DOI:
10.1039/D2MA00941B
(Review Article)
Mater. Adv., 2023,
4, 2062-2069
Bioadhesives for clinical applications – a mini review
Received
30th September 2022
, Accepted 23rd March 2023
First published on 24th April 2023
Abstract
Bioadhesives are highly biocompatible and biodegradable polymers, which are used to join two surfaces where at least one of them is a living tissue. Bioadhesives are used for various purposes, for example, as a replacement for surgical sutures and as a substitute for traditional drug dosage systems. Bioadhesives meet the functional requirements needed for practical use in minimally invasive surgery. Bioadhesives are derived from either synthetic or biological source and their performance largely depends on the bioadhesion bonding state of the biopolymers, which is achieved by their cross-linking properties, chain length, and presence of various functional groups. Due to its biocompatibility, it can get in close contact with the biological substrate or adhere to the biological surface. This review deals with the overview of bioadhesives, their history, and the mechanism of adhesion along with the applications of bioadhesives.
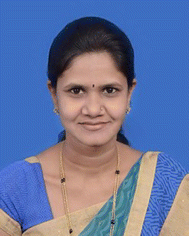
Uma K.
| Dr. Uma K. has received her MSc. degree from Kuvempu University, Shankaraghatta, Karnataka and PhD in Chemistry from Visvesvaraya Technological University, Belagavi, Karnataka. She is currently working as an Assistant Professor in the Department of Chemistry, Siddaganga Institute of Technology, Tumakuru, Karnataka since 2008. Her areas of interest include synthesis and applications of nano metal oxides, antimicrobial studies, biomaterials and peptidomimetics. She has published 8 research articles in international journals. |
Introduction
Wound closure and sealing incisions after surgery through bonding tissue at the injury site uses many tissue adhesives. Research studies have been working towards the replacement of current traditional typical process for wound closure with several bioadhesives for clinical applications.1 Bioadhesives have gained extensive scope because of their advantages, such as noninvasive sealing, easy operation, and instant hemostasis,2 and thereby changing the surgical process with the rapid development of noninvasive sealants.3–5 Currently, the market share for producing adhesives and sealants for surgical applications is $38 billion and it may go beyond $50 billion by 2022.6,7 Therefore, it is imperative to enhance the development of highly noninvasive bioadhesives for clinical applications. The classical invasive wound closure approaches, such as sutures, wires, and staples, are time consuming processes for tissue bonding and may cause tissue damage.8 Bioadhesives have the advantage of a lower possibility of tissue damage, require less time for tissue bonding, and can encourage wound curing via diverse mechanisms.9 For example, bioadhesives possess anti-inflammatory, antibacterial, and antioxidant properties.10,11 Additional properties, such as self-healing and injectability, increase the use of bioadhesives for easier applications.12 Commercially available bioadhesives include FocalSeal® developed to prevent air leakage at the time of lung surgery,13 DuraSeal® designed for the spine and dura sealing,14 and Coseal® employed as an aide of suture to prevent the leakage of blood vessels.15 Few of the currently available bioadhesives are cytotoxic and fragile; hence, major investigations have been concentrated on developing a benign and effective tissue adhesive to overcome these complications.
L. Xiong et al. reported the durable adhesiveness of the hydrogel that was attributed to the presence of a sufficient number of free-catechol groups in the hydrogel,16 which were produced during the dopamine oxidation process induced by clay nanosheets in the confined nanospace between the layers, as shown in Fig. 1.
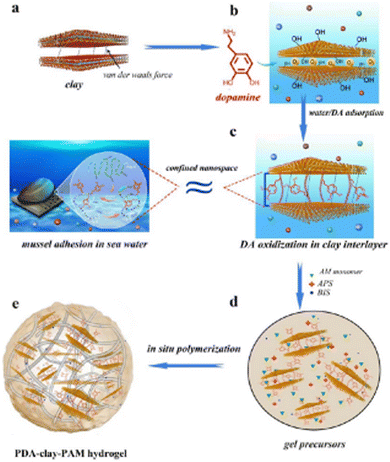 |
| Fig. 1 Design strategy for the preparation of PDA-clay-PAM hydrogel. (a) The layered structure of clay nanosheets. (b) DA molecule intercalated into the nanospace between the nanoclay layers. (c) Clay-induced DA oxidization to PDA in its nanospace, and the interlayer of clay nanosheets mimicked the confined nanospace of mussel's plaque. (d) AM monomers, cross-linkers (BIS), and initiator (APS) were added into the PDA-intercalated clay suspensions to form gel precursors. (e) The PDA-clay-PAM hydrogel was formed by in situ polymerization. Reprinted with permission from ACS Nano 2017, 11, 3, 2561–2574. Copyright © 2017 American Chemical Society. | |
A convenient copper-catalyzed azide-alkyne cycloaddition (CuAAC and click chemistry) was successfully introduced into injectable citrate-based mussel-inspired bioadhesive to improve both cohesive and wet adhesive strengths and elongate the degradation time, providing numerous advantages in surgical applications. The major challenge in developing such adhesives was the mutual inhibition effect between the oxidant used for crosslinking catechol groups and the Cu(II) reductant used for CuAAC (Fig. 2), which was successfully minimized by adding a biocompatible buffering agent typically used in cell culture, 4-(2-hydroxyethyl)-1-piperazineethanesulfonic acid (HEPES) as a copper chelating agent. Among the investigated formulations, the highest adhesion strength achieved (223.11 ± 15.94 kPa) was around 13 times higher than that of commercially available fibrin glue (15.4 ± 2.8 kPa).17
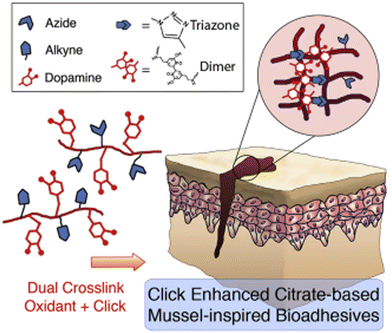 |
| Fig. 2 Dual crosslinking (oxidant and click (CuAAC)) of mussel-inspired bioadhesives. Reprinted with permission from Biomaterials 2017, 112, 275–286. Copyright © 2016 Elsevier Ltd. | |
A novel mussel-inspired BCD tissue glue made of bovine serum albumin (BSA), citrate acid (CA), and dopamine was developed aiming at internal medical applications. BSA was employed as a natural and biocompatible macromolecular backbone; CA was introduced as a dual-functional intermediate to increase the reactive carboxyl sites for the engraftment of dopamine onto the BSA backbone and also block the competing reactive amines from the proteinic backbone. Timely curing and stable adhesion were achieved between the biological tissue substrates via instant chelation and gradual conjugation of DOPA-catechol groups in the BCD glue. Within 30 min, this newly developed BCD tissue glue could provide over 10-fold greater adhesion stress than that of commercially available fibrin glue in a wet environment.18 A new hydrogel made of hyaluronan, poly(vinylphosphonic acid), and chitosan was fabricated and characterized to be used for skin wound healing application by D. H. Phuc et al.19 To design ideal bioadhesives for clinical use, several critical factors are considered, such as matchable mechanical properties to the treated tissue under physiological conditions, biocompatibility and nontoxicity without causing severe infection or inflammation, tunable biodegradability that matches the wound repair/tissue regeneration process, and on-demand operation and easy storage.20 F.V. Zanutto et al. developed a transdermal bioadhesive containing artemether (ART), an alternative, potentially lifesaving, the treatment regimen for malaria in low-resource settings. Bioadhesives were prepared from an aqueous blend of hydroxyethylcellulose (4.5% w/w), ART, propoxylated-ethoxylated-cetyl-alcohol, polysorbate 80, propyleneglycol, glycerine, mineral oil, and oleic acid. In this study, the average pore size of the bioadhesive was 52.6 ± 15.31 μm.21 Further Sutureless repair of corneal injuries using naturally derived bioadhesive hydrogels was investigated by Sani et al. A transparent and adhesive photocrosslinkable hydrogel for the treatment of corneal stromal defects was synthesized. The engineered hydrogel mimicked the mechanical properties of the native cornea and comprised a chemically modified form of hydrolyzed collagen, which provided enzymatic degradation sites and physiological cell adhesion motifs.22 In a study conducted by D. Yang et al., a hybrid photopolymeric bioadhesive system consisting of urethane methacrylated dextran (DexU) and 3, 4-dihydroxyphenyl-L-alanine (DOPA) modified three-arm poly (ethylene glycol)s (PEG-DOPAs) was designed, which showed better biocompatibility.23 In one of the studies, light-activated, mussel protein-based bioadhesive (LAMBA) inspired by mussel adhesion was prepared and LAMBA exhibited substantially stronger bulk wet tissue adhesion than commercially available fibrin glue and showed good biocompatibility in both in vitro and in vivo studies.24
Shagan et al. have developed an adhesive system that is based on the ability of short star-shaped N-hydroxy succinimide-modified polycaprolactone (PCL-NHS) polymers to melt at low temperatures and quickly solidify under physiological conditions.25 Applying this biomaterial using a hot glue gun enables a simple and accessible application to wounds with minimal pain. To address the issues related to tissue damage, such as hemostasis and promotion of angiogenesis, Zou et al., constructed the multifunctional adhesive (G-DLPUs) by compounding GelMA with biobased degradable polyurethane (DLPU), which possesses excellent adhesion and could release L-Arg during its degradation process.26 This double network-structure hydrogel adhesive would absorb exudates from the wound with a porous structure and form strong adhesion at the interface of skin and liver to promote rapid hemostasis.
Further, one of the prominent adhesives, i.e., cyanoacrylates or acrylic tissue adhesive, synthesized by the condensation of a cyanoacetate with formaldehyde, has been used as a surgical glue for over 50 years. Participation from amino groups on the tissue surface can also occur during the polymerization resulting in a strong bond with the tissue. While the cyanoacrylate with the shortest alkyl group (–CH3) produces a rigid polymer, flexibility is improved with the longer alkyl chain and adding plasticizer as well. As a result, many cyanoacrylates with longer chain derivatives have been developed, such as ethyl-2-cyanoacrylate (Epiglu®) and Krazy Glue® (Elmer's Products Inc.), butyl-2-cyanoacrylate (Trufill®), Indermil®, Histoacryl®, 2-octyl-cyanoacrylate (Dermabond®), and Surgiseal. Among these, 2-octyl-cyanoacrylate (Dermabond®) was the first cyanoacrylate approved by FDA (in 1998) to be used as a tissue adhesive. Some of the benefits of cyanoacrylate tissue adhesives are the ease of application for first aid, quick adhesion or sealing of wounded tissues, excellent hemostasis, and potential bacteriostatic or microbial barrier properties.27
2. Mechanism of adhesion
In order to better engineer bioadhesives, the underlying adhesion mechanism has to be explored. The role and effectiveness of varying terminal groups (e.g., OH–, COOH–, NH2–, and CH3–) in bioadhesives to cell adhesion have been systematically studied using self-assembly layers with different terminations. The OH– group is found to have the highest adhesion strength to cells, followed by COOH–, NH2–, and then CH3–. Compared with the hydrophobic CH3–, hydrophilic groups (e.g., OH–, COOH–, and NH2–) have increased flowability, wettability, and penetration through hydrogen bonding. These principles have encouraged the design of synthetic bioadhesives such as cyanoacrylate and its variants. Then, the hydrogels due to their high content of water can accelerate wound healing by maintaining a moist environment. They can be easily loaded with hydrophilic drugs and effectively release upon thermal stimuli for treatments. Due to their great tunability, hydrogel bioadhesives have been functionalized or customized through material and structural engineering, such as dual-networking hydrogels, immunomodulatory hydrogels, and injectable hydrogels, for specific application scenarios.28–30
An alternative tissue adhesive in the form of a dry double-sided tape (DST) is made from a combination of a biopolymer (gelatin or chitosan) and crosslinked polyacrylic acid (PAAc) grafted with N-hydrosuccinimide ester. The adhesion mechanism depends on the removal of the interfacial water from the tissue surface, resulting in fast temporary crosslinking to the surface. Subsequent covalent crosslinking with amine groups on the tissue surface further improves the adhesion stability and strength of the DST. To provide stable adhesion, the NHS ester groups grafted on the PAAc also couple covalently with primary amine groups on various tissues within a few minutes, without the need for further pressure, subsequent covalent crosslinks are necessary for stable and robust adhesion of wet surfaces.31
The mechanisms of bioadhesion may be classified into these main categories: electrostatic bonding, intermolecular bonding, chain entanglement, mechanical interlocking, and surface wetting. Fig. 3 shows pictorial representations of the main mechanisms relevant to bioadhesion.
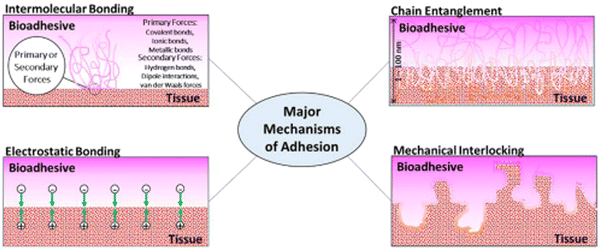 |
| Fig. 3 Classifications of the adhesion mechanism. Reproduced from Biomater. Sci., 2020, 8, 1240–1255 with permission from the Royal Society of Chemistry. | |
2.1. Electrostatic bonding
The proximity of tissue and adhesive surfaces can lead to the transfer of electrons between them due to differences in electron band structures, leading to the formation of an electric double-layered interface, whose adhesive strength is the result of the interaction among the attractive forces present in the dual layer, which accounts for the resistance to separation. Although this mechanism is not relevant for non-metallic adhesion, it seems to play a role in bioadhesion, as in the case of electron transfer at the interface of mucoadhesion.
2.2. Intermolecular bonding
It is considered as the main mechanism of bioadhesion. According to the adsorption theory, the adhesion between adhesive and adherent is due to the surface forces acting between the chemical structures at the two surfaces when they are pressed together. These surface forces are classified as primary and secondary. Examples of primary forces are the so-called chemisorption that encompass cationic, ionic, and metallic chemical bonds, which are high-energy bonds that are formed at the interface and result in strong adhesion. Secondary forces form low-energy bonds, but they have a significant effect resulting from the large number of bonding sites that may exist at any given interface. Some of these secondary forces are hydrogen bonding, van der Waals forces, London dispersion, and dipole–dipole interactions.
2.3. Chain entanglement
Semi-permanent bonds between the adhesive and tissue surface can be formed when polymer chains from the former permeate into the tissue surface and attain a depth of enough penetration to hold the bonding. The interpenetration of the polymer chains is driven by concentration gradients where the polymer chains penetrate the tissue and glycoprotein chains from the tissue in turn penetrate the adhesive until an equilibrium depth is reached. The diffusive depth of the adhesive depends on the diffusion coefficient between tissue and adhesive, which in turn is related to the molecular weight of the polymeric chains and the crosslinking density of the adhesive. A good example is the interpenetration and entanglement of glycoproteins and adhesive polymeric chains at the interface of mucous membranes that occurs in mucoadhesive drug delivery systems.
2.4. Mechanical interlocking
The interaction between adhesive and tissue can result in the adhesive penetrating the tissue micro irregularities and interlocking with the tissue to create the binding. The tissue's surface roughness plays an important role in mechanical interlocking as the adhesive locks onto microscopic roughness. An example of such kind of adhesion is the use of polymer-based adhesives, which penetrate the tissue and later expand once inside the microscopic crevices, thus, mechanically interlocking with the tissue. Another example is the use of polymeric microneedles based on poly(styrene) and poly(acrylic acid), which penetrate the tissue and then later swell in the presence of water inside, leading to local tissue deformation and consequent interlocking within the tissue surface.
2.5. Surface wetting
Although not included in the four major mechanisms of adhesion, surface wetting is an important contributor to this phenomenon. The wetting theory is related to the capacity of the adhesive to disperse and create a molecular binding on the tissue surface. The molecular contact between the adhesive and tissue results in the development of the previously discussed primary and secondary surface forces, which are the source of intermolecular bonding. Wetting is defined as the process of establishing very close and continuous contact between the adhesive and the tissue. An adhesive can effectively wet an adherent if its surface tension is lower than the critical surface tension of the adherent. In order to have a high adhesive bond with the tissue, the adhesive must completely wet the tissue by maximizing contact with the tissue topography by flowing into the defects and crevices of the tissue. High-quality wetting and adhesiveness are achieved when the angle of contact between the adhesive and adherent is minimal, as shown in Fig. 4.32–38
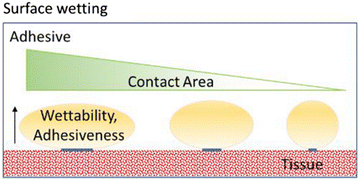 |
| Fig. 4 Surface wetting is considered an important contributor to adhesiveness. If the adhesive makes extended molecular contact with the tissue surface, the contact angle will be close to zero, resulting in high-quality wetting and an adhesive effect. Reproduced from Biomater. Sci., 2020, 8, 1240–1255 with permission from the Royal Society of Chemistry. | |
The properties required from medical glue
Physical attributes, such as hydrophobicity, hydrophilicity, and charge determine biocompatibility. For example, the high charge density of hyper-branched poly(ethylene imine) can disrupt cell membranes, leading to cytotoxic effects.39 These concerns regarding biocompatibility dictate the choice of materials and chemistries used for tissue adhesive. One common example of adhesives exploiting the mechanism of physical entanglement is acrylate-based adhesives that undergo polymerization in the presence of free radical initiators. Photoinitiators are typically utilized to supply free radicals on demand, enabling in situ polymerization. Before cross-linking, prepolymers containing acrylates are allowed to diffuse into the tissue and then cross-linked to form a polymer network interweaved with the tissue.
Therefore, hydrogen bonds are typically combined with other chemical strategies to form stable adhesion. In addition, adhesives using hydrogen bonds usually suffer from high swelling, owing to their hydrophilic characteristics.
Regardless of the chemical strategy utilized for tissue adhesion, fluids at the tissue surface can interfere with bonding. Hydrogels of poly(ethylene glycol) or poly(acrylic acid) are known to rapidly absorb water due to their hydrophilic nature. When these hydrogels are in contact with wet tissues, they are able to remove the interfacial water and achieve robust tissue adhesion through physical and chemical cross-linking, with gentle mechanical pressure.40 This approach can aid in forming fast and robust tissue adhesion, but it can also lead to excessive swelling of the adhesive. Although many strategies have been pursued to attenuate their swelling, their hydrophilic nature typically leads to significant swelling. In addition, adhesives based on NHS esters require dry storage because of the hydrolytic instability of NHS esters. Therefore, their application usually involves multiple preparation steps, including dissolving the polymers, which can extend the application time.
The other example, hyaluronic acid (HA) is one of the most hydrophilic molecules in nature, with high water-binding capacity, and is often described as a natural moisturizer. The major biological functions of HA typically include regulation of the viscoelasticity of biofluids, such as joint synovial and eye vitreous fluid, and maintenance of tissue hydration and water transport. Its hydrophilic nature along with biocompatibility and nonimmunogenicity has led to its use in a wide range of clinical applications, and wound dressing.41
Hydrogen bonding is utilized in tissue adhesives to provide relatively fast, reversible bonding with tissues. Hydrogen bonding arises from partial intermolecular interactions between a hydrogen bonded to a strongly electronegative atom and another electronegative atom with a lone pair of electrons. Many chemical groups, including hydroxyl, carboxylic acid, and primary amines, on the tissue surface can form hydrogen bonds with tissue adhesives. While a single hydrogen bond is relatively weak, significant adhesive strength can be achieved when multiple bonds are formed. Typical examples of adhesives that form hydrogen bonds with tissues are those based on acrylic acid. The repeating carboxylic acid in these adhesives elicits a high density of hydrogen bonds with tissues and allows for adhesion. However, it should be noted that hydrogen bonds can be easily neutralized in the presence of water, resulting in their dissociation. For the successful formation of hydrogen bonds, the tissue surface needs to be dried before the adhesives are applied, but the subsequent presence of blood and body fluids can eventually dissociate the hydrogen bonds. Therefore, hydrogen bonds are typically combined with other chemical strategies to form stable adhesion. In addition, adhesives using hydrogen bonds usually suffer from high swelling, owing to their hydrophilic characteristics.
Another noncovalent interaction used in tissue adhesion is hydrophobic interaction. Hydrophobic interactions involve the tendency of nonpolar hydrophobic molecules, such as alkyl chains, to aggregate with the exclusion of water molecules. When hydrophobic functional groups are present in polymer chains, the polymer chains tend to aggregate at the hydrophobic sites. Studies revealed that an increase in the number of alkyl groups first led to higher adhesion, while alkyl chains with more than 8 carbons decreased adhesion. This suggests that there is an optimal hydrophobicity for tissue adhesion. However, hydrophobic interactions usually exhibit low bonding strength and, therefore, may not significantly contribute to the formation of tissue adhesion. Hydrophobic groups can be alternatively exploited as water repellants to displace water on the tissue surface, preventing the adhesive reactive molecules from being exposed to water and enhancing their reactions. In addition, the hydrophobic nature could also lead to low swelling.42
Swelling is a common feature of hydrophilic polymer adhesives but it can also be detrimental to maintaining adhesion and to surrounding tissues. Classes of adhesives that typically exhibit significant swelling include those based on PEG, acrylic acid, gelatin, and collagen. For example, DuraSeal, a dura sealant based on PEGtrilysine, swells up to 50% in all dimensions after implantation. The volumetric expansion of adhesives during swelling can exert mechanical compression on the surrounding tissue. Depending on the tissue type, the resultant compression may exceed the physical tolerance of the tissue and result in poor physiological outcomes. Swelling also alters the mechanical properties of adhesives, as the increasing amount of water within the adhesive matrix leads to a decrease in the polymer density. Excessive water can also interfere with cross-linking of the matrix. In contrast, swelling can be exploited when adhesives are used for hemostasis. A variety of approaches, including increasing the cross-linking density and increasing hydrophobicity, have been used to attenuate the swelling behavior of adhesives. As a higher crosslink density increases mechanical resistance to expansion, PEG-based adhesives can successfully suppress swelling by introducing more cross-links.43,44
3. Applications
3.1. Wound healing of soft and hard tissues
One of the most common uses of bioadhesives is wound healing. For many years, wound closure has been carried out with wires, sutures, and staples.45 Nevertheless, concerns about the sign of scar, secondary damage, slowed wound healing, and complicated postoperative care have limited their applications. Smart bioadhesives have become more popular because people are more concerned with their physical appearance.46 Zhao et al. created a new stimulus-responsive bioadhesive made up of a prepolymer of poly(glycerol sebacate)-co-poly(ethylene glycol)-g-catechol.47 Three incisions (2 cm) were made on the rat's backs to evaluate their capacity to close wounds. Liang et al. fabricated a smart bioadhesive through dual-dynamic bond cross-linking between Fe, protocatechualdehyde containing catechol and aldehyde groups, and quaternized chitosan.48 A full-thickness incision model was used to examine wound closure's efficacy. On day 7 post-surgery, the sealed incision treated with the bioadhesive exhibited complete epidermis and dermis structures and higher collagen deposition levels than the control group, and the incision closed with surgical sutures. Treatment for wounds of brittle and hard tissues is another type of wound healing where smart bioadhesives are beneficial.49
3.2. Drug delivery
Recent innovations in smart drug carrier systems seem promising, as they supply a means to promote formulations of targeted drug delivery systems, and drug release control based on stimuli response.50 Smart bioadhesives in delivery have an advantage over typical hydrogel delivery systems in that they can fix delivered objects on the site. Mucoadhesion is effective in enhancing the bioavailability of poorly absorbed drugs by lengthening their residence time in the gastrointestinal tract, resulting in lower doses and dosage frequency.51,52 Yan et al. prepared53 adhesive hydrogel with pH, temperature, and NIR light-responsive behavior for use in controlled release systems, and in one of the studies, researchers fabricated a stimuli-responsive bioadhesive by incorporating graphene aerogel into a PNIPAM network with the incorporated PDA nanoparticles. Smart bioadhesives can also be an important topic in both agricultural and environmental chemistry.
3.3. Leak sealants in the medical field
A common complication of surgeries and injuries is leakage. Headaches, meningitis, and seizures can result from cerebrospinal fluid leaks caused by traumas or brain and sinus surgery.54 Gastric fluid leakage, common during surgical operations, can result in infection and significant tissue destruction.55 As a result, leakage control is critical in lowering operation risks, complications, and costs. Tissue sealants, also known as smart bioadhesives for leakage prevention, have piqued the interest of researchers and have shown tremendous promise in the clinic. Blacklow et al. created a thermo-responsive bioadhesive to speed wound healing contracting at body temperature. Bioadhesive dressings could help heal wounds in other epithelial tissues, such as the gut, lung, and liver. Bleeding is one of the most common side effects caused by surgical procedures, injuries, diseases, and medications.56 Hemostasis sealants are widely accessible on the market. However, they have separate limits. Chang et al. presented a hemostatic photo-responsive bioadhesive based on gelatin methacryloyl that was able to prevent bleeding following oral/dental surgical procedures. According to the findings, the bioadhesive could be immediately extruded into the bleeding site and shortened blood clotting time by 45%. Furthermore, it may be easily removed from the bleeding site after clotting and preventing subsequent wound harm. Guo et al.57 prepared a hemostatic smart bioadhesive composed of hemocoagulase (the same as reptilase) and GelMA, inspired by the coagulation function of snake venom. Blood clotting time with visible light-responsive bioadhesive was about 45 s compared with 5 to 6 min without bioadhesive. Hemostatic bioadhesives achieved hemostasis in 45 s on a liver incision and 34 s on a cut rat tail, reducing blood loss by 79% and 78%, respectively.
3.4. Wearable medical devices
Nowadays, implantable and wearable medical devices such as tissue scaffolds, biodetectors, and biosensors are attracting a substantial amount of attention.58–67 However, conformal and stable contact between such devices and the target tissue needs to be established. This fixation requires the use of sutures and wires, raising the risk of infection, scaffold deterioration, and subsequent injury. Smart bioadhesives have the potential to replace invasive fixing procedures with a noninvasive adhesion method. Deng et al. designed an electrical bioadhesive based on a thin layer of graphene nanocomposite that can provide rapid and on-demand detachable integration of bioelectronic devices onto a variety of wet tissues. They then successfully recorded an epicardial electrocardiogram using the synthesized bioadhesive on-site and electrically stimulated a sciatic nerve in a rat model. Zhu et al. prepared a smart ionic gelatin/PAAm/clay bioadhesive with high conductivity and high self-healing efficiency, which can be used as a capacitive pressure sensor for human motion monitoring.
Antibacterial applications of bioadhesives
Bioadhesives are of great interest for tissue/wound closure to reduce surgical time, minimize treatment invasiveness, and prevent body fluid leakage. However, bacterial infections remain a major concern during wound healing and tissue bonding. Hence, the development of bioadhesives with antibacterial properties is necessary. In this study, a hydrogel bioadhesive based on silk fibroin (SF) and tannic acid (TA) was combined with silver nanoparticles (AgNPs) generated in situ during the hydrogel formation to improve its antibacterial abilities. A natural polymer-based bioadhesive with self-healing behavior and improved antibacterial properties were developed. Further, dressings, and hydrogels with great adhesion, permeability, antibacterial properties, and biocompatibility have recently been developed. Pham et al. reported gelatin polyethylene glycol-dopamine (AgNPs-gel-PEG-DA) hydrogels, which improved their adhesiveness by grafting catechol groups onto the main gelatin chain. Simultaneously, hydrogels have excellent antibacterial properties against Gram-positive and Gram-negative bacteria. A plant-inspired long-lasting adhesive bilayer nanocomposite hydrogel based on redox-active Ag/tannic acid-Cellulose nanofibers was achieved. This type of hydrogel has simultaneous, good adhesion and antibacterial effects.68–70
Apart from showing antibacterial activities, bioadhesives find application in bioelectronics. Gan et al. proposed a mussel-inspired strategy to construct hydrophilic conductive polymer nanoparticles (CP NPs) while endowing the CP NPs with redox activity and biocompatibility. This conductive and adhesive hydrogel shows good electroactivity and biocompatibility and therefore has broad applications in the electrostimulation of tissue regeneration and implantable bioelectronics.71 In one of the studies, a green strategy is developed for fabricating conductive, redox-active, water-soluble nanosheets via the self-assembly of poly(3,4-ethylenedioxythiophene) (PEDOT) on the polydopamine-reduced and sulfonated graphene oxide (PSGO) template.72 The conductivity and hydrophilicity of nanosheets were highly improved by PSGO and can be used as versatile nanofillers in developing conductive and adhesive hydrogels.
Potential challenges involved in the clinical translation of bioadhesives
Translating research into clinical practice is a global priority because of its potential impact on health services delivery and outcomes. Despite the ever-increasing depth and breadth of health research, most areas across the globe seem to be slow to translate relevant research evidence into clinical practice. For the past few decades, tissue sealants and adhesives have been developed as an alternative to sutures and staples to close and seal wounds or incisions. These materials are advantageous because of their ease of use, short application time, and minimal tissue damage, making them suitable for minimally invasive procedures. However, there is a large gap between the amount of research on tissue adhesives and the number of products available. To bridge this gap, there is a need to better understand the challenges to the clinical translation of tissue adhesives. In particular, the adhesive design must be informed by a deep understanding of the target tissue's surface characteristics and environment, which vary considerably among the tissue types. Moreover, understanding and monitoring the long-term performance of a material post-implant is crucial; this includes monitoring the chemical and physical properties of the implanted adhesives over time, tissue responses, and the resultant changes in adhesion and cohesion. In addition, early-stage consideration of the unmet clinical need and the regulatory and development paths could lower the barriers in the development cost and effort, facilitating clinical translation. An ideal tissue adhesive should have the following properties: strong adhesion to the tissue, especially under wet conditions, an ability to form a watertight seal, biodegradability, mechanical compliance with the underlying substrate, low cytotoxicity, and minimal inflammatory response. The use of tissue sealants and adhesives can provide significant benefits to patients, surgeons, and healthcare providers by reducing operation time and tissue handling and mitigating surgical complications, such as infection.
The main challenge is achieving adequate adhesion to soft tissues, especially when tissues are wet and under dynamic forces. Careful stratification of target applications and more in-depth analysis of tissue-surface properties and tissue-environmental characteristics are required in the early development phase, an understanding of the characteristics of the tissue surface and the environment (for example, disease type and state), as well as an understanding of the clinical scenario, is crucial for achieving predictive outcomes.73,74
Concluding remarks and future perspectives
Before closing this review, we discuss the future prospects of biomaterials for fundamental biological studies with reference to current limitations. In this review, we briefly summarize the synthesis of various bioadhesives, mechanisms of adhesion, and applications. In addition, the characteristics of bioadhesives create on-demand drug delivery and achieve better therapeutic effects. Bioadhesives are gaining more and more interest in various biomedical and drug delivery markets primarily due to the advancement of synthesis techniques and fabrication technology. Therefore, bioadhesives combined with multifunctional nanomaterials approaches will be beneficial for extending their applications in the future.
Author contributions
Uma K., writing – original draft, review, editing, and supervision.
Conflicts of interest
There are no conflicts to declare.
Acknowledgements
Thanks to all the corresponding authors of the research articles referred, which provided permission to use the figures for free.
References
- F. Scognamiglio, A. Travan, I. Rustighi, P. Tarchi, S. Palmisano and E. Marsich, J. Biomed. Mater. Res., Part B, 2016, 104, 626 CrossRef CAS PubMed
.
- E. J. Lee, B. K. Huh, S. N. Kim, J. Y. Lee, C. G. Park and A. G. Mikos, Prog. Mater. Sci., 2017, 89, 392 CrossRef CAS PubMed
.
- N. Annabi, A. Tamayol, S. R. Shin, A. M. Ghaemmaghami, N. A. Peppas and A. Khademhosseini, Nano Today, 2014, 9, 574 CrossRef CAS PubMed
.
- Bioadhesives market size, share & trends analysis report by source and segment forecasts, 2015–2022. San Fransisco, CA: Grand View Research 2016, 105.
- A. Vernengo, Adhesive Materials for Biomedical Applications, Intech Open, 2016, 6, 111 Search PubMed
.
- W. D. Spotnitz, ISRN Surg., 2014, 2014 Search PubMed
.
- A. M. Handorf, Y. Zhou, M. A. Halanski and W. J. Li, Organogenesis, 2015, 11, 1 CrossRef PubMed
.
- K. Modaresifar, S. Azizian and A. Hadjizadeh, Polym. Rev., 2016, 56, 329 CrossRef CAS
.
-
S. Ebnesajjad and C. F. Ebnesajjad, Surface Treatment of Materials for Adhesion Bonding, William Andrew Pub., Norwich, NY, USA, 2006 Search PubMed
.
- N. Pandey, A. Hakamivala, C. Xu, P. Hariharan, B. Radionov and Z. Huang, Adv. Healthcare Mater., 2018, 7, e1701069 CrossRef PubMed
.
-
K. Xu, Q. Chang, Y. Liu and M. Xing, Racing for the surface, 2020, p. 693 Search PubMed
.
- J. C. Wain, L. R. Kaiser and D. W. Johnstone, Ann. Thorac. Surg., 2001, 71, 1623 CrossRef CAS PubMed
.
-
H. Bianco-Peled and M. Davidovich-Pinhas, Bioadhesion and Biomimetics-From Nature to Applications, CRC Press, Boca Rotan, Florida, 2015, ch. 9, p. 203 Search PubMed
.
- L. Ge and S. Chen, Polymers, 2020, 12, 939 CrossRef CAS PubMed
.
- A. Assmann, A. Vegh, Rad-M. Ghasemi, S. Bagherifard, G. Cheng and E. S. Sani, Biomaterials, 2017, 140, 115 CrossRef CAS PubMed
.
- L. Han, X. Lu, K. Liu, K. Wang, L. Fang, L. T. Weng and Z. Li, ACS Nano, 2017, 11, 2561 CrossRef CAS PubMed
.
- J. Guo, G. B. Kim, D. Shan, J. P. Kim, J. Hu, W. Wang and J. Yang, Biomaterials, 2017, 112, 275 CrossRef CAS PubMed
.
- W. Zhu, Y. Peck, J. Iqbal and D. A. Wang, Biomaterials, 2017, 147, 99 CrossRef CAS PubMed
.
-
D. H. Phuc, N. Thi Hiep, N. P. Do Chau, N. Thi Thu Hoai, H. Chan Khon, V. Van Toi and B. Chi Bao, Int. J. Polym. Sci., 2016.
- J. Li, X. Yu, E. E. Martinez, J. Zhu, T. Wang, S. Shi and C. Guo, Macromol. Biosci., 2022, 2, 2100340 CrossRef PubMed
.
- F. V. Zanutto, E. McAlister, M. M. P. Tangerina, B. Fonseca-Santos, T. Salles, H. C. Souza and M. A. Foglio, J. Pharm. Sci., 2019, 108, 1177 CrossRef PubMed
.
- E. Shirzaei Sani, A. Kheirkhah, D. Rana, Z. Sun, W. Foulsham, A. Sheikhi and N. Annabi, Sci. Adv., 2019, 5, eaav1281 CrossRef PubMed
.
- C. Li, T. Wang, L. Hu, Y. Wei, J. Liu, X. Mu and D. Yang, Mater. Sci. Eng., 2014, 35, 300 CrossRef CAS PubMed
.
- E. Y. Jeon, B. H. Hwang, Y. J. Yang, B. J. Kim, B. H. Choi, G. Y. Jung and H. J. Cha, Biomaterials, 2015, 67, 11 CrossRef CAS PubMed
.
- A. Shagan, W. Zhang, M. Mehta, S. Levi, D. S. Kohane and B. Mizrahi, Adv. Funct. Mater., 2020, 30, 1900998 CrossRef CAS
.
- F. Zou, Y. Wang, Y. Zheng, Y. Xie, H. Zhang, J. Chen, M. I. Hussain, H. Meng and J. Peng, Bioact. Mater., 2022, 17, 471 CrossRef CAS PubMed
.
- S. Tarafder, G. Y. Park, J. Felix and C. H. Lee, Acta Biomater., 2020, 117, 77 CrossRef CAS PubMed
.
- J. Zhu, H. Zhou, E. M. Gerhard, S. Zhang, F. I. P. Rodriguez, T. Pan, H. Yang, Y. Lin, J. Yang and H. Cheng, Bioact. Mater., 2023, 19, 360 CrossRef PubMed
.
- X. Zhao, S. Li, X. Du, W. Li, Q. Wang, D. He and J. Yuan, Bioact. Mater., 2022, 8, 196 CrossRef CAS PubMed
.
- X. Xu, X. Xia, K. Zhang, A. Rai, Z. Li, P. Zhao, K. Wei, L. Zou, B. Yang, W. K. Wong and P. W. Y. Chiu, Sci. Transl. Med., 2020, 12, eaba8014 CrossRef CAS PubMed
.
- H. Yuk, C. E. Varela, C. S. Nabzdyk, X. Mao, R. F. Padera, E. T. Roche and X. Zhao, Nature, 2019, 575, 169 CrossRef CAS PubMed
.
- M. Mehdizadeh and J. Yang, Macromol. Biosci., 2013, 13, 271 CrossRef CAS PubMed
.
- A. Ahuja, R. K. Khar and J. Ali, Drug Dev. Ind. Pharm., 1997, 23, 489 CrossRef CAS
.
- S. J. Marshall, S. C. Bayne, R. Baier, A. P. Tomsia and G. W. Marshall, Dent. Mater., 2010, 26, e11 CrossRef PubMed
.
- K. Modaresifar, S. Azizian and A. Hadjizadeh, Polym. Rev., 2016, 56, 329 CrossRef CAS
.
- N. Pandey, L. F. Soto-Garcia, J. Liao, P. Zimmern, K. T. Nguyen and Y. Hong, Biomater. Sci., 2020, 8, 1240 RSC
.
- Y. Jian, Y. Liping, L. Meng-Fang, M. Jan, L. Xuehong and L. P. See, Small, 2013, 9, 596–603 CrossRef PubMed
.
- F. C. Carvalho, M. L. Bruschi, R. C. Evangelista and M. P. D. Gremiao, Braz. J. Pharm. Sci., 2010, 46, 1 CrossRef CAS
.
- S. Nam and D. Mooney, Chem. Rev., 2021, 121, 11336 CrossRef CAS PubMed
.
- X. Mao, H. Yuk and X. Zhao, J. Mech. Phys. Solids, 2020, 137, 103863 CrossRef CAS
.
- D. Zhou, S. Li, M. Pei, H. Yang, S. Gu, Y. Tao, D. Ye, Y. Zhou, W. Xu and P. Xiao, ACS Appl. Mater. Interfaces, 2020, 12, 18225 CrossRef CAS PubMed
.
- P. Ferreira, A. F. Silva, M. I. Pinto and M. H. Gil, J. Mater. Sci.: Mater. Med., 2008, 19, 111 CrossRef CAS PubMed
.
- H. Zhang, T. Zhao, B. Newland, P. Duffy, A. N. Annaidh, E. D. OCearbhaill and W. Wang, J. Mater. Chem. B, 2015, 3, 6420 RSC
.
- M. C. Preul, P. K. Campbell, D. S. Garlick and R. F. Spetzler, J. Neurosurg., 2010, 12, 381 Search PubMed
.
- B. Schnabel, M. Scharf, K. Schwieger, M. Windolf, B. Van der Pol, V. Braunstein and A. Appelt, Clin. Biomech., 2009, 24, 855 CrossRef PubMed
.
- S. Rathi, R. Saka, A. J. Domb and W. Khan, Polym. Adv. Technol., 2019, 30, 217 CrossRef CAS
.
- X. Zhao, Y. Liang, Y. Huang, J. He, Y. Han and B. Guo, Adv. Funct. Mater., 2020, 30, 1910748 CrossRef CAS
.
- Y. Liang, Z. Li, Y. Huang, R. Yu and B. Guo, ACS Nano, 2021, 15, 7078 CrossRef CAS PubMed
.
- M. Rahimnejad and W. Zhong, RSC Adv., 2017, 7, 47380 RSC
.
- A. R. Shirvan, A. Bashari and N. Hemmatinejad, Eur. Polym. J., 2019, 119, 541 CrossRef
.
- Y. Liang, X. Zhao, T. Hu, B. Chen, Z. Yin, P. X. Ma and B. Guo, Small, 2019, 15, e1900046 CrossRef PubMed
.
- N. A. N. Hanafy, S. Leporatti and M. El-Kemary, Appl. Sci., 2019, 9, 825 CrossRef CAS
.
- Y. H. Yan, L. H. Rong, J. Ge, B. D. B. Tiu and P. F. Cao, Macromol. Mater. Eng., 2019, 304, 1800720 CrossRef
.
- N. Epstein, Surg. Neurol. Int., 2014, 5, 304 CrossRef PubMed
.
- J. Wu, H. Yuk, T. L. Sarrafian, C. F. Guo, L. G. Griffiths, C. S. Nabzdyk and X. Zhao, Sci. Transl. Med., 2022, 14 Search PubMed
.
- H. Kiratli and B. Tarlan, Clin. Ophthalmol., 2013, 7, 1163 CrossRef PubMed
.
- Y. Guo, Y. Wang, X. Zhao, X. Li, Q. Wang, W. Zhong, K. Mequanint, R. Zhan, M. Xing and G. Luo, Sci. Adv., 2021, 7, eabf9635 CrossRef CAS PubMed
.
- A. R. Shirvan, A. Bashari and N. Hemmatinejad, Eur. Polym. J., 2019, 119, 541 CrossRef
.
- N. A. N. Hanafy, S. Leporatti and M. El-Kemary, Appl. Sci., 2019, 9, 825 CrossRef CAS
.
- D. Zheng, B. Bai, H. Zhao, X. Xu, N. Hu and H. Wang, Colloids Surf., B, 2021, 207, 112004 CrossRef CAS PubMed
.
- M. Qu, X. Jiang, X. Zhou, C. Wang, Q. Wu, L. Ren, J. Zhu, S. Zhu, P. Tebon and W. Sun, Adv. Healthcare Mater., 2020, 9, e1901714 CrossRef PubMed
.
- P. Abdollahiyan, B. Baradaran, M. de la Guardia, F. Oroojalian and A. Mokhtarzadeh, J. Controlled Release, 2020, 328, 514 CrossRef CAS PubMed
.
- N. Epstein, Surg. Neurol. Int., 2014, 5, 304 CrossRef PubMed
.
- J. Wu, H. Yuk, T. L. Sarrafian, C. F. Guo, L. G. Griffiths, C. S. Nabzdyk and X. Zhao, Sci. Transl. Med., 2022, 14 Search PubMed
.
- H. Kiratli and B. Tarlan, Clin. Ophthalmol., 2013, 7, 1163 CrossRef PubMed
.
- G. H. Lee, H. Moon, H. Kim, G. H. Lee, W. Kwon, S. Yoo, D. Myung, S. H. Yun, Z. Bao and S. K. Hahn, Nat. Rev. Mater., 2020, 5, 149 CrossRef PubMed
.
- F. Zheng, W. Xiong, S. Sun, P. Zhang and J. J. Zhu, Nanophotonics, 2019, 8, 391 CrossRef CAS
.
- X. Ke, Z. Dong, S. Tang, W. Chu, X. Zheng, L. Zhen, X. Chen, C. Ding, J. Luo and J. Li, Biomater. Sci., 2020, 8, 4346 RSC
.
- Y. Chen, Y. Zhang, A. Mensaha, D. Li, Q. Wang and Q. Wei, Carbohydr. Polym., 2021, 255, 117508 CrossRef CAS PubMed
.
- T. N. Pham, Y. S. Jiang, C. F. Su and J. S. Jan, Int. J. Biol. Macromol., 2020, 146, 1050 CrossRef CAS PubMed
.
- D. Gan, T. Shuai, X. Wang, Z. Huang, F. Ren, L. Fang, K. Wang, C. Xie and X. Lu, Nano-Micro Lett., 2020, 12, 1 CrossRef PubMed
.
- D. Gan, Z. Huang, X. Wang, L. Jiang, C. Wang, M. Zhu, F. Ren, L. Fang, K. Wang, C. Xie and X. Lu, Adv. Funct. Mater., 2020, 30, 1907678 CrossRef CAS
.
- G. M. Taboada, K. Yang, M. J. Pereira, S. S. Liu, Y. Hu, J. M. Karp, N. Artzi and Y. Lee, Nat. Rev. Mater., 2020, 5, 310 CrossRef
.
- H. Zhu, J. Tian, H. Mao and Z. Gu, Curr. Opin. Biomed. Eng., 2021, 18, 100271 CrossRef CAS
.
|
This journal is © The Royal Society of Chemistry 2023 |
Click here to see how this site uses Cookies. View our privacy policy here.