S-doping Fe–Ce composites derived from PBA to accelerate Fe(III)/Fe(II) cycle in the Fenton-like process†
Received
1st November 2023
, Accepted 29th November 2023
First published on 30th November 2023
Abstract
The design of metal–organic framework-derived metal oxides/sulfides is an effective approach for water remediation. A series of Fe–Ce composites (Fe–Ce–S–N) with cluster-like morphology were prepared with prussian blue analogue (PBA) as the precursor in a hydrothermal process. S doping promoted the conversion of Fe(III) to Fe(II), which greatly improved the performance of the Fenton-like reaction. The optimized Fe–Ce–S–N catalyst exhibited superior catalytic activity towards sulfamethoxazole (SMZ) degradation (18.63 mgSMZ gcat−1 min−1), showing the largest k value (0.1862 min−1), which was 2.1 and 8.1 times higher than that of Fe–Ce–S–H and Fe–Ce–N, respectively. Moreover, the Fe–Ce–S–N catalyst displayed a remarkable reusability, stability and adaptability in Fenton-like systems. Overall, this work provides new insights into extending novel PBA-derived catalysts to significantly improve heterogeneous Fenton-like performances.
Environmental significance
Facilitating the reductive regeneration of catalyst metal centers to maintain a stable high catalytic activity is quite a challenge for most Fenton-like processes. The synthesized Fe–Ce–S–N had a three-dimensional cluster-like morphology that favors the exposure of more active sites, while the S doping facilitated the acceleration of the electron transfer in the Fenton process and promoted the conversion of Fe(III) to Fe(II), which greatly improved the performance of the Fenton-like reaction. In addition, the Fe–Ce–S–N/H2O2 system showed excellent catalytic activity and stability over a wide pH range and in the presence of coexisting substances. In conclusion, our work provided a new strategy for designing PBA-derived catalysts for wastewater treatment.
|
1. Introduction
Sulfamethoxazole (SMZ) is a sulfonamide antibiotic widely used in human and animal medicine.1 However, due to its limited bioavailability, over 70% of SMZ is excreted into the natural environment through urine and feces. The discharge of SMZ into aquatic environments poses a significant threat to both human health and environmental safety, given its non-volatility and poor biodegradability.2,3 Therefore, there is a high demand for an efficient method to remove SMZ from water.
Notably, since Fenton found that iron could activate H2O2 to degrade tartaric acid (TA), Fenton and Fenton-like reactions have drawn remarkable attention because of their facile operation, no requirement for extra energy and low cost of H2O2.4–6 However, the disadvantages of homogeneous Fenton reaction including acidic reaction conditions, large consumption of ferrous salts and oxidants, unsatisfied kinetics of iron cycle and iron sludge generation have limited its application. In comparison, the heterogeneous Fenton process has emerged as a prominent research direction in recent years. Nevertheless, the heterogeneous Fenton efficiencies were not desirable because of several inherent shortcomings, such as the insufficient active sites and low H2O2 activation efficiency.7–9
In this case, the primary concern for heterogeneous Fenton technology lies in the development of a catalyst that boasts abundant active sites and high efficiency in generating active species. To this end, researchers have successfully developed an array of novel catalysts by regulating their morphology, composition, facets and surface sites to activate H2O2 into free radicals for organic pollutant removal.10–12 Among these catalysts, Fe-based oxide/sulfide was commonly employed for activating H2O2 due to the intricate structure and variable valence. However, their catalytic abilities may be limited by the inferior stability and low efficiency of the iron cycle.13–15 Therefore, it is crucial to expedite the Fe(II)/Fe(III) cycle and enhance catalyst stability. Constructing bimetallic oxide/sulfide not only possesses a more diverse composition and valence states, leading to a variety of redox reactions, but also can significantly improve the catalytic activity and material stability. Prussian blue analogue (PBA) has the coordination framework structure with cyano-linked bimetallic centers, which can be employed as the desirable template for preparing bimetallic oxide/sulfide due to the ordered crystal structure, large surface area and tunability.16–18
Based on of previous work,19 we found that the partial replacement of Fe with Ce could greatly improve the adsorption affinity of the catalyst for H2O2. However, the obtained Fe–Ce-PBA could not maintain the high catalytic efficiency due to leakage. Inspired by the aforementioned analysis, a novel Fe–Ce composite (composed of FeS, CeS and FeCe2O4) was synthesized through facile sulfurization of PBA using the hydrothermal process. Then, the effects of different reaction conditions in Fenton-like system were thorough investigated. Furthermore, the stability of the catalyst was verified and the degradation pathways and mechanisms of SMZ were elucidated. The Fe–Ce composite/H2O2 system exhibited extensive degradation capabilities towards SMZ as well as many other organic pollutants.
2. Experimental
2.1. Chemicals
The chemicals used in this work were as follows: sulfamethoxazole (SMZ, Aladdin), H2O2 solution (30% w/v, Aladdin), potassium dihydrogen phosphate (KH2PO4, Aladdin), 5,5-dimethyl-1-pyrroline N-oxide (DMPO, Sinopharm), nitroblue tetrazolium (NBT, Sinopharm), tert-butyl alcohol (TBA, Sinopharm), potassium ferricyanide (K3[Fe(CN)6], Sinopharm), cerous chloride (CeCl3, Sinopharm), sublimed sulfur (S, Sinopharm), hydrazine hydrate (H2N4·H2O, Sinopharm). All chemicals were used without further purification.
2.2. Synthesis of Fe–Ce composites
Fe–Ce-PBA was synthesized following the procedure outlined in our previous publication.19 A solution of Fe–Ce-PBA (0.1 g) was dispersed completely by sonication in a mixture of hydrazine hydrate (4 mL) and deionized water (6 mL). Subsequently, a specific amount of sulfur powder (the mass ratio of Fe–Ce-PBA to S powder: 3
:
1) was added to the dispersion and vigorously stirred for 2 h before being transferred to a Teflon-lined steel autoclave with a capacity of 50 mL. The autoclave was heated to 160 °C and maintained at that temperature for 5 h. The obtained Fe–Ce composites (designated as Fe–Ce–S–N) were collected, washed with deionized water and ethyl alcohol multiple times, and dried overnight in a vacuum at 60 °C. Fe–Ce–N and Fe–Ce–S–H were synthesized by the same process as Fe–Ce–S–N, with the difference that sulfur powder or hydrazine hydrate was not used, respectively (Scheme 1).
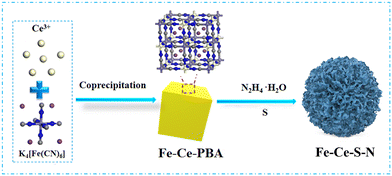 |
| Scheme 1 The preparation processes of Fe–Ce–S–N. | |
2.3. Experiment procedures
In a typical Fenton-like experiment, 10 mg of the prepared catalyst was added to 50 mL of target pollutant solution (20 mg L−1, pH = 5.0) and dispersed by ultrasound for 5 minutes. The sample solution was then stirred for an additional 30 minutes to establish adsorption–desorption equilibrium before introducing a specific amount of H2O2 into the reaction system. At certain time intervals, 0.8 mL of the sample solution was extracted and filtered through a needle filter with a pore size of 0.22 μm before being thoroughly mixed with methanol.
The SMZ concentration was determined using high-performance liquid chromatography (HPLC) equipped with a C18 column and a UV-vis detector. The column temperature was maintained at 30 °C, and the injection volume was set to 10 μL. A mobile phase consisting of KH2PO4 solution (pH 3.0, 5 mmol L−1)/acetonitrile (V/V = 60/40) was used at a flow rate of 1.0 mL min−1, while detection wavelength was set at 270 nm with peak time around 5.5 min. Total organic carbon (TOC) analysis of the sample solution was performed using a TOC analyzer (Multi N/C 3100). Inductively coupled plasma atomic emission spectroscopy (ICP-AES) was used to measure the leaching metal ions in the sample solution. The concentration of H2O2 was determined spectrophotometrically using potassium titanium oxalate, the absorbance of the sample was measured at the maximum absorption wavelength of 386 nm. The fluorescence spectroscopy (FS, FP-6500) was used to semi-quantify the concentration of ·OH produced. Terephthalic acid reacted with ·OH to form 2-hydroxyterephthalic acid and showed fluorescence intensity at a wavelength of 426 nm, and the concentration of ·OH produced was proportional to the peak intensity.19
2.4. Characterization
The microstructure images of prepared samples were recorded on a Field emission scanning electron microscope (FESEM, SU8220, Hitachi, Japan) and high-resolution transmission electron microscopy (HRTEM, TF20, Joel 2100F). X-ray diffraction (XRD) patterns of the prepared catalysts were analyzed on a diffractometer (D8 Advance, Bruker) using Cu Kα radiation. The elemental composition and chemical oxidation state of the samples was determined by X-ray photoelectron spectroscopy (XPS, Escalab 250Xi, Thermo Fisher Scientific). The electron spin resonance (ESR) signals of ·OH and ·O2− spin trapped were performed on a spectrometer (ESR E500, Bruker) by spin-trap reagent of DMPO.
3. Results and discussion
3.1. Characterization
To assess the effect of hydrazine hydrate and sulfur powder on catalyst morphology evolution, the morphology and composition of Fe–Ce–S–N were further characterized by TEM and SEM. Compared to the typical cubic structure of Fe–Ce–S–H (Fig. S1a and b) and the sheet-like structure of Fe–Ce–N (Fig. S1c and d), Fe–Ce–S–N (Fig. S1e and f) showed a more fluffy and dispersed cluster-like structure, which was more favorable for the exposure of active sites and accelerated diffusion and mass transfer. Moreover, the lattice spacing of 0.330 nm and 0.191 nm corresponded to the (111), (220) crystallographic planes of FeS and CeS in Fe–Ce–S–N (Fig. 1b). EDS analysis showed that the surface of Fe–Ce–S–N contains five elements: Fe, Ce, N, O and S (Fig. 3c). The diffraction peaks in the XRD pattern of Fe–Ce–S–N (Fig. 1d) were assigned to the typical diffractions of FeS, CeS and FeCe2O4, which was consistent with EDS mapping.
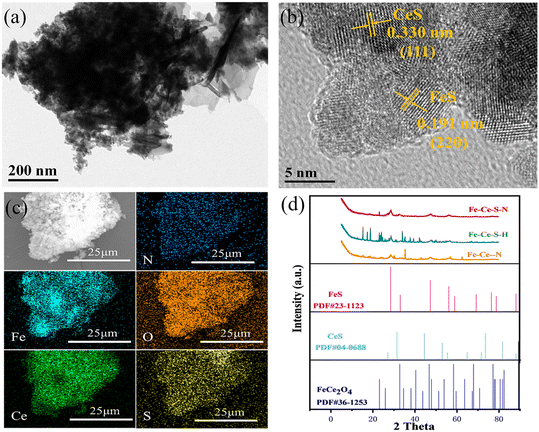 |
| Fig. 1 (a) TEM image, (b) HRTEM image of Fe–Ce–S–N and (c) EDS mapping of N, Fe, Ce, O and S; (d) XRD spectra of Fe–Ce–S–N, Fe–Ce–S–H and Fe–Ce–N. | |
Fig. 2a displayed the XPS spectra of Fe–Ce–N, Fe–Ce–S–H, and Fe–Ce–S–N. The Fe–Ce–S–N displayed obvious S 2p peaks compared with Fe–Ce–S–H (Fig. 2b), which illustrated the positive role of hydrazine hydrate in the introduction of S. Meanwhile, the S 2p high-resolution spectra of Fe–Ce–S–N showed three deconvolution peaks. The peaks at 166.8 eV and 163.8 eV were assigned to metal–S bonds, and the peak at 172.4 eV was ascribed to sulfur atoms of intermediate oxidation state.20 As described in Fig. 2c, both Fe 2p3/2 and Fe 2p1/2 could be divided into double peaks, which corresponded to Fe(II) and Fe(III), respectively.21 The XPS spectrum of Ce 3d could be categorized into two groups (v and u), corresponding to the core-level spin–orbit splitting of Ce 3d3/2 and Ce 3d5/2, respectively (Fig. 2d). The peaks including v1, v3, v4, u1, u3 and u4 corresponded to the Ce(IV) state while the remaining two peaks referred to the Ce(III) state.22 The effective formation of metal–S bonds in Fe–Ce–S–N indicated that hydrazine hydrate not only created an alkaline environment to facilitate the decomposition of the precursor PBA, but also promoted as a reducing agent to reduce the S powder to S2−, thus facilitating the formation of metal sulfides.23
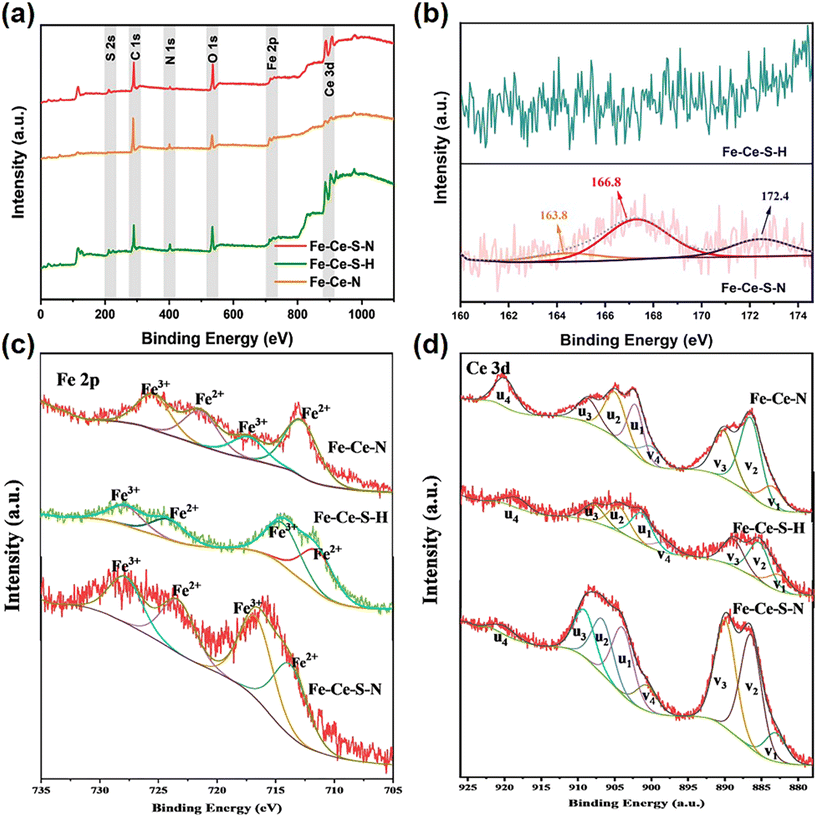 |
| Fig. 2 XPS spectra of Fe–Ce–S–N, Fe–Ce–S–H and Fe–Ce–N: (a) survey; (b) high-resolution of S 2p, (c) Fe 2p and (d) Ce 3d. | |
3.2. Catalytic activities assessment towards SMZ degradation
A series of experiments with different systems were conducted to evaluate the performance of Fe–Ce–S–N in degrading organic pollutants. As shown in Fig. 3a, the removal rate of SMZ by H2O2 or Fe–Ce–S–N system alone was minimal. The Fe–Ce–S–N/H2O2 system showed the best degradation efficiency for SMZ compared to other systems. Specifically, the Fe–Ce–N/H2O2 system showed a 34.5% decrease in the removal rate of SMZ within 30 min compared to the Fe–Ce-PBA/H2O2 system (Fig. 3a). The removal rate of SMZ by the Fe–Ce–N/H2O2 system was negative under the modification of hydrazine hydrate only. This might be due to the fact that the collapsed PBA framework obscured most of the active sites and hindered the mass transfer process. However, the removal rate of SMZ by Fe–Ce–S–H and Fe–Ce–S–N increased by 8.9% and 20.8%, respectively, after sulfidation, suggesting that S doping played a facilitating role in pollutant degradation. Among them, the k value of Fe–Ce–S–N was as high as 0.1863 min−1, which was 2.1 and 8.1 times higher than that of Fe–Ce–S–H and Fe–Ce–N, respectively. (Fig. S2a†). It was noteworthy that the Fe and Ce leaching concentrations in the Fe–Ce–S–N structure after the reaction were 0.56 mg L−1 and 0.17 mg L−1, respectively, which were only 30.1% and 23.3% of those of Fe–Ce–S–H and 37.6% and 31.5% of those of the precursor PBA (Table S1†). This indicated that the PBA-derived catalysts had good stability under the combined action of hydrazine hydrate and S powder. To further verify the superiority of the catalytic performance of Fe–Ce–S–N, the performance of various metal-containing catalysts/H2O2 systems for the removal of sulfonamide pollutants was compared.24–31 The catalytic performance of Fe–Ce–S–N in the presence of moderate H2O2 was favorable.
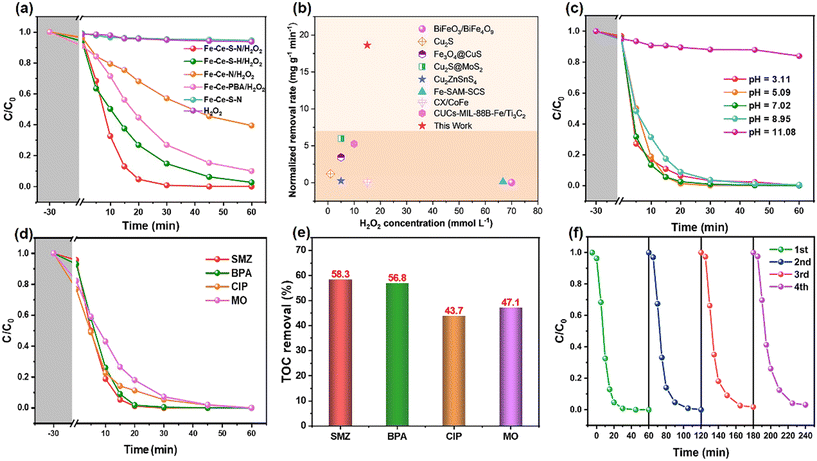 |
| Fig. 3 (a) SMZ degradation curves in various reaction systems; (b) Comparison of the removal rate of sulfonamide pollutants with reported Fenton catalysts;24–31 Fe–Ce–S–N/H2O2 system: (c) removal rate of SMZ under different initial pH, (d) removal rate of different pollutants, (e) TOC removal in 2 h and (f) cycling performances. Reaction conditions: [pollutants] = 20 mg L−1, [catalyst] = 0.2 g L−1, [H2O2] = 15 mM, pH = 5.0, T = 25 °C. | |
As displayed in Fig.
3c, efficient degradation of SMZ (>95% degradation within 30 min) was achieved at initial pH in the range of 3.11–8.95. When the pH was increased to 11.08, the degradation effect of SMZ was significantly inhibited, and the removal rate was only 12.2% in 30 min, which was mainly due to the formation of iron hydroxide precipitate and the ineffective decomposition of H2O2 due to the alkaline condition.32 Besides, the leaching concentration of Fe and Ce in the measured pH conditions was within the EU permitted emission standard (less than 2.0 mg L−1) (Fig. S2b†). Excess H2O2 in Fe–Ce–S–N/H2O2 system would quench the active radicals (eqn (1) and (2)) and competed with SMZ for metal active centers on the surface of Fe–Ce–S–N, and thus hindering the catalytic degradation process (Fig. S3†).
| ·OH + H2O2 → ·OOH + H2O | (1) |
Excess catalysts could also have detrimental effects on the reaction. Therefore, considering the catalytic effect and the cost of chemicals, the optimal experimental conditions were determined to be pH = 5, 0.2 g L
−1 Fe–Ce–S–N and 15 mM H
2O
2.
To verify the applicability of Fe–Ce–S–N/H2O2 system for different organic pollutants, ciprofloxacin (CIP), bisphenol A (BPA) and methyl orange (MO) were selected as the model pollutants to evaluate their degradation performance. Fe–Ce–S–N/H2O2 system could completely remove different types of organic pollutants within 30–45 min (Fig. 3d). Meanwhile, the TOC removal rate of these pollutants ranged from 43.7% to 58.3% after 2 h (Fig. 3e), indicating the superior potential application of Fe–Ce–S–N/H2O2 system.
The reusability and stability of catalysts are the key factors for their practical application. Herein, in order to verify the reusability of Fe–Ce–S–N, the cycling experiment was performed. The degradation efficiency of SMZ could still reach to 97% after four cycles (Fig. 3f), suggesting the excellent reusability and stability of Fe–Ce–S–N. The little loss of catalyst and the adsorption of intermediates on the active site may be the possible reasons for the slight decrease of degradation efficiency. Besides, as shown in Table S2,† after the fourth repetition, the Fe and Ce release was only 0.19 and 0.02 mg L−1. Furthermore, the positions of the characteristic FT-IR peaks of the reacted materials did not change significantly and retained the characteristic functional structure of the sulfide, illustrating the catalytic stability of Fe–Ce–S–N/H2O2 system (Fig. S5†). Moreover, Fe–Ce–S–N can maintain high catalytic activity in complex aqueous environments where lower concentrations of anions and organic matter coexist (Fig. S6†), and has the potential to be applied to the removal of organic pollutants in real water.
3.3. Mechanisms of Fe–Ce–S–N/H2O2 system
To clarify the mechanism of H2O2 activation and SMZ degradation in the Fe–Ce–S–N/H2O2 system, the primary active species were confirmed by quenching experiment and ESR characterization. The degradation efficiency of SMZ decreased by 81.5% in the presence of excess TBA within 30 min, while that decreased by 65.1% in the presence of excess NBT (Fig. 4a). It meant that ·OH and ·O2− were the main active species in Fe–Ce–S–N/H2O2 system, which was consistent with the ESR characterization (Fig. 4c and d).33 The decomposition rate of H2O2 by different catalysts in Fenton-like reaction process was investigated. As shown in Fig. 4b, 60.5% of H2O2 decomposition was achieved after 60 min reaction in the Fe–Ce–S–N/H2O2 system, while only 28.7% of H2O2 was decomposed with the addition of Fe–Ce–N, and the decomposition rate could reach to 49.8% with additive Fe–Ce–S–H. Moreover, FS spectra further verified the changes in ·OH production with time, demonstrating the high efficiency of Fe–Ce–S–N in activating H2O2 to ·OH.
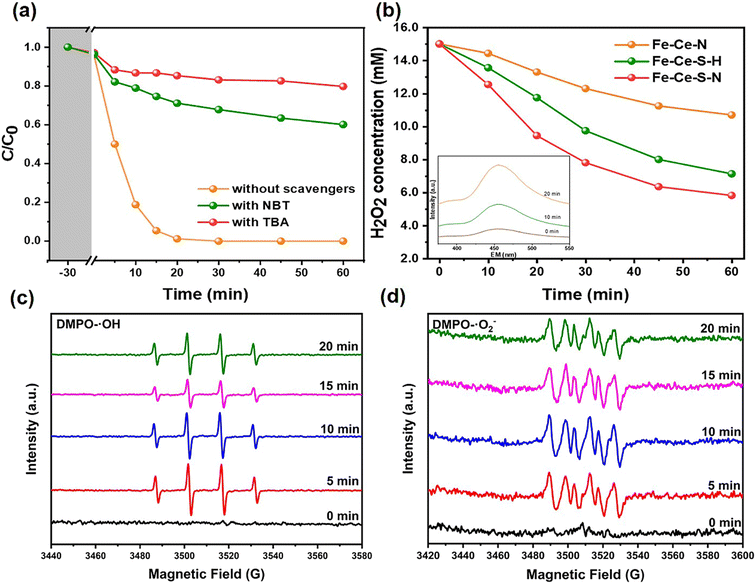 |
| Fig. 4 (a) SMZ degradation in the presence of various active species scavengers; (b) Evolution of H2O2 concentration and ·OH production (inset) in Fe–Ce–S–N, Fe–Ce–S–H and Fe–Ce–N based Fenton-like systems; EPR spectra of (c) DMPO–·OH and (d) DMPO–·O2− in Fe–Ce–S–N/H2O2 systems. | |
The ratio of Fe and Ce in different valence states before and after use was analyzed by XPS and depicted in Fig. S7 and Table S3.† The increase in the proportion of Fe(II) and the decrease in the ratio of Ce(III) after the reaction indicating that Ce(III) acted as electron donor in Fe–Ce–S–N/H2O2 system, and electrons of Ce(III) would migrate to Fe(III) through Fe–S bond. Moreover, due to the participation of S, the conversion of Fe(III) to Fe(II) was further promoted, which accelerated the Fe cycle. The increased cycling efficiency of Fe(III) to Fe(II) favored the degradation process (Fig. 5). The Fe–Ce–S–N/H2O2 system mainly included two mechanisms: (i) Fe–Ce–S–N catalyst had multiple active sites, and Fe(II), Fe(III), Ce(IV) and Ce(III) on the catalyst surface could activate H2O2 to produce active oxygen species;34,35 (ii) The redox potential of Fe(III)/Fe(II) (0.7 V/NHE) is higher than that of S/S2− (−0.48 V/NHE), thus, S2− could act as an electron donor to promote Fe(III)/Fe(II) cycle.36–38 Herein, the effective cycle and regeneration of active sites over the Fe–Ce–S–N resulted in a stable and efficient Fenton-like activities towards SMZ degradation.
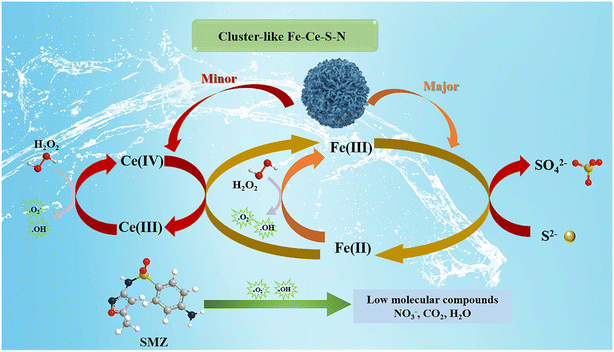 |
| Fig. 5 The possible mechanism in the in Fe–Ce–S–N/H2O2 system. | |
3.4. Degradation products, pathways and toxicity analysis
The possible degradation products and degradation pathways for SMZ were depicted in Fig. 6. The hydroxylation, opening of isoxazole ring and cleavage of SMZ were confirmed as the primary degradation mechanisms. In pathway I, the methyl group in the isoxazole ring of SMZ was replaced by the hydroxyl group and formed the hydroxylated intermediate (P1, m/z = 256) by the attack of ·OH, while the pathway II was due to the dehydrogenation of amino groups in the benzene ring of SMZ (P15, m/z=284). The intermediate (P2, m/z =246) was produced by the opening of isoxazole ring, and its further cleavage could form the intermediates 3 (P3, m/z = 216), 4 (P4, m/z = 198) and 5 (P5, m/z = 158). In pathway IV, the benzene ring of SMZ was attacked by ·OH and produced the hydroxylated intermediate (P6, m/z = 256). The intermediates 7 (P7, m/z = 190) and 8 (P8, m/z = 99) were formed through the rupture of S–N bond in the intermediate 6. Meanwhile, the intermediate 8 could generate the intermediates 9 (P9, m/z = 115) and 10 (P10, m/z = 111) through isoxazole ring hydroxylation and amino group dehydrogenation, respectively. And the intermediate 9 (P9, m/z = 115) could produce the intermediates 11 (P11, m/z = 133), 12 (P12, m/z = 117), 13 (P13, m/z = 118) and 14 (P14, m/z = 102) by a series of reactions including hydroxylation, dehydrogenation, ring opening and bond cleavage.
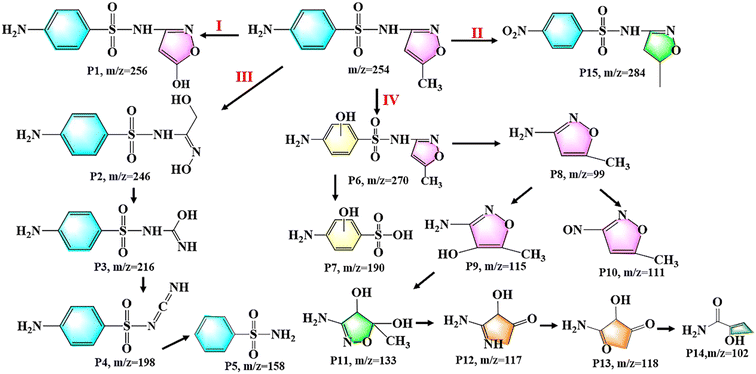 |
| Fig. 6 Possible degradation pathways of SMZ in Fe–Ce–S–N/H2O2 system. | |
Investigation into the toxicity of degradation products was essential for evaluating the environmental risk associated with degradation reactions. Therefore, the biological toxicity of SMZ and its possible intermediate products was estimated by the toxicity assessment software (ECOSAR) based on quantitative structure–activity relationship (QSAR). According to Table S4,† the classification of acute and chronic toxicities can be defined as very toxic, toxic, harmful, and not harmful. The predicted results were presented in Table S5† and Fig. 7. In terms of acute toxicity, most of the intermediate products were referred to as “Not harmful” compounds for the three target species. Meanwhile, only a few intermediate products exhibited obvious higher chronic toxicities than SMZ, such as the predicted ChV to fish of P4, the ChV to Daphnid of P8, and the ChV to Green Alage of P14. Herein, the degradation reaction of this study was of relative low environmental risk.
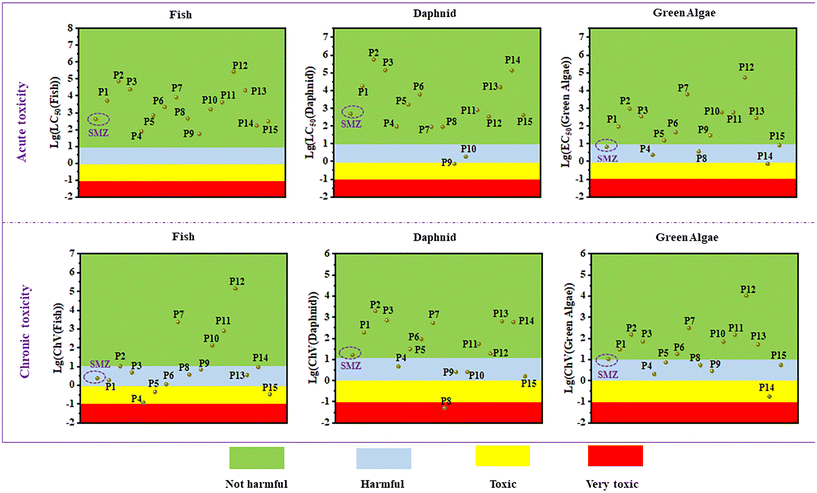 |
| Fig. 7 The predicted toxicity values of SMZ and its products. | |
4. Conclusions
In summary, we successfully synthesized S-modified clustered Fe–Ce–S–N composites by a simple hydrothermal reaction. The obtained Fe–Ce–S–N catalysts, prepared under the optimal conditions, exhibit a unique composition with mixed phases of FeS, CeS and FeCe2O4 that were favorable for the improvement of Fenton-like activities. Benefitting from these merits, the Fe–Ce–S–N materials demonstrate excellent Fenton-like activities with a reaction rate constant as high as 0.1863 min−1, and also exhibit good long-term stability and high efficiency for various organic pollutants, indicating their great potential as promising candidates for practical wastewater treatment. The findings of this study can provide valuable insights into designing highly efficient Fenton-like catalysts and understanding the mechanism of H2O2 activation and contaminant degradation.
Author contributions
Jinli Qiu: conceptualization, investigation, writing–original draft. Huifang Chan: data curation, visualization, writing–review & editing. Wenting Zheng: experiment, methodology. Yao Feng: writing–review & editing. Fuqiang Liu: supervision, conceptualization, validation, writing – review & editing.
Conflicts of interest
There are no conflicts to declare.
Acknowledgements
The authors gratefully acknowledge the support of National Natural Science Foundation of China (No. 51522805), The Natural Science Research Project of Universities in Jiangsu Province of China (No. 22KJB610005), Natural Science Foundation of Nanjing Normal University (No. 184080H202B354) and The National Key Research and Development Program of China (No. 2023YFE0100900). We would like to thank the researchers in the Shiyanjia Lab (https://www.shiyanjia.com/) for their helping with XPS analysis.
References
- Y. Ding, K. Cui, Z. Guo, M. Cui and Y. Chen, Manganese peroxidase mediated oxidation of sulfamethoxazole: Integrating the computational analysis to reveal the reaction kinetics, mechanistic insights, and oxidation pathway, J. Hazard. Mater., 2021, 415, 125719 CrossRef CAS PubMed.
- P. Song, G. Huang, C. An, X. Xin, P. Zhang, X. Chen, S. Ren, Z. Xu and X. Yang, Exploring the decentralized treatment of sulfamethoxazole-contained poultry wastewater through vertical-flow multi-soil-layering systems in rural communities, Water Res., 2021, 188, 116480 CrossRef CAS PubMed.
- Amina, X. Si, K. Wu, Y. Si and B. Yousaf, Mechanistic insights into the reactive radicals-assisted degradation of sulfamethoxazole via calcium peroxide activation by manganese-incorporated iron oxide-graphene nanocomposite: Formation of radicals and degradation pathway, Chem. Eng. J., 2020, 384, 123360 CrossRef CAS.
- X. Wang, J. Jing, M. Zhou and R. Dewil, Recent advances in H2O2-based advance d oxidation processes for removal of antibiotics from wastewater, Chin. Chem. Lett., 2023, 34, 107621 CrossRef CAS.
- C. Dai, X. Tian, Y. Nie, W. Fu and J. Wang, Effect of the interaction mode of H2O2 over CuMnO2 surface on OH generation for efficient degradation of ofloxacin: Activity and mechanism, Chem. Eng. J., 2023, 451, 138749 CrossRef CAS.
- L. Li, C. Lai, F. Huang, M. Cheng, G. Zeng, D. Huang, B. Li, S. Liu, M. Zhang, L. Qin, M. Li, J. He, Y. Zhang and L. Chen, Degradation of naphthalene with magnetic bio-char activate hydrogen peroxide: Synergism of bio-char and Fe-Mn binary oxides, Water Res., 2019, 160, 238–248 CrossRef CAS PubMed.
- Q. Yan, J. Zhang and M. Xing, Cocatalytic Fenton reaction for pollutant control, Cell Rep. Phys. Sci., 2020, 1, 100149 CrossRef CAS.
- D. Meyerstein, Re-examining Fenton and Fenton-like reactions, Nat. Rev. Chem., 2021, 5, 595–597 CrossRef CAS PubMed.
- Z. Yang, C. Shan, B. Pan and J. Pignatello, The Fenton reaction in water assisted by picolinic acid: accelerated iron cycling and Co-generation of a selective Fe-based oxidant, Environ. Sci. Technol., 2021, 55, 8299–8308 CrossRef CAS PubMed.
- A. Mashayekh-Salehi, K. Akbarmojeni, A. Roudbari, J. Hoek, R. Nabizadeh, M. Dehghani and K. Yaghmaeian, Use of mine waste for H2O2-assisted heterogeneous Fenton-like degradation of tetracycline by natural pyrite nanoparticles: Catalyst characterization, degradation mechanism, operational parameters and cytotoxicity assessment, J. Cleaner Prod., 2021, 291, 125235 CrossRef CAS.
- Q. Zhang, M. Zhang, T. Li, R. Du, G. Yu and S. Deng, FeOCl-confined activated carbon for improving intraparticle Fenton-like oxidation regeneration, J. Hazard. Mater., 2023, 442, 130026 CrossRef CAS.
- Y. Hu, Z. Zhong, M. Lu, Y. Muhammad, S. Shah, H. He, W. Gong, Y. Ren, X. Yu and Z. Zhao, Biomimetic O2-carrying and highly in situ H2O2 generation using Ti3C2 MXene/MIL-100(Fe) hybrid via Fe-Protoporphyrin bridging for photo-fenton synergistic degradation of thiacloprid, Chem. Eng. J., 2022, 450, 137964 CrossRef CAS.
- J. Cao, Z. Xiong and B. Lai, Effect of initial pH on the tetracycline (TC) removal by zero-valent iron: Adsorption, oxidation and reduction, Chem. Eng. J., 2018, 343, 492–499 CrossRef CAS.
- K. Hou, Z. Pi, F. Chen, L. He, F. Yao, S. Chen, X. Li, H. Dong and Q. Yang, Trace-dissolved S(-II) triggers the Fe(III)-activated H2O2 process for organic pollutant degradation by promoting the Fe(III)/Fe(II) cycle: Kinetics, toxicity, and mechanisms, ACS ES&T Engg, 2022, 2174–2186 Search PubMed.
- Y. Cai, J. Fan and Z. Liu, Enhanced degradation of tetracycline over FeS-based Fenton-like process: Autocatalytic decomposition of H2O2 and reduction of Fe(III), J. Hazard. Mater., 2022, 436, 129092 CrossRef CAS.
- Y. Yang, X. Li, B. Jie, Z. Zheng, J. Li, C. Zhu, S. Wang, J. Xu and X. Zhang, Electron structure modulation and bicarbonate surrounding enhance Fenton-like reactions performance of Co-Co PBA, J. Hazard. Mater., 2022, 437, 129372 CrossRef CAS.
- W. Xie, Z. Huang, F. Zhou, Y. Li, X. Bi, Q. Bian and S. Sun, Heterogeneous fenton-like degradation of amoxicillin using MOF-derived Fe-o embedded in mesoporous carbon as an effective catalyst, J. Cleaner Prod., 2021, 313, 127754 CrossRef CAS.
- X. Jin, Y. Huang, S. He, G. Chen, X. Liu, C. He, C. Du and Q. Chen, Preparation of Co-Fe based Prussian blue analogs loaded nickel foams for Fenton-like degradation of tetracycline, Appl. Catal., A, 2023, 650, 118985 CrossRef CAS.
- W. Zheng, H. Guo, C. Zhu, C. Yue, W. Zhu, F. Liu and Z. Chen, Ultralow-Energy-Barrier H2O2 Dissociation on Coordinatively Unsaturated Metal Centers in Binary Ce-Fe Prussian Blue Analogue for Efficient and Stable Photo-Fenton Catalysis, Energy Environ. Mater., 2023, e12476 CrossRef.
- C. Xuan, W. Lei, J. Wang, T. Zhao, C. Lai, Y. Zhu, Y. Sun and D. Wang, Sea urchin-like Ni-Fe sulfide architectures as efficient electrocatalysts for the oxygen evolution reaction, J. Mater. Chem. A, 2019, 7, 12350–12357 RSC.
- Y. Hou, Z. Wen, S. Cui, S. Ci, S. Mao and J. Chen, An advanced nitrogen-doped graphene/cobalt-embedded porous carbon polyhedron hybrid for efficient catalysis of oxygen reduction and water splitting, Adv. Funct. Mater., 2015, 25, 872–882 CrossRef CAS.
- L. Li, P. Dai, X. Gu, Y. Wang, L. Yan and X. Zhao, High oxygen reduction activity on a metal–organic framework derived carbon combined with high degree of graphitization and pyridinic-N dopants, J. Mater. Chem. A, 2017, 5, 789–795 RSC.
- Y. Hu, H. Kazemian, S. Rohani, Y. Huang and Y. Song,
In situ high pressure study of ZIF-8 by FTIR spectroscopy, Chem. Commun., 2011, 47, 12694–12696 RSC.
- H. Zhang, C. Zhou, H. Zeng, L. Deng and Z. Shi, Can Cu2ZnSnS4 nanoparticles be used as heterogeneous catalysts for sulfadiazine degradation?, J. Hazard. Mater., 2020, 395, 122613 CrossRef CAS PubMed.
- H. He, M. Sun, D. Wu, G. Di and X. Fei, Cu(III) generation and air sparging extend catalytic effectiveness of Cu2S/H2O2 from neutral to acidic condition: performance and mechanism in comparison with CuS/H2O2, J. Cleaner Prod., 2021, 278, 123572 CrossRef CAS.
- M. Cheng, G. Zeng, D. Huang, C. Lai, Y. Liu, C. Zhang, J. Wan, L. Hu, C. Zhou and W. Xiong, Efficient degradation of sulfamethazine in simulated and real wastewater at slightly basic pH values using Co-SAM-SCS/H2O2 Fenton-like system, Water Res., 2018, 138, 7–18 CrossRef CAS PubMed.
- H. Zhang, C. Zhou, H. Zeng, H. Wu, L. Yang, L. Deng and Z. Shi, ZIF-8 assisted synthesis of magnetic core–shell Fe3O4@CuS nanoparticles for efficient sulfadiazine degradation via H2O2 activation: Performance and mechanism, J. Colloid Interface Sci., 2021, 594, 502–512 CrossRef CAS PubMed.
- H. Zhang, L. Deng, J. Chen, Y. Zhang, M. Liu, Y. Han, Y. Chen, H. Zeng and Z. Shi, How MoS2 assisted sulfur vacancies featured Cu2S in hollow Cu2S @ MoS2 nanoboxes to activate H2O2 for efficient sulfadiazine degradation?, Chem. Eng. J., 2022, 446, 137364 CrossRef CAS.
- M. Ahmad, X. Quan, S. Chen and H. Yu, Tuning Lewis acidity of MIL-88B-Fe with mix-valence coordinatively unsaturated iron centers on ultrathin Ti3C2 nanosheets for efficient photo-Fenton reaction, Appl. Catal., B, 2020, 264, 118534 CrossRef.
- R. Ribeiro, Z. Frontistis, D. Mantzavinos, D. Venieri, M. Antonopoulou, I. Konstantinou, A. Silva, J. Faria and H. Gomes, Magnetic carbon xerogels for the catalytic wet peroxide oxidation of sulfamethoxazole in environmentally relevant water matrices, Appl. Catal., B, 2016, 199, 170–186 CrossRef CAS.
- Z. Hu, J. Liu, J. Zhao, Y. Ding, Z. Jin, J. Chen, Q. Dai, B. Pan, Z. Chen and J. Chen, Enhanced BiFeO3/Bi2Fe4O9/H2O2 heterogeneous system for sulfamethoxazole decontamination: System optimization and degradation pathways, J. Colloid Interface Sci., 2020, 577, 54–65 CrossRef CAS PubMed.
- Q. Yi, J. Ji, B. Shen, C. Dong, J. Liu, J. Zhang and M. Xing, Singlet oxygen triggered by superoxide radicals in a molybdenum cocatalytic Fenton reaction with enhanced REDOX activity in the environment, Environ. Sci. Technol., 2019, 53, 9725–9733 CrossRef CAS.
- Y. Jiang, P. Wang, T. Chen, K. Gao, Y. Xiong, Y. Lu, D. Dionysiou and D. Wang, Efficient 1O2 production from H2O2 over lattice distortion controlled spinel ferrites, Appl. Catal., B, 2024, 343, 123468 CrossRef CAS.
- L. Guo, F. Chen, X. Fan, W. Cai and J. Zhang, S-doped alpha-Fe2O3 as a highly active heterogeneous Fenton-like catalyst towards the degradation of acid orange 7 and phenol, Appl. Catal., B, 2010, 96, 162–168 CrossRef CAS.
- D. Wang, A. Junker, M. Sillanpää, Y. Jiang and Z. Wei, Photo-based advanced oxidation processes for zero pollution: Where are we now?, Engineering, 2023, 23, 19–23 CrossRef.
- Z. Zhong, M. Li, J. Fu, Y. Wang, Y. Muhammad, S. Li, J. Wang and Z. Zhao, Construction of Cu-bridged Cu2O/MIL(Fe/Cu) catalyst with enhanced interfacial contact for the synergistic photo-Fenton degradation of thiacloprid, Chem. Eng. J., 2020, 395, 210067 Search PubMed.
- S. Hu, Y. Li, C. Zang, X. Ma, B. Zhao and F. Chen, Piezoelectric field-promoted heterogeneous sono-Fenton performance of MoS2/alpha-Fe2O3 heterojunction structure, Appl. Surf. Sci., 2020, 534, 250312 CrossRef.
- L. Zhao, Y. Chen, Y. Liu, C. Luo and D. Wu, Enhanced degradation of chloramphenicol at alkaline conditions by S(-II) assisted heterogeneous Fenton-like reactions using pyrite, Chemosphere, 2017, 188, 557–566 CrossRef CAS PubMed.
|
This journal is © The Royal Society of Chemistry 2024 |
Click here to see how this site uses Cookies. View our privacy policy here.