen-containing neutral solution and electricity is not required, and are quite different to the above nonenzymatic electrocatalysts for glucose electro-oxidation. The artificial enzymes here may lead to future amperometric glucose sensors integrating both enzyme-mimic catalysis and electrocatalysis for improved readouts.
3. Three generations of enzyme-based amperometric glucose biosensors
GOx is widely utilized in the majority of commercially available glucose sensors because of its low cost and high selectivity and sensitivity. Since Clark and Lyons proposed the initial concept of glucose enzyme electrodes in 1962,70 tremendous efforts have been made towards the improvement of GOx-based amperometric biosensors for blood glucose determination.71 The first glucose enzyme electrode fabricated by Clark and Lyons took a thin layer of GOx entrapped over an oxygen electrode via a semipermeable dialysis membrane to catalyze glucose oxidation in the presence of O2 as shown in eqn (3), with the O2 consumption detected by a Pt cathode according to eqn (4) and used as the signal for glucose determination. |  | (3) |
The accuracy and precision of a GOx electrode based on O2 consumption were greatly reduced by the variation in background oxygen in the samples. Updike and Hicks provided a smart solution to this problem by using two oxygen working electrodes (one covered with the enzyme) and measuring the current differences.72 The amperometric monitoring of the H2O2 product in GOx-based glucose determination was firstly proposed in 1973 by Guilbault and Lubrano73 and various amperometric enzyme electrodes have since been described.
The biocatalytic reaction of GOx-based glucose biosensing involves the reduction of the flavin group in the enzyme (GOx(FAD)) by reacting with glucose to give the reduced form of the enzyme (GOx(FADH2)) (as shown in eqn (5)), followed by the reoxidation of GOx(FADH2) by the electron acceptor (Medox) to regenerate the oxidized form of the enzyme (GOx(FAD)), as shown in eqn (6). The regeneration of the original state of GOx(FAD) in the enzymatic cycle is vital, otherwise one enzyme molecule can take effect only once and the next enzymatic reaction cycle will cease.
| GOx(FAD) + glucose → GOx(FADH2) + gluconic acid | (5) |
| GOx(FADH2) + Medox → GOx(FAD) + Medred | (6) |
According to the nature of Medox, amperometric glucose biosensors can be classified into the three following generations. The physiological mediator O2, the artificial (synthetic) electron acceptor, and the electrode potentiostated at a potential positive of the formal potential of GOx, are used as the Medox to regenerate GOx(FAD) in the first-, second-, and third-generation amperometric glucose biosensors, respectively, as shown in Fig. 2.
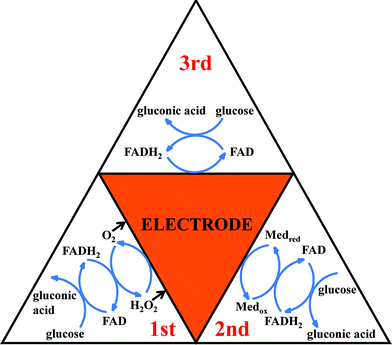 |
| Fig. 2 Summary of enzymatic glucose oxidation mechanisms, presented as the first, second and third generation sensors.51 Redrawn with permission from ref. 51, copyright 2010 by ESG (http://www.electrochemsci.org). Note that glucose assay at a first-generation amperometric enzyme electrode can be conducted in the three following amperometric signaling modes, i.e., cathodic detection of O2 and H2O2 as well as anodic detection of H2O2, and the anodic detection of H2O2 to regenerate/replenish O2 is obviously more favorable for enhancing the enzymatic reaction cycle (versus the other two modes). | |
3.1. First-generation amperometric glucose biosensors
The first-generation amperometric glucose biosensors take the physiological mediator O2 as the Medox to regenerate GOx(FAD) and detect glucose based on monitoring the consumption of O2 or the generation of H2O2 in the process of the enzymatic reaction. O2 is the physiological electron acceptor of GOx and thus the electron communication must be very fast. While electrochemical reduction of O2 is usually used to monitor the O2 consumption for glucose quantification, both anodic oxidation and cathodic reduction of H2O2 can be employed to monitor the enzymatic generation of H2O2, and the anodic oxidation of H2O2 can favorably regenerate/replenish O2 for improving the enzymatic cycling. The first-generation biosensing mode based on the measurement of O2 consumption or H2O2 formation has the advantage of being simple, stable, and being able to be used in miniaturized devices.The response of first-generation glucose sensors is directly related to the O2 concentration in solution, thus oxygen tension plays an important role in the determination. The fact that normal O2 concentrations are about 1 order of magnitude lower than the physiological level of glucose is known as “oxygen deficit”. The upper limit of linearity of first-generation amperometric glucose biosensors is greatly reduced by the “oxygen deficit” and their sensitivity to glucose is also restricted by the O2 concentration in solution. Attempts have been made to conquer these drawbacks of O2-dependent amperometric glucose biosensors. The use of a mass transport-limiting film to increase the O2/glucose permeability ratio was proposed to address the “oxygen deficit”, and the two dimensional cylindrical electrode designed by Gough's group is a successful example.74–76 Scientists also designed oxygen-rich carbon paste enzyme electrodes or constructed an air diffusion biocathode using O2 directly from air to improve the O2 supply to conquer the O2 limitation.77–79 Other materials that can effectively enrich O2 are also expected to be utilized in the first-generation amperometric glucose biosensors to conquer the O2-limit problem.
An anti-interferent ability is one of the prerequisites for an effective biosensor. A lot of coexisting oxidizable species in biological fluids, such as ascorbic acid, uric acids, and some drugs (e.g. acetaminophen), can be co-oxidized at the relatively high potential used for H2O2 electro-oxidation, so they all contribute to the current response and thus compromise the selectivity and the accuracy of the glucose measurement. Considerable efforts have been devoted to improve the anti-interferent ability of first-generation amperometric glucose biosensors. As reported, there are mainly two effective protocols in minimizing interferences: (1) immobilizing the enzyme through a permselective film, which can diminish or inhibit the electroactivity of interferents but can allow the sufficiently high electroactivity of H2O2 or O2 at the enzyme electrode;80,81 (2) decreasing the H2O2-detecting potential by using catalysts immobilized at the enzyme electrode.82 Prussian blue (PB), which is called “artificial peroxidase” because of its high selectivity and catalytic activity for the reduction of H2O2, has been widely utilized in the highly selective biosensing of glucose at low potentials. For instance, Zhao et al. prepared poly(diallyldimethylammonium chloride) (PDDA)-protected PB nanoparticles to catalyze the enzymatically generated H2O2 at low potentials and inhibit the responses of interferents.83 Li et al. fabricated a highly sensitive molecularly imprinted electrochemical sensor based on the double amplification by an inorganic PB catalytic polymer and the enzymatic effect of GOx.84 The incorporation of GOx into Langmuir–Blodgett films based on PB was also applied to an amperometric glucose biosensor operated at very low potential.85 We realized the sensitive determination of glucose in both H2O2-oxidation and H2O2-reduction modes on a PB modified Au electrode with GOx immobilized.86 Electropolymerized poly(toluidine blue O) film was also effectively used as the redox mediator that contributes to the low potential detection of glucose at a carbon nanotube modified glassy carbon electrode.87 Various nanomaterials, such as CNTs,88 platinum nanoparticles,89,90 metallized carbons,91 and composite nanomaterials,92,93 have also been demonstrated to be effective in improving the selectivity of resultant GOx-based amperometric biosensors by lowering the determination potential of H2O2 by virtue of the excellent catalytic effect of nanomaterials.26 In addition, some biological enzymes for H2O2 (e.g. horseradish peroxidase (HRP)) have been co-immobilized to develop bienzymatic amperometric electrodes for cathodic detection of H2O2. For instance, the co-immobilization of HRP to catalyze the reduction of GOx-generated H2O2 at low potentials is effective in improving the selectivity of glucose sensors.94,95 Furthermore, a higher degree of technical sophistication in the amperometric detection device can efficiently improve the selectivity for the glucose assay, e.g. introduction of an interferent-pretreatment unit to electrochemically remove the interfering species before they reach the biosensor surface.96–98
3.2. Second-generation amperometric glucose biosensors
The 10-fold excess of glucose over oxygen in blood makes the “oxygen deficit” the most serious problem for the first-generation amperometric biosensors.99 A highly successful approach to increase the electron-transfer rate of biosensors is the use of another artificial Medox to mediate the GOx cycling instead of oxygen.26 The artificial Medox can be either a solution-state mediator that can diffuse into and out of the enzyme active site,99 or an immobilized mediator by attaching it directly to the enzyme, entrapping it in the enzyme film, or using a redox-conducting polymer that can shuttle its electrons to and from the enzymatic active site.100,101 Biosensors using artificial electron acceptors to shuttle electrons from the redox center of the enzyme to the surface of the electrode are called second-generation biosensors.102–104 The effective mediators for GOx include ferrocene derivatives, conducting organic salts (particularly tetrathiafulvalene-tetracyanoquinodimethane, TTF-TCNQ), ferricyanide, quinone compounds, transition-metal complexes, and phenothiazine and phenoxazine compounds.1,26,89,105–107 Interestingly, ferricyanide is not recommended as a highly efficient electron mediator for GOx in some reports, although the molecular mechanism remains unknown at present.106,108The catalytic process of second-generation amperometric glucose biosensors includes the following three steps: (a) the transfer of electrons (and protons) from glucose to the two FAD reaction centers of GOx, which are reduced to FADH2, (b) the transfer of electrons from the FADH2 centers to the artificial mediators, and thus the mediators transform from Medox to their reduced state Medred, and (c) the transport of electrons through the artificial mediators to the electrode. The current signals produced by the oxidation of Medred are used for glucose determination in the second-generation biosensing mode. So the effective interaction between mediators and enzymes, which is necessary for the realization of the effective shuttling of electrons between redox active centers of GOx and electrode, is essential in the second-generation biosensing mode. Diffusion mediators satisfy well this demand. However, the use of soluble mediating species cannot be used in implantable probes.109,110 Various strategies have been suggested for tailoring the mediators in the electrode-supported enzyme film. Chemically binding the mediators with the polymer backbone used for fabricating the biosensor has been widely utilized to stabilize artificial mediators.109,111 Hale et al. have investigated some systems where the mediating species were chemically bound to the polysiloxane to allow the close contact between the FAD/FADH2 centers of the enzyme and the mediator, yet prevented the latter from diffusing away from the electrode surface.109 Dong's group reported supramolecular organized multilayers constructed by MWCNTs modified with ferrocene derivatized poly(allylamine) redox polymer and GOx by electrostatic self-assembly and thus achieved the construction of a reagentless biosensor.111 Hydrogels containing Os complexes were also used to fabricate second-generation amperometric biosensors with wide use in fundamental science and important practical applications.112,113 An electron-conducting crosslinked polyaniline (PANI)-based redox hydrogel, formed in one step at pH 7.2, was used to electrically wire the GOx and form an effective glucose electro-oxidation catalyst, and the electro-oxidation of glucose at 0.3 V vs. Ag/AgCl was thus realized.114 Willner's group fabricated the integrated electrically contacted CNTs/ferrocene/GOx electrodes and used them for the bioelectrocatalyzed detection of glucose.115 Hydroquinonesulfonate ions were co-immobilized in polypyrrole films with GOx to realize glucose sensing in the absence of mediator in solution.110 The attachment of redox mediators or relays directly to the enzymes is also widely reported. Schuhmann et al. reported the electron-transfer between GOx and electrodes via redox mediators bound with flexible chains to the enzyme surface.101 Scientists have also tried to covalently link the ferrocene derivative to the enzyme molecule, but this was shown to be more complicated and less successful.101 In contrast, Sekretaryova et al. reported the successful stable immobilization of both the enzyme and the mediator, avoiding covalent linking of the latter, by exposing the enzymes to water–organic mixtures with a high content of organic solvent.99 In our opinion, in contrast to a second-generation amperometric enzyme electrode involving a solution-state (diffusion-based) artificial mediator, the immobilized mediator case should provide a compromise between the three simultaneously-occurring but somewhat mutually contradictory events below, i.e., the stable immobilization of the mediator (limited movement), the high electron/proton-exchange efficiency between the immobilized mediator and the immobilized enzyme, and the high electron-exchange efficiency between the immobilized mediator and the electrode surface. Hence, immobilization of the artificial mediator both near the enzyme's redox center and near the electrode surface is very important for the fast mass-/electron-transfer between the mediator, the enzyme, and the electrode, otherwise, the enzyme-mediating efficiency and/or the amperometric signaling efficiency will decrease or even fully die away.
3.3. Third-generation amperometric glucose biosensors
The third-generation amperometric glucose biosensors are fascinating because they function in the ideal biosensing model in the absence of mediators. The direct electrical communication of GOx can also contribute to the detection of glucose at low potentials slightly positive of the redox potential of GOx (around −0.50 V vs. Ag/AgCl).26,116 Achieving the direct electron communication of enzymes depends significantly on the distance between the redox-active cofactor and the electrode surface.117,118 Various attempts to overcome the long electron-tunneling distance were made to realize the direct electrochemistry of enzymes. The reconstitution of apo-proteins on cofactor-modified electrodes and the reconstitution of apo-enzymes on cofactor functionalized Au nanoparticles were extensively employed as a versatile method to align redox enzymes on electrodes.29,119–124 Whereas this method is effective in electrically wiring redox enzymes with electrodes, the complicated procedures inhibit its wide use in practice. Yehezkeli et al. reported a method to electrically wire the enzyme and transform it from an oxidase to a hydrogenase by biocatalytically implanting Pt nanoclusters into GOx via thermodynamically reducing different metal salts to metallic nanoclusters with the reduced cofactor FADH2.125 Various nanomaterials have also been reported to achieve the direct electrochemistry of GOx.126,127 Shan et al. reported the direct electrochemistry of GOx and glucose biosensing based on polyvinylpyrrolidone-protected graphene.126 Alwarappan et al. carried out a series of works to employ graphene-GOx for glucose detection, and the promotion of glucose biosensing and the direct electrochemistry of GOx have been realized with the aid of graphene.117,118,128 Wang et al. detected glucose based on the direct electron transfer reaction of GOx immobilized on highly ordered PANI nanotubes.97 Amine-terminated ionic liquid functionalized CNTs-AuNPs were developed by Gao et al. to investigate the direct electron transfer of GOx.129 Holland et al. enabled the direct electrical communication between GOx and electrode through a simple site-specific modification of GOx to display a free thiol group near its active site and thus facilitated the site-specific attachment of a maleimide modified gold nanoparticle to the enzyme.130Although well-defined voltammetric peaks of direct electrochemistry of GOx have been achieved in a lot of studies, the detection of glucose based on the direct electron transfer of GOx has been rarely realized.97 Most GOx exhibiting good direct electrochemical peaks of GOx still need mediators to catalyze the oxidation of glucose.131,132 In fact, some reports on GOx electrochemistry do not clearly demonstrate that the direct electrochemistry comes from the intact (or still sufficiently bioactive) enzyme, thus any claims on the third-generation amperometric biosensing of glucose should be made very carefully. In our opinion (as also reminded by one of the referees of this article),133 the observed well-defined voltammetric peaks of direct electrochemistry of GOx probably come from the enzyme molecules whose activity has been greatly decreased because of the destruction of enzyme conformation or the release of flavin. With the use of the electrochemical quartz crystal microbalance (EQCM) technique, we measured the electroactivity of sodium dodecyl benzene sulfonate (SDBS)-treated GOx adsorbed on a MWCNTs/Au electrode as a function of the enzymatic specific activity (defined as the enzymatic activity per unit mass of enzyme) of the adsorbed GOx. We thus experimentally found for the first time that the electroactivity and the enzymatic specific activity of the immobilized GOx responded oppositely in the presence of MWCNTs and SDBS, and the portion of the adsorbed GOx showing electrochemical activity exhibited almost no enzymatic activity.133 A similar conclusion has also been experimentally drawn by Wang and Yao.134 Obviously, the significance of realizing such a direct electrochemistry of partially or fully denatured GOx for glucose detection is worthy of reconsideration. It must be emphasized that the determination of glucose based on electroreduction of enzyme-consuming O2 at low potentials (close to the redox potential of GOx) should conceptually belong to the first-generation amperometric glucose biosensors, rather than the third-generation ones. Instead, a distinguished decrease in the reduction current and an increase in the oxidation current of direct electrochemistry of GOx should be simultaneously observed in the presence of glucose (versus its absence) for a successful third-generation GOx-based glucose biosensor. Hence, a third-generation GOx-based glucose biosensor can efficiently work at potentials slightly positive of the redox potential of GOx around −0.50 V vs. Ag/AgCl26,116 (e.g., at −0.30 V vs. Ag/AgCl). Generally, the direct electron communication between the deeply buried redox active center of the enzyme and the electrode surface requires the conformational change of the enzyme, which may result in an obvious loss of enzymatic activity. An appropriate balance between the enzymatic and electrochemical activities is vital for the third-generation amperometric glucose biosensors, and it seems that we still have a long way to go in this respect. In our opinion, the third-generation amperometric biosensing is optimistically expected to be globally realized through effectively connecting the redox active center of the enzyme to the electrode by using nano-/subnanosized conducting wires of little interference to the enzyme conformation.125
4. The construction of electrochemical cells for amperometric glucose biosensors
The fabrication of various electrochemical cells and their utilization in glucose sensing has been widely reported. A flow-through electrochemical detector for glucose based on a GOx-modified microelectrode incorporating redox and conducting polymer materials was developed by Rohde et al.135 Ito et al. fabricated a microfluidic device for glucose detection using a microsized direct methanol fuel cell as an amperometric detection power source.136 The improvement of electrochemical detector cells is highly desirable because of their extensive use in many areas. The effective immobilization of enzymes, the protection of the bioactivity of enzymes, and their integration and applications are the key factors for the fabrication of successful electrochemical cells.4.1. The immobilization of enzymes on sensing electrodes
The immobilization of enzymes on solid interfaces is one of the crucial factors for the fabrication of enzyme-based devices, which has been realized by various strategies, such as physical adsorption, covalent attachment, and physical entrapment or encapsulation, etc.137 Although immobilization can improve the long-term and operational stability of enzymes, this is often at the expense of significant loss in the catalytic activity of the immobilized enzymes. So the maintenance of enzymatic activity, the stability of immobilized enzymes, and the efficiency of the enzyme-based devices for application in biosensing are mainly considered in choosing an optimal immobilizing strategy.Physical adsorption is a simple way to immobilize enzymes, which can effectively maintain enzymatic activity under mild experimental conditions. However, stability is one of the most serious problems for the wide applications of adsorbed enzymes, which is greatly affected by a combination of factors, e.g., pH, ionic strength, temperature, surface tension, charges, and matrix. Scientists have conducted a lot of excellent research on enzyme adsorption and have greatly improved the stability of adsorbed enzymes. For example, He et al. realized the activity and thermal stability improvements of GOx upon adsorption on biocompatible core-shell poly(methyl methacrylate)-bovine serum albumin (PMMA-BSA) nanoparticles.138 Raftlike lipid domains were demonstrated to be an ideal adsorption surface for enhancing the stability of adsorbed proteins.139 The adsorption behavior and mechanism of enzymes on highly oriented pyrolytic graphite, CNTs, nanoporous materials, self-assembled monolayer (SAM) on gold, and dipalmitoylphosphatic acid monolayer have been extensively investigated recently.138–147
Covalent attachment is considered as one of the most effective methods for the stable immobilization of enzymes and is widely employed to fabricate biosensors.148–150 For instance, Xu et al. reported the covalent immobilization of GOx on well-defined poly(glycidyl methacrylate)-Si(111) hybrids from surface-initiated atom-transfer radical polymerization and the immobilized GOx exhibited a corresponding relative activity of about 60% and an improved stability during storage over that of the free enzyme.151 Wan et al. fabricated an amperometric glucose biosensor by covalent immobilization of GOx with the pendant hydroxyl groups of chitosan using 1,4-carbonyldiimidazole as the bifunctional linker and the spatially biocompatible microenvironment greatly enhanced the amount and biocatalytic activity of the immobilized enzyme.152 Pandey et al. realized the significant enhancement in the activity of GOx by covalently immobilizing GOx onto chemically synthesized thiolated gold nanoparticles and the covalently immobilized GOx thiolated nanoparticles exhibited a response time of 30 s, a shelf life of more than 6 months, and improved tolerance to both pH and temperature.153 We suggested a simple and rather universal method for the highly efficient immobilization of enzymes by aqueous electro-deposition of enzyme-tethered chitosan for sensitive amperometric biosensing.154 While many protocols for covalent immobilization of enzymes employ their amino acid residuals, the glycosyl-affinitive boronic acid polymer was suggested to covalently immobilize the enzyme at the glycosyl sites (so-called boronic acid–diol interaction), which should less affect enzymatic activity versus covalent immobilization of enzymes at their amino acid residuals.155
Entrapment or encapsulation of an enzyme inside a solid matrix (e.g. polymers, redox gels, sol–gel-derived glasses, and carbon pastes) is preferred by most workers because of its advantages in enzyme immobilization, e.g., the good maintenance of enzymatic activity and the relatively high stability of immobilized enzymes. The protocol of entrapping enzymes in electrodeposited polymers offers a film-thickness/site controllability higher than many other methods and is thus appropriate for preparing biosensing ultramicroelectrodes in miniaturized devices. Electropolymerized nonconducting films are widely used to prepare enzyme biosensors because of their high resistance against electrode fouling and high anti-interferent capability against some coexisting electroactive substances.156–159 However, a relatively low load of enzymes was observed in the electrosynthesized films because the entrapment of enzymes was mainly due to the presence of enzymes in the vicinity of the growing films.160 Recently, we reported the immobilization of enzymes in dopamine and noradrenalin polymers, which can yield electrode-supported films much thicker than the traditional insulating polymer films owing to their special melanin-like porous structures. To our knowledge, the electropolymerization of dopamine and noradrenalin and the utilization of the resultant polymers to immobilize biomacromolecules were investigated in our group for the first time.161 The dopamine and noradrenalin polymers were demonstrated to be quite useful in entrapping biomacromolecules at high loading/activity, and the glucose-assay selectivity was also very high.86,89,106,161,162 For the first time, we have introduced a preoxidation (chemical, electrochemical, or enzymatic) step before the electropolymerization of monomers, which combines the respective advantages of chemical oxidation polymerization and electropolymerization and largely improves the biosensing performance (Fig. 3).86,89,160,162–164 Chemical oxidation synthesis alone was also utilized by our group to prepare high-performance enzyme films.106,165 However, coating the working electrode with a polymeric film often leads to lower signals and longer response times because substrates have to diffuse into the matrix to interact with the entrapped enzyme, so the exploitation of excellent permselective films that can effectively reject interferences but well keep the biosensing performances is highly desirable.
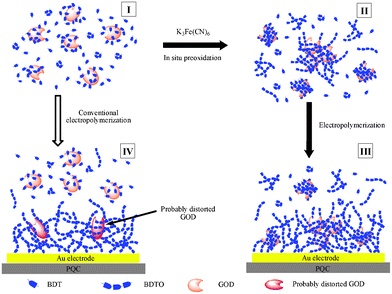 |
| Fig. 3 Procedures for immobilization of GOx via the chemical preoxidation electropolymerization and conventional electropolymerization protocols. Reprinted with permission from ref. 160, copyright 2008, American Chemistry Society. | |
In the last 20 years, the main development of electrochemical glucose biosensors can be attributed to the exploitation of various functional nanomaterials to improve the performance of the resultant biosensors. Conducting nanomaterials can greatly enhance the electron communication between enzymes and electrodes. Amperometric glucose biosensors based on various nanomaterials have been extensively studied in recent years, and some typical examples are listed in Table 1.26,28,93,115,125,166 Zhu et al. wrote a critical review of glucose biosensors based on the carbon nanomaterials of CNTs and graphene.167 Rahman et al. reported a comprehensive review of glucose biosensors based on nanostructured metal oxides.168 A review on glucose and H2O2 biosensors based on a silver nanoparticles-modified electrode was written by Rad et al.169 Iwamoto et al. reported the activity enhancement of a screen-printed carbon electrode by modification with gold nanoparticles for glucose determination.170 Qiu et al. fabricated aligned nanoporous PtNi (np-PtNi) nanorod-like structures and used them in electrocatalysis and biosensing.171 The np-PtNi nanorods exhibit remarkably improved electrocatalytic activity towards ethanol oxidation and H2O2 oxidation/reduction compared with the commercial Pt/C catalyst, and the GOx modified np-PtNi electrode can sensitively detect glucose over a wide linear range.171 Nanocomposites have also been used to fabricate high-performance amperometric glucose biosensors,172,173i.e., nanoleave-shaped copper oxide decorated MWCNTs composites,174 Pt-MWCNTs-alumina-coated silica nanocomposite,175 Pt-dispersed hierarchically porous electrode,176 and boron nitride nanotubes-polyaniline-Pt hybrids.93
Table 1 Examples of nanomaterials widely used for the fabrication of GOx-based amperometric glucose sensors in recent yearsa
Nanomaterial | Electrode composition | Sensitivity/μA mM−1 cm−2 | Linear Range/mM | LOD/μM | Year | Ref. |
---|
LOD: limit of detection; PtNPs: Pt nanoparticles; Pt-DENs: dendrimer-encapsulated Pt nanoparticles; AgNPs: Ag nanoparticles; PANINFs: polyaniline nanofibers; CS: chitosan; ICPTES: 3-isocyanatopropyltriethoxysilane; PVP: poly(vinylpyridine); SWCNH: single-walled carbon nanohorns; PdNPs: Pd nanoparticles; Fc: ferrocene; GO: graphene oxide; ERGO: electrochemically reduced graphene oxide; RGO: reduced graphene oxide; PAMAM: polyamidoamine; AuPdNPs: gold-palladium (1 : 1) bimetallic nanoparticles; PEDOT: poly(3,4-ethylenedioxythiophene); NiO HS: NiO hollow spheres; PDA: polydopamine; PBNCs: polymeric bionanocomposites. |
---|
Pt | boron nitride nanotubes-PANI-PtNPs | 19.0 | 0.01–5.5 | 0.18 | 2011 | 93 |
PtNPs-MWCNTs-PANI | 16.1 | 0.003–8.2 | 1.0 | 2011 | 177 |
Pt-DENs/PANI/CNTs | 42.0 | 0.001–12 | 0.50 | 2009 | 178 |
Pt nanoclusters-MWCNTs | 12.8 | 0.003–12 | 1.0 | 2012 | 179 |
hollow Pt decorated MWCNTs | 22.8 | 0.0012–8.4 | 0.40 | 2011 | 180 |
Au | AuNPs-MWCNT | 19.3 | 0.02–10 | 2.3 | 2011 | 181 |
AuNPs-hydrogel microstructures | 100 | 0.1–10 | 0.37 | 2011 | 182 |
AuNPs | 47.2 | 0.001–5 | 1.0 | 2012 | 183 |
crystalline AuNPs-MWCNTs | 5.7 | 0.05–22 | 20 | 2009 | 184 |
Ag | AgNPs/CNT/chitosan | 136 | 0.0005–0.05 | 0.10 | 2009 | 185 |
PVP-Ag nanowires | 22.4 | 2–20 | N/A | 2012 | 186 |
AgNPs/PANINFs | N/A | 1–12 | 0.25 | 2012 | 187 |
MWCNTs | MWCNTs-CS nanowire | 5.03 μA mM−1 | 1–10 | N/A | 2011 | 188 |
PB/MWCNTs-GOx-CS-ICPTES | 15.2 | 0.025–1.3 | 7.5 | 2011 | 189 |
Pt/FeyOx-MWCNTs/CS | N/A | 0.006–6.2 | 2.0 | 2010 | 190 |
CS-PB-MWNTs-PtCo | 23.4 | 0.0015–1.1 | 0.47 | 2011 | 191 |
PtPd-MWCNTs | 112 | 0.062–14 | 0.031 | 2012 | 192 |
Fe3O4@SiO2/MWNTs | 58.9 | 0.001–30 | 0.80 | 2010 | 193 |
SWCNTs | polyelectrolyte-SWCNTs | 157 | 0.5–5.0 | 5.0 | 2012 | 194 |
SWCNTs-PVP-Os | 56.0 | N/A | N/A | 2009 | 141 |
SWCNH | Nafion-SWCNH | 15.0 | 0–6.0 | 6.0 | 2008 | 132 |
Graphene | PdNPs/CS-graphene | 31.2 | 0.001–1.0 | 0.20 | 2011 | 195 |
TiO2-graphene | 6.20 | N/A | 1.0 | 2012 | 196 |
Pt-Au/AuNPs-graphene | N/A | 30 | 1.0 | 2010 | 197 |
graphene nanosheet | N/A | 2–40 | 3.0 ± 0.5 | 2010 | 117 |
Graphene oxide | CS-Fc/GO nanocomposite | 10.0 | 0.02–6.8 | 7.6 | 2011 | 198 |
ERGO | 22.8 | 0.005–12 | 1.0 | 2011 | 199 |
RGO-PAMAM-Ag | 75.7 | 0.032–1.9 | 4.5 | 2012 | 200 |
ERGO-AuPdNPs | 267 | 0.5–3.5 | 6.9 | 2011 | 201 |
Metal oxides | ZnO nanofiber | 70.2 | 0.25–19 | 1.0 | 2010 | 202 |
19.5 | 0.2–2.0 | 50 | 2010 | 173 |
TiO2 | 9.90 | up to 1.5 | 1.3 | 2011 | 116 |
nanostructured metal-oxides | N/A | N/A | N/A | 2010 | 168 |
PEDOT-NiO HS | 16.9 | up to 1.5 | N/A | 2010 | 203 |
graphene-chitosan-ZrO2 | 7.60 | 0.2–1.6 | 46 | 2012 | 204 |
Others | nanoporous PtNi nanorod | 2.00 | 0.5–21 | 20 | 2012 | 171 |
magnetic polymer | 110 | 0.002–2.6 | 0.33 | 2010 | 165 |
PDA-PtNPs PBNCs | 99.0 | 0.0005–4.5 | 0.09 | 2009 | 89 |
polyaniline nanotubes | 97.2 ± 4.6 | 0.01–5.5 | 0.3 ± 0.1 | 2009 | 97 |
exfoliated graphite nanoplatelets | 14.2 | up to 6.0 | 10 | 2007 | 205 |
Si nanowire-AuNPs | N/A | 0.1–0.8, 1–16 | 50 | 2010 | 206 |
4.2. The determination of enzymatic activity
The tremendous potential of enzymes as highly efficient catalysts is commonly recognized, but the poor stability of enzymes greatly limits their practical applications in many cases. As a kind of high-performance biocatalyst, enzymes express their unique catalysis on specific reactions based on their enzymatic activity, which is one of the key factors of enzymes. But the enzymatic activity tends to be influenced by the external environment. The protection of the activity of enzymes is of great importance in various enzyme-based devices. So the development of novel strategies which can effectively protect the activity of enzymes is important and relevant.The analysis and determination of the enzymatic activity of immobilized enzymes is an important approach and provides critical evidence for investigating the enzyme reaction mechanism, evaluating the enzyme immobilization procedure, improving the enzyme immobilization material and constructing novel high-performance enzyme-based biosensors. Wang et al. reported new insights into the effects of thermal treatment on the catalytic activity and conformational structure of GOx studied by electrochemistry, IR spectroscopy, and theoretical calculations.207 Willner's group developed a novel protocol to follow the biocatalytic activities of GOx by electrochemically crosslinked enzyme-Pt nanoparticles composite electrodes.90 Wu et al. investigated the effects of different types of ionic liquids on enzymatic catalysis of GOx and pointed out that the nature of ionic liquids is the main factor that affects the electrocatalytic activity of the GOx towards the oxidation of glucose.18 Recently, Jensen et al. presented a novel method combining protein adsorption studies at nanostructured quartz crystal microbalance sensor surfaces (QCM-D) with optical (SPR) and electrochemical methods (cyclic voltammetry), allowing quantification of both bound protein amount and activity.208
In addition to the analysis of total enzymatic activity of immobilized enzymes by measuring the amount of products (e.g. H2O2) in the oxidation of β-D-glucose via a spectroscopic method, electrochemical method, or titration, the quantitative determination of enzymatic specific activity is also very important. Bourdillon et al. used a radioactive 125I labeling method to quantify the immobilized enzyme and realized the detection of enzyme activity by analyzing the cyclic voltammetric responses recorded in the presence of glucose with ferrocene methanol as the mediator.209 The quantification of enzymatic specific activity includes the quantification of the amount of immobilized enzymes (mGOx) and the detection of total enzymatic activity of immobilized enzymes. Our group conducted a series of biosensing studies based on evaluating the enzymatic specific activity of the immobilized enzyme for optimizing biomolecule-immobilization materials and methods.86,133,154,160,164 Enzymatic specific activity (ESA) is expressed as
, where
is the amount of enzymatically generated H2O2 in the first 60 s of enzymatic reaction on the electrode and mGOx is the mass of GOx involved in enzymatic reactions. The quantification of immobilized enzymes was realized via the quartz crystal microbalance (QCM) technique, which can detect an electrode-mass change down to the nanogram level (generally equivalent to submonolayer modification). The immobilized enzyme (the mGOx in this case) can be quantified from its resultant frequency decrease according to the Sauerbrey equation. The enzymatic specific activity of GOx immobilized on poly(o-phenylenediamine)/GOx-glutaraldehyde/PB/Au electrode was determined to be 2.4 kU g−1.82 Later, the dopamine and noradrenalin polymers of special melanin-like porous structure were reported to be able to encapsulate GOx with an enzymatic specific activity as high as (16 ± 0.6) kU g−1 by a conventional electropolymerization protocol and (34 ± 1) kU g−1 by a chemical preoxidation-involved electropolymerization protocol.86 The determination of enzymatic specific activity is expected to be effective in evaluating the performance of materials and protocols used in biomacromolecular immobilization.
The enzymatic activity can also be utilized in inhibitive analysis of environmental pollutants. Toxic Hg2+, Cu2+, Pb2+, Cd2+, and Ag+ were reported to be able to strongly interact with enzymes and inhibit the bioactivity of enzymes.210,211 Many immobilized enzymes have been used for inhibitive assays of heavy metal ions.212–216 GOx of low cost, good stability, and high specific activity has been widely used for the inhibitive assays of several heavy metal ions. Malitesta et al. reported heavy metal ion determination by GOx-based amperometric biosensors.217 The inhibitive determination of heavy metal ions by an amperometric GOx biosensor was also investigated by Guascito et al.218 Enzyme inhibition-based biosensors for food safety and environmental monitoring were extensively reviewed by Amine et al. in 2006.219 Recently, we have developed a comprehensive experimental platform based on QCM and electroanalysis techniques to quantitatively study heavy metal ions–enzyme interactions and amperometric inhibitive assays of heavy metal ions and realized the quantification of the number of the bound heavy metal ions per GOx molecule at various inhibition percentages. This platform is expected to find wide applications in enzymatic inhibitive assays and quantitative studies of the inhibition effects of heavy metal ions on many other redox-event-relevant enzymes.220
4.3. The integration of electrochemical cells for biosensors
Electrochemical glucose biosensors have played a key role in the move to simple, one-step blood glucose testing because they satisfy well the demands of personal (home) glucose testing. Various novel glucose biosensors are expected to be applied to fabricate blood glucose test strips, which are manufactured and marketed by numerous companies and have been popularly used by diabetic patients. Implantable glucose sensors221,222 are highly expected to serve well as point-of-care probes. So the integration of electrochemical cells is one of the key points for the application of amperometric glucose biosensors in many areas. In conventional laboratories, the electrochemical cells for biosensors are commonly integrated with three separated electrodes inserted in the corresponding electrolyte solution, and the corresponding enzymatic reactions take place on the enzyme-modified working electrode. The developed screen-printed three-electrodes realized the effective simplification and miniaturization of integrated electrochemical cells.223 The majority of personal blood glucose monitors rely on disposable screen-printed enzyme electrode test strips.224 Such electrode strips are commercially produced by the screen-printing micro-fabrication or vapor deposition process.225 The construction of strips is illustrated in Fig. 4 (left). Each strip contains the printed working and reference electrodes, and the working electrode is modified with enzyme, mediator, stabilizer, surfactant, linking, and binding agents. These reagents are commonly dispensed by ink-jet printing technology and deposited in the dry form.26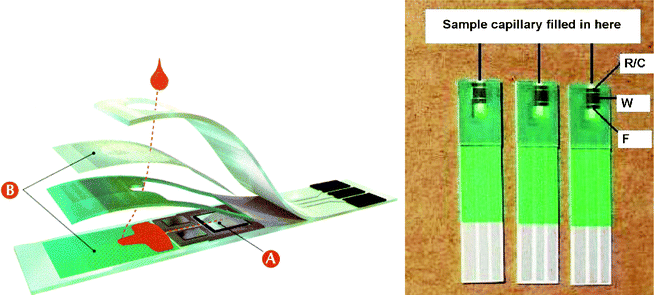 |
| Fig. 4 Cross section of a commercial strip for self-testing of blood glucose (left, reprinted with permission from ref. 26, copyright 2008, American Chemistry Society) and the test strips designed in 2008 (right, reprinted with permission from ref. 225, copyright 2009, Elsevier). | |
The miniaturization of device and acceleration of analytical rate are pursued in the development of test strips. The test strips made in the 1990s utilized a carbon-working electrode and an Ag/AgCl reference electrode system. For these strips, a high operation voltage (400 mV), a large volume of blood (20 μL) and a long test time (around 20–25 s) were required. For the test strips made in late 1999, the operation voltage was lowered to 300 mV and the blood sample was automatically transferred into the reaction area at the edge of the strip by a capillary mechanism, with the sample volume reduced to 3 μL and the test time reduced to 10 s by utilizing a spacer and a hydrophilic membrane cover to restrict the reaction zone. The sample volume was further reduced to 0.5–2 μL and the test time was shortened to 5 s with the great improvement in quality of screen-printing for an example made in mid-2000. Three electrodes are adapted and printed on the support on the latest test strips (Fig. 4 (right)) to improve the test accuracy and minimize interference from the blood sample. Various membranes such as mesh, filter, and surfactants are often incorporated into the test strips to ensure a uniform coverage of sample and separate the blood cells. Cross contamination and drift are eliminated from these single-use devices and high clinical accuracy is ensured with a high degree of sophistication being essential.
The biofuel cell is another example of the integration of GOx-based amperometric glucose biosensors.226 An enzyme-based biofuel cell can work in the following way: the oxidase-modified electrode in the anodic compartment oxidizes the fuel substrate, while transferring electrons to the electrode; the enzyme electrode in the cathodic compartment reduces the oxidizer, while transferring electrons from the electrode to the oxidizer. Biofuel cells are considered as a novel kind of energy conversion technology which can be operated under mild conditions and serve as in vivo power sources for bioelectronics,227 which have become one of the most popular research areas and hold great promise in the many applications of biomolecules. Integrated, electrically contacted thin film-modified enzyme electrodes (the second and the third generation of enzyme electrodes) are widely utilized in fabricating biofuel cells.228 Our group has conducted a series of investigations on biofuel cells by utilizing an electrochemical noise device.66,105–107,162,229 Currently, GOx as biocatalyst at the anode is considered as the most promising type of implantable biofuel cell.230 Biofuel cells are also expected to be used in implanted cardiac pacemakers and self-powered biosensing devices, which have been studied and reported by some scientists and deserve continued efforts.231–236 A number of excellent reviews on biofuel cells have been reported,228,237–240 and the interested reader may read them for details.
5. Conclusions
Numerous publications on electrochemical glucose sensors and the undamped activity of this research field persuasively reflect the importance of glucose sensing. Major fundamental and technological advances have been utilized to enhance the capabilities and improve the reliability of glucose measuring devices. GOx-based amperometric glucose biosensors are expected to be ideal models for the fabrication of diverse biosensing devices. However, there are still a lot of challenges ahead of us, the exploitation of excellent materials, the detailed investigation of the tricky problems related to the enzyme-based devices, the development of miniaturized implantable amperometric biosensors, and the investigation of the relationship of the catalytic function of enzymes with the conformation of the enzyme are still worthy of special research and are also of great significance for the real revolution and innovation of enzymatic biosensors. The exploitation of ideal sensors with reliable real-time continuous monitoring of all blood glucose variations with high selectivity and speed over extended periods is still a challenge worthy of further research efforts. The development of miniaturized implantable biosensors and biofuel cells are expected to find wide applications. Nonenzymatic electrocatalytic materials and artificial enzymes are also very important research topics at present, which may lead to high-performance catalysts for glucose sensing and relevant organic electrosynthesis.Acknowledgements
This work was supported by the National Natural Science Foundation of China (Grants 21075036, 21175042, and 21105026), Hunan Lotus Scholars Program, the Foundations of Hunan Provincial Education Department (Grants 11B078 and 11A069) and Hunan Province (Grant 11JJ4014), Program for Science and Technology Innovative Research Team in Higher Educational Institutions of Hunan Province and State Key Laboratories of Chemo/Biosensing and Chemometrics and of Electroanalytical Chemistry.References
- A. Heller and B. Feldman, Electrochemical glucose sensors and their applications in diabetes management, Chem. Rev., 2008, 108, 2482–2505 CrossRef CAS.
- M. M. W. Muscatello, L. E. Stunja and S. A. Asher, Polymerized crystalline colloidal array sensing of high glucose concentrations, Anal. Chem., 2009, 81, 4978–4986 CrossRef.
- D. Odaci, B. N. Gacal, B. Gacal, S. Timur and Y. Yagci, Fluorescence sensing of glucose using glucose oxidase modified by PVA-pyrene prepared via “click” chemistry, Biomacromolecules, 2009, 10, 2928–2934 CrossRef CAS.
- T. Saxl, F. Khan, M. Ferla, D. Birch and J. Pickup, A fluorescence lifetime-based fibre-optic glucose sensor using glucose/galactose-binding protein, Analyst, 2011, 136, 968–972 RSC.
- M-S. Steiner. A. Duerkop and O. S. Wolfbeis, Optical methods for sensing glucose, Chem. Soc. Rev., 2011, 40, 4805–4839 RSC.
- X. Wu, L. R. Lin, Y. J. Huang, Z. Li and Y. B. Jiang, A 2
:
2 stilbeneboronic acid–c-cyclodextrin fluorescent ensemble highly selective for glucose in aqueous solutions, Chem. Commun., 2012, 48, 4362–4364 RSC. - C. Yu and V. W-W. Yam, Glucose sensing via polyanion formation and induced pyrene excimer emission, Chem. Commun., 2009, 45, 1347–1349 RSC.
- R. J. McNichols and G. L. Cote, Optical glucose sensing in biological fluids: An overview, J. Biomed. Opt., 2000, 5, 5–16 CrossRef CAS.
- V. Sanz Marcos and S. d. J. Galbán, Direct glucose determination in blood using a reagentless optical biosensor, Biosens. Bioelectron., 2007, 22, 2876–2883 CrossRef.
- A. M. Winkler, G. T. Bonnema and J. K. Barton, Optical polarimetry for noninvasive glucose sensing enabled by Sagnac interferometry, Appl. Opt., 2011, 50, 2719–2731 CrossRef.
- E. W. Stein, P. S. Grant, H. Zhu and M. J. McShane, Microscale enzymatic optical biosensors using mass transport limiting nanofilms. 1. Fabrication and characterization using glucose as a model analyte, Anal. Chem., 2007, 79, 1339–1348 CrossRef CAS.
- C-H. Chou J.-C. Chen, C.-C. Tai, I. W. Sun and J.-M. Zen, A nonenzymatic glucose sensor using nanoporous platinum electrodes prepared by electrochemical alloying/dealloying in a water-insensitive zinc chloride-1-ethyl-3-methylimidazolium chloride ionic liquid, Electroanalysis, 2008, 20, 771–775 CrossRef.
- C. Su, C. Zhang, G. Lu and C. Ma, Nonenzymatic electrochemical glucose sensor based on Pt nanoparticles/mesoporous carbon matrix, Electroanalysis, 2010, 22, 1901–1905 CrossRef CAS.
- J. Wang, D. F. Thomas and A. Chen, Nonenzymatic electrochemical glucose sensor based on nanoporous PtPb networks, Anal. Chem., 2008, 80, 997–1004 CrossRef CAS.
- Y. Myung, D. M. Jang, Y. J. Cho, H. S. Kim, J. Park, J. U. Kim, Y. Choi and C. J. Lee, Nonenzymatic amperometric glucose sensing of platinum, copper sulfide, and tin oxide nanoparticle-carbon nanotube hybrid nanostructures, J. Phys. Chem. C, 2009, 113, 1251–1259 CAS.
- L. Meng, J. Jin, G. Yang, T. Lu, H. Zhang and C. Cai, Nonenzymatic electrochemical detection of glucose based on palladium-single-walled carbon nanotube hybrid nanostructures, Anal. Chem., 2009, 81, 7271–7280 CrossRef CAS.
- S. Park, T. D. Chung and H. C. Kim, Nonenzymatic glucose detection using mesoporous platinum, Anal. Chem., 2003, 75, 3046–3049 CrossRef CAS.
- D. Luo, L. Wu and J. Zhi, Fabrication of boron-doped diamond nanorod forest electrodes and their application in nonenzymatic amperometric glucose biosensing, ACS Nano, 2009, 3, 2121–2128 CrossRef CAS.
- E. Shoji and M. S. Freund, Potentiometric sensors based on the inductive effect on the pKa of poly(aniline): A nonenzymatic glucose sensor, J. Am. Chem. Soc., 2001, 123, 3383–3384 CrossRef CAS.
- Y. Sun, H. Buck and T. E. Mallouk, Combinatorial discovery of alloy electrocatalysts for amperometric glucose sensors, Anal. Chem., 2001, 73, 1599–1604 CrossRef CAS.
- I. G. Casella, A. Destradis and E. Desimoni, Colloidal gold supported onto glassy carbon substrates as an amperometric sensor for carbohydrates in flow injection and liquid chromatography, Analyst, 1996, 121, 249–254 RSC.
- S. Fei, J. Chen, S. Yao, G. Deng, L. Nie and Y. Kuang, Electroreduction of (-glucose on CNT/graphite electrode modified by Zn and Zn–Fe alloy, J. Solid State Electrochem., 2005, 9, 498–503 CrossRef CAS.
- J. H. Zhu, J. Jiang, J. P. Liu, R. M. Ding, Y. Y. Li, H. Ding, Y. M. Feng, G. M. Wei and X. T. Huang, CNT-network modified Ni nanostructured arrays for high performance non-enzymatic glucose sensors, RSC Adv., 2011, 1, 1020–1025 RSC.
- Y. Ding, Y. Wang, L. Su, H. Zhang and Y. Lei, Preparation and characterization of NiO–Ag nanofibers, NiO nanofibers, and porous Ag: Towards the development of a highly sensitive and selective non-enzymatic glucose sensor, J. Mater. Chem., 2010, 20, 9918–9926 RSC.
- R. Wilson and A. P. F. Turner, Glucose-oxidase-An ideal enzyme, Biosens. Bioelectron., 1992, 7, 165–185 CrossRef CAS.
- J. Wang, Electrochemical glucose biosensors, Chem. Rev., 2008, 108, 814–825 CrossRef CAS.
- F. S. Saleh, L. Mao and T. Ohsaka, A promising dehydrogenase-based bioanode for a glucose biosensor and glucose/O2 biofuel cell, Analyst, 2012, 137, 2233–2238 RSC.
- O. Yehezkeli, R. Tel-Vered, S. Raichlin and I. Willner, Nano-engineered flavin-dependent glucose dehydrogenase/gold nanoparticle-modified electrodes for glucose sensing and biofuel cell applications, ACS Nano, 2011, 5, 2385–2391 CrossRef CAS.
- M. Zayats, E. Katz, R. Baron and I. Willner, Reconstitution of apo-glucose dehydrogenase on pyrroloquinoline quinone-functionalized Au nanoparticles yields an electrically contacted biocatalyst, J. Am. Chem. Soc., 2005, 127, 12400–12406 CrossRef CAS.
- M. N. Zafar, X. J. Wang, C. Sygmund, R. Ludwig, D. Leech and L. Gorton, Electron-transfer studies with a new flavin adenine dinucleotide dependent glucose dehydrogenase and osmium polymers of different redox potentials, Anal. Chem., 2012, 84, 334–341 CrossRef CAS.
- F. S. Saleha, L. Maob and T. Ohsakac, Development of a dehydrogenase-based glucose anode using a molecular assembly composed of nile blue and functionalized swcnts and its applications to a glucose sensor and glucose/O2 biofuel cell, Sens. Actuators, B, 2011, 152, 130–135 CrossRef.
- T. Yamazaki, K. Kojima and K. Sode, Extended-range glucose sensor employing engineered glucose dehydrogenases, Anal. Chem., 2000, 72, 4689–4693 CrossRef CAS.
- P. Du, P. Wu and C. X. Cai, A glucose biosensor based on electrocatalytic oxidation of NADPH at single-walled carbon nanotubes functionalized with poly(nile blue A), J. Electroanal. Chem., 2008, 624, 21–26 CrossRef CAS.
- R. Golbik, M. Naumann, A. Otto, E. C. Müller, J. Behlke, R. Reuter, G. Hübner and T. M. Kriegel, Regulation of phosphotransferase activity of hexokinase 2 from saccharomyces cerevisiae by modification at serine-14, Biochemistry, 2001, 40, 1083–1090 CrossRef CAS.
- B. E. Lewis and V. L. Schramm, Binding equilibrium isotope effects for glucose at the catalytic domain of human brain hexokinase, J. Am. Chem. Soc., 2003, 125, 4785–4798 CrossRef CAS.
- N. Anicet, C. Bourdillon, J. Moiroux and J.-M. Savéant, Step-by-step avidin-biotin construction of bienzyme electrodes. Kinetic analysis of the coupling between the catalytic activities of immobilized monomolecular layers of glucose oxidase and hexokinase, Langmuir, 1999, 15, 6527–6533 CrossRef CAS.
- A. Caduff, M. S. Talary and P. Zakharov, Cutaneous blood perfusion as a perturbing factor for noninvasive glucose monitoring, Diabetes Technol. Ther., 2010, 12, 1–9 CrossRef CAS.
- J. N. Roe and B. R. Smoller, Bloodless glucose measurements, Crit. Rev. Ther. Drug Carr. Syst., 1998, 15, 199–241 CAS.
- C. E. F. do Amaral and B. Wolf, Current development in non-invasive glucose monitoring, Med. Eng. Phys., 2008, 30, 541–549 CrossRef.
- M. Mueller, M. Grunze, E. H. Leiter, P. C. Reifsnyder, U. Klueh and D. Kreutzer, Non-invasive glucose measurements in mice using mid-infrared emission spectroscopy, Sens. Actuators, B, 2009, 142, 502–508 CrossRef.
- O. K. Cho, Y. Y. Kim, H. Mitsumaki and K. Kuwa, Noninvasive measurement of glucose by metabolic heat conformation method, Clin. Chem., 2004, 50, 1894–1898 CAS.
- R. O. Potts, J. A. Tamada and M. J. Tierney, Glucose monitoring by reverse iontophoresis, Diabetes/Metab. Res. Rev., 2002, 18, S49–S53 CrossRef CAS.
- J. T. Baca, D. N. Finegold and S. A. Asher, Tear glucose analysis for the noninvasive detection and monitoring of diabetes mellitus, Ocul. Surf., 2007, 5, 280–293 CrossRef.
- Q. Yan, B. Peng, G. Su, B. E. Cohan, T. C. Major and M. E. Meyerhoff, Measurement of tear glucose levels with amperometric glucose biosensor/capillary tube configuration, Anal. Chem., 2011, 83, 8341–8346 CrossRef CAS.
- B. Beden, F. Largeaud, K. B. Kokoh and C. Lamy, Fourier transform infrared reflectance spectroscopic investigation of the electrocatalytic oxidation of D-glucose: Identification of reactive intermediates and reaction products, Electrochim. Acta, 1996, 41, 701–709 CrossRef CAS.
- M. W. Hsiao, R. R. Adzic and E. B. Yeager, The dissipated energy of electrode surfaces: Temperature jumps from coupled transport processes, J. Electrochem. Soc., 1996, 143, 759–767 CrossRef CAS.
- Y. Xia, W. Huang, J. F. Zheng, Z. J. Niu and Z. L. Li, Nonenzymatic amperometric response of glucose on a nanoporous gold film electrode fabricated by a rapid and simple electrochemical method, Biosens. Bioelectron., 2011, 26, 3555–3561 CrossRef CAS.
- H. Gao, F. Xiao, C. B. Ching and H. Duan, One-step electrochemical synthesis of PtNi nanoparticle-graphene nanocomposites for nonenzymatic amperometric glucose detection, ACS Appl. Mater. Interfaces, 2011, 3, 3049–3057 CAS.
- Y. Y. Song, D. Zhang, W. Gao and X. H. Xia, Nonenzymatic glucose detection by using a three-dimensionally ordered, macroporous platinum template, Chem.–Eur. J., 2005, 11, 2177–2182 CrossRef CAS.
- S. Park, H. Boo and T. D. Chung, Electrochemical non-enzymatic glucose sensors, Anal. Chim. Acta, 2006, 556, 46–57 CrossRef CAS.
- K. E. Toghill and R. G. Compton, Electrochemical non-enzymatic glucose sensors: A perspective and an evaluation, Int. J. Electrochem. Sci., 2010, 5, 1246–1301 CAS.
- M. S. Celej and G. Rivas, Amperometric glucose biosensor based on gold-dispersed carbon paste, Electroanalysis, 1998, 10, 771–775 CrossRef CAS.
- M. W. Hsiao, R. R. Adzic and E. B. Yeager, The effects of adsorbed anions on the oxidation of D-glucose on gold single crystal electrodes, Electrochim. Acta, 1992, 37, 357–363 CrossRef CAS.
- L. D. Burke, Premonolayer oxidation and its role in electrocatalysis, Electrochim. Acta, 1994, 39, 1841–1848 CrossRef CAS.
- X. M. Chen, Z. J. Lin, D. J. Chen, T. T. Jia, Z. M. Cai, X. R. Wang, X. Chen, G. N. Chen and M. Oyamad, Nonenzymatic amperometric sensing of glucose by using palladium nanoparticles supported on functional carbon nanotubes, Biosens. Bioelectron., 2010, 25, 1803–1808 CrossRef CAS.
- C. X. Wang, L. W. Yin, L. Y. Zhang and R. Gao, Ti/TiO2 nanotube array/Ni composite electrodes for nonenzymatic amperometric glucose sensing, J. Phys. Chem. C, 2010, 114, 4408–4413 CAS.
- W. Wang, Z.
Y. Li, W. Zheng, J. Yang, H. N. Zhang and C. Wang, Electrospun palladium(IV)-doped copper oxide composite nanofibers for non-enzymatic glucose sensors, Electrochem. Commun., 2009, 11, 1811–1814 CrossRef CAS.
- H. Yin, C. Zhou, C. Xu, P. Liu, X. Xu and Y. Ding, Aerobic oxidation of D-glucose on support-free nanoporous gold, J. Phys. Chem. C, 2008, 112, 9673–9678 CAS.
- X. Kang, Z. Mai, X. Zou, P. Cai and J. Mo, A sensitive nonenzymatic glucose sensor in alkaline media with a copper nanocluster/multiwall carbon nanotube-modified glassy carbon electrode, Anal. Biochem., 2007, 363, 143–150 CrossRef CAS.
- T. You, O. Niwa, Z. Chen, K. Hayashi, M. Tomita and S. Hirono, An amperometric detector formed of highly dispersed Ni nanoparticles embedded in a graphite-like carbon film electrode for sugar determination, Anal. Chem., 2003, 75, 5191–5196 CrossRef CAS.
- H. Bai, M. Han, Y. Du, J. Bao and Z. Dai, Facile synthesis of porous tubular palladium nanostructures and their application in a nonenzymatic glucose sensor, Chem. Commun., 2010, 46, 1739–1741 RSC.
- P. F. Luo and T. Kuwana, Nickel-titanium alloy electrode as a sensitive and stable LCEC detector for carbohydrates, Anal. Chem., 1994, 66, 2775–2782 CrossRef CAS.
- J. Zhao, F. Wang, J. J. Yu and S. S. Hua, Electro-oxidation of glucose at self-assembled monolayers incorporated by copper particles, Talanta, 2006, 70, 449–454 CrossRef CAS.
- J. H. Yuan, K. Wang and X. H. Xia, Highly ordered platinum-nanotubule arrays for amperometric glucose sensing, Adv. Funct. Mater., 2005, 15, 803–809 CrossRef CAS.
- H. Pang, Q. Y. Lu, J. J. Wang, Y. C. Lia and F. Gao, Glucose-assisted synthesis of copper micropuzzles and their application as nonenzymatic glucose sensors, Chem. Commun., 2010, 46, 2010–2012 RSC.
- F. Y. Xie, Z. Huang, C. Chen, Q. J. Xie, Y. Huang, C. Qin, Y. Liu, Z. H. Su and S. Z. Yao, Preparation of Au-film electrodes in glucose-containing Au-electroplating aqueous bath for high-performance nonenzymatic glucose sensor and glucose/O2 fuel cell, Electrochem. Commun., 2012, 18, 108–111 CrossRef CAS.
- M. Comotti, C. D. Pina, R. Matarrese and M. Rossi, The catalytic activity of “naked” gold particles, Angew. Chem., Int. Ed., 2004, 43, 5812–5815 CrossRef CAS.
- P. Beltrame, M. Comotti, C. Della Pina and M. A. Rossi, Oxidation of glucose II. Catalysis by colloidal gold, Appl. Catal., A, 2006, 297, 1–7 CrossRef CAS.
- W. J. Luo, C. F. Zhu, S. Su, D. Li, Y. He, Q. Huang and C. H. Fan, Self-catalyzed, self-limiting growth of glucose oxidase-mimicking gold nanoparticles, ACS Nano, 2010, 4, 7451–7458 CrossRef CAS.
- L. Clark Jr. and C. Lyons, Electrode systems for continuous monitoring in cardiovascular surgery, Ann. N. Y. Acad. Sci., 1962, 102, 29–45 CrossRef CAS.
- J. Wang, Glucose biosensors: 40 years of advances and challenges, Electroanalysis, 2001, 13, 983–988 CrossRef CAS.
- S. Updike and G. Hicks, The enzyme electrode, Nature, 1967, 214, 986–988 CrossRef CAS.
- G. Guilbault and G. Lubrano, An enzyme electrode for the amperometric determination of glucose, Anal. Chim. Acta, 1973, 64, 439–455 CrossRef CAS.
- G. Reach and G. S. Wilson, Can continuous glucose monitoring be used for the treatment of diabetes?, Anal. Chem., 1992, 64, 381A–386A CAS.
- D. Gough, J. Lucisano and P. Tse, Two-dimensional enzyme electrode sensor for glucose, Anal. Chem., 1985, 57, 2351–2357 CrossRef CAS.
- J. Armour, J. Lucisano and D. Gough, Application of chronic intravascular blood glucose sensor in dogs, Diabetes, 1990, 39, 1519–1526 CAS.
- J. Wang and F. Lu, Oxygen-rich oxidase enzyme electrodes for operation in oxygen-free solutions, J. Am. Chem. Soc., 1998, 120, 1048–1050 CrossRef CAS.
- J. Wang, J. W. Mo, S. F. Li and J. Porter, Comparison of oxygen-rich and mediator-based glucose-oxidase carbon-paste electrodes, Anal. Chim. Acta, 2001, 441, 183–189 CrossRef CAS.
- R. Kontani, S. Tsujimura and K. Kano, Air diffusion biocathode with CueO as electrocatalyst adsorbed on carbon particle-modified electrodes, Bioelectrochemistry, 2009, 76, 10–13 CrossRef CAS.
- F. Moussy, S. Jakeways, D. J. Harrison and R. V. Rajotte, In vitro and in vivo performance and lifetime of perfluorinated ionomer-coated glucose sensors after high-temperature curing, Anal. Chem., 1994, 66, 3882–3888 CrossRef CAS.
- S. Emr and A. Yacynych, Use of polymer films in amperometric biosensors, Electroanalysis, 1995, 7, 913 CrossRef CAS.
- C. Deng, M. Li, Q. Xie, M. Liu, Y. Tan, X. Xu and S. Yao, New glucose biosensor based on a poly(o-phenylendiamine)/glucose oxidase-glutaraldehyde/prussian blue/Au electrode with QCM monitoring of various electrode-surface modifications, Anal. Chim. Acta, 2006, 557, 85–94 CrossRef CAS.
- W. Zhao, J. J. Xu, C. G. Shi and H.-Y. Chen, Multilayer membranes via layer-by-layer deposition of organic polymer protected prussian blue nanoparticles and glucose oxidase for glucose biosensing, Langmuir, 2005, 21, 9630–9634 CrossRef CAS.
- J. Li, Y. Li, Y. Zhang and G. Wei, Highly sensitive molecularly imprinted electrochemical sensor based on the double amplification by an inorganic prussian blue catalytic polymer and the enzymatic effect of glucose oxidase, Anal. Chem., 2012, 84, 1888–1893 CrossRef CAS.
- H. Ohnuki, T. Saiki, A. Kusakari, H. Endo, M. Ichihara and M. Izumi, Incorporation of glucose oxidase into Langmuir–Blodgett films based on prussian blue applied to amperometric glucose biosensor, Langmuir, 2007, 23, 4675–4681 CrossRef CAS.
- C. Chen, Y. C. Fu, C. H. Xiang, Q. J. Xie, Q. F. Zhang, Y. H. Su, L. H. Wang and S. Z. Yao, Electropolymerization of preoxidized catecholamines on prussian blue matrix to immobilize glucose oxidase for sensitive amperometric biosensing, Biosens. Bioelectron., 2009, 24, 2726–2729 CrossRef CAS.
- Y. L. Yao and K. K. Shiu, Low potential detection of glucose at carbon nanotube modified glassy carbon electrode with electropolymerized poly(toluidine blue O) film, Electrochim. Acta, 2007, 53, 278–284 CrossRef CAS.
- J. Wang, Carbon-nanotube based electrochemical biosensors: A review, Electroanalysis, 2005, 17, 7–14 CrossRef CAS.
- Y. C. Fu, P. H. Li, Q. J. Xie, X. H. Xu, L. H. Lei, C. Chen, C. Zou, W. F. Deng and S. Z. Yao, One-pot preparation of polymer–enzyme–metallic nanoparticle composite films for high-performance biosensing of glucose and galactose, Adv. Funct. Mater., 2009, 19, 1–8 Search PubMed.
- L. Bahshi, M. Frasconi, R. Tel-Vered, O. Yehezkeli and I. Willner, Following the biocatalytic activities of glucose oxidase by electrochemically cross-linked enzyme-Pt nanoparticles composite electrodes, Anal. Chem., 2008, 80, 8253–8259 CrossRef CAS.
- J. Newman, S. White, I. Tothill and A. P. Turner, Catalytic materials, membranes, and fabrication technologies suitable for the construction of amperometric biosensors, Anal. Chem., 1995, 67, 4594–4599 CrossRef CAS.
- S. Hrapovic, Y. L. Liu, K. B. Male and J. H. T. Luong, Electrochemical biosensing platforms using platinum nanoparticles and carbon nanotubes, Anal. Chem., 2004, 76, 1083–1088 CrossRef CAS.
- J. M. Wu and L. W. Yin, Platinum nanoparticle modified polyaniline-functionalized boron nitride nanotubes for amperometric glucose enzyme biosensor, ACS Appl.
Mater. Interfaces, 2011, 3, 4354–4362 CAS.
- I. Willner and E. Katz, Integration of layered redox proteins and conductive supports for bioelectronic applications, Angew. Chem., Int. Ed., 2000, 39, 1180–1218 CrossRef.
- E. W. Stein, D. V. Volodkin, M. J. McShane and G. B. Sukhorukov, Real-time assessment of spatial and temporal coupled catalysis within polyelectrolyte microcapsules containing coimmobilized glucose oxidase and peroxidase, Biomacromolecules, 2006, 7, 710–719 CrossRef CAS.
- G. Cui, S. J. Kim, S. H. Choi, H. Nam and G. S. Cha, A disposable amperometric sensor screen printed on a nitrocellulose strip: A glucose biosensor employing lead oxide as an interference-removing agent, Anal. Chem., 2000, 72, 1925–1929 CrossRef CAS.
- Z. Y. Wang, S. N. Liu, P. Wu and C. X. Cai, Detection of glucose based on direct electron transfer reaction of glucose oxidase immobilized on highly ordered polyaniline nanotubes, Anal. Chem., 2009, 81, 1638–1645 CrossRef CAS.
- K. Wang, D. Zhang, T. Zhou and X. H. Xia, A dual-electrode approach for highly selective detection of glucose based on diffusion layer theory: Experiments and simulation, Chem.–Eur. J., 2005, 11, 1341–1347 CrossRef CAS.
- A. N. Sekretaryova, D. V. Vokhmyanina, T. O. Chulanova, E. E. Karyakina and A. A. Karyakin, Reagentless biosensor based on glucose oxidase wired by the mediator freely diffusing in enzyme containing membrane, Anal. Chem., 2012, 84, 1220–1223 CrossRef CAS.
- R. Rajagopalan, A. Aoki and A. Heller, A effect of quaternization of the glucose oxidase “wiring” redox polymer on the maximum current densities of glucose electrodes, J. Phys. Chem., 1996, 100, 3719–3727 CrossRef CAS.
- W. Schuhmann, T. J. Ohara, H. L. Schmidt and A. Heller, Electron-transfer between glucose oxidase and electrodes via redox mediators bound with flexible chains to the enzyme surface, J. Am. Chem. Soc., 1991, 113, 1394–1397 CrossRef CAS.
- F. Mao, N. Mano and A. Heller, Long tethers binding redox centers to polymer backbones enhance electron transport in enzyme “wiring” hydrogels, J. Am. Chem. Soc., 2003, 125, 4951–4957 CrossRef CAS.
- V. Flexer, E. S. Forzani, E. J. Calvo, S. J. Ludueña and L. I. Pietrasanta, Structure and thickness dependence of “molecular wiring” in nanostructured enzyme multilayers, Anal. Chem., 2006, 78, 399–407 CrossRef CAS.
- L. Z. Zheng, J. H. Li, J. P. Xu, L. Y. Xiong, D. Zheng, Q. Liu, W. Liu, Y. D. Li, S. M. Yang and J. Xia, Improvement of amperometric glucose biosensor by the immobilization of FcCD inclusive complex and carbon nanotube, Analyst, 2010, 135, 1339–1344 RSC.
- Y. M. Tan, Q. J. Xie, J. H. Huang, W. S. Duan, M. Ma and S. Z. Yao, Study on glucose biofuel cells using an electrochemical noise device, Electroanalysis, 2008, 20, 1599–1606 CrossRef CAS.
- C. Chen, L. H. Wang, Y. M. Tan, C. Qin, F. Y. Xie, Y. C. Fu, Q. J. Xie, J. H. Chen and S. Z. Yao, High-performance amperometric biosensors and biofuel cell based on chitosan-strengthened cast thin films of chemically synthesized catecholamine polymers with glucose oxidase effectively entrapped, Biosens. Bioelectron., 2011, 26, 2311–2316 CrossRef CAS.
- C. Qin, C. Chen, Q. J. Xie, L. H. Wang, X. H. He, Y. Huang, Y. P. Zhou, F. Y. Xie, D. W. Yang and S. Z. Yao, Amperometric enzyme electrodes of glucose and lactate based on poly(diallyldimethylammonium)-alginate-metal ion-enzyme biocomposites, Anal. Chim. Acta, 2012, 720, 49–56 CrossRef CAS.
- E. J. Calvo and R. Etchenique, Electrical communication between electrodes and enzymes mediated by redox hydrogels, Anal. Chem., 1996, 68, 4186–4193 CrossRef CAS.
- P. D. Hale, T. Inagaki, H. I. Karan, Y. Okamoto and T. A. Skotheim, A new class of amperometric biosensor incorporating a polymeric electron-transfer mediator, J. Am. Chem. Soc., 1989, 111, 3482–3484 CrossRef CAS.
- Y. Kajiya, H. Sugai, C. Iwakura and H. Yoneyama, Glucose sensitivity of polypyrrole films containing immobilized glucose oxidase and hydroquinonesulfonate ions, Anal. Chem., 1991, 63, 49–54 CrossRef CAS.
- L. Deng, Y. Liu, G. C. Yang, L. Shang, D. Wen, F. A. Wang, Z. A. Xu and S. J. Dong, Molecular “wiring” glucose oxidase in supramolecular architecture, Biomacromolecules, 2007, 8, 2063–2071 CrossRef CAS.
- T. J. Ohara, R. Rajagopalan and A. Heller, Glucose electrodes based on cross-linked bis(2,2(-bipyridine)chloroosmium(+/2+) complexed poly(1-vinylimidazole) films, Anal. Chem., 1993, 65, 3512–3517 CrossRef CAS.
- B. A. Gregg and A. Heller, Cross-linked redox gels containing glucose oxidase for amperometric biosensor applications, Anal. Chem., 1990, 62, 258–263 CrossRef CAS.
- N. Mano, J. E. Yoo, J. Tarver, Y. L. Loo and A. Heller, An electron-conducting cross-linked polyaniline-based redox hydrogel, formed in one step at pH 7.2, wires glucose oxidase, J. Am. Chem. Soc., 2007, 129, 7006–7007 CrossRef CAS.
- Y. M. Yan, I. Baravik, O. Yehezkeli and I. Willner, Integrated electrically contacted glucose oxidase/carbon nanotube electrodes for the bioelectrocatalyzed detection of glucose, J. Phys. Chem. C, 2008, 112, 17883–17888 CAS.
- P. Si, S. Ding, J. Yuan, X. W. D. Lou and D.-H. Kim, Hierarchically structured one-dimensional, TiO2 for protein immobilization, direct electrochemistry, and mediator-free glucose sensing, ACS Nano, 2011, 5, 7617–7626 CrossRef CAS.
- S. Alwarappan, C. Liu, A. Kumar and C. Z. Li, Enzyme-doped graphene nanosheets for enhanced glucose biosensing, J. Phys. Chem. C, 2010, 114, 12920–12924 CAS.
- S. Alwarappan, S. R. Singhd, S. Pillaid, A. Kumarab and S. Mohapatra, Direct electrochemistry of glucose oxidase at a gold electrode modified with graphene nanosheets, Anal. Lett., 2012, 45, 746–753 CrossRef CAS.
- I. Willner, V. Heleg-Shabtai, R. Blonder, E. Katz, G. Tao, A. F. Bückmann and A. Heller, Electrical wiring of glucose oxidase by reconstitution of FAD-modified monolayers assembled onto Au-electrodes, J. Am. Chem. Soc., 1996, 118, 10321–10322 CrossRef CAS.
- L. Fruk, C. H. Kuo, E. Torres and C. M. Niemeyer, Apoenzyme reconstitution as a chemical tool for structural enzymology and biotechnology, Angew. Chem., Int. Ed., 2009, 48, 1550–1574 CrossRef CAS.
- Y. Xiao, F. Patolsky, E. Katz, J. F. Hainfeld and I. Willner, “Plugging into enzymes” nanowiring of redox enzymes by a gold nanoparticle, Science, 2003, 299, 1877–1881 CrossRef CAS.
- F. Patolsky, Y. Weizmann and I. Willner, Long-range electrical contacting of redox enzymes by SWCNT connectors, Angew. Chem., Int. Ed., 2004, 43, 2113–2117 CrossRef CAS.
- M. Zayats, E. Katz and I. Willner, Electrical contacting of glucose oxidase by surface-reconstitution of the apo-protein on a relay-boronic acid-FAD cofactor monolayer, J. Am. Chem. Soc., 2002, 124, 2020–2021 Search PubMed.
- M. Zayats, B. Willner and I. Willner, Design of amperometric biosensors and biofuel cells by the reconstitution of electrically contacted enzyme electrodes, Electroanalysis, 2008, 20, 583–601 CrossRef CAS.
- H. O. Yehezkeli, S. Raichlin, R. Tel-Vered, E. Kesselman, D. Danino and I. Willner, Biocatalytic implant of Pt nanoclusters into glucose oxidase: A method to electrically wire the enzyme and to transform it from an oxidase to a hydrogenase, J. Phys. Chem. Lett., 2010, 1, 2816–2819 CrossRef.
- C. Shan, H. Yang, J. Song, D. Han, A. Ivaska and L. Niu, Direct electrochemistry of glucose oxidase and biosensing for glucose based on graphene, Anal. Chem., 2009, 81, 2378–2382 CrossRef CAS.
- Z. J. Wang, X. Z. Zhou, J. Zhang, F. Boey and H. Zhang, Direct electrochemical reduction of single-layer graphene oxide and subsequent functionalization with glucose oxidase, J. Phys. Chem. C, 2009, 113, 14071–14075 CAS.
- S. Alwarappan, S. Boyapalle, A. Kumar, C. Z. Li and S. Mohapatra, Comparative study of single-, few-, and multilayered graphene toward enzyme conjugation and electrochemical response, J. Phys. Chem. C, 2012, 116, 6556–6559 CAS.
- R. F. Gao and J. B. Zheng, Amine-terminated ionic liquid functionalized carbon nanotube-gold nanoparticles for investigating the direct electron transfer of glucose oxidase, Electrochem. Commun., 2009, 11, 608–611 CrossRef CAS.
- J. T. Holland, C. Lau, S. Brozik, P. Atanassov and S. Banta, Engineering of glucose oxidase for direct electron transfer via site-specific gold nanoparticle conjugation, J. Am. Chem. Soc., 2011, 133, 19262–19265 CrossRef CAS.
- C. Y. Deng, J. H. Chen, X. Chen, C. Xiao, L. H. Nie and S. Z. Yao, Direct electrochemistry of glucose oxidase and biosensing for glucose based on boron-doped carbon nanotubes modified electrode, Biosens. Bioelectron., 2008, 23, 1272–1277 CrossRef CAS.
- X. Liu, L. Shi, W. Niu, H. Li and G. Xu, Amperometric glucose biosensor based on single-walled carbon nanohorns, Biosens. Bioelectron., 2008, 23, 1887–1890 CrossRef CAS.
- Y. H. Su, Q. J. Xie, C. Chen, Q. F. Zhang, M. Ma and S. Z. Yao, Electrochemical quartz crystal microbalance studies on enzymatic specific activity and direct electrochemistry of immobilized glucose oxidase in the presence of sodium dodecyl benzene sulfonate and multiwalled carbon nanotubes, Biotechnol. Prog., 2008, 24, 262–272 CrossRef CAS.
- Y. Wang and Y. J. Yao, Direct electron transfer of glucose oxidase promoted by carbon nanotubes is without value in certain mediator-free applications, Microchim. Acta, 2012, 176, 271–277 CrossRef CAS.
- E. Rohde, E. Dempsey, M. R. Smyth, J. G. Vos and H. Emons, Development of a flow-through electrochemical detector for glucose based on a glucose oxidase-modified microelectrode incorporating redox and conducting polymer materials, Anal. Chim. Acta, 1993, 278, 5–16 CrossRef CAS.
- T. Ito, M. Kunimatsu, S. Kaneko, S. Ohya and K. Suzuki, Microfluidic device for the detection of glucose using a micro direct methanol fuel cell as an amperometric detection power source, Anal. Chem., 2007, 79, 1725–1730 CrossRef CAS.
- C. Bunte, O. Prucker, T. König and J. R. Rühe, Enzyme containing redox polymer networks for biosensors or biofuel cells: A photochemical approach, Langmuir, 2010, 26, 6019–6027 CrossRef CAS.
- C. X. He, J. H. Liu, L. Y. Xie, Q. L. Zhang, C. H. Li, D. Y. Gui, G. Z. Zhang and C. Wu, Activity and thermal stability improvements of glucose oxidase upon adsorption on core-shell PMMA-BSA nanoparticles, Langmuir, 2009, 25, 13456–13460 CrossRef CAS.
- J. Litt, C. Padala, P. Asuri, S. Vutukuru, K. Athmakuri, S. Kumar, J. Dordick and R. S. Kane, Enhancing protein stability by adsorption onto raftlike lipid domains, J. Am. Chem. Soc., 2009, 131, 7107–7111 CrossRef CAS.
- M. Wang, S. Bugarski and U. Stimming, Topological and electron-transfer properties of glucose oxidase adsorbed on highly oriented pyrolytic graphite electrodes, J. Phys. Chem. C, 2008, 112, 5165–5173 CAS.
- T.-W. Tsai, G. Heckert, L. F. Neves, Y. Tan, D.-Y. Kao, R. G. Harrison, D. E. Resasco and D. W. Schmidtke, Adsorption of glucose oxidase onto single-walled carbon nanotubes and its application in layer-by-layer biosensors, Anal. Chem., 2009, 81, 7917–7925 CrossRef CAS.
- S. Shrikrishnan, K. Sankaran and V. Lakshminarayanan, Electrochemical impedance analysis of adsorption and enzyme kinetics of calf intestine alkaline phosphatase on SAM-modified gold electrode, J. Phys. Chem. C, 2012, 116, 16030–16037 CAS.
- L. C. Sang, A. Vinu and M. O. Coppens, General description of the adsorption of proteins at their iso-electric point in nanoporous materials, Langmuir, 2011, 27, 13828–13837 CrossRef CAS.
- Y.-L. Lee, J.-Y. Lin and S. Lee, Adsorption behavior of glucose oxidase on a dipalmitoylphosphatic acid monolayer and the characteristics of the mixed monolayer at air/liquid interfaces, Langmuir, 2007, 23, 2042–2051 CrossRef CAS.
- J. L. Felhofer, J. D. Caranto and C. D. Garcia, Adsorption kinetics of catalase to thin films of carbon nanotubes, Langmuir, 2010, 26, 17178–17183 CrossRef CAS.
- K. P. Fears, B. Sivaraman, G. L. Powell, Y. Wu and R. A. Latour, Probing the conformation and orientation of adsorbed enzymes using side-chain modification, Langmuir, 2009, 25, 9319–9327 CrossRef CAS.
- K. P. Fears and R. A. Latour, Assessing the influence of adsorbed-state conformation on the bioactivity of adsorbed enzyme layers, Langmuir, 2009, 25, 13926–13933 CrossRef CAS.
- J. Méndez, A. Monteagudo and K. Griebenow, Stimulus-responsive controlled release system by covalent immobilization of an enzyme into mesoporous silica nanoparticles, Bioconjugate Chem., 2012, 23, 698–704 CrossRef.
- G. Fernandez-Lorente, C. A. Godoy, A. A. Mendes, F. Lopez-Gallego, V. Grazu, B. d. l. Rivas, J. M. Palomo, J. Hermoso, R. Fernandez-Lafuente and J. M. Guisan, Solid-phase chemical amination of a lipase from bacillus thermocatenulatus to improve its stabilization via covalent immobilization on highly activated glyoxyl-agarose, Biomacromolecules, 2008, 9, 2553–2561 CrossRef CAS.
- Y. Zhang and C. Ji, Electro-induced covalent cross-linking of chitosan and formation of chitosan hydrogel films: Its application as an enzyme immobilization matrix for use in a phenol sensor, Anal. Chem., 2010, 82, 5275–5281 CrossRef CAS.
- F. J. Xu, Q. J. Cai, Y. L. Li, E. T. Kang and K. G. Neoh, Covalent immobilization of glucose oxidase on well-defined poly(glycidyl methacrylate)-Si(111) hybrids from surface-initiated atom-transfer radical polymerization, Biomacromolecules, 2005, 6, 1012–1020 CrossRef CAS.
- D. Wan, S. J. Yuan, G. L. Li, K. G. Neoh and E. T. Kang, Glucose biosensor from covalent immobilization of chitosan-coupled carbon nanotubes on polyaniline-modified gold electrode, ACS Appl. Mater. Interfaces, 2010, 2, 3083–3091 CAS.
- P. Pandey, S. P. Singh, S. K. Arya, V. Gupta, M. Datta, S. Singh and B. D. Malhotra, Application of thiolated gold nanoparticles for the enhancement of glucose oxidase activity, Langmuir, 2007, 23, 3333–3337 CrossRef CAS.
- Y. M. Tan, W. F. Deng, C. Chen, Q. J. Xie, L. H. Lei, Y. Y. Li, Z. F. Fang, M. Ma, J. H. Chen and S. Yao, Immobilization of enzymes at high load/activity by aqueous electrodeposition of enzyme-tethered chitosan for highly sensitive amperometric biosensing, Biosens. Bioelectron., 2010, 25, 2644–2650 CrossRef CAS.
- Y. Huang, X. L. Qin, Z. Li, Y. C. Fu, C. Qin, F. Wu, Z. H. Su, M. Ma, Q. J. Xie, S. Z. Yao and J. M. Hu, Fabrication of a chitosan/glucose oxidase–poly(anilineboronic acid)–Aunano/Au-plated, Au electrode for biosensor and biofuel cell, Biosens. Bioelectron., 2012, 31, 357–362 CrossRef CAS.
- C. P. McMahon, G. Rocchitta, P. A. Serra, S. M. Kirwan, J. P. Lowry and R. D. O'Neill, Control of the oxygen dependence of an implantable polymer/enzyme composite biosensor for glutamate, Anal. Chem., 2006, 78, 2352–2359 CrossRef CAS.
- J. Li and X. Q. Lin, Glucose biosensor based on immobilization of glucose oxidase in poly(o-aminophenol) film on polypyrrole-Pt nanocomposite modified glassy carbon electrode, Biosens.
Bioelectron., 2007, 22, 2898–2905 CrossRef CAS.
- Z. E. Zhang, H. Y. Liu and J. Q. Deng, A glucose biosensor based on immobilization of glucose oxidase in electropolymerized o-aminophenol film on platinized glassy carbon electrode, Anal. Chem., 1996, 68, 1632–1638 CrossRef CAS.
- J. C. Vidal, S. Méndez and J. R. Castillo, Electropolymerization of pyrrole and phenylenediamine over an organic conducting salt based amperometric sensor of increased selectivity for glucose determination, Anal. Chim. Acta, 1999, 385, 203–211 CrossRef CAS.
- Y. C. Fu, C. Chen, Q. J. Xie, X. H. Xu, C. Zou, Q. M. Zhou, L. Tan, H. Tang, Y. Y. Zhang and S. Z. Yao, Immobilization of enzymes through one-pot chemical preoxidation and electropolymerization of dithiols in enzyme-containing aqueous suspensions to develop biosensors with improved performance, Anal. Chem., 2008, 80, 5829–5838 CrossRef CAS.
- H. He, Q. J. Xie and S. Z. Yao, An electrochemical quartz crystal impedance study on anti-human immunoglobulin G immobilization in the polymer grown during dopamine oxidation at an Au electrode, J. Colloid Interface Sci., 2005, 289, 446–454 CrossRef CAS.
- Y. M. Tan, W. F. Deng, Y. Y. Li, Z. Huang, Y. Meng, Q. J. Xie, M. Ma and S. Z. Yao, Polymeric bionanocomposite cast thin films with in situ laccase-catalyzed polymerization of dopamine for biosensing and biofuel cell applications, J. Phys. Chem. B, 2010, 114, 5016–5024 CrossRef CAS.
- Y. C. Fu, C. Zou, Q. J. Xie, X. H. Xu, C. Chen, W. F. Deng and S. Z. Yao, Highly sensitive glucose biosensor based on one-pot biochemical preoxidation and electropolymerization of 2,5-dimercapto-1,3,4-thiadiazole in glucose oxidase-containing aqueous suspension, J. Phys. Chem. B, 2009, 113, 1332–1340 CrossRef CAS.
- Y. Y. Li, Y. M. Tan, W. F. Deng, Q. J. Xie, Y. Y. Zhang, J. H. Chen and S. Z. Yao, Electropolymerization of catecholamines after laccase-catalyzed preoxidation to efficiently immobilize glucose oxidase for sensitive amperometric biosensing, Sens. Actuators, B, 2010, 151, 30–38 CrossRef.
- C. Zou, Y. C. Fu, Q. J. Xie and S. Z. Yao, High-performance glucose amperometric biosensor based on magnetic polymeric bionanocomposites, Biosens. Bioelectron., 2010, 25, 1277–1282 CrossRef CAS.
- V. Vamvakaki, K. Tsagaraki and N. Chaniotakis, Carbon nanofiber-based glucose biosensor, Anal. Chem., 2006, 78, 5538–5542 CrossRef CAS.
- Z. G. Zhu, L. Garcia-Gancedo, A. J. Flewitt, H. Q. Xie, F. Moussy and W. I. Milne, A critical review of glucose biosensors based on carbon nanomaterials: carbon nanotubes and graphene, Sensors, 2012, 12, 5996–6022 CrossRef.
- M. M. Rahman, A. J. S. Ahammad, J. H. Jin, S. J. Ahn and J. J. Lee, A comprehensive review of glucose biosensors based on nanostructured metal-oxides, Sensors, 2010, 10, 4855–4886 CrossRef CAS.
- A. S. Rad, A. Mirabi, E. Binaian and H. Tayebi, A review on glucose and hydrogen peroxide biosensor based on modified electrode included silver nanoparticles, Int. J. Electrochem. Sci., 2011, 6, 3671–3683 CAS.
- M. Iwamoto, S. Tokonami, H. Shiigi and T. Nagaoka, Activity enhancement of a screen-printed carbon electrode by modification with gold nanoparticles for glucose determination, Res. Chem. Intermed., 2009, 35, 919–930 CrossRef CAS.
- H. J. Qiu, L. Li, Q. L. Lang, F. X. Zou and X. R. Huang, Aligned nanoporous PtNi nanorod-like structures for electrocatalysis and biosensing, RSC Adv., 2012, 2, 3548–3554 RSC.
- R. B. Rakhi, K. Sethupathi and S. Ramaprabhu, A glucose biosensor based on deposition of glucose oxidase onto crystalline gold nanoparticle modified carbon nanotube electrode, J. Phys. Chem. B, 2009, 113, 3190–3194 CrossRef CAS.
- D. Pradhan, F. Niroui and K. T. Leung, High-performance, flexible enzymatic glucose biosensor based on ZnO nanowires supported
on a gold-coated polyester substrate, ACS Appl. Mater. Interfaces, 2010, 2, 2409–2412 CAS.
- Z. Y. Yang, J. S. Feng, J. S. Qiao, Y. Yan, Q. Y. Yu and K. N. Sun, Copper oxide nanoleaves decorated multi-walled carbon nanotube as platform for glucose sensing, Anal. Methods, 2012, 4, 1924–1926 RSC.
- M. C. Tsai and Y. C. Tsai, Adsorption of glucose oxidase at platinum-multiwalled carbon nanotube-alumina-coated silica nanocomposite for amperometric glucose biosensor, Sens. Actuators, B, 2009, 141, 592–598 CrossRef.
- M. J. Song, S. W. Hwang and D. Whang, Amperometric glucose biosensor based on a Pt-dispersed hierarchically porous electrode, J. Korean Phys. Soc., 2009, 54, 1612–1618 CrossRef CAS.
- H. Zhong, R. Yuan, Y. Chai, W. Li, X. Zhong and Y. Zhang, In situ chemo-synthesized multi-wall carbon nanotube-conductive polyaniline nanocomposites: Characterization and application for a glucose amperometric biosensor, Talanta, 2011, 85, 104–111 CrossRef CAS.
- L. H. Xu, Y. H. Zhu, X. L. Yang and C. Z. Li, Amperometric biosensor based on carbon nanotubes coated with polyaniline/dendrimer-encapsulated Pt nanoparticles for glucose detection, Mater. Sci. Eng., C, 2009, 29, 1306–1310 CrossRef CAS.
- C. Y. Wang, X. R. Tan, S. H. Chen, F. X. Hu, H. A. Zhong and Y. Zhang, The construction of glucose biosensor based on platinum nanoclusters-multiwalled carbon nanotubes nanocomposites, Appl. Biochem. Biotechnol., 2012, 166, 889–902 CrossRef CAS.
- Y. Wang, R. Yuan, Y. Q. Chai, W. J. Li, Y. Zhuo, Y. Yuan and J. J. Li, Direct electron transfer: Electrochemical glucose biosensor based on hollow Pt nanosphere functionalized multiwall carbon nanotubes, J. Mol. Catal. B: Enzym., 2011, 71, 146–151 CrossRef CAS.
- P. Si, P. Kannan, L. H. Guo, H. Son and D. H. Kim, Highly stable and sensitive glucose biosensor based on covalently assembled high density Au nanostructures, Biosens. Bioelectron., 2011, 26, 3845–3851 CrossRef CAS.
- V. A. Pedrosa, J. Yan, A. L. Simonian and A. Revzin, Micropatterned nanocomposite hydrogels for biosensing applications, Electroanalysis, 2011, 23, 1142–1149 CrossRef CAS.
- S. Sharma, N. Gupta and S. Srivastava, Modulating electron transfer properties of gold nanoparticles for efficient biosensing, Biosens. Bioelectron., 2012, 37, 30–37 CrossRef CAS.
- R. B. Rakhi, K. Sethupathi and S. Ramaprabhu, A glucose biosensor based on deposition of glucose oxidase onto crystalline gold nanoparticle modified carbon nanotube electrode, J. Phys. Chem. B, 2009, 113, 3190–3194 CrossRef CAS.
- J. H. Lin, C. Y. He, Y. Zhao and S. S. Zhang, One-step synthesis of silver nanoparticles/carbon nanotubes/chitosan film and its application in glucose biosensor, Sens. Actuators, B, 2009, 137, 768–773 CrossRef.
- X. J. Yang, J. Bai, Y. H. Wang, X. E. Jiang and X. Y. He, Hydrogen peroxide and glucose biosensor based on silver nanowires synthesized by polyol process, Analyst, 2012, 137, 4362–4367 RSC.
- G. H. Chang, Y. L. Luo, W. B. Lu, X. Y. Qin, A. M. Asiri, A. O. Al-Youbi and X. P. Sun, Ag nanoparticles decorated polyaniline nanofibers: Synthesis, characterization, and applications toward catalytic reduction of 4-nitrophenol and electrochemical detection of H2O2 and glucose, Catal. Sci. Technol., 2012, 2, 800–806 CAS.
- P. Gomathi, K. Min, P. Kwan, D. Je Jung, A. Ragupathy, L. Rajendran, S. Chool, K. Jae Chang, L. S. Hak and G. Han, Do Multiwalled carbon nanotubes grafted chitosan nanobiocomposite: A prosperous functional nanomaterials for glucose biosensor application, Sens. Actuators, B, 2011, 155, 897–902 CrossRef.
- G. L. Fu, X. L. Yue and Z. F. Dai, Glucose biosensor based on covalent immobilization of enzyme in sol–gel composite film combined with prussian blue/carbon
nanotubes hybrid, Biosens. Bioelectron., 2011, 26, 3973–3976 CrossRef CAS.
- J. J. Li, R. Yuan, Y. Q. Chai and X. Che, Fabrication of a novel glucose biosensor based on Pt nanoparticles-decorated iron oxide-multiwall carbon nanotubes magnetic composite, J. Mol. Catal. B: Enzym., 2010, 66, 8–14 CrossRef CAS.
- X. Che, R. Yuan, Y. Q. Chai, J. J. Li, Z. J. Song, W. J. Li and X. Zhong, A glucose biosensor based on chitosan-prussian blue-multiwall carbon nanotubes-hollow PtCo nanochains formed by one-step electrodeposition, Colloids Surf., B, 2011, 84, 454–461 CrossRef CAS.
- K. J. Chen, C. F. Lee, J. Rick, S. H. Wang, C. C. Liu and B. J. Hwang, Fabrication and application of amperometric glucose biosensor based on a novel PtPd bimetallic nanoparticle decorated multi-walled carbon nanotube catalyst, Biosens. Bioelectron., 2012, 33, 75–81 CrossRef CAS.
- T. T. Baby and S. Ramaprabhu, SiO2 coated Fe3O4 magnetic nanoparticle dispersed multiwalled carbon nanotubes based amperometric glucose biosensor, Talanta, 2010, 80, 2016–2022 CrossRef CAS.
- X. Pang, P. Imin, I. Zhitomirsky and A. Adronov, Conjugated polyelectrolyte complexes with single-walled carbon nanotubes for amperometric detection of glucose with inherent anti-interference properties, J. Mater. Chem., 2012, 22, 9147–9154 RSC.
- Q. Zeng, J. S. Cheng, X. F. Liu, H. T. Bai and J. H. Jiang, Palladium nanoparticle/chitosan-grafted graphene nanocomposites for construction of a glucose biosensor, Biosens. Bioelectron., 2011, 26, 3456–3463 CrossRef CAS.
- H. D. Jang, S. K. Kim, H. Chang, K.-M. Roh, J.-W. Choi and J. Huang, A glucose biosensor based on TiO2-graphene composite, Biosens. Bioelectron., 2012, 38, 184–188 CrossRef CAS.
- T. T. Baby, S. S. J. Aravind, T. Arockiadoss, R. B. Rakhi and S. Ramaprabhu, Metal decorated graphene nanosheets as immobilization matrix for amperometric glucose biosensor, Sens. Actuators, B, 2010, 145, 71–77 CrossRef.
- J. D. Qiu, J. Huang and R. P. Liang, Nanocomposite film based on graphene oxide for high performance flexible glucose biosensor, Sens. Actuators, B, 2011, 160, 287–294 CrossRef CAS.
- J. F. Ping, Y. X. Wang, K. Fan, J. Wu and Y. B. Ying, Direct electrochemical reduction of graphene oxide on ionic liquid doped screen-printed electrode and its electrochemical biosensing application, Biosens. Bioelectron., 2011, 28, 204–209 CrossRef CAS.
- Z. M. Luo, L. H. Yuwen, Y. J. Han, J. Tian, X. R. Zhu, L. X. Weng and L. H. Wang, Reduced graphene oxide/PAMAM-silver nanoparticles nanocomposite modified electrode for direct electrochemistry of glucose oxidase and glucose sensing, Biosens. Bioelectron., 2012, 36, 179–185 CrossRef CAS.
- J. Yang, S. Y. Deng, J. P. Lei, H. X. Ju and G. Sundaram, Electrochemical synthesis of reduced graphene sheet-AuPd alloy nanoparticle composites for enzymatic biosensing, Biosens. Bioelectron., 2011, 29, 159–166 CrossRef CAS.
- M. Ahmad, C. Pan, Z. Luo and J. Zhu, A single ZnO nanofiber-based highly sensitive amperometric glucose biosensor, J. Phys. Chem. C, 2010, 114, 9308–9313 CAS.
- C. X. Guo and C. M. Li, Direct electron transfer of glucose oxidase and biosensing of glucose on hollow sphere-nanostructured conducting polymer/metal oxide composite, Phys. Chem. Chem. Phys., 2010, 12, 12153–12159 RSC.
- C. J. Cai, M. W. Xu, S. J. Bao, C. Lei and D. Z. Jia, A facile route for constructing a graphene-chitosan-ZrO2 composite for direct electron transfer and glucose sensing, RSC Adv., 2012, 2, 8172–8178 RSC.
- J. Lu, L. T. Drzal, R. M. Worden and I. Lee, Simple fabrication of a highly sensitive glucose biosensor using enzymes immobilized in exfoliated graphite nanoplatelets nafion membrane, Chem. Mater., 2007, 19, 6240–6246 CrossRef CAS.
- S. Su, Y. He, S. P. Song, D. Li, L. Wang, C. H. Fan and S.-T. Lee, A silicon nanowire-based electrochemical glucose biosensor with high electrocatalytic activity and sensitivity, Nanoscale, 2010, 2, 1704–1707 RSC.
- Q. Wang, W. Xu, P. Wu, H. Zhang, C. Cai and B. Zhao, New insights into the effects of thermal treatment on the catalytic activity and conformational structure of glucose oxidase studied by electrochemistry, IR spectroscopy, and theoretical calculation, J. Phys. Chem. B, 2010, 114, 12754–12764 CrossRef CAS.
- U. B. Jensen, E. E. Ferapontova and D. S. Sutherland, Quantifying protein adsorption and function at nanostructured materials: Enzymatic activity of glucose oxidase at glad structured electrodes, Langmuir, 2012, 28, 11106–11114 CrossRef CAS.
- C. Bourdillon, C. Demaille, J. Gueris. J. Moiroux and J. M. Saveant, A fully active monolayer enzyme electrode derivatized by antigen-antibody attachment, J. Am. Chem. Soc., 1993, 115, 12264–12269 CrossRef CAS.
- T. K. v. Krawczyk, M. Moszczyńska and M. Trojanowicz, Inhibitive determination of mercury and other metal ions by potentiometric urea biosensor, Biosens. Bioelectron., 2000, 15, 681–691 CrossRef.
- A. L. Kukla, N. I. Kanjuk, N. F. Starodub and Y. M. Shirshov, Multienzyme electrochemical sensor array for determination of heavy metal ions, Sens. Actuators, B, 1999, 57, 213–218 CrossRef.
- D. Bagal-Kestwal, M. S. Karvea, B. Kakade and V. K. Pillai, Invertase inhibition based electrochemical sensor for the detection of heavy metal ions in aqueous system: Application of ultra-microelectrode to enhance sucrose biosensor's sensitivity, Biosens. Bioelectron., 2008, 24, 657–664 CrossRef CAS.
- S. Cosnier, C. Mousty, X. Q. Cui, X. R. Yang and S. J. Dong, Specific determination of As(v) by an acid phosphatase-polyphenol oxidase biosensor, Anal. Chem., 2006, 78, 4985–4989 CrossRef CAS.
- M. E. Ghica and C. M. A. Brett, Glucose oxidase inhibition in poly(neutral red) mediated enzyme biosensors for heavy metal determination, Microchim. Acta, 2008, 163, 185–193 CrossRef CAS.
- S. B. Han, M. Zhu, Z. B. Yuan and X. Li, A methylene blue-mediated enzyme electrode for the determination of trace mercury(II), mercury(I), methylmercury, and mercury–glutathione complex, Biosens. Bioelectron., 2001, 16, 9–16 CrossRef CAS.
- H. C. Tsai and R. A. Doong, Simultaneous determination of pH, urea, acetylcholine and heavy metals using array-based enzymatic optical biosensor, Biosens. Bioelectron., 2005, 20, 1796–1804 CrossRef CAS.
- C. Malitesta and M. R. Guascito, Heavy metal determination by biosensors based on enzyme immobilised by electropolymerisation, Biosens. Bioelectron., 2005, 20, 1643–1647 CrossRef CAS.
- M. R. Guascito, C. Malitesta, E. Mazzotta and A. Turco, Inhibitive determination of metal ions by an amperometric glucose oxidase biosensor study of the effect of hydrogen peroxide decomposition, Sens. Actuators, B, 2008, 131, 394–402 CrossRef.
- A. Amine, H. Mohammadi, I. Bourais and G. Palleschi, Enzyme inhibition-based biosensors for food safety and environmental monitoring, Biosens. Bioelectron., 2006, 21, 1405–1423 CrossRef CAS.
- C. Chen, Q. J. Xie, L. H. Wang, C. Qin, F. Y. Xie, S. Z. Yao and J. H. Chen, Experimental platform to study heavy metal ion–enzyme interactions and amperometric inhibitive assay of Ag+ based on solution state and immobilized glucose oxidase, Anal. Chem., 2011, 83, 2660–2666 CrossRef CAS.
- E. S. Wilkins, Towards implantable glucose sensors-a review, J. Biomed. Eng., 1989, 11, 354–361 CrossRef CAS.
- M. Gerritsen, J. A. Jansen, A. Kros, R. J. M. Nolte and J. A. Lutterman, Performance of subcutaneously implanted glucose sensors: A review, J. Invest. Surg., 1998, 11, 163–174 CrossRef CAS.
- A. Erlenkötter, M. Kottbus and G. C. Chemnitius, Flexible amperometric transducers for biosensors based on a screen printed three electrode system, J. Electroanal. Chem., 2000, 481, 82–94 CrossRef.
- J. D. Newman and A. P. F. Turner, Home blood glucose biosensors: A commercial perspective, Biosens. Bioelectron., 2005, 20, 2435–2453 CrossRef CAS.
- J. Hu, The evolution of commercialized glucose sensors in China, Biosens. Bioelectron., 2009, 24, 1083–1089 CrossRef CAS.
- M. J. Moehlenbrock, R. L. Arechederra, K. H. Sjöholm and S. D. Minteer, Analytical techniques for characterizing enzymatic biofuel cells, Anal. Chem., 2009, 81, 9538–9545 CrossRef CAS.
- S. C. Barton, J. Gallaway and P. Atanassov, Enzymatic biofuel cells for implantable and microscale devices, Chem. Rev., 2004, 104, 4867–4886 CrossRef CAS.
- I. Willner, Y. M. Yan, B. Willner and R. Tel-Vered, Integrated enzyme-based biofuel cells-a review, Fuel Cells, 2009, 9, 7–24 CrossRef CAS.
- Y. M. Tan, W. F. Deng, B. Ge, Q. J. Xie, J. H. Huang and S. Z. Yao, Biofuel cell and phenolic biosensor based on acid-resistant laccase–glutaraldehyde functionalized chitosan–multiwalled carbon nanotubes nanocomposite film, Biosens. Bioelectron., 2009, 24, 2225–2231 CrossRef CAS.
- F. Tasca, L. Gorton, W. Harreither, D. Haltrich, R. Ludwig and G. Nöll, Highly efficient and versatile anodes for biofuel cells based on cellobiose dehydrogenase from myriococcum thermophilum, J. Phys. Chem. C, 2008, 112, 13668–13673 CAS.
- M. Zhou, Y. Du, C. G. Chen, B. L. Li, D. Wen, S. J. Dong and E. Wang, Aptamer-controlled biofuel cells in logic systems and used as self-powered and intelligent logic aptasensors, J. Am. Chem. Soc., 2010, 132, 2172–2174 CrossRef CAS.
- M. Zhou and S. J. Dong, Bioelectrochemical interface engineering: Toward the fabrication of electrochemical biosensors, biofuel cells, and self-powered logic biosensors, Acc. Chem. Res., 2011, 44, 1232–1243 CrossRef CAS.
- Z. H. Liu, B. Cho, T. M. Ouyang and B. Feldman, Miniature amperometric self-powered continuous glucose sensor with linear response, Anal. Chem., 2012, 84, 3403–3409 CrossRef CAS.
- M. T. Meredith and S. D. Minteer, Inhibition and activation of glucose oxidase bioanodes for use in a self-powered EDTA sensor, Anal. Chem., 2011, 83, 5436–5441 CrossRef CAS.
- L. Deng, C. G. Chen, M. Zhou, S. J. Guo, E. Wang and S. Dong, Integrated self-powered microchip biosensor for endogenous biological cyanide, Anal. Chem., 2010, 82, 4283–4287 CrossRef CAS.
- E. Katz, A. F. Bückmann and I. Willner, Self-powered enzyme-based biosensors, J. Am. Chem. Soc., 2001, 123, 10752–10753 CrossRef CAS.
- M. Zhou and J. Wang, Biofuel cells for self-powered electrochemical biosensing and logic biosensing: A review, Electroanalysis, 2012, 24, 197–209 CrossRef CAS.
- D. J. Caruana and S. Howorka, Biosensors and biofuel cells with engineered proteins, Mol. BioSyst., 2010, 6, 1548–1556 RSC.
- M. J. Cooney, V. Svoboda, C. Lau, G. Martina and S. D. Minteer, Enzyme catalysed biofuel cells, Energy Environ. Sci., 2008, 1, 320–337 CAS.
- M. J. Moehlenbrock and S. D. Minteer, Extended lifetime biofuel cells, Chem. Soc. Rev., 2008, 37, 1188–1196 RSC.
|
This journal is © The Royal Society of Chemistry 2013 |
Click here to see how this site uses Cookies. View our privacy policy here.