DOI:
10.1039/D4RA02564D
(Review Article)
RSC Adv., 2024,
14, 21915-21937
Unveiling the potential of photodynamic therapy with nanocarriers as a compelling therapeutic approach for skin cancer treatment: current explorations and insights
Received
5th April 2024
, Accepted 2nd July 2024
First published on 10th July 2024
Abstract
Skin carcinoma is one of the most prevalent types of carcinomas. Due to high incidence of side effects in conventional therapies (radiotherapy and chemotherapy), photodynamic therapy (PDT) has gained huge attention as an alternate treatment strategy. PDT involves the administration of photosensitizers (PS) to carcinoma cells which produce reactive oxygen species (ROS) on irradiation by specific wavelengths of light that result in cancer cells' death via apoptosis, autophagy, or necrosis. Topical delivery of PS to the skin cancer cells at the required concentration is a challenge due to the compounds' innate physicochemical characteristics. Nanocarriers have been observed to improve skin permeability and enhance the therapeutic efficiency of PDT. Polymeric nanoparticles (NPs), metallic NPs, and lipid nanocarriers have been reported to carry PS successfully with minimal side effects and high effectiveness in both melanoma and non-melanoma skin cancers. Advanced carriers such as quantum dots, microneedles, and cubosomes have also been addressed with reported studies to show their scope of use in PDT-assisted skin cancer treatment. In this review, nanocarrier-aided PDT in skin cancer therapies has been discussed with clinical trials and patents. Additionally, novel nanocarriers that are being investigated in PDT are also covered with their future prospects in skin carcinoma treatment.
1 Introduction
Skin carcinoma is the fourth most common type of cancer in the whole world. The main two subtypes that cover 95% of all cases are melanoma and non-melanoma skin cancer. The remaining is caused by a few extremely rare and aggressive subtypes of skin cancer.1–3 Due to various contributing factors, the occurrence of skin cancers has been increasing steadily over the past few decades. The exponentially increasing prevalence rate of skin cancer is alarming. Non-melanoma skin cancer is more prevalent than melanoma skin cancer and it exhibits four times greater occurrence than melanoma. In contrast, as melanoma shows a higher chance of metastasizing and resistance to therapies, it is associated with 70% of all skin cancer-related deaths.1,4
The strategies for the therapy of skin cancer vastly depend on the type of skin cancer. Excision biopsy stands as the primary treatment modality for skin malignancies. However, certain non-melanoma skin cancers (NMSC) not amenable to elliptical excision necessitate alternative approaches.5 These include curettage and diathermy, liquid nitrogen, imiquimod, 5-fluorouracil (5FU), radiotherapy, or excision with flap repair/grafting. Notably, for facial lesions, excision or radiotherapy are preferred options due to cosmetic considerations. Electrodesiccation and curettage or diathermy are advantageous for superficial basal cell carcinomas (BCCs) while liquid nitrogen is suitable for superficial lesions on the trunk and extremities. Topical agents such as 5-FU and imiquimod are indicated for Bowen's disease and superficial BCCs, respectively, in cases where surgical intervention is not feasible. Radiotherapy, typically reserved for elderly patients, offers a high cure rate and is particularly beneficial for margin control or treating extensive or anatomically challenging lesions. However, excision or Mohs micrographic surgery remains the preferred approach whenever feasible, especially for tumors with unclear margins, recurrence, or complex anatomical locations.6–8 The extensive array of unfavorable factors linked with each of these conditions prompted researchers to develop safer, patient-friendly, novel alternatives such as photothermal therapy (PTT), sonodynamic therapy, and photodynamic therapy (PDT).9 Conditions like patient willingness and the delicate position of the tumor determine whether surgical intervention is appropriate for a patient or not. Surgical excision is not recommended on low-risk tumors either.10,11 Radiotherapy is an alternative where surgeries cannot be performed. The only drawback of radiation therapy is the high incidence of radiation-mediated adverse effects. The immediate post-radiation adverse effects include skin toxicity, structural changes, and in cell morphology whereas the late adverse effects may include cartilage necrosis, secondary malignancy, and alopecia. Lastly, the most followed approach is chemotherapy. Despite the high occurrence of severe adverse effects, the use of chemotherapeutic agents alone or in combination with other strategies is the most conventional form of treatment for skin cancer.12,13 These chemical agents are used in combination due to their ability to block one or more cancer development pathways completely. Using chemotherapeutic agents in low doses alongside PDT and PTT, novel therapies is a promising upcoming practice. In Fig. 1, illustrates skin anatomy highlighting localization sites of various skin cancer types, the potential routes for nanocarriers targeting melanoma and non-melanoma skin cancers and the distinct challenges in targeting each type.
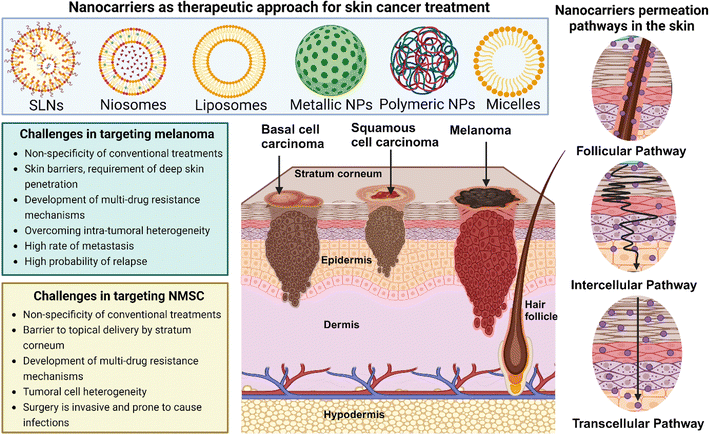 |
| Fig. 1 Schematic representation of skin anatomy highlighting localization sites of various skin cancer types. Illustration of the potential routes for nanocarriers targeting melanoma and non-melanoma skin cancers and the distinct challenges in targeting each type. Created with https://Biorender.com. | |
PDT is a comparatively newly developed non-invasive strategy, the effectiveness of which depends on three elements; light source, presence of oxygen in the cellular microenvironment, and photosensitizers (PS). The PS gets activated by light and produces reactive oxygen species (ROS) that obliterate cancer cells (Fig. 2).14 The excessive thickness of the tumor, more collagen content inside the tumor, ulceration, infiltrative growth tissue, and the presence of melanin can cause resistance to PDT.7,15 However, the very low chances of severe side effects associated with this therapy makes it appealing. Most skin cancer cases are not life-threatening if diagnosed early. PDT offers the perfect treatment strategy against minor to moderately severe skin cancers like basal cell carcinoma (BCC), squamous cell carcinoma (SCC), and early-stage melanomas as surgery in such cases shows very little patient compliance and surgical intervention may complicate the case further.16,17 A non-invasive strategy is therefore a suitable approach. In metastasizing skin cancers like malignant melanoma (MM), nanocarrier-mediated cellular targeting alongside PDT has shown promising results decreasing the need of surgical intervention and chemotherapy in patients.18,19 In this review, the benefits of nanocarrier-aided PDT in the treatment of the three most prevalent types of skin cancers, BCC, SCC, and MM are discussed thoroughly with proper scientific proof. The mechanism of PDT is explained in detail to enhance comprehension of how it eliminates cancer cells and to display the potential approaches for combination therapies. Additionally, the current patent scenario and clinical trial status are discussed to give an idea about the present-day significance of PDT in the medical and pharmaceutical field.
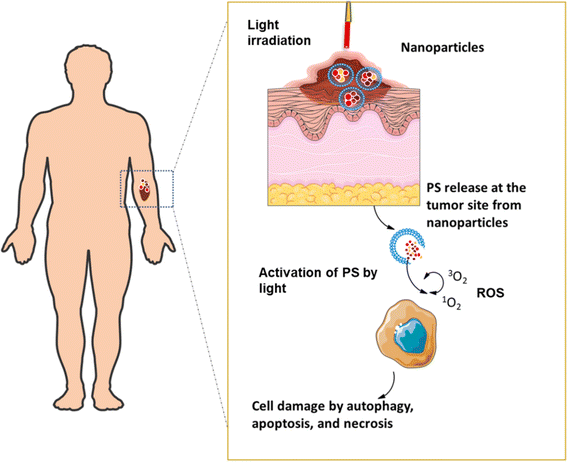 |
| Fig. 2 Topical application of PS using nanocarriers in PDT. | |
2 Mechanistic aspects of photodynamic therapy
2.1 Mechanism of photodynamic therapy
The mechanism of PDT comprises three key elements i.e., light source, photosensitizers, and presence of ROS at tumor microenvironment. Photosensitizers are molecules that are excited by light of a particular wavelength and generate ROS in the cellular microenvironment. Fig. 3 illustrates the mechanism of PDT. Any photosensitizer molecule at its ground state carries two oppositely spinning electrons in a low-energy molecular orbital.20 This is called the singlet state. After it absorbs light energy, one such electron jumps to a higher energy state and withholds its initial spin. This is the excited singlet state which cannot retain its energy for a long time and releases it either by emitting fluorescent light or by converting it to heat.21 In another possibility, the electron from the excited singlet state reverses its spin and forms a more stable excited triplet state with two electrons in parallel spins.22,23 Although stable, this excited triplet state follows two paths of reaction. In type 1 reaction, this directly interacts with a molecule or the cell membrane. The shift of an electron or a proton creates a radical cation or anion respectively. The further reaction between the radicals and oxygen results in ROS.24,25 In type 2 reaction, the electron transfer happens from the excited triplet state to the molecular oxygen directly i.e. an excited state singlet oxygen. Both of these oxygen species resulting from the simultaneously occurring reactions 1 and 2 are highly reactive and short-lived. Thus, they only react to the proximal area of the production allowing a site-specific therapy. The healthy cells near the cancerous cells don't get affected significantly.26–28 There are a few studies that have mentioned the presence of a third type of reaction mechanism. In type 1, where the PS at the excited triplet state reacts with adjacent cellular materials, the presence of oxygen may not be necessary to elucidate action. The energy transfer may happen between the excited PS molecules and the biological substrate directly, leading to their destruction in the cellular microenvironment. In solid tumors residing in hypoxic milieu, this type of reaction may happen.29
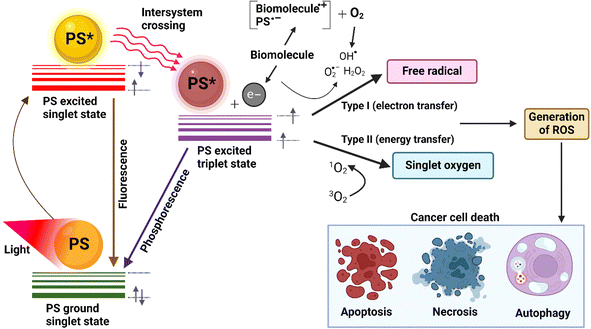 |
| Fig. 3 Jablonski diagram illustrating the excitation states and energy transfer processes of photosensitizers. Created with https://Biorender.com. | |
2.2 Timeline of photosensitizers
PS are not classified based on some inherent physicochemical parameters. They are mostly showcased in different generations, the first generation of PS being the very first molecules that showed in vivo ROS generating activities and thus were involved in the development of PDT. Due to further extensive research, a new set of molecules immerged as the second generation of PS while overcoming most of the drawbacks showed by the previous candidates. The first generation of PS consists of hydrophilic hematoporphyrin derivatives which were obtained by chemical modification of hematoporphyrin, the first ever porphyrin used as a PS.30 These first-generation PS molecules showed substandard chemical purity, and due to their long half-lives accompanied with higher tendency of skin accumulation, prolonged photosensitivity was reported.31 The light with smaller wavelength has limited tissue penetrability which is a challenge as their activation wavelength is low. Whereas, in the case of second-generation PS, the activation wavelength is on the higher range that is in the red and far-red wavelength range that results in deeper tissue penetration. This characteristic shift in higher wavelength activation was achieved by chemically modifying the macrocycles and the substituents of the first-generation PS molecules. The synthetic derivatives also showed improved selectivity, and faster elimination.32 5-Aminolevulinic acid (5-ALA) is one of the most frequently used second-generation PS. 5-ALA functions as a precursor in the heme biosynthesis pathway, which is integral to its photosensitizing properties. Upon exogenous administration, 5-ALA leads to the intracellular accumulation of protoporphyrin IX (PpIX). In this pathway, 5-ALA undergoes enzymatic conversion, with two 5-ALA molecules condensing to form porphobilinogen. This compound then sequentially transforms into hydroxymethylbilane, uroporphyrinogen III, coproporphyrinogen III, and finally protoporphyrinogen IX, culminating in the production of PpIX. Interestingly, PpIX has been utilized independently as a PS in several research studies.33,34 The main drawback of these molecules is their low water-solubility which poses a significant challenge in intravenous applications. However, in case of topical application which is prevalent in skin cancer therapy, second generation PS are still in use.35,36 The third generation of PS has been engineered to enhance tissue selectivity and minimize side effects. This objective has been accomplished through conjugation with targeting molecules that bind to specific receptors or through incorporation into nanoplatforms to facilitate enhanced delivery.37 For example, in a study by Kataoka et al., the Warburg effect was utilized to develop a third generation PS with better selectivity. The Warburg effect is a phenomenon characterized by cancer cells displaying an elevated uptake of glucose via glucose transporters, GLUT 1, 3 and 4 compared to that of normal cells. The scientists conjugated four molecules of D-glucose with a PS, chlorin to prepare G-chlorin. Additionally, they also synthesized M-chlorin and maltotriose-conjugated chlorin by conjugating four molecules of D-mannose and four molecules of maltotriose respectively. M-chlorin was observed to target the tumor associated macrophages and maltotriose-conjugated chlorin was investigated for its hydrophilic behavior. The newly modified G-chlorin showed 20–50 times more cytotoxicity than talaporfin (used as standard) in gastric and colon cancer.38 Given that many first-generation photosensitizers are no longer in use and third-generation ones often require extensive chemical modification and complex synthesis, studies involving second-generation photosensitizers are more prevalent. However, this review prioritizes individual studies over the specific PS utilized. Promising outcomes, even with the use of early generation PS molecules, were deemed noteworthy and thus included.
2.3 Molecular pathway of cell destruction by photodynamic therapy
There are three pathways following which PDT can cause cell death. Apoptosis is called programmed cell death as it does not disturb any adjacent cells or elicit any inflammatory response. Apoptosis generally happens when the apoptosis inducing factor gets triggered and further initiates chromatin condensation, cell shrinkage, cell surface blebbing, and DNA fragmentation. Apoptosis by PDT either follows the death receptor pathway or the mitochondrial pathway. However, caspase activation is common in both. PDT directly influences the Fas L death receptor which in turn binds with FADD proteins inside the cell. FADD interacts with caspase 8. BH3-only proteins like Bid protein are a natural substrate for caspase 8. BH3-only proteins are the connecting step between the extrinsic death receptor pathway and the intrinsic mitochondrial pathway. The activation of mitochondrial pathway occurs by BH3-only proteins, excessive Ca2+ in the cytoplasm, or increases ROS. Mitochondria releases Bcl-2-controlled pro-apoptotic molecules such as cytochrome C (cyt C). Cyt C acts as a co-factor and forms a complex with pro-caspase 9 and APAF-1 with the help of dATP/ATP. This activates caspase 9, the primary precursor of cell death. Caspase 9 also gets activated through the cysteine-cathepsin lysosomal pathway. Caspase 9 activates other caspases (caspase 3, 6, and 7) and ultimately leads the cell toward apoptosis (Fig. 4). Unlike apoptosis, in necrosis, cellular contents get expelled out in the intercellular spaces thus creating a strong immune response that can further escalate to inflammation. This happens when the light intensity of PDT or the dose of PS is too high, crossing the cellular threshold of resistance to non-physiological disturbances. Ca2+, Na+, and water influx inside the cell from extracellular space increases cell volume and disrupts cell membrane integrity. Depolarisation of the mitochondrial membrane creates ion imbalance. Disruption of the endoplasmic reticulum releases Ca2+ inside the cell uncontrollably. Activation of DNA nucleases and release of lysosomal hydrolytic enzymes lead to complete cell lysis. Lastly, autophagy is a process of selective degradation of cell organelles with double membranes via the formation of a special structure called the autophagosome. Lysosome merges with autophagosome and degrades the inner content. It is still not clear how PDT triggers autophagy, and if autophagy at all always stays associated with cell death. Some studies have related cellular survival with autophagy. Nevertheless, this surely elicits an immune response, and thus photodamage may trigger additional immune responses that are involved in cancer control.26,39
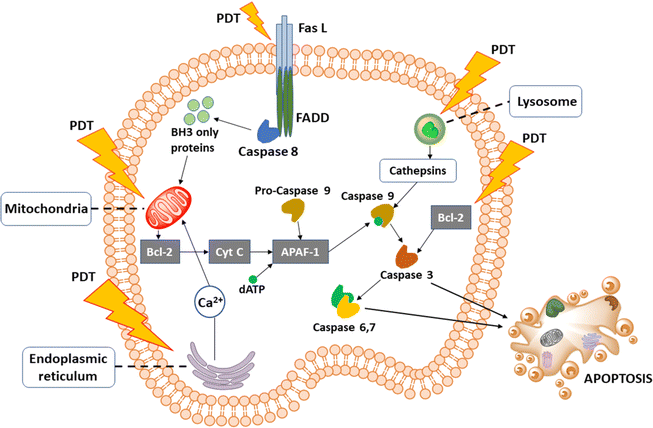 |
| Fig. 4 Cellular signaling pathways initiated by PDT. PDT shows a direct effect on cell membrane receptors, mitochondria, endoplasmic reticulum, and lysosomes. | |
3 Nanocarriers as delivery vehicles in photodynamic therapy
PDT is a scientifically established treatment that holds huge potential as a non-invasive strategy for curing different types of cancers. PDT is mainly used in association with other therapies for improved efficacy and lesser incidence of drug resistance. For example, a combination of PDT with imiquimod has been used in the treatment of BCC.40,41 Moreover, PDT is preferred over other available anti-cancer treatment strategies due to various reasons, the most important being the specificity that PDT offers. Accurate tumor site targeting can be achieved with a precise light source and a proper PS molecule. Currently, a large number of phototoxic compounds are being tested as potential PS in PDT.42 PDT is an efficient strategy for tumor treatment, however, the low solubility and low stability of the PS often limit its biomedical application.43 Nevertheless, the majority of these PS exhibit lipophilic property that can significantly influence their preferential accumulation in cellular hydrophobic regions, as they must traverse lipid membranes to enter cells. However, their limited solubility in water greatly impedes their intravenous administration.44,45 Additionally, side effects like skin photosensitivity and reduced effectiveness have been reported while using free PS molecules in PDT. To enhance PS performance in PDT, a new approach was needed that can ensure targeted delivery, prolonged circulation, improved tumor accumulation, and increased cancer cell uptake. Early attempts with lipid and organic formulations faced issues like systemic toxicity and abnormal biodistribution. This led to research in the field of nanoscience and nanotechnology for designing an ideal vehicle for PS in PDT.42,46
Topical therapy in skin cancer management is invaluable due to its minimally invasive nature, offering localized treatment with reduced systemic side effects. It enables direct application of therapeutic agents to the affected skin area, making it particularly suitable for superficial lesions and precancerous conditions.47,48 Additionally, topical treatments are often well-tolerated by patients, enhancing compliance and treatment outcomes.49,50 In the context of PDT, topical application of photosensitizers allows for precise targeting of malignant cells while sparing healthy tissue, making it an attractive option for superficial skin cancers like basal cell carcinoma and actinic keratosis.51,52 When combined with nanocarriers, the treatment strategy becomes superior as the delivery of photosensitizers gets optimized. Inadequate tissue penetration poses challenges to PDT effectiveness, which nanocarriers address by encapsulating photosensitizers, ensuring their stability, and facilitating active targeting of cancerous cells.53,54 Surface modifications enable precise drug delivery, minimizing off-target effects, while also enabling deeper penetration into skin layers, surmounting barriers like the stratum corneum [one]. Controlled drug release mechanisms in responsive nanocarriers minimize collateral damage, while the ability to co-deliver photosensitizers with other agents allows for synergistic therapeutic effects.55 The integration of nanocarriers into PDT holds significant promise for personalized and efficacious skin cancer treatment strategies, underscoring the importance of ongoing research in nanotechnology to fully leverage their potential. In this review, all the topically administered nanocarriers (Fig. 5) that have been thoroughly investigated with PDT against skin cancer are discussed systemically to showcase the advantages of such therapy and the huge scope that awaits in the future. Additionally, in vitro studies that focused on the efficacy of a certain chemical entity against any form of skin cancer while used with PDT are referred.
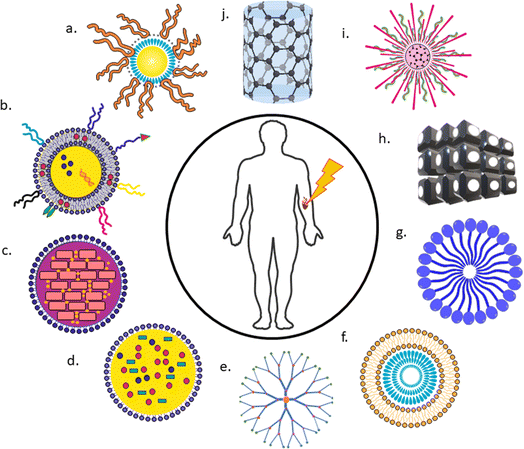 |
| Fig. 5 Nanocarriers as delivery vehicles in PDT. (a) Polymeric nanoparticle, (b) liposomes, (c) solid lipid nanoparticle, (d) nanostructured lipid nanocarrier, (e) dendrimer, (f) ethosomes, (g) micelles, (h) cubosomes, (i) quantum dot, (j) carbon nanotubes. | |
3.1 Polymeric and micellar nanoparticles
Polymeric nanocarriers present substantial advantages in topical formulations owing to their capacity to ameliorate drug solubility, augment cutaneous permeation, and furnish controlled drug release kinetics. Their nanoscale dimensions facilitate enhanced dermal drug deposition, mitigating systemic uptake and associated adverse reactions, rendering them a propitious choice for precise and efficacious skin cancer therapy.56 With polymers, the physical, chemical, as well as biological properties of nanocarriers, can be customized according to specific needs. Biodegradable polymers are more convenient as these break down in physiological condition that in turn reduces the chance of severe toxicity and facilitate complete drug release.57 Polymers can be of natural origin like poly(hydroxyalkanoates) or synthetic like poly(orthoesters), poly(β-amino esters). Poly(α-hydroxy esters) is a significant group of synthetic polymers that include poly (D, L-lactide) (PLA) and poly(glycolide) (PGA).55,58
In several studies, both PLA, as well as PLGA NPs, were modified by various methods and evaluated for better biodistribution, release kinetics, and other specific requirements. Silva et. al. investigated PLGA-encapsulated PpIX with free PpIX for topical melanoma therapy. Results revealed that singlet oxygen production remained unaffected by NPs. Both free and NP-formulated with 3.91 μg mL−1 PpIX dose showed similar cell viability (∼34%). However, at 7.91 μg mL−1 dose, phototoxicity increased 4 times with PLGA NPs. Notably, NPs exhibited lower dark cytotoxicity (∼90.6% viable cells) compared to free PpIX (∼49%). This suggests PLGA-NPs mitigate PpIX's dark cytotoxicity while maintaining phototoxicity, underscoring their potential as melanoma PDT delivery systems.59
In a study by Wang et al. topical PDT utilizing PLGA NPs loaded with 5-ALA demonstrated effectiveness against cutaneous SCC in mouse model. 5-ALA PLGA NPs exhibited superior efficacy compared to free ALA. 5-ALA PLGA NPs notably suppressed the tumor growth with reduced in volume by an average of 68%, with some smaller tumors completely eliminated after one or three sessions. These findings underscore the potential of this approach for SCC treatment.60 Polysaccharide-based NPs have also been investigated extensively for PDT in skin cancer. The polysaccharide-based NPs are often preferred for their excellent biocompatibility, ease of preparation, and minimal toxicity.61,62 A multi-responsive alginate nanogel was evaluated in vitro in B16F10 melanoma cell line. The formulation with light irradiation showed almost ∼4 times higher intensity than control in fluorescent microscopic evaluation of ROS using the dichlorodihydrofluorescein diacetate fluorogenic probe. Similar results were observed in cell viability assay where the formulation showed the least cell viability compared to doxorubicin and hydrazide-functionalized pheophorbide-a in the highest dose.63
Polymeric micelles are well suited for carrying poorly water-soluble drugs or bioactive in a targeted delivery approach. These can be defined as nanosized core/shell structures that are generally made up of amphiphilic block polymers.64 These copolymers have the potential to self-assemble into micelles in presence of water. Generally, several hundred block copolymers make one micelle. Each micelle consists of two regions, a dense hydrophobic core and a shell of poly(ethylene oxide).65–67 Polymeric micelles have numerous benefits over other drug nanocarriers such as large solubilization power, higher loading capacity, high stability, and more longevity. In an aqueous environment, micelles act as amphiphilic systems by excluding the hydrophobic core from the outside environment. Their core/shell structure provides a hydrophobic core for the encapsulation of lipophilic molecules while the brush-like hydrophilic outer shell creates a barrier between the outer environment and the hydrophobic cargo. The outer shell is especially significant because it stops biological invasion as well as reduces the adsorption of protein on the nanocarrier surface.68
Skidan et al. prepared PEG-diacyl lipid micelles encapsulating meso-5,10,15,20-tetraphenyl-21H,23H-porphine (TPP) and evaluated their therapeutic efficacy in PDT against B-16F10 melanoma cell lines. The micelles exhibited a remarkable 150-fold increase in TPP solubilization. Modification with cancer-specific monoclonal antibody 2C5 further enhanced targeting and phototoxic effects. TPP-micelles and immunomicelles exhibited 75% and 90% reduction in tumor load, respectively after 9 hours with 15 minutes of irradiation.69 In Lamch et al.'s study, zinc(II) phthalocyanine (ZnPc) was entrapped in folate-functionalized micelles and evaluated in metastatic melanoma (Me45) cell lines. Study results exhibited that designed micelles with 4 μM ZnPc concentration showed decreased cell viability to 70% after 24 hours. The study suggests polymeric micelle formulation as a stable, safe, and effective strategy for targeted tissue PDT in skin cancer therapeutics.70
3.2 Vesicular lipid nanocarriers
3.2.1 Liposomes. Liposomes are concentric phospholipid spheres with single (or multiple) bilayers made up of natural or synthetic lipids.71,72 The cholesterol present in the liposomal structure increases the bilayer rigidity, hindering the encapsulated PS from permeating out. Furthermore, after the liposomes reach systemic circulation, the lipid exchange between these nanocarriers and high-density lipids results in the disintegration of the liposomes which in turn causes a untimely release of PS in the circulation instead of the target tissue. Lastly, the opsonization of liposomes by plasma proteins results in quick removal from the bloodstream by the MPS.55,73 In several studies, these disadvantages were overcome by modifications in the liposome structure. PEGylation of liposomes is analogous to stealth NPs that effectively dodge various eliminating pathways in blood circulation and also protects from opsonization. A comparison study between Foslip® and polyethylene glycosylated (PEGylated) liposomes (Fospeg®) of temoporfin was conducted by Reshetov et al. in tumor-grafted mice. PEGylated liposomes exhibited stability in the circulation, and controlled release properties and combination of enhanced permeability and retention-based tumor accumulation, these resulted to a higher efficacy of the treatment with Fospeg® compared to Foslip®.74Some studies showed a combination of PTT and PDT with liposomes in cancer. Dai et al. prepared multifunctional theranostic liposomes loaded with a prodrug that got activated in hypoxic conditions. In PDT, the hypoxic microenvironment is a big disadvantage to overcome and several researchers have relied upon a combination of PTT and PDT. In Fig. 6 simultaneous activation of PS-enclosed liposomes at 808 nm (PTT) and 660 nm (PDT) can be seen with tirapazamine (TPZ) prodrug.75 Although this study was designed for lung cancer, a similar feat can be achieved for skin cancer also.
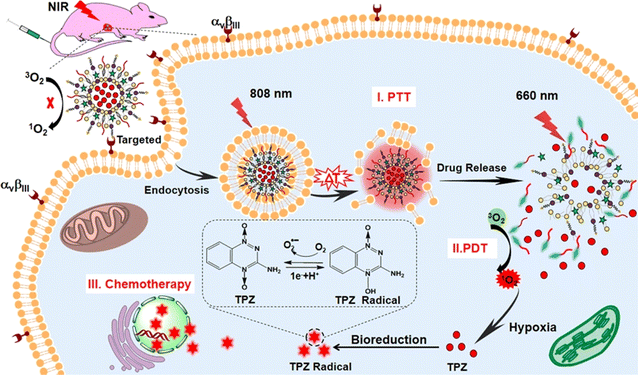 |
| Fig. 6 Combination therapy comprising PDT, PTT, and chemotherapy through liposomal carriers. Reprinted with the permission from cited ref. 75. Copyright© 2019, American Chemical Society. | |
The poor aqueous solubility of porphyrin causes hindrance to properly targeted delivery of the agents at the tumor site.76 In an in vitro assessment by Pierre et al. 5-ALA was utilized despite its low permeability through the stratum corneum. 5-ALA liposomes with lipid composition of mammalian stratum corneum were prepared and evaluated. The stratum corneum lipid liposomes were prepared by reverse-phase evaporation technique and with 5-ALA and lipids in a 1
:
3 ratio. The degree of encapsulation was reported to be 5.7 ± 0.17%. The average particle size was 500 nm which got reduced to 400 nm in presence of 5-ALA indicating a possible drug–vesicle interaction. Slower permeation and delivery of 5-ALA into the epidermis was shown to be 3 times more than the 5-ALA solution usually used in PDT even after 36 hours of administration. A similar lipid bilayer structure to the stratum corneum was reported to be the reason behind such good performance of stratum corneum lipid liposomes.77
3.2.2 Ethosomes. Ethosomes are lipid vesicles with fluidic nature that contains a comparatively greater amount of ethanol.78 These nanocarriers are being extensively studied for their potential application in transdermal drug delivery. In the year of 1996, Touitou et al. prepared Ethosomes for the first time with 20–45% of ethanol content. This high ethanol content makes the nanocarriers soft and pliable. Each NP can show a unilamellar or multilamellar structure with concentric bilayers of phospholipid that surround an aqueous phase. The main function of ethanol is to increase the flexibility of the nanocarrier membrane.79,80 Furthermore, ethanol is a penetration enhancer, and the percentage of ethanol directly affects the nanocarrier size, zeta potential, stability, entrapment efficiency, and permeability. However, the ethanol concentration in ethosomes is critical as a high concentration beyond the optimum level can result in a leaky bilayer, increased vesicular size, and poor entrapment efficiency. Classical ethosomes are composed of phospholipids, ethanol, and water while binary ethosomes additionally contain an extra type of alcohol, mostly isopropyl alcohol, and propylene glycol. Transferosomes are also ethosomal compounds that are modified by inducing edge activators or penetration enhancers.78,81 Due to its several advantages over conventional nanocarriers like liposomes, ethosomes are being explored as PS carrying vehicles in PDT for skin cancer treatment.In an investigation, Nasr et al. employed chlorin e6 (Ce6) encapsulated within ultradeformable ethosomes for the PDT of SCC. Encapsulation of Ce6 into ethosomes did not significantly impact singlet oxygen generation upon laser exposure. PDT induced dose-dependent cytotoxicity in SCC cells, marked by elevated mitochondrial superoxide levels and caspase 3/7 activity. Ce6 ethosomes demonstrated effective penetration into SCC spheroids, leading to diminished size, proliferation, and viability post-irradiation. Notably, Ce6 ethosomes exhibited heightened cytotoxicity against SCC spheroids relative to normal skin fibroblast spheroids. Additionally, PDT treatment of SCC xenografts elicited reductions in tumor size, angiogenesis, and cellular proliferation, alongside augmented apoptosis. These findings underscore the potential of Ce6-loaded ethosomes as a promising modality for targeted PDT in SCC therapy, demonstrating efficacy across both in vitro and in vivo models.82 Similar in vitro study was conducted by Curic and colleagues where they investigated the efficacy of temoporfin (mTHPC)-loaded invasomes (modified ethosomes) for topical PDT of skin cancers using the A431 cell line, an epidermoid tumor model. mTHPC, being highly hydrophobic, poses challenges for skin delivery due to low absorption. The formulated mTHPC-loaded invasomes, containing terpenes and ethanol, demonstrated enhanced skin penetration. In vitro studies on A431 cells revealed low dark toxicity and significant phototoxicity of mTHPC-invasomes at a concentration of 2 μM after 24 hours of incubation. The phototoxic effect was more pronounced with invasomes containing 1% penetration enhancer or 1% citral compared to mTHPC-ethanolic solution. These results suggest the potential of mTHPC-loaded invasomes for topical PDT of cutaneous malignancies, as evidenced by the substantial reduction in A431 cell viability post-treatment. The study highlights the promise of mTHPC-invasomes as an effective and minimally invasive therapeutic approach for skin cancer treatment, offering advantages such as enhanced drug delivery and reduced residual photosensitivity confined to the treatment site.83
In study by Nasr et al., ethosomes with a PS, ferrous chlorophyllin (Fe-CHL) were characterized, evaluated for skin retention and penetration, and compared to two other formulations, uncoated Fe-CHL loaded chitosan nanocarriers and lipid-coated Fe-CHL loaded chitosan nanocarriers (PC/CHI) across mouse skin (ex vivo). Ethosomal preparation showed the highest entrapment efficiency at 78.2 ± 1.35%. PC/CHI at 0.01 mM showed a 52.4% decrease in cell viability. In brief, both the Fe-CHL ethosomes and PC/CHI showed better results in every aspect compared to Fe-CHL solution alone. Although, with a comparatively greater particle size ethosomes showed deeper skin penetration than PC/CHI. Both formulations have the potential to be used as nano-vehicles for PS in PDT for skin cancer treatment. However, ethosomes performed slightly better in skin penetration, skin retention as well as cell viability.84
A recent study compared the overall performance of 5-ALA-loaded ethosomes with liposomes for PDT and experimented on enhancing the skin production of PpIX. Three ethosomal formulations were considered with varying phosphatidylethanolamine (PE), cholesterol (CH), and sodium stearate (SS) levels. A 7 folds increase in entrapment efficiency was observed after the incorporation of SS into the ethosome composition, PE/CH/SS (2
:
1
:
2.5) compared to PE and PE/CH (2
:
1) ethosomes. However, the incorporation of SS, an anionic surfactant increased the vesicular size by 35.32% and 45.8% in liposomal and ethosomal systems respectively over storage for 32 days at 4 °C. In the confocal laser scanning microscopy study, contrasting trends in penetration behavior were observed in liposomes and ethosomes. The PpIX intensity showed in liposomal formulations was highest in PE/CH/SS and lowest in H2O. However, PE/CH/SS ethosomal ALA delivery indicated a reduced PpIX content in the skin. In brief, with regards to PpIX deposition in the skin, the penetrability of ethosomes was found to be superior than that of the liposomes. It was also concluded that due to the fluidizing effect of ethosomal lipids as well as the stratum corneum lipid bilayer, ethanol was the one responsible for the higher penetrative behavior of the ethosomes.78,85
3.2.3 Niosomes. Niosome is a novel drug delivery approach that is extensively being used for attaining sustained or controlled-release drug profiles. These vesicular NPs are used in targeted drug delivery also. Structurally, niosomes can be of three types: unilamellar, oligolamellar, and multilamellar. Niosomes were developed in the first place to overcome disadvantages like toxicity and stability issues shown by liposomes.86 Structurally, liposomes and niosomes are similar, the only difference being the double layer of the respective nanoparticle. While liposomes contain a phospholipid bilayer, niosomes are made up of a non-ionic surfactant layer87 that also contributes to its superior stability, biocompatibility, low toxicity, and ease of handling and storage without requiring special conditions.86,88 A few most commonly used surfactants are derivatives of alkyl esters, sorbitan fatty acid esters, and alkyl ethers.89 Just like liposomes niosomes can function as local depots for sustained release of dermally active compounds, penetration enhancers, PS, or as rate-limiting membrane barriers to modulate the systemic absorption of drugs.90 These non-ionic surfactant-based vesicles improve drug stability, biocompatibility, and skin penetration, ensuring higher concentrations of therapeutic agents reach target tissues.91 Recent advancements include stimuli-responsive niosomes that release drugs in response to pH, temperature, or light, allowing for controlled and targeted delivery.92 Moreover, surface modifications with targeting ligands enhance specificity towards cancer cells, improving uptake and minimizing side effects. The incorporation of PS into niosomes began when a group of scientists comprehended the advantages niosomes offer over targeted PS delivery through liposomes in PDT. Other than lesser toxicity and greater stability as mentioned earlier, niosome is a better alternative because it also offers an easier and cost effective production process, longer storage shelf-life, and an extensive formulation adaptability.93 Here, Fig. 7 represents the application of niosomes used in PDT.94
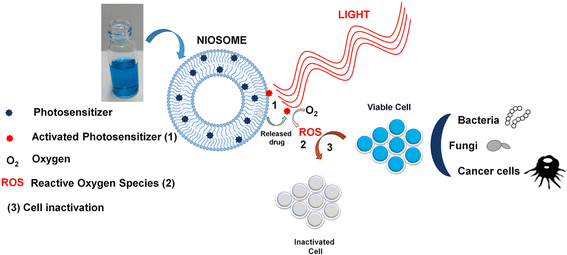 |
| Fig. 7 PS-loaded niosomes used in PDT for effective antitumor and antimicrobial effects. Reproduced with the permission from cited ref. 94 Copyright© 2022, Elsevier. | |
In a study by Bragagni M. et al. various dispersions of niosomes were formulated following two separate methods with the aim to develop an optimum niosomal formulation that can deliver a better skin permeation and penetration of 5-ALA in skin cancer treatment when used in association with PDT. The thin-layer evaporation method and reverse-phase evaporation method were followed in the study while preparing the niosomal dispersions. %EE values were found to be close in 1
:
1 sorbitan monostearate (Span 60) and cholesterol (CHL) niosomes prepared by thin-layer and reverse-phase methods, ∼75% and ∼81% respectively. An elastic niosomal dispersion with 1
:
1
:
0.28 Span 60, CHL, and dicetyl phosphate (DCP) showed ∼40% EE. An ex vivo study was carried out using excised human skin. In comparison to the aqueous 5-ALA solution currently used in clinical practice, both classical niosomes and elastic niosomal formulations showed strikingly better results. An 80% and 40% increase in drug permeation was observed for classical and elastic niosomes respectively. Similarly, 100% and 50% increases were observed in 5-ALA deep skin retention in classical niosomes and elastic niosomes respectively, compared to simple 5-ALA solution.93 This study proves the effectiveness of NPs especially niosomes to enhance PS permeation and penetration in deep skin layers.
3.3 Lipid nanocarriers
Solid lipid NPs (SLNs) were first developed as a substitute to emulsions, polymeric NPs, and liposomes. The liquid oil phase of an o/w emulsion was replaced with a solid lipid or a mixture of solid lipids. This enabled the SLNs to be stable in the solid phase at both room temperature as well as body temperature.95 The drug of choice is always incorporated or attached to the solid lipid core. As the SLNs retain their solid structure even after administration, a controlled release profile for the loaded drug was achieved.96 Among all the lipid nanocarriers available, SLNs show high drug-loading capacity and a better-controlled release profile. In SLNs preparation, lipids that are used are also generally inexpensive than the lipids used in liposomal preparation.97 Nanostructured Lipid Carriers (NLCs) are considered the second generation of lipid NPs. In NLCs, the lipid matrix contains a mixture of solid and liquid lipids (oils) instead of only solid lipids like SLNs. Although the presence of oils reduces the melting point of the solid lipid, it still remains solid at room as well as at body temperatures. The presence of oils is advantageous because it prevents recrystallization of solid lipids over storage which in turn allows more loading volume for drugs compared to SLNs. Furthermore, the presence of oil also produces a more thermodynamically stable environment.98,99 NLCs can also be stabilized in an aqueous solution with the help of single or multiple surfactants. A thermodynamically stable system is also less likely to prematurely release the payload. Consequently, NLCs offer a better release profile. The average particle size for both SLNs and NLCs ranges between 40 to 1000 nm. The size depends on the composition of the lipid matrix and the method of preparation.96,100
In a study by Goto et al. aluminum chloride phthalocyanine-loaded SLNs were used for the photodynamic deactivation of melanoma cells. Although aluminium chloride phthalocyanine (ClAlPc) was a popular PS used in PDT, its highly hydrophobic nature limits its application. A direct emulsion method was employed to prepare the ClAlPc-loaded SLNs. B16-F10 melanoma cells were taken for the study. The same concentration of ClAlPc-400-SLN (containing 400 μg mL−1 of ClAlPc) or free ClAlPc (ClAlPc-Et) was introduced to the cells before incubation. Cell viability was evaluated for both formulations with varying light doses. At the lowest light dose, cell viability decreased to ∼54.1% and ∼64.4% for ClAlPc/SLN and ClAlPc-Et. With doubled light dose, the SLNs showed a reduction of 50% in cell viability while free PS exhibited nothing significant. Further increasing the light intensity ∼15.1% cell viability was achieved with SLNs while 48.9% was observed in free PS. On average, SLNs showed a 3.2 times more decrease in cell viability than free ClAlPc.101
Qidwai et al. prepared NLCs with a second-generation PS, with 5-ALA inside it. As mentioned earlier, the low permeability of 5-ALA creates a severe challenge in the topical delivery of the compound. Additionally, its hydrophilicity and charge characteristics are also not ideal for lipid-based delivery. For the preparation of NLCs, microemulsion technique was used. For liquid lipids, castor oil, soybean oil, peanut oil, ethyl oleate, and oleic acid were considered and evaluated. 5-ALA showed satisfactory solubility in oleic acid and a 70
:
30 ratio of Compritol ATO 888 (solid lipid) and oleic acid was chosen for NLC preparation. The NLC formulation exhibited 4 times greater cytotoxicity than the normal drug solution which indicates increased cellular uptake in the case of NLC.102
In a study by Almedia et al. in 2018, SLNs and NLCs of chloroaluminum phthalocyanine (ClAlPc) were evaluated in melanoma BF16-F10 cell lines. Stearic acid was used as solid lipid while oleic acid was utilised as liquid lipid in NLCs. Two different NLC formulations, namely NLC20 and NLC40 were prepared with 20% and 40% oleic acid concentrations respectively. Permeation studies show zero permeation for free ClAlPc even after 24 hours of administration. The amount of drug retained in the deep skin layer was more in NLC40 than NLC20 and SLN-ClAlPc. 73% of the penetrated drug was retained in stratum corneum in the control formulation while only 10.5% was retained in the case of NLC40. This implies that 89.5% of the drug showed deep skin penetration in the case of NLC40. NLC40 also showed a rapid decrease in cell viability to 36% and 0.93% at 0.1 μg mL−1 and 0.2 μg mL−1 respectively.103 All the above-mentioned investigations were carried out in vitro. The lack of detailed in vivo studies in nanocarrier-mediated PDT in skin cancer may have restricted the potential of the lipid-based nanocarrier strategies in skin cancer treatment.
3.4 Dendrimers
A dendrimer is a highly branched macromolecule with repetitive units joined on a central multivalent molecule. There are two positions where the PS can be incorporated, on the periphery of the branches or in the multivalent core.104 The stepwise synthetic preparation of dendrimers makes their architecture extremely ordered and well-defined.55,105 The empty internal cavities and open conformation of lower-generation dendrimers facilitate the encapsulation of hydrophobic molecules. Additionally, the number of surface functional groups is much higher in dendrimers compared to other NPs.106 With the increase in generation, the number of branches also increases. The presence of surface groups is utilized to target different molecules while delivering PS. The size and lipophilicity can also be tailored to optimize biodistribution and cellular uptake.55,107 Both hydrophilic, as well as hydrophobic PS, can be encapsulated in dendrimers. The small particle size of these NPs (1 to 100 nm) also makes them comparatively resistant to reticuloendothelial system. A combinatorial therapy with dendrimer based delivery systems provide numerous benefits, including reduced drug concentrations, lower multidrug resistance, enhanced specificity with selective targeting capabilities, and ultimately, increased therapeutic efficacy.108 Due to these reasons drug delivery using dendrimers is being extensively explored in the scientific community specifically focusing on the targeted and sustained release profiles.109
Rodriguez and colleagues delved into the utilization of ALA dendrimers in PDT for cancer. Specifically, ALA dendrimers containing 6 and 9 ALA residues demonstrate heightened porphyrin production in cancer cells and a notable preference for macrophages over endothelial cells.110 In a similar study, Karthikeyan et al. addressed the limitations of Rose Bengal (RB) as a PS molecule. This study assesses polyamidoamine (PAMAM) dendrimers' potential in delivering RB and enhancing its phototoxicity on cancer cells while overcoming the issues related to toxicity and poor lipophilicity. Dendrimers efficiently encapsulate RB, exhibit characteristic spectral responses, and enable controlled drug release. ROS generation occurs upon white light exposure, leading to significant photocytotoxicity in cancer cells. Crucially, dendrimer delivery mitigates RB's dark toxicity, showcasing promise for improved PDT efficacy.111 Sztandera et al. investigated phosphorous dendrimers as potential carriers for cancer PDT. Three dendrimer generations were prepared using RB as the photosensitizer, tyramine as a linker, and P(S)Cl2 as terminal groups. Pyrrolidine was grafted onto the outer dendrimer shell. Spectrofluorometric analysis showed a 2-fold reduction in fluorescence intensity due to RB modification with tyramine. 3rd generation-3RB-pyrrolidine dendrimer exhibited optimal size and zeta potential for further evaluation. RB release was consistent at pH 5 and 7.4. MTT assays on AsZ, BsZ, and CsZ murine basal cell carcinoma cell lines revealed decreased phototoxicity with increased RB modification. Free RB exhibited the highest toxicity, while G3-3RB-pyrro showed minimal toxicity without irradiation. Tyramine modification decreased cellular uptake by approximately 50% in all cell lines. The study suggests exploring other linker compounds for dendrimer modification to enhance PDT efficacy.112
In another experiment, Sztandera et al. loaded the photosensitizer RB into 2nd and 3rd generation amphiphilic triazine–carbosilane dendrons and evaluated in basal cell skin carcinoma cell lines. Singlet oxygen levels in cells were 3-fold and 10-fold higher in 2nd generation-RB and 3rd generation-RB, respectively, compared to free RB. Cellular uptake of RB from both 2nd generation-RB and 3rd generation-RB reached 80% within 30 minutes, while free RB showed around 10% uptake in the first hour. Cytotoxicity evaluation demonstrated approximately 25% reduction in cell viability with free RB at 2 μM concentration, while 2nd generation-RB and 3rd generation-RB exhibited approximately 63% and 88% reduction, respectively. Overall, the study underscores the advantages of dendrimeric PS delivery in PDT for skin cancer treatment.113
3.5 Metallic nanoparticles
Metallic NPs have been used in several studies over the past few decades. Other than a few therapeutic and toxicological limitations, barrier effect of the cell membrane, occurrence of drug resistance, and drug disposition, metallic NPs provide a wide array of advantages in drug delivery, especially targeted drug delivery. However metallic NPs having high extinction coefficient, more stability, and low enzymatic degradation, have been used extensively in PDT.114,115 Here, gold and magnetic nanocarriers are mentioned in Table 1 that have been used in PDT for skin cancer specifically.
Table 1 Summary of recent investigations of metallic nanoparticles used in PDT for the treatment of skin carcinoma
Type of nanoparticle |
PS used |
Cell type |
Outcome |
Gold nanorods |
Ce6 |
Squamous carcinoma (SCC7) tumor cells |
Dual function nanosystem was obtained by Ce6 and GNR loading into a chitosan-embedded pluronic nanogel. No quenching was observed between PS and GNR |
EE ∼67% for Ce6. 43% Ce6 release in 3 days was observed116 |
Titanium-dioxide-nanoparticle–gold-nanocluster–graphene (TAG) |
Titanium-dioxide (TiO2) |
B16F1 melanoma cells |
The loading percentage of AuNcs and graphene in TAG composite was 9.5% and 9.2% by weight respectively. TAG composites were found to be separating electron hole pairs leading to the generation of oxygen radicals by utilizing sunlight. In vivo tumor inhibition study showed 75.89% inhibition in TAG-treated mice117 |
Gold nanorods (Au NRs) |
None |
B16F0 melanoma cells |
Upon light irradiation, Au NRs were observed to be acting as PS. No additional organic PS was present. Complete destruction of B16F0 tumor (in mice) was observed at optimized concentration118 |
Gold nanoparticles |
5-ALA |
Human neonatal dermal fibroblasts (NHDF; passage 1) |
5-ALA was conjugated on GNPs and the biocompatibility of the system facilitated their preferential capture by the diseased fibrosarcoma cells. A considerable quantity of PpIX got accumulated with an increase in ROS formation119 |
Gold nanorods (GNRs) |
Rose Bengal (RB) |
Oral squamous carcinoma cells |
Multifunctional RB-GNRs show satisfying ROS generation (confirmed by fluorescence study) after irradiation. In comparison with available oral therapies for cancer, PDT-PTT dual therapy using RB-GNRs showed better targetability and therapeutic effects120 |
Magnetic nanoemulsion (MNE) |
Foscan® |
— |
A rise in diffusional flux was observed for Foscan® when incorporated in MNE which led to improved skin penetration and satisfying drug accumulation in the tumor cells121 |
Photosensitizer-conjugated magnetic nanoparticles (PS-MNPs |
Polyaminated chlorin p6 |
B16F10 and B16G4F melanoma cell line (with or without melanin respectively) |
Two water-soluble PS-conjugated magnetic nanoparticle formulations were reported. A magnetic core of iron oxide with a dextran shell coating incorporated with polyaminated chlorin p6 was the structural design of the nanocarriers. Cell viability was evaluated through relative dehydrogenase activity that showed promising results122 |
Magnetic nanoemulsion |
Zn(II) phtalocyanine (ZnPc) |
— |
Pig skin was used for ex vivo study. Entrapment of ZnPc showed satisfying results (especially the deep skin penetration data) which points out the potential of applying PDT and HPT in conjugation123 |
3.6 Cubosomes
Cubosomes are self-aggregated liquid crystalline particles that are composed of specific amphiphilic lipids majorly mono-olein or phytantriol in a certain ratio to water.124,125 These nanostructured particles consist of three-dimensionally arranged curved lipid bilayers, organized as honeycombs. The unique structure comprises two internal aqueous channels that are exploited for carrying payloads like drugs and PS.125 Cubosomes provide the native symmetry of the cargo in their nanosized shapes due to the high solid-like viscosity it inherently acquires from its bicontinuous structure. This bicontinuous structure also facilitates the solubilization of a wide range of molecules from high molecular weight proteins to low molecular weight drugs. The tortuosity of the bicontinuous layer provides diffusion-controlled release of the molecular cargos.126,127 The recent increase in the popularity of cubosomes as molecular carriers is mainly due to their high skin permeability attribute. The primary excipients of a cubosome like glycerylmonooleate and poloxamer act as permeation enhancers. Additionally, the overall structure of the cubosomes resembles to the structure of the skin that enables it to enter through the apertures of the stratum corneum ultimately resulting in deep skin penetration.128 Bicontinuous cubic phase formation of a cubosome depends on the self-assembling nature of the lipid mixture with a stabilizer and the molecule to be encapsulated.129 The most common application of cubosome is as a controlled release vehicle. Although patent art already exists on cubosomes, further exploration is still very much needed.128,130
Bazylińska U et al. designed a type of polymer-free cubosomes using a monoolein molecular building block. They used stabilizers like phospholipids and propylene glycol (PG) as a hydrotrope as well as a humectant. After analyzing the backscattering profile to identify the most stable formulations, they used those as potential PS carriers for PDT in human melanoma cells. The assembly strategy used for PS-loaded cubosomes is depicted in Fig. 8. Following the same technique, five different cubosome formulations were prepared without any PS loaded in those, and the best formulation with 0.75 wt% PG (more negative zeta potential, smallest average size, and lowest PDI) was chosen for PS loading further in the study. Two hydrophobic PS TPP-Mn and Ce6 were loaded in the optimum cubosome formulation with an encapsulation efficiency of 91% and 97% respectively. Photodynamic activity and cytocompatibility were evaluated in Me45 and MeWo melanoma cell lines. In a dark cytotoxicity study, Me45 cells showed more than 50% viability in both the PS at 1 and 2 μM. MeWo showed the best biocompatibility with Ce6 at a nontoxic concentration of 5 μM. Excellent photodynamic properties were observed when Ce6 at 1 μM concentration in Me45 cells was applied through cubosomes. It exhibited low toxicity in the dark as well as a 90% reduction in cell viability after irradiation. Similar results were observed with MeWo cells. In conclusion, PG content was found to be directly affecting the physicochemical properties of the cubosomes. Ce6-loaded cubosomes showed satisfying biocompatibility, low toxicity, and excellent photodynamic activity in both melanoma cell lines.131
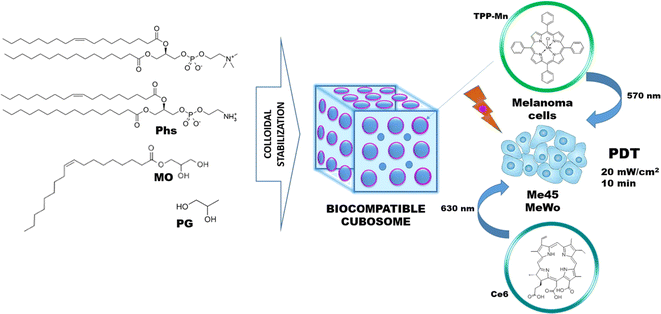 |
| Fig. 8 Strategy applied for the assembly of Ce6, and TPP-Mn loaded cubosomes for PDT. Reproduced with the permission from cited ref. 131 Copyright© 2018, Elsevier. | |
3.7 Quantum dots
Semiconductor nanocrystals or quantum dots (QDs) are becoming one of the newest members of the nanocarrier class that is being explored extensively by the scientific community. QDs offer significant potential for both photodynamic and photothermal cancer therapies. Their remarkable properties, including superior photoluminescence, efficient photothermal conversion, and versatile surface functionalization, position them as ideal candidates for targeted treatment approaches. In PDT, QD-based nanosystems serve as excellent photosensitizers, capable of absorbing light across a wide spectrum, and their nanoscale dimensions enable deeper tissue penetration, thereby enhancing therapeutic outcomes. Moreover, their ability to transform light energy into heat makes them highly effective for PTT. The combination of PTT and PDT using QDs presents vast opportunities for advancing cancer treatment.132,133 Additionally, QDs provide unique optical characteristics such as, minimal photobleaching, low degradation, high quantum yield which make them suitable for bio-imaging and as labeling probes. In contrast with organic dyes, QDs provide several advantages like size-tunable light emission, and better signal brightness. In several studies, QDs have been designed to carry specific classes of therapeutic agents.134,135 Considering QDs potential to have inherent phototoxic properties, it is only natural to use these nanocarriers in PDT with136 or without137,138 additional PS molecules entrapped inside it.139
Ahirwar S et al. experimented with graphene quantum dots (GQD) and graphene oxide quantum dots (GOQD) and explored their potential as PS (due to excellent photoluminescence properties) in PDT on B16F10 melanoma cell line. This study focuses on a novel approach by utilizing the inherent phototoxic characteristics of GQD and GOQD to avoid lesser release efficiency observed in GO–PEG–Ce6 and GO–Pluronic–MB NPs. Both the GQD and GOQD were synthesized by exfoliating graphene rods electrochemically. The average size was observed as 1.5–5.5 nm. Singlet oxygen generation, low toxicity, and easy cellular uptake were observed. With varying excipients, three quantum dots were prepared namely, GQD1, GQD2, and GQD3. All three QD showed close to 100% cell viability after 24 hours without any irradiation. GQD1 exhibited an over 88% reduction in cell viability with only 2 minutes of UV exposure. The same was observed in GQD3 (80% reduction). GQD2 took 5 minutes to reduce the cell viability of B16F10 cells by 93%. The study concluded GQD/GOQD as a potential photosensitizing candidate for PDT in skin cancer treatment.136 Although a few drawbacks like lower singlet oxygen generation efficiency,138 and lower tumor cell accumulation137 was observed with quantum dot-PS conjugates, it was postulated that changing the PS or introducing surface groups can overcome those disadvantages. QD as a PS carrier system shows enormous potential that needs to be explored further.
3.8 Carbon nanotubes
After drawing influence from the fullerenes, a group of scientists created the first nanocarrier system which had a close-ended long structure, and the walls were made up of hexagonal carbon units. This nanocarrier is what we now call carbon nanotubes (CNTs).140 CNTs are of two types depending on the number of layers they possess: single-walled CNTs and multi-walled CNTs. In general, CNT shows many properties that demand its application as a drug delivery carrier. Along with drug delivery, CNTs are also utilized in environmental protection, pathogen detection, and the diagnosis and treatment of diseases. These applications enhance the pharmacological profiles of numerous therapeutic molecules and greatly benefit tissue bioengineering practices.141 Specifically, the cylindrical shape of the nanoparticle facilitates transmembrane penetration and the large aspect ratio of the CNTs offers a greater drug-loading capacity. This also facilitates chemical functionalization with a wide array of chemical moieties. Moreover, the extraordinary photothermal feature showcased by CNTs is that they tend to act as electrical conductors. Additionally, CNTs are capable of absorbing optical intensity and photoluminescence.142 These characteristics have established the ground for using CNTs as PS carriers in PDT for cancer treatment. Fig. 9 represents a schematic diagram of nanocarriers such as liposomes incorporated into CNTs to target specific cells in vivo.143
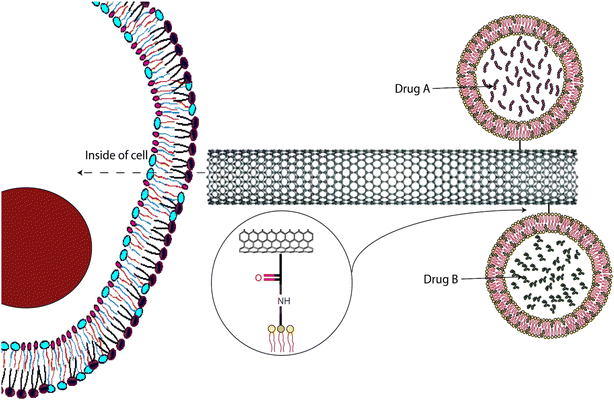 |
| Fig. 9 Application of CNT in carrying other nanocarriers for specific cell targeting is presented through a schematic diagram. Reproduced with the permission from cited ref. 143 Copyright© 2012, Elsevier. | |
The investigation delves into the PDT potential of zinc monoamino phthalocyanine linked to folic acid (ZnMAPc–FA), both in isolation and when bound to single-walled carbon nanotubes (SWCNTs), in melanoma A375 cells. ZnMAPc–FA and ZnMAPc–FA–SWCNT exhibited considerable PDT efficacy, inducing 60% and 63% cell death, respectively, under laser irradiation at 676 nm and 5 J cm−2. In contrast, SWCNT–FA, devoid of ZnMAPc, displayed minimal PDT impact, with only 23% cell mortality observed. Cytotoxicity assessments verified the non-toxic attributes of all compounds at various concentrations. The incorporation of SWCNTs in ZnMAPc–FA–SWCNT likely facilitated more effective drug delivery, enhancing PDT effectiveness. Despite ZnMAPc–FA–SWCNT's lower singlet oxygen quantum yield compared to ZnMAPc–FA, PDT efficacy was markedly improved, suggesting enhanced drug delivery efficiency. Subsequent post-treatment proliferation assays corroborated the absence of cell growth, affirming treatment efficacy.144 In a recent in vitro study by Jafeer and Mahdi, similar findings were reported. The objective of the in vitro investigation was to evaluate the influence of blue light, single-walled carbon nanotube –OH (SWCNT–OH), and their combined effect on the viability of A431 skin cancer cells following a 24 hour incubation period. A431 cells were subjected to direct irradiation with blue light, exposed to SWCNT–OH nanoparticles, or treated with a combination of both, followed by a 24 hour incubation. Cell viability was assessed using a crystal violet assay, revealing a significant decrease in viability across all treatments. Blue light exposure for 240 seconds resulted in notable viability reduction, while the most pronounced effect was observed with SWCNT–OH at a concentration of 200 μg mL−1. Combined treatment further reduced viability, suggesting potential synergistic effects.145
4 Microneedles as a transdermal delivery approach
Topical creams and hypodermic needles are very common strategies followed when drug delivery through the skin has to be obtained. Compliance is less with needles because of the pain during and after administration and bioavailability is often not satisfying with topical creams.146 A typical microneedle (MN) is 150–1500 μm in height, 50–250 μm in width (base), and has a tip diameter of 1–25 μm. This micron-sized needle penetrates the stratum corneum without engaging any nerve or damaging any blood vessel. Better drug bioavailability, higher safety, and minimal invasiveness are the primary reasons for the attention MNs are getting as potential topical and transdermal delivery systems.147 MN patches comprise of hundreds of micron-sized needles. As mentioned earlier, MN patches are beneficial mostly for drugs with low bioavailability, low lipophilicity, and thus poor skin penetration characteristics.148 PS can be delivered into deep skin by using various types of MNs available. Solid MNs follow the “poke and patch” approach. These metallic MNs are used in pre-treatment to create micropores on the skin. Coated MNs follow the “coat and poke” approach. Dissolving MNs follow the “poke and release” approach while hollow MNs follow the “poke and flow” approach. Additionally, biodegradable MNs degrade in the skin after drug release.147 Here, MNs were discussed as potential carriers of PS in PDT for the treatment of skin cancer. In Fig. 10 a schematic representation of the self-degradable MN-assisted platform used in PDT in combination with immunotherapy is presented.149
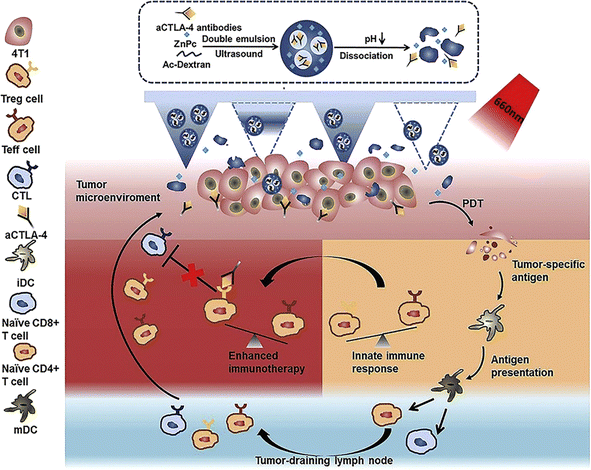 |
| Fig. 10 Schematic representation of the self-degradable MN-assisted platform used in PDT in combination with immunotherapy. The MN-assisted approach was to achieve controlled co-delivery of PS and anti-CTLA4 antibody. The hyaluronic acid MNs were loaded with pH-sensitive dextran nanoparticles which were designed to encapsulate the hydrophobic PS molecules (ZnPc) as well as the hydrophilic anti-CTLA4 antibodies. Reproduced with the permission from cited ref. 149 Copyright© 2020, Elsevier. | |
In Tham et al.'s investigation, a mesoporous silica nanocarrier loaded with the photosensitizer phthalocyanine (Pc) was combined with MN patches to enhance skin penetration. MNs created microchannels in the stratum corneum, allowing the topical application of drug-loaded NPs. Following penetration into deeper skin layers through these microchannels, PDT was performed. Dabrafenib and trametinib were utilized in combination. Three groups were studied: PDT alone, targeted therapy, and combination therapy. While all groups exhibited reduced cell viability with increasing PS and drug concentrations, PDT alone reached its efficacy threshold at lower concentrations. Combination therapy with MNs demonstrated the most significant reduction in cell viability on the A375 human melanoma cell line, followed by targeted therapy and PDT alone.150 MNs appear to be a perfect PS-carrier for their accurate on-site delivery, prolonged release (despite the shorter half-lives of most PS), and lesser chance of photosensitivity due to abnormal accumulation of PS on skin. More research should be focused on this physical technique to improve drug delivery into the skin and in vivo studies should be conducted thoroughly.
5 Clinical trials and patents
The application of NPs for PDT of skin carcinoma is a comparatively novel strategy, most of the research is being done on a laboratory scale for now. The positive results exhibited in the case studies above need furthermore vigorous research to ensure reproducibility. Preclinical and clinical trials for the formulation that shows promising results can only be done with enough funding and interest from different companies or institutes or organizations. Table 2 contains details about various clinical trials that are correlated with PDT, NPs, and skin cancer.
Table 2 Clinical trials associated with PDT and skin cancer
S. no. |
Study title |
Interventions |
Status |
Phase |
1 |
Metvix PDT versus cryotherapy in patients with primary superficial basal cell carcinoma151 |
Procedure: cryotherapy or PDT with methyl aminolevulinate cream |
Completed |
Phase 3 |
2 |
Photodynamic therapy and microvesicles152 (microvesicles) |
Device: PDT |
Recruiting |
Early phase 1 |
Drug: 4% imipramine |
Drug: base cream |
3 |
Metvix PDT in patients with “high risk” basal cell carcinoma153 |
Procedure: PDT with metvix 160 mg g−1 cream |
Completed |
Phase 3 |
4 |
Fractional CO2 laser assisted photodynamic therapy154 |
Drug: conventional PDT |
Completed |
Phase 2 |
Procedure: fractional CO2 laser-assisted PDT |
Phase 3 |
5 |
Safety study of photodynamic therapy using photocyanine injection in treating patients with malignant tumors155 |
Procedure: PDT |
Unknown |
Phase 1 |
6 |
Photodynamic therapy with HPPH compared to standard of care surgery in treating patients with oral cavity cancer156 |
Drug: HPPH |
Terminated |
Phase 2 |
Procedure: PDT, therapeutic conventional surgery |
7 |
Photodynamic therapy using silicon phthalocyanine 4 in treating patients with actinic keratosis, Bowen's disease, skin cancer, or stage I or stage II mycosis fungoides157 (microvesicles) |
Drug: Silicon phthalocyanine 4 |
Completed |
Phase 1 |
8 |
Randomized comparison of low and conventional irradiance PDT for skin cancer158 |
Device: ambulight (ambicare health) |
Completed (has results) |
NA |
9 |
Interstitial photodynamic therapy in treating patients with recurrent head and neck cancer159 |
Procedure: PDT |
Terminated (has results) |
Phase 2 |
Drug: porfimer sodium |
10 |
Alteration of the immune microenvironment in basal cell carcinoma following photodynamic therapy160 |
Drug: ALA |
Recruiting |
Phase 2 |
Procedure: PDT |
11 |
Interstitial photodynamic therapy (PDT) with temoporfin for advanced head and neck cancers161 |
Drug: temoporfin |
Terminated (has results) |
Phase 2 |
Device: medical diode laser emitting light at a wavelength of 652 nm. (ceralas PDT 652, CeramOptec GmbH) |
12 |
Use of jet-injection in photodynamic therapy for basal cell carcinoma162 (jet-injection) |
Drug: jet injection of ALA |
Recruiting |
Phase 2 |
Procedure: surgical excision, illumination, incubation |
There are a considerable number of patents available regarding using various NPs in association with PDT for the treatment of cancer and other diseases. Globally, various novel techniques have been patented in the same field. Patents for treating skin cancer through PDT with the help of different NPs are mentioned in Table 3.
Table 3 Patents related to the application of nanoparticles in PDT
S. no. |
Patent no. |
Year |
Nanoparticle used with PDT |
1 |
EP3157337B1 |
2019 |
Strontium aluminium oxide nanoparticle embedded with a rare earth element (Eu, Dy, or Nd) as transducers. Two types of NPs were used having the formula SraAlbOc:RaE and SraAlbOc:(RaE)2. These NPs were used for PDT based cancer therapy, where the core of NPs is enclosed by mesoporous substance with dispersed PS163 |
2 |
US9956426B2 |
2018 |
A nanoparticle comprising a rare earth metal fluoride composition (M1M2F4), where M1 is sodium and M2 is rare earth (ytterbium, erbium, yttrium), and PS. These particles can be used to target deep tumor tissues164 |
3 |
RU2405600C2 |
2011 |
Magnetic microcontainers with photodynamic or photothermal dyes that also contains magnetite NPs (Fe2O4) in the shell. These polymeric microcontainers ensure efficient accumulation in tumor tissue and tumor cell destruction, while ensuring minimal damage to the normal cells165 |
4 |
KR101035269B1 |
2011 |
Novel PS based on polymer derivatives-PS conjugates for PDT (polymer-chitosan, glycol chitosan, poly-L-lysine, or poly(ethyleneglycol), photosensitiser- porphyrins, chlorins, bacteriochlorins, or porphycenes). The NPs are claimed to have preferential accumulation in carcinoma tissue and generate ROS on irradiation166 |
5 |
CN109432422B |
2021 |
Black phosphorus quantum dot/platinum hybrid mesoporous silica NPs with improved stability and catalytic reaction of platinum NPs and hydrogen peroxide in tumor cells lead to oxygen generation enhancing PDT effect167 |
6 |
EP2741775B1 |
2017 |
A biodegradable polymeric nanoparticle made of a either PGA, PLA, or PLGA in combination with hydrogen peroxide and two photodynamic agents (SL052 and SL017), to provide singlet oxygen species for anti-cell proliferation activity168 |
7 |
US10420346B2 |
2019 |
Nanostructure with a silver nanoparticle core, a mesoporous silica shell, and a PS to form a blend. It is developed to kill fungi169 |
8 |
EP2198885B1 |
2012 |
A nanoparticle containing a crystalline or amorphous calcium phosphate biodegradable ceramic core, PS, and a stabilizing agent with an improved therapeutic efficacy and storage stability for topical or intravenous administration170 |
9 |
US20210268129A1 |
2021 |
A nanoparticle with a nanoscintillator that can emit light upon radiation-exposure, a PS that can absorb the light emitted by the nanoscintillator to generate singlet oxygen species and an additional therapeutic agent171 |
10 |
US20100262115A1 |
2010 |
A nanoparticle with polyvinylpyrrolidone encapsulated hypocrellin-B derivative. These core/shell NPs showed cytotoxicity at tumor cells upon exposure to light or ultrasound172 |
11 |
US20200069727A1 |
2022 |
Calcium peroxides NPs with a pH responsive coating for adjuvant therapy of hypoxic cancer tissues. The co-polymer used in the coating has stability at physiological pH but undergoes degradation at a pH lower than pH 7.4 (ref. 173) |
12 |
US20080139993A1 |
2012 |
NPs capable of absorbing electrons and photons (produced by PDT), that in turn produce oxygen radicals that can initiate a confined PDT effect174 |
13 |
US20170000887A1 |
2017 |
Uniform core–shell TiO2 coated upconversion NPs. The shell is made up of semiconductor material. The core emits wavelength that can excite the valance band electrons of the shell material. This in turn generates ROS in the cells175 |
14 |
CN107670040B |
2020 |
Gold nanocage-manganese dioxide composite NPs (gold nanocage inner core and manganese dioxide outer shell)176 |
15 |
CN111249461A |
2020 |
Phycocyanin-chlorin e6 covalent NPs with enhanced oxygen production in PDT, reduction in tumor growth and improving curative effect177 |
6 Future prospects
The tendency to use PDT extensively in the treatment of cancer, especially skin cancer is increasing day by day. Novel techniques are continuously being developed and tested. To design a more target-specific PDT regimen, molecular strategies are being considered. Details about numerous organic as well as inorganic NPs are already there in the literature.178 Although many governments and industries are investing a lot of resources in the nanocarrier-based approaches in PDT for cancer therapy, nanoformulations in general, face obstacles while getting translated into clinical practice. The most common adversities of nanoformulations include their irregular bio adhesiveness, unpredictable tumor permeation, inconsistent drug release profile, and inadequate data on long-term toxicity. Despite numerous scientific publications regarding PDT in skin carcinoma treatment, most of the nanoformulations are at the stage of in vivo clinical evaluation.7 Recent trends show that to enhance the efficacy of PDT in cancer treatment, NPs with active179–181 and passive functional roles are being explored now. Due to the massive advancement in nanotechnology and nanoscience in the last few years, several NPs with novel architecture, form, size, and mechanism of release have been developed to overcome the obstacles present in the incorporation of NPs in PDT for cancer treatment.178 One such obstacle is that the fluorescence imaging of PS deployed in PDT is restricted to superficial and only two-dimensional identification of tumors. To prepare a more effective treatment strategy, more emphasis on positron emission tomography/computed tomography agents as well as MRI probes in multimodal NPs can be seen in recent literature.182 However, focusing on incorporating NPs with PDT would not solve the innate disadvantages most NPs have. More attention should be given to the structural improvement of the NPs. The safety of NPs in long-term metabolism in our body, biocompatibility, targetability, and clinical applicability as disease-specific reactions should be explored and improved further.183 Ultimately, the invention of more safe, effective, and efficacious NP formulations is the primary goal to achieve. No matter how it is done, either by achieving an optimum excipient-API interaction or by incorporating a novel NP, the number one priority should be the patients' wellbeing.
7 Conclusion
PDT is one of the least invasive cancer treatment procedures available in the medical field. Other than melanoma and non-melanoma cancers, PDT is also used in oncological diseases of other organs and in several non-oncological disorders. The main benefits of PDT over other typical approaches are low systemic toxicity and high tumor targetability. PDT also causes lesser side effects and patients vulnerable to conventional treatments like radiation and chemotherapy can avail of this modality. PDT does not possess any drug resistance, thus repeating treatment without precipitating any cumulative toxicity is another beneficial feature. However, the tumor to be treated must be accessible to the light source. Endoscopically accessible tumors in the lung, bladder, and GIT are also being treated with PDT. There are a few drawbacks of PDT that are yet to be overcome. The pain experienced by the subject during the therapy is one of them. The long-term photosensitivity due to residual PS is also a major downside of PDT. These two factors jointly contribute to poor patient compliance. Moreover, the oxygen dependency of the therapy is another disadvantage. This need for oxygen limits the use of PDT in hypoxic tumors. The use of NPs in PDT resolves the problem of long-term toxicity but scientists must come forward with more novel ideas to increase patient compliance and broaden the limits of the application of PDT. The optimal choice of nanocarriers in PDT for skin cancer treatment is contingent upon several key considerations, foremost among them being the selection of the PS, the depth of the tumor, and the type of skin cancer being treated. PS selection hinges on its efficacy against the specific cancer type and its delivery feasibility. Finding the right balance is crucial, even though it's difficult. Subsequently, the choice of nanocarriers is influenced by tumor depth and the hydrophilic/hydrophobic nature of the PS molecule. Hydrophilic PS necessitates liposomal, niosomal, ethosomal, or polymeric nanocarriers for enhanced loading, whereas hydrophobic PS favors lipid nanocarriers such as SLNs or NLCs. However, considerations extend beyond mere hydrophilicity or hydrophobicity; deeper tumors which are often encountered in melanoma requiring targeted delivery may mandate surface-modified nanocarriers like functionalized nanoparticles, while microneedle patches may prove efficacious for widespread lesions. Quantum dots and carbon nanotubes offer unique advantages for specific conditions, such as low entrapment efficiency of certain PS molecules or high PS toxicity. Additionally, co-delivery scenarios involving multiple PS molecules or PS combined with chemotherapeutic agents necessitate tailored nanocarriers based on the latter's characteristics. In conclusion, the judicious selection of nanocarriers in topical PDT for skin cancer treatment demands a nuanced understanding of tumor characteristics and PS properties, underscored by the imperative of optimizing therapeutic efficacy and patient safety.
This review aims to succinctly summarize all the current information known about NPs that are topically administered in PDT, especially for skin carcinoma. In order to provide the reader a sense of contentment about the topic of employing nanocarriers in PDT for the treatment of skin cancer, both melanoma and non-melanoma skin cancer therapies have been covered in a systematic manner. Finally, it can be claimed that using nanocarriers in PDT for cancer therapy demonstrates immense capability and prospects towards a more dependable, and patient-compliant treatment method that must further be explored. Even though there is requirement of more research in this area, the nanoformulations should be pushed into clinical trials as soon as they provide encouraging findings in safety and therapeutic effectiveness.
Consent for publication
All authors are willing to publish the review article.
Data availability
No primary research results, software or code have been included and no new data were generated or analysed as part of this review.
Author contributions
Shambo Mohanty: writing – original draft; Vaibhavi Meghraj Desai: writing – review and editing; Rupesh Jain: writing – review and editing; Mukta Agrawal: writing – review and editing; Sunil Kumar Dubey: conceptualization, writing – review and editing; Gautam Singhvi: conceptualization, writing – review and editing, resources, project administration.
Conflicts of interest
The authors declare no competing interests.
Acknowledgements
This research did not receive any specific grant from funding agencies in the public, commercial, or not-for-profit sectors.
References
- J. B. Loureiro, M. Abrantes, P. A. Oliveira and L. Saraiva, Biochim. Biophys. Acta, Rev. Cancer, 2020, 1874, 188438 CrossRef CAS PubMed.
- G. Sánchez, J. Nova, A. E. Rodriguez-Hernandez, R. D. Medina, C. Solorzano-Restrepo, J. Gonzalez, M. Olmos, K. Godfrey and I. Arevalo-Rodriguez, Cochrane Database Syst. Rev., 2016, 2016, 1–32 Search PubMed.
- V. Krishnan and S. Mitragotri, Adv. Drug Deliv. Rev., 2020, 153, 87–108 CrossRef CAS PubMed.
- K. D. Miller, R. L. Siegel, C. C. Lin, A. B. Mariotto, J. L. Kramer, J. H. Rowland, K. D. Stein, R. Alteri and A. Jemal, CA A Cancer J. Clin., 2016, 66, 271–289 CrossRef PubMed.
- P. Clarke, Aust. Fam. Physician, 2012, 41, 474–480 Search PubMed.
- J. C. Martinez and C. C. Otley, Mayo Clin. Proc., 2001, 76, 1253–1265 CrossRef CAS PubMed.
- M. Lalan, P. Shah, K. Barve, K. Parekh, T. Mehta and P. Patel, Futur. J. Pharm. Sci., 2021, 7, 1–25 CrossRef.
- M. C. F. Simões, J. J. S. Sousa and A. A. C. C. Pais, Cancer Lett., 2015, 357, 8–42 CrossRef PubMed.
- S. Amini, M. H. Viera, W. Valins and B. Berman, J Clin Aesthet Dermatol., 2010, 3, 20–34 Search PubMed.
- B. Chua, J. E. Jackson, C. Lin and M. J. Veness, Oral Oncol., 2019, 98, 96–101 CrossRef PubMed.
- J. D. Birkmeyer, B. N. Reames, P. McCulloch, A. J. Carr, W. B. Campbell and J. E. Wennberg, Lancet, 2013, 382, 1121–1129 CrossRef PubMed.
- M. Veness and S. Richards, Australas. J. Dermatol., 2003, 44, 159–168 CrossRef PubMed.
- Y. Rong, L. Zuo, L. Shang and J. G. Bazan, Expert Rev. Anticancer Ther., 2015, 15, 765–776 CrossRef CAS PubMed.
- S. R. Lucena, N. Salazar, T. Gracia-Cazaña, A. Zamarrón, S. González, Á. Juarranz and Y. Gilaberte, Int. J. Mol. Sci., 2015, 16, 25912–25933 CrossRef CAS PubMed.
- R. Jain, R. Pradhan, S. Hejmady, G. Singhvi and S. K. Dubey, Spectrochim. Acta, Part A, 2021, 244, 118823 CrossRef CAS PubMed.
- M. Nakayama, K. Tabuchi, Y. Nakamura and A. Hara, J. skin cancer, 2011, 2011, 1–9 CrossRef PubMed.
- F. C. Ames and R. C. Hickey, South. Med. J., 1982, 75(920–3), 932 Search PubMed.
- C. Naidoo, C. A. Kruger and H. Abrahamse, Technol. Cancer Res. Treat., 2018, 17, 153303381879179 CrossRef PubMed.
- N. W. Nkune and H. Abrahamse, Int. J. Mol. Sci., 2021, 22, 12549 CrossRef CAS PubMed.
- M. Spiegel and C. Adamo, J. Phys. Chem. A, 2023, 127, 3625–3635 CrossRef CAS PubMed.
- R. Tong and D. S. Kohane, Wiley Interdiscip. Rev. Nanomed. Nanotechnol., 2012, 4, 638–662 CrossRef CAS PubMed.
- H. Abrahamse and M. R. Hamblin, Biochem. J., 2016, 473, 347–364 CrossRef CAS PubMed.
- A. P. Castano, T. N. Demidova and M. R. Hamblin, Photodiagnosis Photodyn. Ther., 2004, 1, 279–293 CrossRef CAS PubMed.
- M. Garcia-Diaz, Y.-Y. Huang and M. R. Hamblin, Methods, 2016, 109, 158–166 CrossRef CAS PubMed.
- Y.-Y. Wang, Y.-C. Liu, H. Sun and D.-S. Guo, Coord. Chem. Rev., 2019, 395, 46–62 CrossRef CAS.
- C. A. Robertson, D. H. Evans and H. Abrahamse, J. Photochem. Photobiol., B, 2009, 96, 1–8 CrossRef CAS PubMed.
- K. Plaetzer, B. Krammer, J. Berlanda, F. Berr and T. Kiesslich, Laser Med. Sci., 2009, 24, 259–268 CrossRef CAS PubMed.
- S. Parab, P. K. Achalla, N. Yanamandala, G. Singhvi, P. Kesharwani and S. K. Dubey, Nanomater. Photodyn. Ther., 2023, 81–103 Search PubMed.
- Q. Yao, J. Fan, S. Long, X. Zhao, H. Li, J. Du, K. Shao and X. Peng, Chem, 2022, 8, 197–209 CAS.
- S. Kwiatkowski, B. Knap, D. Przystupski, J. Saczko, E. Kędzierska, K. Knap-Czop, J. Kotlińska, O. Michel, K. Kotowski and J. Kulbacka, Biomed. Pharmacother., 2018, 106, 1098–1107 CrossRef PubMed.
- J. Kou, D. Dou and L. Yang, Oncotarget, 2017, 8, 81591–81603 CrossRef PubMed.
- T. K. Horne and M. J. Cronjé, Chem. Biol. Drug Des., 2017, 89, 221–242 CrossRef CAS PubMed.
- S. Kaneko and S. Kaneko, Int. J. Biomed. Imag., 2016, 2016, 6135293 Search PubMed.
- Y. Harada, Y. Murayama, T. Takamatsu, E. Otsuji and H. Tanaka, Int. J. Mol. Sci., 2022, 23, 6478 CrossRef CAS PubMed.
- D. Wöhrle, A. Hirth, T. Bogdahn-Rai, G. Schnurpfeil and M. Shopova, Russ. Chem. Bull., 1998, 47, 807–816 CrossRef.
- I. Yoon, J. Z. Li and Y. K. Shim, Clin. Endosc., 2013, 46, 7 CrossRef PubMed.
- J. F. Algorri, M. Ochoa, P. Roldán-Varona, L. Rodríguez-Cobo and J. M. López-Higuera, Cancers, 2021, 13, 1–29 Search PubMed.
- H. Kataoka, H. Nishie, N. Hayashi, M. Tanaka, A. Nomoto, S. Yano and T. Joh, Ann. Transl. Med., 2017, 5, 183 CrossRef PubMed.
- J. M. Dąbrowski and L. G. Arnaut, Photochem. Photobiol. Sci., 2015, 14, 1765–1780 CrossRef PubMed.
- R. Jain, S. K. Dubey and G. Singhvi, Drug Discov. Today, 2022, 27, 1176–1183 CrossRef CAS PubMed.
- L. Held, T. K. Eigentler, U. Leiter, C. Garbe and M. J. Berneburg, BioMed Res. Int., 2013, 2013, 102698 Search PubMed.
- K. Sztandera, M. Gorzkiewicz and B. Klajnert-Maculewicz, Wiley Interdiscip Rev Nanomed Nanobiotechnol, 2020, 12, 1–24 Search PubMed.
- S. Hejmady, R. Pradhan, A. Alexander, M. Agrawal, G. Singhvi, B. Gorain, S. Tiwari, P. Kesharwani and S. K. Dubey, Drug Discov. Today, 2020, 25, 2227–2244 CrossRef CAS PubMed.
- Y. N. Konan, R. Gurny and E. Allémann, J. Photochem. Photobiol., B, 2002, 66, 89–106 CrossRef CAS PubMed.
- M. J. Garland, C. M. Cassidy, D. Woolfson and R. F. Donnelly, Future Med. Chem., 2009, 1, 667–691 CrossRef CAS PubMed.
- B. W. Henderson and D. A. Bellnier, Ciba Found. Symp., 1989, 146, 112–130 CAS.
- J. K. Cullen, J. L. Simmons, P. G. Parsons and G. M. Boyle, Adv. Drug Deliv. Rev., 2020, 153, 54–64 CrossRef CAS PubMed.
- E. Eriksson, G. L. Griffith and K. Nuutila, Pharmaceutics, 2023, 15, 1–11 Search PubMed.
- C. H. Hong, K. A. Papp, K. W. Lophaven, P. Skallerup and S. Philipp, J. Eur. Acad. Dermatol. Venereol., 2017, 31, 1876–1883 CrossRef CAS PubMed.
- J. Sun, H. Zhao, L. Fu, J. Cui and Y. Yang, Clin. Cosmet. Invest. Dermatol., 2023, 16, 479–498 CrossRef CAS PubMed.
- I. J. Tan, G. N. Pathak and F. H. Silver, Cancers, 2023, 15, 3927 CrossRef CAS PubMed.
- M. Kim, H. Y. Jung and H. J. Park, Int. J. Mol. Sci., 2015, 16, 23259–23278 CrossRef CAS PubMed.
- V. K. Rapalli, A. Khosa, G. Singhvi, V. Girdhar, R. Jain and S. K. Dubey, Pharm. Qual. by Des. Princ. Appl., 2019, pp. 255–296 Search PubMed.
- V. K. Rapalli, T. Waghule, N. Hans, A. Mahmood, S. Gorantla, S. K. Dubey and G. Singhvi, J. Mol. Liq., 2020, 315, 113771 CrossRef CAS.
- S. S. Lucky, K. C. Soo and Y. Zhang, Chem. Rev., 2015, 115, 1990–2042 CrossRef CAS PubMed.
- S. Parveen and S. K. Sahoo, J. Drug Target., 2008, 16, 108–123 CrossRef CAS PubMed.
- J. Karlsson, H. J. Vaughan and J. J. Green, Annu. Rev. Chem. Biomol. Eng., 2018, 9, 105–127 CrossRef CAS PubMed.
- C. S. K. Reddy, R. Ghai, Rashmi and V. C. Kalia, Bioresour. Technol., 2003, 87, 137–146 CrossRef CAS PubMed.
- D. B. da Silva, C. L. da Silva, N. N. Davanzo, R. da Silva Souza, R. J. Correa, A. C. Tedesco and M. B. Riemma Pierre, Photodiagnosis Photodyn. Ther., 2021, 35, 102317 CrossRef CAS PubMed.
- X. Wang, L. Shi, Z. Huang and X. Wang, Twelfth Int. Conf. Photonics Imaging Biol. Med. (PIBM 2014), 2014, vol. 9230, p.92301D Search PubMed.
- Q. Meng, S. Zhong, L. Xu, J. Wang, Z. Zhang, Y. Gao and X. Cui, Carbohydr. Polym., 2022, 279, 119013 CrossRef CAS PubMed.
- D. Ion, A.-G. Niculescu, D. N. Păduraru, O. Andronic, F. Muşat, A. M. Grumezescu and A. Bolocan, Pharmaceutics, 2021, 14, 18 CrossRef PubMed.
- S. Pillarisetti, V. Vijayan, J. Rangasamy, R. Bardhan, S. Uthaman and I.-K. Park, J. Ind. Eng. Chem., 2023, 123, 361–370 CrossRef CAS.
- A. Paprikar, A. Soni, N. Kaushal and S. Lin, Nanotechnol. Life Sci., 2021, 345–372 Search PubMed.
- G. S. Kwon and T. Okano, Adv. Drug Deliv. Rev., 1996, 21, 107–116 CrossRef CAS.
- K. Miyata, R. J. Christie and K. Kataoka, React. Funct. Polym., 2011, 71, 227–234 CrossRef CAS.
- H. M. Aliabadi and A. Lavasanifar, Expet Opin. Drug Deliv., 2006, 3, 139–162 CrossRef CAS PubMed.
- Z. Ahmad, A. Shah, M. Siddiq and H. B. Kraatz, RSC Adv., 2014, 4, 17028–17038 RSC.
- I. Skidan, P. Dholakia and V. P. Torchilin, J. Drug Target., 2008, 16, 486–493 CrossRef CAS PubMed.
- Ł. Lamch, J. Kulbacka, M. Dubińska-Magiera, J. Saczko and K. A. Wilk, Photodiagnosis Photodyn. Ther., 2019, 25, 480–491 CrossRef PubMed.
- G. Bozzuto and A. Molinari, Int. J. Nanomed., 2015, 10, 975 CrossRef CAS PubMed.
- A. K. Jain and K. Mishra, Nanoparticles and Nanocarriers Based Pharmaceutical Formulations, Bentham Science Publishers, 2022 Search PubMed.
- A. Sharma and U. S. Sharma, Int. J. Pharm., 1997, 154, 123–140 CrossRef CAS.
- V. Reshetov, H. P. Lassalle, A. François, D. Dumas, S. Hupont, S. Gräfe, V. Filipe, W. Jiskoot, F. Guillemin, V. Zorin and L. Bezdetnaya, Int. J. Nanomed., 2013, 8, 3817–3831 CrossRef PubMed.
- Y. Dai, B. Wang, Z. Sun, J. Cheng, H. Zhao, K. Wu, P. Sun, Q. Shen, M. Li and Q. Fan, ACS Appl. Mater. Interfaces, 2019, 11, 39410–39423 CrossRef CAS PubMed.
- E. Temizel, T. Sagir, E. Ayan, S. Isik and R. Ozturk, Photodiagnosis Photodyn. Ther., 2014, 11, 537–545 CrossRef CAS PubMed.
- M. B. R. Pierre, A. C. Tedesco, J. M. Marchetti and M. V. L. B. Bentley, BMC Dermatol., 2001, 1, 1–6 CrossRef PubMed.
- I. M. Abdulbaqi, Y. Darwis, N. A. K. Khan, R. A. Assi and A. A. Khan, Int. J. Nanomed., 2016, 11, 2279–2304 CrossRef CAS PubMed.
- N. Dayan and E. Touitou, Biomaterials, 2000, 21, 1879–1885 CrossRef CAS PubMed.
- A. Zeb, S. T. Arif, M. Malik, F. A. Shah, F. U. Din, O. S. Qureshi, E. S. Lee, G. Y. Lee and J. K. Kim, Potential of Nanoparticulate Carriers for Improved Drug Delivery via Skin, Springer Singapore, 2019, vol. 49 Search PubMed.
- M. M. A. Elsayed, O. Y. Abdallah, V. F. Naggar and N. M. Khalafallah, Int. J. Pharm., 2006, 322, 60–66 CrossRef CAS PubMed.
- S. Nasr, M. Rady, A. Sebak, I. Gomaa, W. Fayad, M. El Gaafary, M. Abdel-kader, T. Syrovets and T. Simmet, Pharmaceutics, 2020, 12, 494 CrossRef CAS PubMed.
- N. Dragicevic-Curic, S. Gräfe, B. Gitter and A. Fahr, J. Photochem. Photobiol., B, 2010, 101, 238–250 CrossRef CAS PubMed.
- S. Nasr, M. Rady, I. Gomaa, T. Syrovet, T. Simmet, W. Fayad and M. Abdel-Kader, Int. J. Pharm., 2019, 568, 118528 CrossRef CAS PubMed.
- Y. P. Fang, Y. H. Tsai, P. C. Wu and Y. Bin Huang, Int. J. Pharm., 2008, 356, 144–152 CrossRef CAS PubMed.
- P. Bhardwaj, P. Tripathi, R. Gupta and S. Pandey, J. Drug Deliv. Sci. Technol., 2020, 56, 101581 CrossRef CAS.
- Y. M. V. Lohumi Ashutosh, R. Suman, S. Sidhyartha and S. Altaf bhai, J. Drug Deliv. Therapeut., 2012, 2, 129–135 Search PubMed.
- S. Liga, C. Paul, E. A. Moacă and F. Péter, Pharmaceutics, 2024, 16, 223 CrossRef CAS PubMed.
- S. Chen, S. Hanning, J. Falconer, M. Locke and J. Wen, Eur. J. Pharm. Biopharm., 2019, 144, 18–39 CrossRef CAS PubMed.
- H. Schreier and J. Bouwstra, J. Contr. Release, 1994, 30, 1–15 CrossRef CAS.
- D. B. Momekova, V. E. Gugleva and P. D. Petrov, ACS Omega, 2021, 6, 33265–33273 CrossRef CAS PubMed.
- M. Akhlaghi, M. Taebpour, N. N. Lotfabadi, S. M. Naghib, N. Jalili, L. Farahmand, B. F. Haghiralsadat, M. Rahmanian and D. Tofighi, Nanotechnol. Rev., 2022, 11, 1364–1385 CrossRef CAS.
- M. Bragagni, A. Scozzafava, A. Mastrolorenzo, C. T. Supuran and P. Mura, Int. J. Pharm., 2015, 494, 258–263 CrossRef CAS PubMed.
- L. B. de Oliveira de Siqueira, A. P. dos Santos Matos, P. E. Feuser, R. A. Machado-de-Ávila, R. Santos-Oliveira and E. Ricci-Júnior, J. Drug Deliv. Sci. Technol., 2022, 68, 103031 CrossRef CAS.
- G. Singhvi, V. K. Rapalli, S. Nagpal, S. K. Dubey and R. N. Saha, Nanocarriers as Potential Targeted Drug Delivery for Cancer Therapy, Nanoscience in Medicine, 2020, vol. 1, pp. 51–88 Search PubMed.
- E. B. Souto, I. Baldim, W. P. Oliveira, R. Rao, N. Yadav, F. M. Gama and S. Mahant, Expet Opin. Drug Deliv., 2020, 17, 357–377 CrossRef CAS PubMed.
- V. K. Rapalli, S. Sharma, A. Roy, A. Alexander and G. Singhvi, J. Drug Deliv. Sci. Technol., 2021, 63, 102442 CrossRef CAS.
- B. Lasa-Saracibar, A. Estella-Hermoso de Mendoza, M. Guada, C. Dios-Vieitez and M. J. Blanco-Prieto, Expet Opin. Drug Deliv., 2012, 9, 1245–1261 CrossRef CAS PubMed.
- S. I. Bukhari, S. S. Imam, M. Z. Ahmad, P. R. Vuddanda, S. Alshehri, W. A. Mahdi and J. Ahmad, Pharmaceutics, 2021, 13, 840 CrossRef CAS PubMed.
- S. Weber, A. Zimmer and J. Pardeike, Eur. J. Pharm. Biopharm., 2014, 86, 7–22 CrossRef CAS PubMed.
- P. L. Goto, M. P. Siqueira-Moura and A. C. Tedesco, Int. J. Pharm., 2017, 518, 228–241 CrossRef CAS PubMed.
- A. Qidwai, S. Khan, S. Md, M. Fazil, S. Baboota, J. K. Narang and J. Ali, Drug Deliv., 2016, 23, 1476–1485 CrossRef CAS PubMed.
- E. D. P. Almeida, L. V. Dipieri, F. C. Rossetti, J. M. Marchetti, M. V. L. B. Bentley, R. de S. Nunes, V. H. V. Sarmento, M. E. G. Valerio, J. J. Rodrigues Júnior, M. M. Montalvão, C. B. Correa and A. A. M. Lira, Photodiagnosis Photodyn. Ther., 2018, 24, 262–273 CrossRef CAS PubMed.
- S. A. Sibani, P. A. McCarron, A. D. Woolfson and R. F. Donnelly, Expet Opin. Drug Deliv., 2008, 5, 1241–1254 CrossRef CAS PubMed.
- R. W. J. Scott, O. M. Wilson and R. M. Crooks, J. Phys. Chem. B, 2005, 109, 692–704 CrossRef CAS PubMed.
- A. Shah and G. Singhvi, PharmaTutor, 2014, 2, 83–97 Search PubMed.
- C. H. Kim, S. G. Lee, M. J. Kang, S. Lee and Y. W. Choi, J. Pharm. Invest., 2017, 47, 203–227 CrossRef CAS.
- Y. Arif, N. Hasan, B. H. J. Gowda, G. Gupta, A. Alsayari, S. Wahab and P. Kesharwani, J. Drug Deliv. Sci. Technol., 2024, 105755 CrossRef CAS.
- U. Gupta, H. B. Agashe, A. Asthana and N. K. Jain, Nanomed.: Nanotechnol. Biol. Med., 2006, 2, 66–73 CrossRef CAS PubMed.
- L. Rodriguez, P. Vallecorsa, S. Battah, G. Di Venosa, G. Calvo, L. Mamone, D. Sáenz, M. C. Gonzalez, A. Batlle, A. J. MacRobert and A. Casas, Photochem. Photobiol. Sci., 2015, 14, 1617–1627 CrossRef CAS PubMed.
- K. Karthikeyan, A. Babu, S. J. Kim, R. Murugesan and K. Jeyasubramanian, Cancer Nanotechnol., 2011, 2, 95–103 CrossRef CAS PubMed.
- K. Sztandera, M. Marcinkowska, M. Gorzkiewicz, A. Janaszewska, R. Laurent, M. Zabłocka, S. Mignani, J. P. Majoral and B. Klajnert-Maculewicz, Int. J. Mol. Sci., 2020, 21, 1–20 Search PubMed.
- K. Sztandera, M. Gorzkiewicz, M. Bątal, V. Arkhipova, N. Knauer, J. Sánchez-nieves, F. Javier, D. Mata, R. Gómez, E. Apartsin and B. Klajnert, Int. J. Nanomed., 2022, 1139–1154 CrossRef CAS PubMed.
- A. Qidwai, Annu, B. Nabi, S. Kotta, J. K. Narang, S. Baboota and J. Ali, Photodiagnosis Photodyn. Ther., 2020, 30, 101782 CrossRef CAS PubMed.
- M. Lismont, L. Dreesen and S. Wuttke, Adv. Funct. Mater., 2017, 27, 1606314 CrossRef.
- J. Y. Kim, W. Il Choi, M. Kim and G. Tae, J. Contr. Release, 2013, 171, 113–121 CrossRef CAS PubMed.
- Y. Cheng, Y. Chang, Y. Feng, N. Liu, X. Sun, Y. Feng, X. Li and H. Zhang, Small, 2017, 13, 1–12 Search PubMed.
- R. Vankayala, Y. K. Huang, P. Kalluru, C. S. Chiang and K. C. Hwang, Small, 2014, 10, 1612–1622 CrossRef CAS PubMed.
- M. K. Khaing Oo, X. Yang, H. Du and H. Wang, Nanomedicine, 2008, 3, 777–786 CrossRef PubMed.
- B. Wang, J. H. Wang, Q. Liu, H. Huang, M. Chen, K. Li, C. Li, X. F. Yu and P. K. Chu, Biomaterials, 2014, 35, 1954–1966 CrossRef CAS PubMed.
- F. L. Primo, L. Michieleto, M. A. M. Rodrigues, P. P. Macaroff, P. C. Morais, Z. G. M. Lacava, M. V. L. B. Bentley and A. C. Tedesco, J. Magn. Magn. Mater., 2007, 311, 354–357 CrossRef CAS.
- J. P. Mbakidi, N. Drogat, R. Granet, T. S. Ouk, M. H. Ratinaud, E. Rivière, M. Verdier and V. Sol, Bioorg. Med. Chem. Lett., 2013, 23, 2486–2490 CrossRef CAS PubMed.
- F. L. Primo, M. M. A. Rodrigues, A. R. Simioni, M. V. L. B. Bentley, P. C. Morais and A. C. Tedesco, J. Magn. Magn. Mater., 2008, 320, 211–214 CrossRef.
- T. Waghule, N. Dabholkar, S. Gorantla, V. K. Rapalli, R. N. Saha and G. Singhvi, Biomed. Pharmacother., 2021, 141, 111940 CrossRef CAS PubMed.
- Z. Karami and M. Hamidi, Drug Discov. Today, 2016, 21, 789–801 CrossRef CAS PubMed.
- V. K. Rapalli, Y. Tomar, S. Sharma, A. Roy and G. Singhvi, Biomed. Pharmacother., 2023, 162, 114634 CrossRef CAS PubMed.
- S. A. Gaballa, O. H. El Garhy and H. Abdelkader, J. Adv. Biomedical Pharm. Sci., 2020, 3, 1–9 Search PubMed.
- D. Patel, B. Patel and H. Thakkar, Eur. J. Lipid Sci. Technol., 2021, 123, 1–12 CrossRef.
- H. M. G. Barriga, M. N. Holme and M. M. Stevens, Angew. Chem. Int. Ed., 2019, 58, 2958–2978 CrossRef CAS PubMed.
- M. Kluzek, A. I. I. Tyler, S. Wang, R. Chen, C. M. Marques, F. Thalmann, J. M. Seddon and M. Schmutz, Soft Matter, 2017, 13, 7571–7577 RSC.
- U. Bazylińska, J. Kulbacka, J. Schmidt, Y. Talmon and S. Murgia, J. Colloid Interface Sci., 2018, 522, 163–173 CrossRef PubMed.
- A. Zarepour, A. Khosravi, N. Y. Ayten, P. Ç. Hatır, S. Iravani and A. Zarrab, J. Mater. Chem. B, 2024, 12, 4307–4334 RSC.
- M. H. Karami, M. Abdouss, A. Rahdar and S. Pandey, Inorg. Chem. Commun., 2024, 161, 112032 CrossRef CAS.
- L. Qi and X. Gao, Expet Opin. Drug Deliv., 2008, 5, 263–267 CrossRef CAS PubMed.
- V. Bagalkot, L. Zhang, E. Levy-Nissenbaum, S. Jon, P. W. Kantoff, R. Langer and O. C. Farokhzad, Nano Lett., 2007, 7, 3065–3070 CrossRef CAS PubMed.
- S. Ahirwar, S. Mallick and D. Bahadur, J. Solid State Chem., 2020, 282, 121107 CrossRef CAS.
- A. Sahu, W. Il Choi, J. H. Lee and G. Tae, Biomaterials, 2013, 34, 6239–6248 CrossRef CAS PubMed.
- B. Tian, C. Wang, S. Zhang, L. Feng and Z. Liu, ACS Nano, 2011, 5, 7000–7009 CrossRef CAS PubMed.
- S. Pathak, E. Cao, M. C. Davidson, S. Jin and G. A. Silva, J. Neurosci., 2006, 26, 1893–1895 CrossRef CAS PubMed.
- M. S. Hasnain, S. A. Ahmad, M. N. Hoda, S. Rishishwar, P. Rishishwar and A. K. Nayak, Stimuli-responsive Carbon Nanotubes for Targeted Drug Delivery, Elsevier Ltd, 2018 Search PubMed.
- C. L. Brito, J. V. Silva, R. V. Gonzaga, M. A. La-Scalea, J. Giarolla and E. I. Ferreira, ACS Omega, 2024, 9, 8687–8708 CrossRef CAS PubMed.
- Q. Guo, X. tao Shen, Y. yuan Li and S. qing Xu, J. Huazhong Univ. Sci. Technol.–Med. Sci., 2017, 37, 635–641 CAS.
- F. Karchemski, D. Zucker, Y. Barenholz and O. Regev, J. Contr. Release, 2012, 160, 339–345 CrossRef CAS PubMed.
- R. O. Ogbodu, I. Ndhundhuma, A. Karsten and T. Nyokong, Spectrochim. Acta, Part A, 2015, 137, 1120–1125 CrossRef CAS PubMed.
- D. Jaafer and S. A. Mahdi, J. Kufa Phys., 2023, 15, 52–61 CrossRef.
- V. M. Desai, S. Priya, S. Gorantla and G. Singhvi, Pharmaceutics, 2022, 15, 14 CrossRef PubMed.
- N. Dabholkar, S. Gorantla, T. Waghule, V. K. Rapalli, A. Kothuru, S. Goel and G. Singhvi, Int. J. Biol. Macromol., 2021, 170, 602–621 CrossRef CAS PubMed.
- S. Gorantla, N. Dabholkar, S. Sharma, V. K. Rapalli, A. Alexander and G. Singhvi, Int. J. Biol. Macromol., 2021, 184, 438–453 CrossRef CAS PubMed.
- S. X. Chen, M. Ma, F. Xue, S. Shen, Q. Chen, Y. Kuang, K. Liang, X. Wang and H. Chen, J. Contr. Release, 2020, 324, 218–227 CrossRef CAS PubMed.
- H. P. Tham, K. Xu, W. Q. Lim, H. Chen, M. Zheng, T. G. S. Thng, S. S. Venkatraman, C. Xu and Y. Zhao, ACS Nano, 2018, 12, 11936–11948 CrossRef CAS PubMed.
- Galderma R&D, Metvix PDT Versus Cryotherapy in Patients With Primary Superficial Basal Cell Carcinoma, https://clinicaltrials.gov/study/NCT00469417?cond=MetvixPDTVersusCryotherapyinPatientsWithPrimarySuperficialBasalCellCarcinoma%26rank=1, accessed 21 October 2022.
- Case Comprehensive Cancer, Photodynamic Therapy Using Silicon Phthalocyanine 4 in Treating Patients With Actinic Keratosis, Bowen's Disease, Skin Cancer, or Stage I or Stage II Mycosis Fungoides, https://clinicaltrials.gov/study/NCT00103246?cond=PhotodynamicTherapyUsingSiliconPhthalocyanine4inTreatingPatientsWithActinicKeratosis,Bowen%27sDisease,SkinCancer,orStageIorStageIIMycosisFungoides%26rank=1, accessed 21 October 2022.
- Galderma R&D, Metvix PDT in Patients With ‘High Risk’ Basal Cell Carcinoma, https://clinicaltrials.gov/study/NCT00473343, accessed 21 October 2022.
- C. Haak, Fractional CO2 Laser Assisted Photodynamic Therapy, https://clinicaltrials.gov/study/NCT01260987?cond=FractionalCO2LaserAssistedPhotodynamicTherapy&rank=1, accessed 21 October 2022.
- Fujian Longhua Pharmaceutical Co. Ltd, Safety Study of Photodynamic Therapy Using Photocyanine Injection in Treating Patients With Malignant Tumors, https://clinicaltrials.gov/study/NCT01043016?cond=SafetyStudyofPhotodynamicTherapyUsingPhotocyanineInjectioninTreatingWithMalignant%26rank=1, accessed 21 October 2022.
- Roswell Park Cancer Institute, Photodynamic Therapy With HPPH Compared to Standard of Care Surgery in Treating Patients With Oral Cavity Cancer, https://clinicaltrials.gov/study/NCT03090412?cond=PhotodynamicTherapyWithHPPHComparedtoStandardofCareSurgeryinTreatingPatientsWithOralCavityCancer%26rank=1, accessed 21 October 2022.
- Wright State University, Photodynamic Therapy and Microvesicles, https://clinicaltrials.gov/study/NCT03960125?cond=PhotodynamicTherapyandMicrovesicles%26rank=1, accessed 21 October 2022.
- S. Ibbotson, Randomized Comparison of Low and Conventional Irradiance PDT for Skin Cancer, https://clinicaltrials.gov/study/NCT02872909?cond=RandomizedComparisonofLowandConventionalIrradiancePDTforSkinCancer%26rank=1, University of D., accessed 21 October 2022.
- Roswell Park Cancer Institute, Interstitial Photodynamic Therapy in Treating Patients With Recurrent Head and Neck Cancer, https://clinicaltrials.gov/study/NCT02068157?cond=InterstitialPhotodynamicTherapyinTreatingPatientsWithRecurrentHeadandNeckCancer%26rank=1, accessed 21 October 2022.
- Case Comprehensive Cancer, Alteration of the Immune Microenvironment in Basal Cell Carcinoma Following Photodynamic Therapy, https://clinicaltrials.gov/study/NCT05020912?cond=AlterationoftheImmuneMicroenvironmentinBasalCellCarcinomaFollowingPhotodynamicTherapy%26rank=1, accessed 21 October 2022.
- University of Arkansas, Interstitial Photodynamic Therapy (PDT) With Temoporfin for Advanced Head and Neck Cancers, https://clinicaltrials.gov/study/NCT01415986?cond=InterstitialPhotodynamicTherapy(PDT)WithTemoporfinforAdvancedHeadandNeckCancers%26rank=1, accessed 21 October 2022.
- Memorial Sloan Kettering Cancer, Use of Jet-injection in Photodynamic Therapy for Basal Cell Carcinoma, https://clinicaltrials.gov/study/NCT04552990?cond=UseofJet-injectioninPhotodynamicTherapyforBasalCellCarcinoma%26rank=1, accessed 21 October 2022.
- J. Xie, H. Chen and G. D. Wang, Induced photodynamic therapy using nanoparticle scintillators as transducers, EP3157337B1, https://patents.google.com/patent/EP3157337B1/en?oq=EP3157337B1, accessed 18 January 2024.
- A. Punjabi, Upconverting nanoparticles, US Pat., 9956426B2, https://patents.google.com/patent/US9956426B2/en?oq=US9956426B2, accessed 18 January 2024 Search PubMed.
- A. G. Gazizovich, A. G. Garifovich, G. D. Alexandrovich, K. T. Alexandrovna, P. S. Alekseevich, S. A. Gennadievich, S. G. Borisovich, K. B. Nikolaevich and K. N. Grigorievich, Method of selective destruction of malignant cells by magnetic microcontainers with photodynamic or photothermal dyes, RU2405600C2, https://patents.google.com/patent/RU2405600C2/en?oq=RU2405600C2+, accessed 18 January 2024.
- I. Kwon, G. Choi, G. Kim, I. Yoon, J. Kim and G. Park, Novel photosensitizer based on polymer derivatives-photosensitizer conjugates for photodynamic therapy, KP101035269B1, https://patents.google.com/patent/KR101035269B1/en?oq=KR101035269B1, accessed 18 January 2024.
- D. Zhang, Y. Zeng, J. Liu and X. Liu, Black phosphorus quantum dot/platinum hybrid mesoporous silica nanoparticle and preparation method and application thereof, CN109432422B, https://patents.google.com/patent/CN109432422B/en?oq=CN109432422B, accessed 18 January 2024.
- A. Haddadi, R. Madiyalakan and T. Woo, Polymeric nanoparticles for photosensitizers, EP2741775B1, https://patents.google.com/patent/EP2741775B1/en?oq=EP2741775B1, accessed 18 January 2024.
- P. Zhang, N. Wijesiri and H. Tang, Silver nanoparticle-enhanced photosensitizers, US Pat., 10420346B2, https://patents.google.com/patent/US10420346B2/en?oq=US10420346B2 Search PubMed.
- A. Volker, G. Burkhard, G. Susanna, W. Arno, E. Matthias, S. Janine and K. Ganesan, Calcium phosphate nanoparticles as dye carrier for photodynamic therapy, EP2198885B1, https://patents.google.com/patent/EP2198885B1/en?oq=EP2198885B1, accessed 18 January 2024.
- D. Dinakaran, R. Moore, J. Lewis, R. NARAIN, P. Kumar and N. Usmani, Theranostic Radiophotodynamic Therapy Nanoparticles, US Pat., 20210268129A1, https://patents.google.com/patent/US20210268129A1/en?oq=US20210268129A1, accessed 18 January 2024 Search PubMed.
- R. Madiyalakan, J. XING, T. Woo and E. Swanson, Nanoparticles for cancer sonodynamic and photodynamic therapy, US Pat., 20100262115A1, https://patents.google.com/patent/US20100262115A1/en?oq=US20100262115A1, accessed 18 January 2024 Search PubMed.
- A. McHale, J. Callan and Y. Sheng, Calcium peroxides nanoparticles as adjuvant therapy, US Pat., 20200069727A1, https://patents.google.com/patent/US20200069727A1/en?oq=US20200069727A1, accessed 18 January 2024 Search PubMed.
- A. Bensaoula, J. C. Boney, S. A. Beddar, T. M. Briere, S. Krishnan and A. K. Lee, Use of nanoparticles in the photodynamic treatment of tumors and non-destructive testing, US Pat., 20080139993A1, https://patents.google.com/patent/US20080139993A1/en?oq=US20080139993A1, accessed 18 January 2024 Search PubMed.
- Y. Zhang, Z. Li, N. M. Idris, K. C. Soo and S. S. Lucky, Uniform core-shell TiO2 coated upconversion nanoparticles and use thereof, US Pat., 20170000887A1, https://patents.google.com/patent/US20170000887A1/en?oq=US20170000887A1, accessed 18 January 2024 Search PubMed.
- L. Cai, R. Liang, L. Liu, Z. Han, H. He and M. Zheng, Gold nanocage-manganese dioxide composite nanoparticle and preparation method and application thereof, CN107670040B, https://patents.google.com/patent/CN107670040B/en?oq=CN107670040B, accessed 18 January 2024.
- J. Huang, D. Zhu, C. Lin and Y. Zhang, Preparation and application of phycocyanin-chlorin e6 covalent nanoparticles, CN111249461A, https://patents.google.com/patent/CN111249461A/en?oq=CN111249461A, accessed 18 January 2024.
- Y. J. Hou, X. X. Yang, R. Q. Liu, D. Zhao, C. X. Guo, A. C. Zhu, M. N. Wen, Z. Liu, G. F. Qu and H. X. Meng, Int. J. Nanomed., 2020, 15, 6827–6838 CrossRef CAS PubMed.
- W. T. Li, J. R. Peng, L. W. Tan, J. Wu, K. Shi, Y. Qu, X. W. Wei and Z. Y. Qian, Biomaterials, 2016, 106, 119–133 CrossRef CAS PubMed.
- H. Montaseri, C. A. Kruger and H. Abrahamse, Pharmaceutics, 2021, 13, 296 CrossRef CAS PubMed.
- A. Dey, G. Singhvi, A. Puri, P. Kesharwani and S. K. Dubey, J. Drug Deliv. Sci. Technol., 2022, 76, 103751 CrossRef PubMed.
- D. van Straten, V. Mashayekhi, H. S. de Bruijn, S. Oliveira and D. J. Robinson, Cancers, 2017, 9, 1–54 CrossRef PubMed.
- H. O. Alsaab, M. S. Alghamdi, A. S. Alotaibi, R. Alzhrani, F. Alwuthaynani, Y. S. Althobaiti, A. H. Almalki, S. Sau and A. K. Iyer, Cancers, 2020, 12, 1–26 CrossRef PubMed.
Footnote |
† Authors contributed equally. |
|
This journal is © The Royal Society of Chemistry 2024 |
Click here to see how this site uses Cookies. View our privacy policy here.