Behaviour of advanced materials in environmental aquatic media – dissolution kinetics and dispersion stability of perovskite automotive catalysts†
Received
26th September 2023
, Accepted 2nd December 2023
First published on 15th December 2023
Abstract
Tighter regulations on exhaust emission limits require more effective catalysts and here advanced materials (AdMa) play an increasingly important role. Perovskite-based catalysts are among the promising candidates. However, like other automotive catalysts, they contain metal elements of potential concern like nickel, cobalt and noble metals; hence, their likelihood to be released and their fate under environmentally relevant conditions must be assessed at the early stages of material development, so as to align with the goals of the EU Chemical Strategy for Sustainability. The aim of this study is to provide insights into the dissolution and agglomeration behaviour of perovskites in aqueous media with different ionic strengths and salt contents, as well as the influence of the presence of natural organic matter (NOM). The current OECD guidance document and testing guidelines (GD 318 and TG 318, respectively) for nanomaterial testing were applied to three different perovskite AdMa with a lanthanum–cobalt–nickel (LaCoNi) structure with and without doping with palladium or platinum. These tests resulted in a range of practical insights into the feasibility of this methodological cross-over as well as evidence on transferability and applicability to other case studies. Our findings rank the dissolution kinetics of these perovskites to lie between the two reference nanomaterials ZnO and BaSO4. Dissolution rates were found to be, respectively, for ZnO NM110, LaCoNi, and BaSO4 NM220: 0.13, 0.041, and 0.013 μg m−2 s. The ionic strength of the media used in this study did not seem to impact the overall leachable amount of metals (% w/w); however, we found that metal release was mostly incongruent and metal specific i.e., a lower lanthanum ratio with respect to either cobalt or nickel. The presence of NOM increased the dissolution of both benchmark materials; however, no strong influence on dissolution was observed for the perovskite materials. The dispersion stability of perovskites in solution was substantially increased by the presence of NOM and decreased by increased hardness in the test media. Finally, this study provides methodological insights and practical recommendations for testing the dissolution and dispersion stability in different media relevant for ecotoxicological testing and environmental risk assessment.
Environmental significance
To ensure product safety, reliable and regulatory relevant and compliant data are needed to support companies during the innovation phases. We propose and test a combination of a continuous flow system for dissolution kinetics with methods for dispersion stability for early screening of advanced materials to provide regulatory adequate data of importance for their environmental behaviour and effects. Specifically, given the presence of nickel in the perovskites in analysis, the aqueous complexes formed by nickel can be of concern with regard to bioaccumulation and toxicity in the aquatic compartment. Our work aligns with the Safe and Sustainable by Design (SSbD) concept for chemicals and materials, where it is recommended to start the screening of environmental impacts at the design phase, assessing the material's fate in the environment.
|
Introduction
While industry develops increasingly complex and advanced materials (AdMa) and applies the Safe and Sustainable by Design (SSbD) concept into R&D goals, considerations of environmental fate and behaviour of advanced materials (AdMa) become increasingly important. Perovskite-based three-way catalytic converters are examples of AdMa that may potentially outperform and replace some of the currently used materials in the field of automotive catalysis. Since the introduction of catalyst equipped cars in Europe in 1985,1 emission limits have gradually been lowered2 and in 2022, the EU proposed the new Euro 7 emission standards in alignment with the European Green Deal's zero-pollution ambition.3 Globally around 140 million automotive catalytic converter units were sold in 2019 and increased sales are expected.4 This high and widespread use of catalytic converters will consequently increase the release of converter materials during use as well as at their end-of-life.5
Perovskite-based catalysts are multicomponent materials, complying with current AdMa definitions6 since they are made by rational design, using precise control of their composition and internal structure, and have specific functionalities that differentiate them from conventional materials due to e.g., oxygen storage capacity and enhanced turnover activity. It should be noted here that even though perovskite catalysts include nano-structured materials, they are not considered as nanomaterials themselves in accordance with the REACH definition, as primary particle sizes exceed 100 nm.7 To function properly, all automotive catalytic converters contain noble and/or heavy metals. The metals of potential concern in the perovskite materials of this study are cobalt (Co) and nickel (Ni) which, along with lanthanum (La), form the catalyst structure that additionally can be doped with platinum (Pt) or palladium (Pd) reaction centers.
If fractions of the catalyst are released during the decade-long use time, road runoff to surface water may result in environmental impacts from released metals. In this scenario as well as for hazard identification purposes, quantification of the leachable metal fraction from a given material can be crucial to document the fate and behaviour of AdMa in the aquatic phase (ions vs. particles). However, this is not meant to replace other descriptors that use stoichiometry to assess particle persistence. In the case of metal-containing nanomaterials, the processes of dissolution and agglomeration are of particular importance in this respect.8 While specific testing procedures for AdMa are currently lacking, methodological crossover from existing guidance for nanomaterials may be possible. For nanomaterials, the OECD Guidance Document 318 (GD318)9 describes in detail suitable experimental parameters for simulating dissolution in the lungs (e.g., amount of material, flow rate, buffer composition, time frame of the experiment) but provides much less specific guidance for the environmental simulation. In fact, it is only stated that: ‘considerably different concentrations (e.g. media composition, temperature) and flow rates (μg and ml min−1) should be used compared to those used for the dissolution testing in biological media (mg and ml h−1), thus saturation effects are unlikely to occur’.
In the OECD Technical Guideline 318 (TG318),10 procedures for dispersion, aggregation, and dissolution determination of nanomaterials are given. However, current dynamic release studies assessing the agglomeration/sedimentation are limited to few individual benchmark nanomaterials tested in simple electrolyte solutions.11 Complex AdMa, like perovskites, have not yet been tested and hence the role of environmentally relevant parameters such as ionic strength and natural organic matter (NOM) also remains to be studied.12,13
The aim of the current study is therefore two-fold: to study the dissolution and agglomeration behaviour of perovskite-based AdMa in aqueous media and to provide methodological insights in the testing of the environmental behaviour of complex advanced materials. Specifically, the dispersion stability, dissolution and agglomeration dynamics of three perovskites and two reference nanomaterials (ZnO NM110 and BaSO4 NM220) will be studied using three detection methods, in three standardised testing media with different ionic strengths, water hardness, and NOM addition. Finally, the experiences and results obtained will be used to reflect on the applicability and transferability of OECD methods developed for nanomaterials to multicomponent and “not-easy-to-disperse” AdMa. The present work contributes to the current development of an OECD TG for aquatic dissolution of nanomaterials and provides practical methodologies with potential to assist in safe and sustainable material design.
Materials and methods
Materials & benchmarks
Multicomponent perovskites with the general chemical formula AB0.8O3−δ (with and without noble metal doping) were provided by BASF R&D (Ludwigshafen, Germany) in three different versions of LaCo0.5Ni0.3O2.9: undoped, doped with palladium and doped with platinum, and for simplicity in the following text named, respectively: LaCoNi, LaCoNi_Pd and LaCoNi_Pt. Lanthanum is located in the A position whereas Co and Ni occupy the B position. Physicochemical characterization for the three perovskites, including BET, XRD, UV-vis, DLS, SEM, ICPMS and XPS, is reported in the ESI† (Fig. S1 and Table S1). Given the novelty and complexity of these AdMa, two nanomaterial reference materials, ZnO NM110 and BaSO4 NM220 (supplied by the European Commission's Joint Research Centre, Ispra, Italy), were included as benchmarks for methodological calibration and material comparison. Stock dispersions for each material were prepared following the SOP developed for the preparation of general batch dispersions for ecotoxicity testing in the NANoREG project.14 A 2.56 mg mL−1 stock solution was prepared by dispersing the test material in MilliQ water using probe sonication (Branson, Model SFX550, microtip 3 mm diameter). The stock was then further diluted to reach the desired concentration for each experiment. All samples weighted as powder in the dry loading mode were prewetted with 2/3 drops of ethanol.
Continuous flow system
The aquatic dissolution setup was originally developed for engineered nanomaterials (ENMs) as described in OECD GD318 (ref. 9) and selected as the method of choice by Stetten et al.15 As shown in Fig. 1B, it consists of a reservoir containing the aquatic media to test and two HPLC pumps (FLUSYS GmbH, PLM 707-1, Offenbach am Main, Germany) connected with a 12-port valve injector (manual, VICI model, DC12CW, VICI-Valco Instruments, Houston, TX, USA). The material dispersion was injected into a metal-free flow-through cell and deposited onto a cellulose triacetate membrane with a 5 kDa pore size (47 mm diameter, Sartorius Stedim Bio-tech GmbH, Goettingen, Germany). In addition, a variant of the system where the material is loaded as dry powder was developed and tested (Fig. 1A). More details are given in section 3.1. The flow rate was kept constant during all experiments. Different flow rates, in the range from 0.1 to 1 mL min−1, were screened, as well as the amount of nanomaterial injected (from 1 μg to 100 μg) to allow for dissolution kinetic considerations. Water samples were collected using an autosampler at 13 sampling time points during a 48-hour experiment. The element concentration was determined by inductively coupled plasma mass spectrometry (ICP-MS, PerkinElmer NexION 2000). All samples were acidified by diluting 1
:
10 with 2% (v/v) HNO3 prior to analysis. This ensured low salt content from the buffers. All experiments were performed in duplicate. The flow cells used for the experiments were modified in house at BASF so as to enhance the temporal resolution of quickly dissolving materials. Our modified flow cells have a reduced dead volume of 2.3 mL (against 9.5 of the original ones that can be bought i.e., BOLA cells). It is to be noted that for low flow rates (i.e., lung simulant conditions, order of 2 mL h−1), dead volumes are particularly relevant as they constitute the first hour of sampling. In the present paper, as flow rates are in the order of mL min−1, 2.3 mL of dead volume accounts for the first minutes of the experiment, therefore it is foreseen to not impact consistently the final outcome.
 |
| Fig. 1 (A) Dry powder loading set up consisting of a reservoir, HPLC pumps, filter holders where the dry sample is loaded and prewetted, and the autosampler. (B) Injection setup consisting of a reservoir, HPLC pumps, a 12-port injection valve equipped with 100 μL sample loops, filter holders and the autosampler. | |
Test media
Three different standard media for aquatic testing were selected: EPA soft, M7 media and L-15/ex. Table 1 shows the ionic strength (IS), hardness and Ca concentration for each medium. The pH was kept at 8.0 ± 0.2 for the duration of all experiments. The full media composition can be found in Weber et al., OECD guideline 211 and Yue et al., respectively for EPA soft, M7 and L-15/ex.16–18
Table 1 Ionic strength (IS), water hardness, and calcium concentration for the three testing media. More details on the media description and characteristics can be found in Weber et al., OECD guideline 211 and Yue et al., respectively for EPA soft, M7 and L-15/ex16,17,19
|
EPA soft (low IS) |
M7 (mid IS) |
L-15/ex (high IS) |
Ionic strength (mM) |
2.28 |
9.06 |
173.0 |
Water hardness (mM) |
0.42 |
2.50 |
2.08 |
Calcium concentration (mM) |
0.17 |
2.00 |
1.26 |
Moreover, the influence of 10 mg L−1 NOM addition (Suwannee river, purchased from IHSS https://www.humic-substances.org/) to the selected media was tested in accordance with TG318.10
Data treatment
Continuous flow systems for nanomaterial dissolution are already well-established to simulate lung conditions. Hence extensive literature is available on how to account for the kinetic of nanomaterial dissolution in such systems. Briefly, for each experiment, instantaneous dissolution rates were calculated by eqn (1), in accordance with Keller et al.20 | 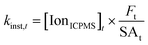 | (1) |
where kinst,t is the instantaneous dissolution rate at a specific time interval, t, expressed in μg s−1 m−2, [IonICPMS]t is the ion concentration measured by ICPMS in μg mL−1, Ft is the flow rate of the eluent in mL s−1, and SAt is the surface area in m2 of the total amount of material loaded. SAt depends on the undissolved concentration of the material and on the specific surface area obtained by BET measurements. For all materials tested, it was assumed a shrinking sphere geometry.21 All dissolution data will be uploaded on eNanomapper, enabling data re-use.
For the perovskite materials, an average cumulative dissolution rate at each time point was calculated to account for the multiple ions contained in their structure. A new descriptor called ‘leachable mass %’ was introduced as a mass-based accounting of the total mass of metal ions released from the sample as either leached or dissolved ions relative to the initial mass of the sample; this means that if 1 μg of material is loaded and a total of 0.25 μg of metal ions is recovered in the water samples, the leachable mass is 25%. In cases of congruent dissolution, this descriptor can be re-expressed as the amount dissolved by adjusting the sample's metal ion content, which is 80% for ZnO and 59% for BaSO4. A 25% leachable mass for ZnO would be 31.3% expressed as the dissolved mass and 42.4% for BaSO4.
Dispersion stability
The OECD TG318 prescribes testing under different hydro-chemical conditions representing the stability relevant composition of many fresh waters with varying parameters such as pH, ionic strength, and the presence of natural organic matter (NOM).10 We selected two out of the three pH proposed by the guideline (7.0 and 9.0) and two calcium concentrations (1 mM and 10 mM). The NOM influence at 10 mg L−1 was additionally considered. All experiments were performed on LaCoNi, given that previous studies already addressed the stability in dispersion of the reference materials selected.22 Based on the dissolution studies, the doping of Pd or Pt was not relevant enough to account for differences in the perovskites' behaviour in dispersion. The concentration of LaCoNi stock suspension was in the range recommended by the OECD guideline to achieve comparable results among materials with different sizes, i.e., 0.5 × 1012 to 5 × 1012 particles L−1. A pre-screening by dynamic light scattering and the UV-vis absorption wavelength of LaCoNi are reported in the ESI† (Fig. S1).
Three different detection methods were compared to allow for inter validation of the method and comparison. Two of the methods are suggested in TG318: UV-vis (Perkin Elmer, Lambda 35) and ICPMS detection. Prior to ICPMS analysis, the acidified samples were digested for 20 min at 120 °C in an autoclave (Tuttnauer, EL-D Line model). In addition, analytical ultracentrifugation (AUC) was used as an alternative method to assess LaCoNi stability in dispersion (Beckman Ultracentrifuge XLI™ with integrated interference optics, Beckman Coulter, USA). Sedimentation data were evaluated with Sedfit v15.0 software. By fitting the ultracentrifuge with an optical detection system, the sedimentation process of dispersed particles could be observed in real time, providing a distribution of sedimentation velocities,23 which can be integrated to “% sedimented” for a known settling depth and time, to compare to the TG318 approach by ICPMS and UV-vis.
Results and discussion
Comparison of loading conditions in continuous flow system setups
The use of continuous flow systems (CFSs) is recommend by ISO TR 19057 as a suitable method for measuring nanomaterial durability, in contrast to static experiments, where problems of supersaturation can arise.24 The CFS is now an established and standardised method for the screening of the dissolution kinetics of nanomaterials under lung simulant conditions.20,25,26 However, for environmentally relevant media and conditions, standardisation is still lacking. When developing the continuous flow dissolution setup used and evaluated in this study, two options for loading of the test materials were compared: 1. dry powder loading (Fig. 1A); 2. dispersion loading (Fig. 1B). The first option is intended to be more suitable for materials which cannot be homogeneously dispersed (e.g., hydrophobic pigments, silica aerogels). The two CFS versions were tested with two aqueous media, characterized by different hardness and ionic strength (see Table 1), using the two nanomaterials ZnO and BaSO4 and the AdMa LaCoNi. The results of these experiments are shown in Fig. 2 and summarized, in terms of steady-state dissolution rates, in Table 2 where the dissolution rate for LaCoNi is the sum of the individual elemental metal ion concentrations. In addition, for the EPAs medium, we report in Fig. S2† the % undissolved metal during the time of the experiment at each sampling point, so as to have a visual impression of mass loss over time.
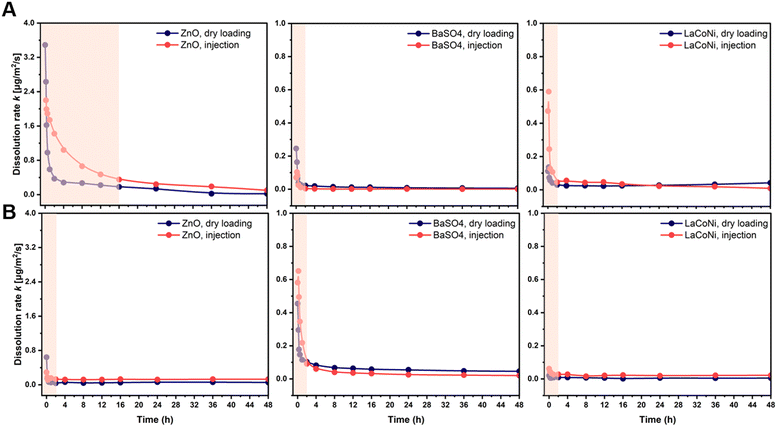 |
| Fig. 2 Instantaneous dissolution rates (kinst) as a function of time for experiments in (A) EPA soft media and (B) M7 media for the materials. (1) ZnO NM110, (2) BaSO4 NM220, and (3) LaCoNi perovskite. A comparison of the dry loading (blue) and dispersion loading by injection (red) is shown for each material. Shaded areas indicate the initial phase before the system equilibrates into a steady state. This area was not considered when calculating the average flow rate for each material. Note the different scaling of the secondary axes for ZnO experiments compared to the secondary axes in BaSO4 and LaCoNi experiments. | |
Table 2 Steady-state dissolution rates for the nanomaterials ZnO and BaSO4 and the perovskite LaCoNi obtained with two different media using dry powder loading and dispersion loading in continuous flow systems. Values are averages [μg m−2 s] with the respective standard deviations. Material loading: 100 μg L−1, flow rate: 1 mL min−1
Medium |
Material |
Dissolution rate ka [μg m−2 s] |
Dry powder loading |
Dispersion loading |
Dissolution rates were calculated in the steady state.
The dissolution rate for LaCoNi is a cumulative rate which includes all main metal components.
|
EPA soft |
ZnO |
0.045 ± 0.02 |
0.13 ± 0.10 |
BaSO4 |
0.013 ± 0.008 |
0.013 ± 0.007 |
LaCoNib |
0.025 ± 0.008 |
0.041 ± 0.007 |
M7 |
ZnO |
0.06 ± 0.02 |
0.13 ± 0.08 |
BaSO4 |
0.05 ± 0.02 |
0.05 ± 0.01 |
LaCoNib |
0.012 ± 0.006 |
0.026 ± 0.005 |
A different behaviour between dry loading and injection was observed only in the first 20–30 minutes of the experiment. For both methods, the material was in contact with the test media (dry loading) or MilliQ water (injection loading) before the beginning of the experiment. Hence the dissolution process had in both cases started before the first sampling point in the experiment. This initial dissolution behaviour was material dependent, as could be expected. However, as also described by Stetten et al. (2022), the results obtained in the first 20–30 min of the experiments reflect a stabilization phase for the system to reach a steady state. Dissolution rates calculated in the first phases of the experiments do not reflect the actual dissolution kinetics for longer term contact between the perovskites and the media. Independent of the loading method, stable instantaneous dissolution rates were obtained after 2 hours for BaSO4 and LaCoNi in both media (Fig. 2). This was also the case for ZnO in M7 medium, whereas in EPA soft water, steady state was reached after 16 h, following a mono-exponential decay in the initial hours of the experiment. This type of kinetics for ZnO nanoparticles was previously observed in different test media.26 The data shown in Table 2 (i.e., the average instantaneous dissolution rates) document a general good coherence and overlap in steady-state dissolution rates for both media using the two loading methods. However, the precision was better for the BaSO4 for which dissolution rates were also independent of media and the loading method. For LaCoNi, the average steady-state dissolution rates in EPAs were observed in the range from 0.025–0.041 μg m−2 s, whereas for M7, the dissolution rates ranged from 0.012–0.026 μg m−2 s. For ZnO, higher dissolution rates were observed when using dispersion loading which, together with higher standard deviation between replicates, suggests that the initial material loading mode plays a role in the determination of the dissolution kinetics. Elevated polydispersity of the size distribution of dissolving particles can add to the trend of slowing of dissolution,27 but not to the extent observed here. The relatively low polydispersity of the perovskites of σg = 1.05 is still below the applicability limit of polydispersity-based models of dissolution.27 SEM images are reported in Fig. S1.†
For all the materials studied here, sufficiently homogeneous dispersions were prepared, allowing for reproducible sampling and analysis (see Fig. S1†). This was done by following the Nanogenotox protocol as described in the Materials and Methods section. For our materials, and probably also for other dispersible materials, the dispersion method therefore allows for higher accuracy and precision in the amount of material loaded in the system. From a practical point of view, the injection method using dispersion was therefore preferable in our experiments. Indeed, within the ranges of concentrations suggested by GD318 (μg and ml min−1), a higher uncertainty in the exact amount of dry material weighted on a scale is inevitable. This may have contributed to the variation in results obtained for LaCoNi and ZnO in using the two different loading methods. However, it must be noted that if higher loading (i.e., in the order of mg) is allowed or considered relevant for the materials and the conditions investigated, the dry loading method is preferable due to a lower tendency of the material to agglomerate in the time between dispersion and injection. Indeed, particle agglomeration would lead to filter saturation, breaking off the monolayer conditions and therefore limiting material's dissolution.
Influence of the flow rate and material loading on dissolution rates
In Fig. 3, instantaneous dissolution rates for ZnO, BaSO4 and LaCoNi obtained at different flow rates (0.1 mL min−1, 0.5 mL min−1, 1 mL min−1) and at different loaded masses (1 μg, 10 μg, 100 μg) are plotted against the surface area per volume flow ratio. Individual instantaneous dissolution rates for each material and testing condition are listed in Table S2.† It was previously shown by Keller et al. that variation of the initial mass loaded on the CFS or of the flow rate leads to an inverse-linear relationship between the dissolution rate and the surface area to volume flow ratio (SA/
).20 They also demonstrated that this is a material specific phenomenon. These observations were made under considerably different experimental conditions than in the current study (i.e., flow rates in mL h−1, mg of material loaded, lung simulant conditions and acidic pH). Here, we test these findings under conditions relevant for ecotoxicological testing, using mL min−1 flow, initial mass in μg and EPA soft as a buffer simulant at pH 8. The results confirm a correlation between the surface area per volume (SA/
) and dissolution rate k for all three materials. For BaSO4, dissolution rates had a steeper dependence on the surface area per volume flow compared to ZnO. Indeed, for each material, data from several measurement series with different mass and flow conditions all collapse into one log–log linear curve that is characteristic of the material (more details in Fig. S3†).
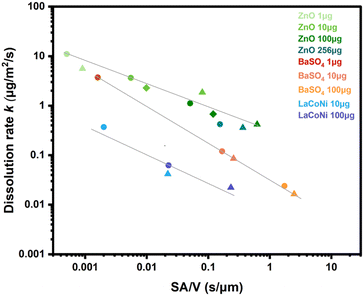 |
| Fig. 3 Dissolution rate (k) shown as a function of the surface area to volume flow ratio (SA/ ) for different materials (ZnO, BaSO4, LaCoNi), flow rates and loaded amounts. Different symbols show the different flow rates investigated, i.e., dot for 1 mL min−1; triangle for 0.1 mL min−1; diamond for 0.5 mL min−1. Different colors refer to different amounts of material loaded. The log–log linear relationship is reported for all three materials and further described in Fig. S3.† | |
By comparing the slope of the three curves, LaCoNi rates have an intermediate dependence on SA/
, in between those of the two benchmark nanomaterials. By extrapolation, we can predict that at high flow rates, saturation conditions for the perovskite material will be reached, whereas at low flow rates, the dissolution kinetics will be more dependent on the SA/
ratio. Keeping in mind these considerations, we selected 100 μg of material loading and 1 mL min−1 flow rate for further investigation of the dissolution behaviour of the two benchmark nanomaterials (ZnO and BaSO4) and the three selected perovskites. These conditions are in line with what is recommended by GD318 to ensure high reproducibility and are meaningful for ecotoxicological testing.
Influence of the ionic strength on metal leaching
Fig. 4A shows the leachable mass % as a function of time for the two benchmark materials and the three perovskites in the three different media. As described above, a flow rate of 1 mL min−1 and an initial dispersion loaded mass of 100 μg were chosen as the most suitable test conditions. ZnO dissolved up to 70% in EPA soft media (low IS), whereas in the M7 medium which has a higher ionic strength, lower dissolution was observed. In L-15/ex, a leachability up to 50% of the total particle mass was observed. This corresponds to almost 70% of the total Zn content. On the one hand, higher dissolution rates can be expected with increasing ionic strength due to surface protonation displacing ions from surface sites. This is visible in the first eight hours of the experiment, where a faster dissolution is observed in L-15/ex before reaching saturation, compared to the lower kinetics in the low IS media EPA soft. On the other hand, by increasing the ionic strength, the electrical double layers surrounding the particle can break, decreasing its surface charge and leading to increased agglomeration.19 In other words, the electrostatic repulsion between particles is weakened, losing its primary role of preventing particle aggregation. This might explain what is observed for ZnO in the M7 media, where the ionic strength is not high enough to displace ions from surface sites whereas the higher salt concentrations weaken the repulsion between particles causing agglomeration. For BaSO4, in agreement with previous studies, our results show coherent leaching of barium ions, with increasing ionic strength: from 7% in EPA soft to 20% in M7, reaching almost 30% in L-15/ex. In terms of total mass of barium, his corresponds to 12%, 34% and 51%, respectively. For the perovskite series, a higher leachability was observed for the Pt doped material. Though the differences between the perovskite materials were small, they rank as LaCoNi_Pt > LaCoNi_Pd > LaCoNi. In general, leaching of ions from the perovskites was not dependent on the ionic strength in the media. Previous studies have shown that for perovskites with the ABO3 crystal structure, one of the main drivers for dissolution is the acidic pH.28 Indeed, there was no significant dissolution for any of the media investigated at the pH used (pH 8). However, as can be seen in Fig. 4B for LaCoNi, incongruent leaching was observed but Ni was the element showing the highest release compared to La and Co. This can be explained by the nickel surface enrichment found by XPS analysis (Fig. S1†). Given the initial 100 μg of material loaded in the CFS, approximately 2% of the total mass of the perovskite (corresponding to 16% of the total mass of nickel in the material) has leached from the material after 24 h, corresponding to a total nickel concentration of 1.4 ng L−1. It is worthwhile highlighting that our discussion is based on the fact that the dissolution kinetics depends on the balance between the energy needed to break the solvent structure and the energy gained on hydrating the ions potentially released from the material, which are specific for different electrolyte solutions (i.e., different test media). Dissolution is assisted by increased affinity of the buffer with the material crystal structure and by decreased buffer affinity to the other solvent molecules in the respective test media.29 The three test media here investigated differ in IS and in electrolyte composition, making predictions of ion leaching in solution more challenging.
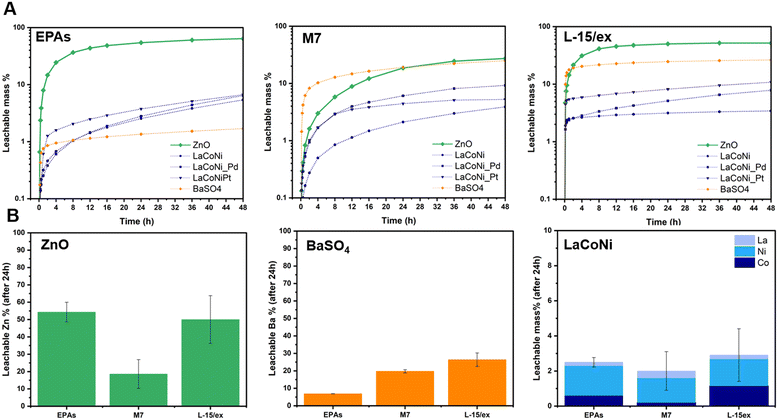 |
| Fig. 4 (A) The cumulative leachable mass (in % of total mass) of ZnO and BaSO4 and the three perovskites (LaCoNi, LaCoNi_Pd and LaCoNi_Pt) monitored over a 48 h period in three different test media: EPA soft, M7, L-15/ex. (B) Comparison of the total mass leached 24 hours after the steady state was reached in different test media for ZnO, BaSO4 and LaCoNi. The standard deviation between replicates is included. For LaCoNi, the single elements contributing to the total leachable mass are highlighted. All results were obtained in the continuous flow system in dispersion injection mode with 100 μg material and a flow rate of 1 mL min−1. | |
Influence of NOM on metal leaching
Fig. 5 describes the influence of NOM on the dissolution behaviour of ZnO, BaSO4 and LaCoNi in two aquatic media. We know that the interaction between organic matter and colloidal suspensions is governed by adsorption which in turn is influenced by sorbent (AdMa), sorbate (NOM), and medium characteristics. Solution properties such as pH, ionic strength, and valence of ions influence both sorbent and sorbate characteristics.30 In our specific case, the sorbate consists of 15% fulvic acid and 85% humic acid added in a concentration of 10 mg L−1. For ZnO, the point of zero charge is reported to be at pH 9.31 Its surface is therefore positively charged and saturated with zinc ions under the conditions investigated. Humic substances can therefore readily adsorb onto ZnO particles, preventing them from agglomeration and acting as a stabilizing agent in ZnO dispersions. The decrease of particle aggregates, caused by NOM addition, increases the total surface of the particles exposed to the aqueous media, making the process of dissolution faster. However, these phenomena can be observed under conditions where aggregation is present, leading us to the hypothesis that ZnO in EPA soft media is stable in dispersion (see Fig. 5A). This theory is confirmed by the experimental evidence provided by Lee et al.22 They performed dispersion stability studies on ZnO NPs following TG318 and observed that ZnO is highly stable in low IS media and low hardness media (comparable to EPA soft) without NOM addition. They monitored stability higher than 90% for more than 48 h. In contrast to a mid IS medium with increased hardness, comparable to M7, complete sedimentation was achieved already in the first 6 h; upon NOM addition, a drastic increase in the stability was observed, up to 90% of particles were in dispersion for more than 48 h. This is in line with the significant increase in dissolution observed for ZnO in M7 media, shown in Fig. 5A.
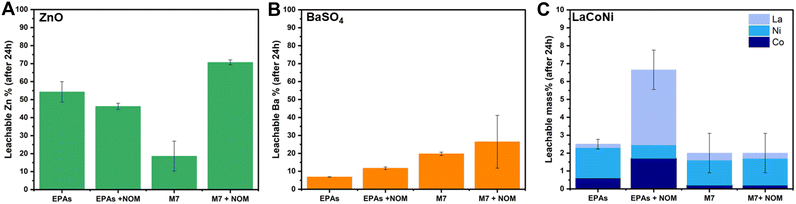 |
| Fig. 5 The influence of NOM addition on the total dissolution of ZnO (A), BaSO4 (B) and LaCoNi (C) during 24 hours of contact with two different aqueous media (EPA soft and M7) with and without the presence of natural organic matter (NOM, 10 mg L−1). The standard deviation between replicates is included (n = 2). For LaCoNi, the single elements contributing to the total leachable mass are highlighted. All results were obtained in the continuous flow system in dispersion injection mode with 100 μg material and a flow rate of 1 mL min−1. | |
For BaSO4, an increase in dissolution by NOM addition was observed in both media with a total amount of 12% and 30% in soft EPA and M7 media, respectively (Fig. 5B). This corresponds to 20% and 51%, respectively, of the total mass of barium in the material. Meagher et al. reported that BaSO4 tends to agglomerate in aqueous media due to a low, nearly isoelectric zeta potential at neutral pH.32 This suggests that NOM addition may play a role in particle stabilization, increasing the total amount of available particle surface in contact with the media though encouraging barium ion leaching. When it comes to LaCoNi (Fig. 5C), no relevant increase in dissolution took place in M7 upon addition of NOM. In the EPA medium, we observe a difference in the ion ratios in the presence or absence of NOM: the initial sample’s metal ion mass ratio is 0.75/0.15/0.1 (La/Co/Ni), the ratio in the EPA medium is 0.08/0.24/0.68, and the ratio in EPA + NOM is 0.64/0.26/0.10. The nickel concentration in the EPA medium is enriched relative to the starting material and depleted in the EPA + NOM medium. However, although the constituent metal ratios differ in the presence or absence of NOM in EPAs, by looking at the total mass dissolved, the observed differences in nickel, cobalt or lanthanum leached ions are less than 5%, therefore it is possible that the NOM concentration in solution is not high enough. Indeed, the results reported in section 3.6 suggest that 10 mg L−1 NOM in the different media was not able to prevent agglomeration in a 24 hour time period. Previous studies from Cupi et al. tested the addition of 5 mg L−1, 10 mg L−1, and 20 mg L−1 Suwannee River NOM in M7 medium, observing a material/metal dependent behaviour.13 This was further confirmed by Pradhan et al. which showed how NOM addition had a major effect on the dissolution rate of Cu and Al, from Cu NPs and Al NPs, respectively, whereas no significant effect was observed on the release of Mn from MnNPs.33 These findings may have concrete implications when carrying out ecotoxicity tests; indeed, Arvidsson et al. in a recent review discussed about the influence of NOM on the aquatic ecotoxicity of engineered nanoparticles. They found out that EC50, LC50, and IC50 values obtained in experiments with NOM present tended to be a factor of 1–10 higher than those without NOM.34 For ZnO NPs, ion induced toxicity is one of the most likely mode of action;35,36 hence, the increased dissolution upon NOM addition relates to the higher ecotoxicity values reported. For LaCoNi, the mode of action is not yet established. However, from the results obtained in this study, NOM addition does not seem to trigger further release of the more toxic Ni and Co ions. Thus, if the ecotoxicity of LaCoNi is mainly driven by ion release, similar ecotoxicity values is to be expected with and without NOM addition. In contrast, if the particles are the major driver for toxicity, a decrease in toxicity might be expected because of NOM adsorption on the surface of the particles through electrostatic and van der Waals interactions.
Dispersion stability of LaCoNi in ecotoxicity testing media as influenced by calcium and NOM content
Fig. 6 shows the dispersion stability results for LaCoNi, obtained by three methods in two test media, with and without NOM addition (i.e., EPA soft, EPA soft + NOM, M7, M7 + NOM). Additionally, specific TG318 conditions were screened with the standard detection methods and are reported in the ESI† (Fig. S4). Both detection methods recommended by TG318 for determining dispersion stability were used and compared (i.e., monitoring by UV-vis spectrophotometry for 24 hours and analysis of the supernatant during 6 hours by ICPMS, or ICPOES, after digestion). In addition, analytical ultracentrifugation (AUC) was used to assess dispersion stabilities via the directly measured distribution of sedimentation velocities. UV-vis spectrophotometry is a robust, easy to use and automated method. However, a limited number of samples can be run in a day and several weeks can be needed to screen the complete multi-dimensional environmental matrix recommended by OECD TG 318. Moreover, it is required that the sample absorb in the range between 200 and 800 nm, meaning that samples must be chromophores. The second method proposed by the guideline is the detection of the total metal content in the supernatant by ICPMS/ICPOES. Aliquots are taken manually from each test condition every hour for six hours. The main drawback of the method is that it is time consuming, poorly automated and operator dependent. Nevertheless, this method can be faster and high throughput, given that more samples can be run in a day by ICPMS/ICPOES. All considered, alternative detection methods which can merge advantages of both techniques are truly valuable. Analytical ultracentrifugation (AUC) is proposed as an alternative and pro and cons are evaluated. This technique has been explored for the dosimetry of ENMs in in vitro testing.37 The exact delivered doses of ENMs in cells were measured by AUC, allowing for re-calculation of dose–response curves. In analogy, we apply the same method and model, to evaluate the stability in dispersion of AdMa by measuring the sedimentation coefficients of single particles in the ENM dispersion. As shown in the table provided in Fig. 6, all three methods provide coherent results, ranking equally the conditions investigated. Addition of NOM plays a consistent stabilizing role for LaCoNi in the two test media investigated. Higher instability was observed for the perovskite in M7 media without the NOM presence. With addition of NOM, up to 70% of the perovskite was dispersed at the same point in time. For EPA soft, an increase in dispersion from 50–60% up to 70–80% was observed by adding NOM to the media. The AUC method indicated an increase of stability with nearly the same numerical values at the 6 h time point (Fig. 6), whereas the UV-vis method confirmed the trend, at lower numerical values, probably due to the overproportional light scattering of the largest agglomerates that sediment first.
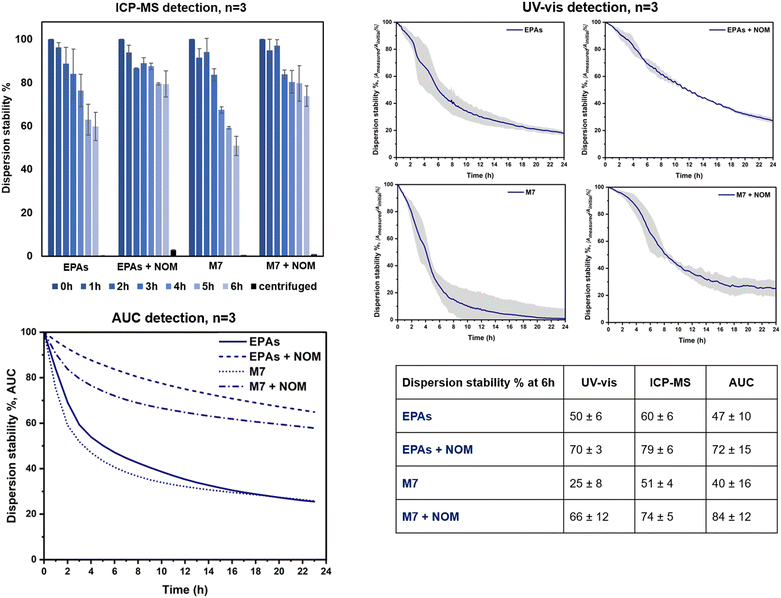 |
| Fig. 6 Dispersion stability of LaCoNi in two test media, with and without NOM (i.e., EPA soft, EPA soft + NOM, M7, M7 + NOM). Three different detection methods are compared: on the top left, ICPMS detection; on the top right, UV-vis detection; on the bottom left, AUC detection. All measurements were done in triplicate and standard deviations are reported in the table on the bottom right. | |
Even though stabilization was observed by NOM, it was not enough to keep more than half of the material dispersed for longer than 12 hours. This is in line with what was described in section 3.4 regarding the influence of the presence of NOM. Based on previous work, it seems possible that a higher NOM concentration (i.e., >10 mg L−1) could provide more stable dispersions.33 By considering the ICP-MS results, it is apparent that in the presence of NOM, a small fraction of dissolved material is still present in solution after centrifugation. Centrifugation conditions (time and rpm) were optimized specifically for LaCoNi, by using the OECD TG318 excel worksheet, so that no particle is supposed to be found in the supernatant. It is known that NOM facilitates metal transport in aqueous systems, forming metal–NOM complexes.38 In our case, we find absolute agreement between the dissolution studies in the EPA soft test media and the dissolved fraction found in the supernatant during the dispersion stability studies. Indeed, we used lanthanum as a tracer to determine dispersion stability in time (Fig. 6). Approximately 4–5% of lanthanum is found in the supernatant fraction (i.e., after removal of particles by sedimentation) of EPA soft test media in the presence of NOM. Less than 1% is dissolved in the remaining three test media. This perfectly fits in ranking with the experimental data from the continuous flow system shown in Fig. 5C.
In TG318, calcium nitrate (Ca(NO3)2) has been chosen as the major electrolyte, influencing the stability of particles in dispersion, due to the dominant effect of multivalent cations (in this case Ca2+) on particle aggregation. NOM has the ability to create complexes with cations, reducing the activity of calcium in the medium, thereby reducing the de-stabilizing effect of calcium. Fig. 7 shows the dispersion stability of LaCoNi in the first 12 hours of the experiment, calculated as the ratio between the measured absorbance and the initial absorbance, read using a UV-vis spectrometer. Measurements were performed for all conditions in the full 24-hour time range and are reported in the ESI.† It is clearly visible how the increase of calcium in the media strongly destabilizes the LaCoNi suspensions. In the absence of NOM, the dispersion stability drops to less than 10% already in the first 6 hours, when more than 1 mM calcium is in solution (Fig. 7A). In the presence of NOM, destabilization is attenuated and the contribution of calcium content to destabilization shows a clear trend: EPAs +NOM > 1mmCa > M7 +NOM > 10 mM Ca (Fig. 7B). Variation in pH from 7 to 9 did not play a role nor in the stabilization or destabilization of LaCoNi particles. The hardness calculated for the media EPA soft and M7, respectively 0.4 mM and 2.5 mM, lies within the range for the standard conditions recommended in the TG318 guideline.
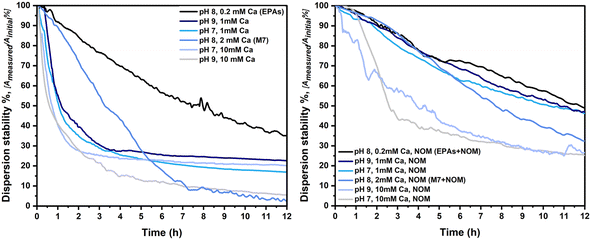 |
| Fig. 7 On the right, influence of calcium concentration and pH; on the left, additional presence of NOM and influence on the dispersion stability of LaCoNi (10 mg L−1) measured by UV-vis. The dispersion stability is expressed as the ratio (in %) between the measured absorbance and the initial absorbance. OECD TG318 standard test conditions (1 mM and 10 mM Ca at pH 7 and 9) are compared with the results found for the test media EPA soft and M7 with and without NOM. | |
Conclusions
Based on the results obtained with two benchmark materials (ZnO and BaSO4) and three AdMa, we recommend the use of a continuous flow system for establishing dissolution kinetics of AdMa under environmentally relevant conditions (i.e., low material loading and high flow rates). It is easy to operate and eco-friendly as all the main components are ready to be re-used after each cycle with minimal waste. Our study showed that, depending on the material in analysis, dissolution rates were related to the materials’ stability in dispersion.
For dispersible materials with medium to slow dissolution kinetics, we found that the dispersion loading method was easier to operate and offered higher precision in the rate constant determination. When dealing with fast dissolving materials, like ZnO, the dispersion loading method is challenged by the fast initial dissolution kinetics and here complementary use of the powder loading method is recommended, as it deposits the material in a single layer on the filter used in the continuous flow system, avoiding agglomeration issues during the dispersion/injection time frame. However, to achieve high accuracy and precision in the weighted particle mass on the scale, higher mass loading is required (i.e., order of mg).
We found that the presence of NOM is likely to decrease agglomeration of the study materials investigated, though at the same time, increasing the fraction of leachable ions at a given ionic strength and water hardness of the test medium. This can be of primary importance for ecotoxicologists, when planning aquatic toxicity experiments, as in many cases, the toxicity of metal-containing materials is linked to the metal ion release. In support of GD318, we suggest the combination of the methods used in the present study for dissolution and dispersion stability, as a useful pre-screening tool for scientists working in the environmental hazard assessment of AdMa; this combination gives a fast and clear overview of the basic extrinsic physicochemical properties influencing the behaviour of AdMa in the environment.
Furthermore, we propose the use of analytical ultracentrifugation as a useful pre-screening tool for ecotoxicologists, allowing for another line of evidence on the role of NOM in dispersion stability. The main advantage of the method is that it is fast and depends minimally on the operator. Results can be obtained in less than an hour, allowing for a high throughput screening of different test conditions. However, with a replicate standard deviation of 10–15%, it was not as precise as the two standard methods (UV-vis and ICPMS). Nevertheless, it clearly offered the possibility of discriminating between stable and unstable dispersions in two simulant media.
Our results are documented on eNanomapper templates, meant to be accessible to the public and therefore reusable in the future; this contributes to establishing data for early screening in the environmental safety of perovskites, as an example of novel advanced materials of future high importance. For metal containing AdMa, dissolution and dispersion stability data are relevant for establishing design principles in Safe and Sustainable by Design (SSbD) practices.
Author contributions
Veronica Di Battista: conceptualization; data curation; formal analysis; investigation; methodology; visualization; writing – original draft. Kai Werle: methodology. Lars Skjolding: conceptualization; supervision; writing – review and editing. Wendel Wohlleben: conceptualization; project administration; resources; supervision; writing – review and editing. Anders Baun: conceptualization; project administration; resources; supervision; writing – review and editing.
Conflicts of interest
WW is an employee of BASF, a company producing catalysts. There are no other conflicts to declare.
Acknowledgements
The research for this work has received funding from the European Union's Horizon 2020 Research and Innovation Programme under grant agreement no. 953183 (HARMLESS). We thank Nina Jeliazkova for her support with the collection of data in eNanomapper templates.
Notes and references
- D. Bosteels and R. A. Searles, Exhaust emission catalyst technology: new challenges and opportunities in Europe, Platinum Met. Rev., 2002, 46, 27–36 CAS.
- T. Selleri, A. D. Melas, A. Joshi, D. Manara, A. Perujo and R. Suarez-Bertoa, An overview of lean exhaust denox aftertreatment technologies and nox emission regulations in the european union, Catalysts, 2021, 11, 404 CrossRef CAS.
- EC, Commission proposes new Euro 7 standards to reduce pollutant emissions from vehicles and improve air quality, can be found under https://ec.europa.eu/commission/presscorner/detail/en/ip_22_6495.Journal, 2022.
- K. N. Islam, J. Hildenbrand and M. M. Hossain, Life cycle impacts of three-way ceramic honeycomb catalytic converter in terms of disability adjusted life year, J. Cleaner Prod., 2018, 182, 600–615 CrossRef CAS.
- N. Bahaloo-Horeh and S. M. Mousavi, Comprehensive characterization and environmental risk assessment of end-of-life automotive catalytic converters to arrange a sustainable roadmap for future recycling practices, J. Hazard. Mater., 2020, 400, 123186 CrossRef CAS PubMed.
- A. Kennedy, J. Brame, T. Rycroft, M. Wood, V. Zemba, C. Weiss Jr., M. Hull, C. Hill, C. Geraci and I. Linkov, A Definition and Categorization System for Advanced Materials: The Foundation for Risk-Informed Environmental Health and Safety Testing, Risk Anal., 2019, 39, 1783–1795 CrossRef PubMed.
-
EC, Commission Regulation (EU) 2018/1881 of 3 December 2018 amending Regulation (EC) No 1907/2006 of the European Parliament and of the Council on the Registration, Evaluation, Authorisation and Restriction of Chemicals (REACH) as regards Annexes I, III,VI, VII, VIII, IX, X, XI, and XII to address nanoforms of substances, it can be found under https://eur-lex.europa.eu/legal-content/EN/TXT/PDF/?uri=CELEX:32018R1881, 2018.
- A. Baun, P. Sayre, K. G. Steinhäuser and J. Rose, Regulatory relevant and reliable methods and data for determining the environmental fate of manufactured nanomaterials, NanoImpact, 2017, 8, 1–10 CrossRef.
-
OECD, GD318: Guidance Document for the testing of dissolution and dispersion stability and the use of the data for further environemntal testing and assessing strategies, it can be found under https://one.oecd.org/document/ENV/JM/MONO(2020)9/en/pdf, 2020.
-
OECD, GD318: Guidance Document for the testing of dissolution and dispersion stability and the use of the data for further environemntal testing and assessing strategies, it can be found under https://one.oecd.org/document/ENV/JM/MONO(2020)9/en/pdf, 2020.
- W. K. Dodds, J. P. Guinnip, A. E. Schechner, P. J. Pfaff and E. B. Smith, Fate and toxicity of engineered nanomaterials in the environment: A meta-analysis, Sci. Total Environ., 2021, 796, 148843 CrossRef CAS PubMed.
- K. Hund-Rinke, A. Baun, D. Cupi, T. F. Fernandes, R. Handy, J. H. Kinross, J. M. Navas, W. Peijnenburg, K. Schlich and B. J. Shaw, Regulatory ecotoxicity testing of nanomaterials–proposed modifications of OECD test guidelines based on laboratory experience with silver and titanium dioxide nanoparticles, Nanotoxicology, 2016, 10, 1442–1447 CrossRef CAS PubMed.
- D. Cupi, N. B. Hartmann and A. Baun, The influence of natural organic matter and aging on suspension stability in guideline toxicity testing of silver, zinc oxide, and titanium dioxide nanoparticles with Daphnia magna, Environ. Toxicol. Chem., 2015, 34, 497–506 CrossRef CAS PubMed.
-
K. Jensen, Y. Kembouche, E. Christiansen, N. Jacobsen, H. Wallin, C. Guiot, O. Spalla and O. Witschger, The generic NANOGENOTOX dispersion protocol—standard operation procedure (SOP), it can be found under https://webgate.ec.europa.eu/chafea_pdb/assets/files/pdb/20092101/20092101_d03-00_en_ps.pdf, 2011, p. 3.
- L. Stetten, A. Mackevica, N. Tepe, T. Hofmann and F. von der Kammer, Towards Standardization for Determining Dissolution Kinetics of Nanomaterials in Natural Aquatic Environments: Continuous Flow Dissolution of Ag Nanoparticles, Nanomaterials, 2022, 12, 519 CrossRef CAS PubMed.
-
C. I. Weber, Methods for measuring the acute toxicity of effluents and receiving waters to freshwater and marine organisms, it can be found under https://www.epa.gov/sites/default/files/2015-08/documents/acute-freshwater-and-marine-wet-manual_2002.pdf, 1991.
-
OECD, Test Guideline No. 211: Daphnia Magna Reproduction Test, it can be found under https://www.oecd-ilibrary.org/docserver/9789264185203-en.pdf?expires=1702454406&id=id&accname=guest&checksum=278CD92A3A9CAE2FFD02CC37FCF8BABB, 2012.
-
OECD, Test No. 249: Fish Cell Line Acute Toxicity-The RTgill-W1 Cell Line Assay, it can be found under https://www.oecd-ilibrary.org/docserver/c66d5190-en.pdf?expires=1702454373&id=id&accname=guest&checksum=D3343F99B8B6F1C08BA4EFA6B6CEC25C, 2021.
- Y. Yue, X. Li, L. Sigg, M. J. Suter, S. Pillai, R. Behra and K. Schirmer, Interaction of silver nanoparticles with algae and fish cells: a side by side comparison, J. Nanobiotechnol., 2017, 15, 1–11 CrossRef PubMed.
- J. G. Keller, U. M. Graham, J. Koltermann-Jülly, R. Gelein, L. Ma-Hock, R. Landsiedel, M. Wiemann, G. Oberdörster, A. Elder and W. Wohlleben, Predicting dissolution and transformation of inhaled nanoparticles in the lung using abiotic flow cells: The case of barium sulfate, Sci. Rep., 2020, 10, 458 CrossRef CAS PubMed.
- W. Utembe, K. Potgieter, A. B. Stefaniak and M. Gulumian, Dissolution and biodurability: Important parameters needed for risk assessment of nanomaterials, Part. Fibre Toxicol., 2015, 12, 11 CrossRef PubMed.
- B.-C. Lee, G. Hong, H. Lee, P. Kim, D.-Y. Seo, G. Hwang, G. Kim and P. Kim, Influence of NOM on the stability of zinc oxide nanoparticles in ecotoxicity tests, Appl. Sci., 2020, 10, 6431 CrossRef CAS.
- U. G. Sauer, A. Aumann, L. Ma-Hock, R. Landsiedel and W. Wohlleben, Influence of dispersive agent on nanomaterial agglomeration and implications for biological effects in vivo or in vitro, Toxicol. In Vitro, 2015, 29, 182–186 CrossRef CAS PubMed.
-
OECD, PD ISO/TR 19057:2017 - Nanotechnologies. Use and application of acellular in vitro tests and methodologies to assess nanomaterial biodurability, it can be found under https://shop.bsigroup.com/en/ProductDetail/?pid=000000000030348648, 2017.
- J. G. Keller, W. Peijnenburg, K. Werle, R. Landsiedel and W. Wohlleben, Understanding Dissolution Rates via Continuous Flow Systems with Physiologically Relevant Metal Ion Saturation in Lysosome, Nanomaterials, 2020, 10, 311 CrossRef CAS PubMed.
- J. Keller, M. Persson, P. Müller, L. Ma-Hock, K. Werle, J. Arts, R. Landsiedel and W. Wohlleben, Variation in dissolution behavior among different nanoforms and its implication for grouping approaches in inhalation toxicity, NanoImpact, 2021, 100341 CrossRef CAS PubMed.
- T. Mercer, On the role of particle size in the dissolution of lung burdens, Health Phys., 1967, 13, 1211–1222 CrossRef CAS PubMed.
- Y. Chen, Y. Sun, M. Wang, J. Wang, H. Li, S. Xi, C. Wei, P. Xi, G. E. Sterbinsky and J. W. Freeland, Lattice site–dependent metal leaching in perovskites toward a honeycomb-like water oxidation catalyst, Sci. Adv., 2021, 7, 1788 CrossRef PubMed.
- M. Kowacz and A. Putnis, The effect of specific background electrolytes on water structure and solute hydration: Consequences for crystal dissolution and growth, Geochim. Cosmochim. Acta, 2008, 72, 4476–4487 CrossRef CAS.
- A. Philippe and G. E. Schaumann, Interactions of dissolved organic matter with natural and engineered inorganic colloids: a review, Environ. Sci. Technol., 2014, 48, 8946–8962 CrossRef CAS PubMed.
- M. O. Fatehah, H. A. Aziz and S. Stoll, Stability of ZnO nanoparticles in solution. Influence of pH, dissolution, aggregation and disaggregation effects, J. Colloid Sci. Biotechnol., 2014, 3, 75–84 CrossRef CAS.
- M. J. Meagher, B. Leone, T. L. Turnbull, R. D. Ross, Z. Zhang and R. K. Roeder, Dextran-encapsulated barium sulfate nanoparticles prepared for aqueous dispersion as an X-ray contrast agent, J. Nanopart. Res., 2013, 15, 1–10 CrossRef.
- S. Pradhan, J. Hedberg, J. Rosenqvist, C. M. Jonsson, S. Wold, E. Blomberg and I. Odnevall Wallinder, Influence of humic acid and dihydroxy benzoic acid on the agglomeration, adsorption, sedimentation and dissolution of copper, manganese, aluminum and silica nanoparticles–A tentative exposure scenario, PLoS One, 2018, 13, e0192553 CrossRef PubMed.
- R. Arvidsson, S. F. Hansen and A. Baun, Influence of natural organic matter on the aquatic ecotoxicity of engineered nanoparticles: Recommendations for environmental risk assessment, NanoImpact, 2020, 100263 CrossRef.
- N. M. Franklin, N. J. Rogers, S. C. Apte, G. E. Batley, G. E. Gadd and P. S. Casey, Comparative toxicity of nanoparticulate ZnO, bulk ZnO, and ZnCl2 to a freshwater microalga (Pseudokirchneriella subcapitata): the importance of particle solubility, Environ. Sci. Technol., 2007, 41, 8484–8490 CrossRef CAS PubMed.
- M. Heinlaan, A. Ivask, I. Blinova, H.-C. Dubourguier and A. Kahru, Toxicity of nanosized and bulk ZnO, CuO and TiO2 to bacteria Vibrio fischeri and crustaceans Daphnia magna and Thamnocephalus platyurus, Chemosphere, 2008, 71, 1308–1316 CrossRef CAS PubMed.
- J. G. Keller, D. F. Quevedo, L. Faccani, A. L. Costa, R. Landsiedel, K. Werle and W. Wohlleben, Dosimetry in vitro–exploring the sensitivity of deposited dose predictions vs. affinity, polydispersity, freeze-thawing, and analytical methods, Nanotoxicology, 2021, 15, 21–34 CrossRef CAS PubMed.
- D. Schmitt, F. Saravia, F. Frimmel and W. Schuessler, NOM-facilitated transport of metal ions in aquifers: importance of complex-dissociation kinetics and colloid formation, Water Res., 2003, 37, 3541–3550 CrossRef CAS PubMed.
|
This journal is © The Royal Society of Chemistry 2024 |
Click here to see how this site uses Cookies. View our privacy policy here.