DOI:
10.1039/C5RA21313D
(Paper)
RSC Adv., 2016,
6, 22827-22838
Synthesis and evaluation of neuroprotective 4-O-substituted chrysotoxine derivatives as potential multifunctional agents for the treatment of Alzheimer's disease†
Received
14th October 2015
, Accepted 12th February 2016
First published on
22nd February 2016
Abstract
A series of 4-O-substituted chrysotoxine (CTX) derivatives were designed, synthesized and evaluated as multifunctional agents for the treatment of Alzheimer's disease (AD). In vitro assays indicated that four ring substituted compounds (2a, 2b, 3i and 3j) exhibited significant neuroprotective effects against Aβ25–35-induced toxicity in PC12 cells. The four compounds also inhibited self- and Cu2+-induced Aβ1–42 aggregation and acted as biometal chelators. In particular, compound 2a was a potential lead compound for AD treatment (cell viability up to 100.78% at 50 µM in Aβ25–35-treated PC12 cells, 51.88% and 58.03% inhibition at 25 µM for self- and Cu2+-induced Aβ1–42 aggregation, respectively). A metal chelating experiment showed that compound 2a had a moderate interaction with Cu2+ and Al3+. Moreover, western blot analysis showed that compound 2a attenuated Aβ-induced tau protein hyperphosphorylation at Ser199/202 and Ser396 sites. Furthermore, compound 2a could efficiently cross the blood-brain barrier (BBB) by a parallel artificial membrane permeability assay (PAMPA). In summary, these results suggested that compound 2a was a promising multifunctional compound for AD therapy.
1. Introduction
Alzheimer's disease (AD), characterized by a progressive loss of memory and cognitive impairments, is one of the most common neurodegenerative diseases mainly occurring in the elderly.1 The World Alzheimer Report 2015 showed that 46.8 million people have been suffering from AD and this number is expected to exceed 131.5 million in 2050.2 Current therapeutic drugs for AD include acetylcholinesterase inhibitors (tacrine, donepezil, rivastigmine and galantamine)3 and N-methyl-D-aspartate (NMDA) receptor antagonists (memantine).4 Although these single-target drugs have a modest and transient improvement in memory and cognitive function, they cannot prevent progressive neurodegeneration.3 The etiology of AD still remains elusive, but several factors, such as β-amyloid (Aβ) deposits,5 τ-protein hyperphosphorylation,6 metal dyshomeostasis,7 oxidative stress8 and low levels of acetylcholine,9 have been greatly implicated in AD pathogenesis.
Among the multiple factors that induce AD, Aβ plays a primary role.10 The Aβ hypothesis states that the production and accumulation of Aβ peptides in the brain are central events in the AD pathogenesis.11,12 Aβ is a peptide chain cleaved from amyloid precursor protein (APP) ranging in length from 39 to 43 amino acids.13 Aβ1–40 and Aβ1–42 are the main isoforms of Aβ peptides.14 A sustained imbalance between the production and clearance of Aβ40–42 fragments leads to the accumulation of Aβ peptide monomers, oligomers, and finally promotes the large aggregated Aβ plaques, which are thought to initiate the pathogenic cascade, ultimately leading to neuronal loss and dementia.15–17 Another hallmark of AD pathology is the intracellular accumulation of hyperphosphorylated tau protein, which leads to neurofibrillary tangles in the brain.18 Therefore, an inhibition of Aβ aggregation or tau hyperphosphorylation has been recognized as an effective therapeutic strategy for AD.
In addition, recent studies have indicated that excessive biometals (such as Cu2+, Fe2+, Zn2+ and Al3+) exist in the brains of AD patients.19 The abnormal accumulation of biometal ions is closely associated with the formation of Aβ plaques and neurofibrillary tangles, which have been found to induce neurotoxicity.20,21 The modulation of brain biometals represents an additional rational approach for the treatment of AD.
A major impediment to the development of effective compounds for AD therapy is that essentially 100% of large-molecule drugs and >98% of small-molecule drugs fail to cross the blood-brain-barrier (BBB).22 Increasing the lipid solubility of target compounds is regarded as a promising way to improve their BBB permeability.23
Chrysotoxine (CTX), a natural bibenzyl (4-hydroxy-3,5,3′,4′-tetramethoxybibenzyl), has been isolated from plants in genus Dendrobium, the source of a valuable and scarce traditional medicine named “Shihu” in China.24–26 Recent studies have revealed that CTX can inhibit SH-SY5Y cells apoptosis induced by neurotoxins 6-hydroxydopamine and 1-methyl-4-phenyl pyridinium (MPP+) in Parkinson's disease model, which suggested its potential protective effect against neurodegeneration.27,28 Similar to resveratrol, CTX contains two aromatic end groups and a linker region in the middle, which are critical for the efficient bond at the Aβ peptide guided π-stacking.29 However, the scarce source of CTX restrict its further development.
In this paper, we synthesized CTX and its derivatives modified by several series of hydrophobic moieties as potential neuroprotective agents for AD. Then we chose four compounds with significant neuroprotective effects to explore their potential multifunctional properties for AD therapy by the pharmacological evaluations, including self- and Cu2+-induced Aβ aggregation, tau phosphorylation and metal chelation. Meanwhile, theoretical prediction and experimental assay were performed to explore their BBB permeability.
2. Result and discussion
2.1. Chemistry
The natural compound CTX was totally synthesized with Wittig reaction as a key step, which was widely used for the synthesis of bibenzyl skeleton.30 Syringaldehyde (4-hydroxy-3,5-dimethoxylbenzaldehyde, 2) was used as the starting material. Based on the acetylation protection of hydroxyl group, 4-hydroxymethyl-2,6-dimethoxyphenyl acetate (4) was obtained by aldehyde reduction with sodium borohydride. With further hydroxyl chlorination of compound 4, we got the intermediate 4-chloromethyl-2,6-dimethoxyphenyl acetate (5), which was reacted with triphenylphosphine to prepare the phosphonium salt (6), the key material for the Wittig reaction. The phosphonium salt was dissolved completely with 3,4-dimethoxybenzaldehyde in THF and deprotonated with NaH to yield a mixture of Z- and E-stilbenes, which were reduced by treatment with catalytic amounts of 10% Pd–C over H2 (4 atm) to afford bibenzyl skeleton. After deacetylation with sodium methylate, CTX was finally obtained through a total 7-step procedure, and the whole yield of our 7-step reaction was up to 54.1%, which was summarized in Scheme 1.
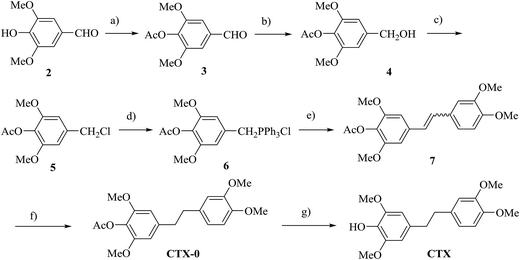 |
| Scheme 1 Synthesis steps of lead compound chrysotoxine (CTX). (a) Acetic anhydride, DCM, DMAP, rt, 30 min; (b) NaBH4, EtOH, 0 °C, rt, 30 min; (c) SOCl2, Et3N, DCM, 0 °C, rt, 3 h; (d) PPh3, toluene, reflux, 5 h; (e) 3,4-dimethoxybenzaldehyde, NaH, THF, Ar, 0 °C, rt, overnight; (f) H2, 10% Pd/C, EtOAc, 4 atm; (g) MeONa/MeOH, rt, 30 min. | |
The chrysotoxine-4-O-substituted esterified derivatives 1a–1f were catalyzed by EDC·HCl in just one step, while compounds 2a–2e were obtained through the esterification with the acid chloride intermediate. These procedures were shown in Scheme 2. The 4-O-substituted chrysotoxine ether derivatives 3a–3j were synthesized by the combination of halon compounds and CTX in DMF, which was described in Scheme 3.
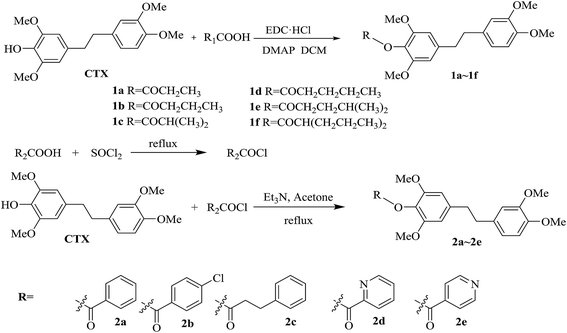 |
| Scheme 2 Synthetic procedure of 4-O-ester substituted CTX derivatives. | |
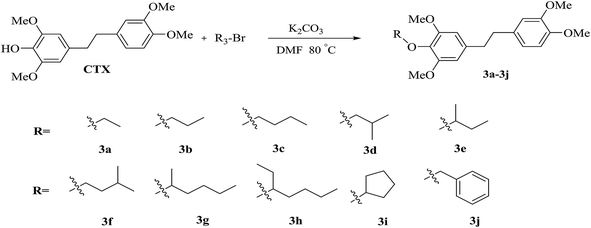 |
| Scheme 3 Synthetic procedure of 4-O-ether substituted CTX derivatives. | |
All compounds were purified by recrystallization or chromatography technology. The analytical and spectroscopic data confirmed their structures, which were shown as details in the Experimental section.
2.2. Pharmacology
2.2.1. Neuroprotective activity against Aβ25–35-induced toxicity in PC12 cells.
To investigate the neuroprotective activity of all designed compounds, we used MTT assay to explore their effects on Aβ25–35-induced toxicity in PC12 cells.31–33 Cells were pretreated with varying concentrations (50 µM, 25 µM and 10 µM) of test compounds for 0.5 h, and then Aβ25–35 (10 µM) was added for an additional 24 h. For model group, only Aβ25–35 was added. Data were expressed as percent of control group. As shown in Table 1, Aβ25–35 significantly decreased the cell viability compared with control group (53.82% of control, p < 0.001). Four ring substituted compounds (2a, 2b, 3i and 3j) showed significant neuroprotective activity with cell viability higher than 70% compared to model group. Among the four compounds, 2a (R = benzoyl) displayed the most potent neuroprotective activity (cell viability up to 100.78% at 50 µM) and 2b (R = p-chlorobenzoyl) exhibited slightly weaker activity than 2a. Replacement of benzoyl group (2a, 2b) by pyridine carbonyl group (2d, 2e) killed the neuroprotective activity, which revealed that pyridine ring had negligible effect on Aβ-induced toxicity. When benzoyl substitution (2a, 2b) was replaced by alkyl chain substitution (1a–1f and 3a–3h), the neuroprotective activity dramatically decreased. However, the activity reversed when alkyl chain substitution was replaced by benzyl (3i) or cyclohexyl (3j), which confirmed that ring substitution was beneficial to the neuroprotective activity. The most possible reason was that there was an interaction between tricyclic system of CTX derivatives and Aβ peptides. Therefore, the four ring substituted compounds (2a, 2b, 3i and 3j) with significant neuroprotection were selected to assess their potential multifunctional properties for AD treatment.
Table 1 Neuroprotective activity of 23 bibenzyl derivatives against Aβ25–35-induced toxicity in PC12 cellsa
Com. |
Cell viabilityb (%) |
Com. |
Cell viabilityb (%) |
50 µM |
25 µM |
10 µM |
50 µM |
25 µM |
10 µM |
10 µM Aβ25–35 was added to all the test groups and model group to develop the nerve damage model for 24 h. Compounds were added 0.5 h prior to Aβ25–35 addition. Cell viability was assessed by measuring the MTT reduction.
Data were the mean ± SD expressed as percent of the untreated Aβ25–35 control group. Results are the mean of 3 independent experiments in triplicate. ###p < 0.001 vs. control; ***p < 0.001, **p < 0.01, *p < 0.05 vs. model (ANOVA followed by Newman–Keuls test).
|
CTX
|
58.89 ± 2.47 |
55.02 ± 2.91 |
52.73 ± 4.18 |
2e
|
46.56 ± 1.42 |
50.71 ± 2.85 |
53.53 ± 3.51 |
CTX-0
|
48.87 ± 3.51 |
50.01 ± 4.01 |
48.47 ± 3.93 |
3a
|
46.15 ± 3.14 |
50.92 ± 3.42 |
53.84 ± 2.57 |
1a
|
44.40 ± 6.21 |
44.21 ± 4.30 |
45.31 ± 5.11 |
3b
|
48.81 ± 1.55 |
49.11 ± 1.43 |
49.21 ± 2.62 |
1b
|
49.37 ± 4.02 |
50.12 ± 2.21 |
54.89 ± 3.56 |
3c
|
53.44 ± 1.80 |
52.03 ± 3.44 |
51.79 ± 3.09 |
1c
|
45.91 ± 1.91 |
47.33 ± 3.54 |
49.34 ± 2.81 |
3d
|
49.89 ± 2.47 |
50.12 ± 1.96 |
54.53 ± 1.88 |
1d
|
48.63 ± 3.14 |
41.90 ± 2.91 |
49.73 ± 4.18 |
3e
|
48.75 ± 2.02 |
49.73 ± 4.72 |
49.02 ± 3.61 |
1e
|
49.57 ± 4.15 |
50.40 ± 2.04 |
49.83 ± 1.01 |
3f
|
49.48 ± 3.72 |
50.16 ± 4.70 |
50.21 ± 1.51 |
1f
|
60.02 ± 1.89(*) |
55.21 ± 2.71 |
50.11 ± 1.85 |
3g
|
52.18 ± 1.99 |
50.25 ± 3.08 |
50.67 ± 1.40 |
2a
|
100.78 ± 2.51(***) |
80.12 ± 2.56(***) |
58.18 ± 2.09 |
3h
|
58.88 ± 3.51 |
54.78 ± 2.51 |
51.03 ± 2.77 |
2b
|
95.62 ± 1.22(***) |
69.24 ± 1.52(**) |
56.41 ± 3.04 |
3i
|
72.64 ± 5.12(**) |
61.02 ± 1.05(*) |
54.77 ± 2.79 |
2c
|
49.84 ± 1.09 |
50.22 ± 2.47 |
54.00 ± 1.85 |
3j
|
71.00 ± 2.45(***) |
60.89 ± 1.67(*) |
58.22 ± 2.84 |
2d
|
54.60 ± 3.02 |
50.10 ± 6.07 |
52.97 ± 3.76 |
Model |
53.82 ± 2.72(###) |
Control |
100.05 ± 3.12 |
2.2.2. Inhibition of self-induced Aβ1–42 aggregation by compounds 2a, 2b, 3i and 3j.
Thioflavin T (ThT) fluorescence assay34 was used to assess the ability of the four selected compounds (2a, 2b, 3i and 3j) to inhibit the self-induced Aβ1–42 aggregation. Curcumin (Cur) and resveratrol (Res) were used as reference compounds (Fig. 1). From the results, four compounds showed moderate-to-good activities (inhibition ranged from 34.52% to 57.20% at 50 µM) and exhibited concentration-dependent effects. Benzoyl substituted CTX derivatives 2a and 2b displayed better activities than 3i and 3j, which was consistent with their neuroprotective activities. Notably, the inhibition of compound 2a was 51.88% at 25 µM (57.20% at 50 µM), which was competitive with the two reference compounds (Cur 42.70% and Res 50.20% at 25 µM). Therefore, compound 2a might serve as a potent Aβ1–42 self-aggregation inhibitor for AD treatment.
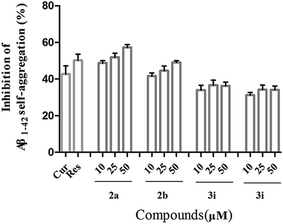 |
| Fig. 1 Inhibition of Aβ1–42 self-induced aggregation by compounds 2a, 2b, 3i and 3j. Curcumin (Cur) and resveratrol (Res) were reference compounds. The thioflavin T fluorescence method was used and the measurements were carried out in the presence of test compounds in different concentrations. The mean ± SD values from three independent experiments were shown. | |
2.2.3. Inhibition of Cu2+-induced Aβ1–42 aggregation by compounds 2a, 2b, 3i and 3j.
To investigate the inhibition of these selected compounds in Cu2+-induced Aβ1–42 aggregation, we conducted a ThT-binding assay.35 Resveratrol and curcumin were chosen as reference compounds (Fig. 2). The fluorescence of Aβ1–42 treated with Cu2+ is 148.78% compared to that of Aβ1–42 alone, which indicated that Cu2+ could accelerate Aβ1–42 aggregation. By contrast, the fluorescence of Aβ1–42 treated with Cu2+ and test compounds decreased dramatically (2a, 58.03% inhibition of Cu2+-induced Aβ1–42 aggregation; 2b, 54.13% inhibition; 3i, 45.81% inhibition; 3j, 43.17% inhibition; Cur, 61.35% inhibition; Res, 66.13% inhibition). These results suggested that these four compounds could inhibit Cu2+-induced Aβ aggregation by chelating Cu2+.
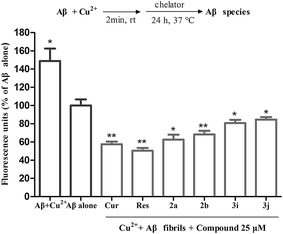 |
| Fig. 2 Inhibition of Cu2+-induced Aβ1–42 aggregation by compounds 2a, 2b, 3i and 3j. The final concentration of Aβ1–42, Cu2+ and test compounds were 25 µM. Values are reported as the mean ± SD of three independent experiments.*p < 0.05, **p < 0.01 vs. Aβ alone group. | |
2.2.4. Metal chelating effect of compound 2a.
Cu2+-induced Aβ1–42 aggregation assays showed that four compounds 2a, 2b, 3i and 3j inhibited Aβ aggregation by chelating Cu2+. Since compound 2a exhibited the most potency, it was chosen to study its metal chelating effect. The chelating effect of compounds 2a for metals such as Cu2+ and Al3+ in methanol was studied by UV-vis spectrometry with wavelength ranging from 200 to 500 nm.36 In Fig. 3a, UV-vis spectra of compound 2a with increasing Cu2+ concentrations from 2 to 50 µM was shown as an example. The increase in absorbance, which could be clearly estimated by an inspection of the differential spectra (Fig. 3b), indicated that there was an interaction between Cu2+ and compound 2a. Similar behavior was also observed when using Al3+. These observations revealed that compound 2a could effectively chelate Cu2+ and Al3+ and could serve as a metal chelator in treating AD. The ratio of ligand/metal ion in the complex was investigated by mixing the fixed amount of metal ion with increasing ligand. The maximum intensity of difference spectra was reached at about 1
:
1 ratio, which was taken as an indication of the stoichiometry of the complex.
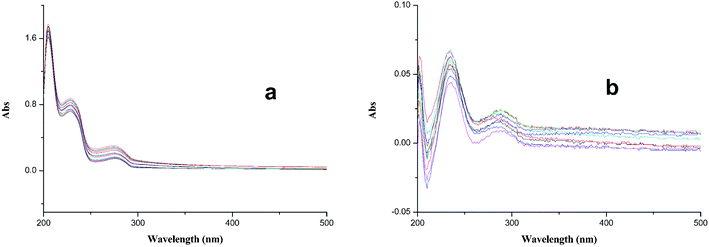 |
| Fig. 3 (a) UV-vis (200–500 nm) absorption spectra of compound 2a (25 µM in methanol) alone or in the presence of Cu2+ (2–50 µM in methanol); (b) the differential spectra due to 2a–Cu2+ complex formation obtained by numerical subtraction from the above spectra of those of Cu2+ and 2a at the corresponding concentrations. | |
2.2.5. Effect of compound 2a on Aβ-induced tau protein hyperphosphorylation.
Aβ-Induced tau protein hyperphosphorylation, a cardinal feature of AD, can induce destabilization of microtubules and eventual death of the neurons.37,38 Therefore, we tested the effect of compound 2a on Aβ-induced tau protein hyperphosphorylation by western blot analysis. As shown in Fig. 4, the phosphorylation of tau protein at Ser199/202 and Ser396 sites increased after treatment with 10 µM Aβ25–35 for 6 h. However, phosphorylation of tau protein was prevented by pretreatment with compound 2a (25 and 50 µM) for 30 min. Meanwhile, the total tau protein didn't change much after Aβ25–35 exposure with or without pretreatment with compound 2a, resulting in an attenuation of p-tau/total tau. Based on the results, we concluded that compound 2a could inhibit tau protein hyperphosphorylation, consequently resulting in a neuroprotective effect on Aβ-induced PC12 cells.
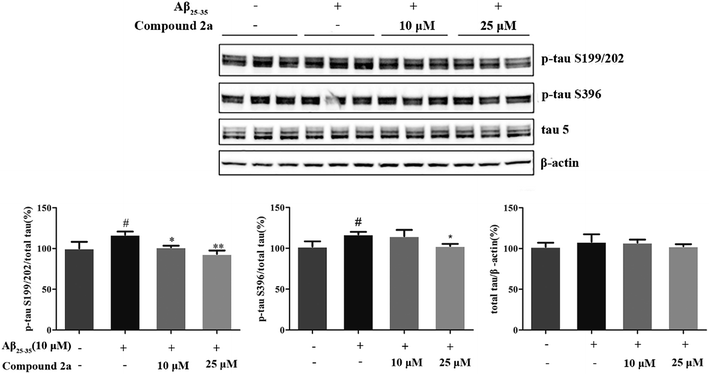 |
| Fig. 4 Compound 2a attenuated Aβ25–35-induced tau protein hyperphosphorylation in PC12 cells. PC12 cells were pretreated with compound 2a (10 and 25 µM) for 30 min, and then treated with Aβ25–35 (10 µM) for 6 h. Western blot analysis of phosphorylated tau protein (at Ser199/202 and Ser396 sites) and total tau was performed. Values are mean ± S.D. from triplicate independent experiments. #p < 0.05 vs. control; *p < 0.05, **p < 0.01 vs. Aβ25–35 treatment group. | |
2.2.6. Docking study of compound 2a with Aβ1–42.
To further study the interaction mode of compound 2a with Aβ1–42, molecular modeling calculations and docking studies were performed using Molecular Operating Environment (MOE) software version 2009.10 (Chemical Computing Group, Montreal, Canada). The X-ray crystal structure of Aβ1–42 (PDB 1IYT) used in the docking study was obtained from the Protein Data Bank (http://www.rcsb.org).13 In Fig. 5a showed the interaction conformation between 2a and Aβ1–42; (b) showed that the substituted benzene ring of compound 2a had a π–π interaction with Aβ (Tyr10) with the distance centroid of 2.27 Å and it could insert into the hydrophobic pocket (colored yellow, Glu3, His6, Asp7 and Tyr10). This docking simulation theoretically indicated the formation of Aβ/compound 2a complex by an effective π–π interaction.
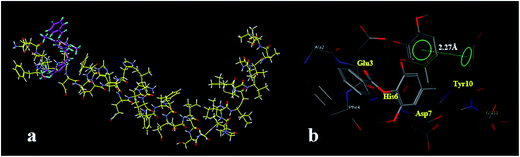 |
| Fig. 5 Docking results of compound 2a and Aβ1–42 (PDB code: 1IYT). (a) Cartoon representations of compound 2a (colored purple) interacting with Aβ1–42 (colored yellow); (b) association of compound 2a (colored grey) and Aβ1–42 obtained from docking calculations. The interactions between the ligand and residue Tyr10 are indicated by the green line. | |
2.2.7. Prediction of BBB penetration based on Lipinski's rules.
A major barrier to the development of effective anti-Aβ compounds for AD therapy is that essentially >98% of small-molecule compounds failed to cross the BBB.39,40 For drug-like properties, molecules should satisfy the terms of Lipinski's rules:41 molecular weight (MW) less than 500, the calculated logarithm of the octanol–water partition coefficient (C
log
P) less than 5, the number of hydrogen bond donor atoms (HBD) less than 5, the number of hydrogen bond acceptor atoms (HBA) less than 10 and the small polar surface area less than 90 Å2. Filter for prediction of BBB penetration involves calculation of log
BB by means of the equation shown in the footnote of Table 2 and compounds with log
BB higher than −1.0 could cross BBB.42 Defined by the terms of Lipinski's rules and calculated log
BB for potential applications in brains, compounds 2a, 2b, 3i and 3j fulfill drug-like criteria in general and could possibly penetrate the BBB better than CTX.
Table 2 Physical properties of compounds 2a, 2b, 3i, 3j and CTX
Compounds |
MWa |
C log Pa |
HBAa |
HBDa |
PSAa |
log BBa |
MW, molecular weight; C log P, calculated logarithm of the octanol–water partition coefficient; HBA, hydrogen-bond acceptor atoms; HBD, hydrogen-bond donor atoms; PSA, polar surface area; log BB = −0.0148 × PSA + 0.152 × C log P + 0.139.42
|
CTX
|
318 |
3.211 |
6 |
1 |
57.15 |
−0.218 |
2a
|
422 |
5.105 |
6 |
0 |
63.22 |
−0.021 |
2b
|
456 |
5.818 |
6 |
0 |
63.22 |
0.079 |
3i
|
386 |
5.018 |
5 |
0 |
46.15 |
0.210 |
3j
|
408 |
5.314 |
5 |
0 |
46.15 |
0.264 |
Rules |
≤500 |
≤5.0 |
≤10 |
≤5 |
≤90 |
≥−1.0 |
2.2.8.
In vitro blood-brain barrier permeability assay.
In order to verify the in vitro BBB permeability of the four active compounds, the parallel artificial membrane permeability assay of BBB (PAMPA-BBB) described by Di et al. was performed.43 This method could give reasonably good predictions on the passive BBB permeability of commercial centrally active drugs except for compounds which involved active uptake/efflux transport or were heavily metabolized in vivo. First, we compared the permeability of 10 commercial drugs with reported values to validate the assay (shown in Table 3). A plot of the experimental data versus the reported values produced a good linear correlation: Pe(exp.) = 1.054Pe(bibl.) + 0.5582 (R2 = 0.987) (see the ESI, Fig. S2†). From this equation and considering the limit established by Di et al. for BBB permeation, we classified compounds as follows: compounds with Pe (10−6 cm s−1) > 4.8 for high BBB permeation (CNS+), compounds with Pe (10−6 cm s−1) < 2.7 for low BBB permeation (CNS−) and compounds with 2.7 < Pe (10−6 cm s−1) < 4.8 for uncertain BBB permeation (CNS±). Finally, compounds 2a, 2b, 3i, 3j and CTX were tested through PAMPA-BBB (summarized in Table 3). Compounds 2a, 2b, 3i and 3j showed higher Pe value than 4.8, which indicated that they were able to cross the BBB and may reach the therapeutic targets in the CNS.
Table 3 Permeability (Pe, 10−6 cm s−1) of 10 commercial drugs and active compounds in the PAMPA-BBB assay
Commercial drugs |
Bibliographya |
Peb (10−6 cm s−1) |
Compounds |
Peb (10−6 cm s−1) |
Data obtained from ref. 43.
Experimental data are the mean ± SD of 3 independent experiments, with PBS : EtOH (70 : 30) as solvent.
|
Chlorpromazine |
6.5 |
7.1 ± 0.9 |
CTX
|
4.1 ± 0.5(CNS±) |
Clonidine |
5.3 |
6.5 ± 1.5 |
2a
|
6.9 ± 0.7(CNS+) |
Desipramine |
12.0 |
14.8 ± 3.3 |
2b
|
6.2 ± 0.5(CNS+) |
Lomefloxacin |
1.1 |
0.9 ± 0.4 |
3i
|
7.6 ± 1.1(CNS+) |
Progesterone |
9.3 |
10.5 ± 1.8 |
3j
|
8.2 ± 0.9(CNS+) |
Dopamine |
0.2 |
0.3 ± 0.1 |
— |
— |
Ofloxacin |
0.8 |
1.5 ± 0.5 |
— |
— |
Piroxacin |
2.5 |
3.9 ± 1.1 |
— |
— |
Testosterone |
17.0 |
18.1 ± 1.9 |
— |
— |
Verapamil |
16.0 |
16.5 ± 1.7 |
— |
— |
3. Conclusion
In summary, a series of 4-O-substituted chrysotoxine (CTX) derivatives were designed, synthesized and evaluated as multifunctional agents for the treatment of Alzheimer's disease (AD). Among the four ring substituted compounds (2a, 2b, 3i and 3j) with good neuroprotective activities, compound 2a exhibited significant inhibition of self- and Cu2+-induced Aβ1–42 aggregation and metal-chelating ability. Moreover, compound 2a was also capable of crossing the BBB in a parallel artificial membrane permeability assay. Furthermore, the result of western blot analysis showed that compound 2a was able to reduce Aβ-induced tau protein hyperphosphorylation. Taken together, these results suggest that compound 2a is a promising lead compound for multifunctional AD treatment. Further investigations of AD therapeutic candidates based on these results are in progress.
4. Experimental
4.1. Chemistry
All chemicals (reagent grade) used were purchased from Aladdin Shanghai Biochemical Technology Co., Ltd. or Sinopharm Chemical Reagent Co., Ltd. (China). Reaction progress was monitored using analytical thin layer chromatography (TLC) on precoated silica gel GF254 (Qingdao Haiyang Chemical Plant, Qingdao, China) plates and the spots were detected under UV light (λ = 254 nm). IR spectra were recorded on a Nicolet iS10 spectrophotometer using KBr disks. Melting point was measured on an XT-4 micro melting point apparatus and uncorrected.
1H and 13C NMR spectra were measured on a Bruker 300 MHz spectrometer at 298 T and referenced to TMS. Chemical shifts were reported in ppm using the residual solvent line as internal standard. Splitting patterns were designed as s (singlet), d (doublet), t (triplet), m (multiplet).
Mass spectra were obtained on an MS Agilent 1100 Series LC/MSD Trap mass spectrometer (ESI-MS). A Shimadzu LC-2010 series HPLC system (Kyoto, Japan) was used for the purity detection. Samples were separated on a YMC-Pack ODS-A column (50 mm × 4.6 mm, 3 µm, YMC Co. Ltd., Kyoto, Japan) with the column temperature set at 30 °C. The mobile phase consisted of acetonitrile–water (90
:
10, v/v), delivered at a flow rate of 1 mL min−1. The injection volume was 10 µL. Column chromatography was performed on silica gel (200–300 mesh; Qingdao Marine Chemical Inc.).
4.1.1. Synthetic intermediates.
4.1.1.1. 4-Formyl-2,6-dimethoxyphenyl acetate (3).
Syringaldazine (8.33 g, 0.046 mol) and catalyst DMAP were dissolved in DCM (10 mL), then acetic anhydride (4.75 mL, 0.051 mol) was slowly added. After the mixture was stirred at room temperature for 1 h, the solution was washed with diluted water (10 mL × 3). Organic layer was dried over anhydrous Na2SO4 and concentrated to get white crystals 10.19 g, 99.8% yield; mp 118–119 °C; IR vmax (KBr, cm−1): 3099, 2940, 1759, 1691, 1603, 1499; 1H-NMR (300 MHz, CDCl3) δ 9.93 (1H, s, –CHO), 7.17 (2H, s, H-3, H-5), 3.92 (6H, s, 2 × –OCH3), 2.38 (3H, s, –COCH3); 13C-NMR (75 MHz, CDCl3) δ 191.1, 168.1, 152.8, 134.3, 115.0, 106.0, 56.3, 20.4; ESI-MS m/z: 225.1 [M + H]+.
4.1.1.2. 4-(Hydroxymethyl)-2,6-dimethoxyphenyl acetate (4).
To a solution of 3 (8.6 g, 0.038 mol) in EtOH (10 mL), NaBH4 (0.53 g, 0.014 mol) was slowly added under ice bath. After stirred at room temperature for 30 min, 5% HCl was added to adjust pH to 7. Concentrated under vacuum, residue was extracted with DCM (10 mL × 3), then organic layer was dried over anhydrous Na2SO4 and concentrated to get white solid 8.34 g, 96.5% yield; mp 98–99 °C; IR vmax (KBr, cm−1): 3530, 3126, 2944, 1748, 1605, 1508; 1H-NMR (300 MHz, CDCl3) δ 6.66 (1H, s, H-3, H-5), 4.69 (2H, s, Ar-CH2–), 3.84 (6H, s, 2 × –OCH3), 2.36 (3H, s, –COCH3); 13C-NMR (75 MHz, CDCl3) δ 168.8, 152.1, 139.6, 115.3, 103.1, 65.2, 56.1, 20.5; ESI-MS m/z: 227.1 [M + H]+.
4.1.1.3. 4-(Chloromethyl)-2,6-dimethoxyphenyl acetate (5).
To a solution of 4 (8.3 g, 0.037 mol) in DCM (10 mL), Et3N (6.2 mL, 0.043 mol), SOCl2 (3.0 mL, 0.043 mol) was slowly dropped under ice salt bath. After stirred at room temperature for 3 h, the solution was washed with diluted water (10 mL × 3). Organic layer was dried over anhydrous Na2SO4 and concentrated to get light yellow solid 7.96 g, 88.7% yield; mp 75–76 °C; IR vmax (KBr, cm−1): 3162, 2971, 1759, 1606, 1508; 1H-NMR (300 MHz, CDCl3) δ 6.85 (1H, s, H-3, H-5), 4.73 (2H, s, Ar-CH2–), 3.76 (6H, s, 2 × –OCH3), 2.24 (3H, s, –COCH3); 13C-NMR (75 MHz, CDCl3) δ 168.3, 152.0, 139.4, 115.0, 103.2, 60.7, 56.1, 20.4; ESI-MS m/z: 227.1 [M + H]+.
4.1.1.4. (4-Acetoxy-3,5-dimethoxybenzyl)triphenylphosphonium chloride (6).
5 (7.8 g, 0.032 mol) and PhP3 (8.4 g, 0.032 mol) were dissolved in toluene (5 mL) under reflux for 6 h. After cooled to temperature, the mixture was filtrated and washed with toluene under vacuum. Dried under infrared light and 14.63 g white powder was obtained, 90.2% yield; 1H-NMR (300 MHz, DMSO-d6) δ 7.92 (3H, m, ArH), 7.76 (6H, dt, J = 3.6 Hz, 8.1 Hz, ArH), 7.69 (6H, m, ArH), 6.30 (2H, d, J = 2.4 Hz, H-3, H-5), 5.09 (2H, J = 15 Hz, Ar-CH2–), 3.37 (6H, s, 2 × –OCH3), 2.21 (3H, s, × –OCH3); 13C-NMR (75 MHz, DMSO-d6) δ 168.4, 152.0, 151.9, 135.5, 134.8, 134.6, 130.6, 130.4, 126.9, 126.7, 118.8, 117.6, 108.5, 108.4, 56.2, 29.4, 28.8, 20.6; ESI-MS m/z: 541.2 [M + Cl]−.
4.1.1.5. 4-[(Z/E)-3,4-Dimethoxystyryl]-2,6-dimethoxyphenyl acetate (7).
6 (3.0 g, 5.920 mmol) and 3,4-dimethoxybenzaldehyde were dissolved in anhydrous THF (3 mL) under Ar protection. NaH (0.15 g, 6.25 mmol) was added slowly under ice salt bath. After overnight reaction in room temperature, the solution was concentrated. Then the mixture of trans- and cis-stilbenes was recrystallized in methanol with 70.4% yield. trans- and cis-Stilbenes could be separated by fast chromatography, and the ratio of trans- and cis- was 3
:
1. 4-[(Z)-3,4-Dimethoxystyryl]-2,6-dimethoxyphenyl acetate: 1H-NMR (300 MHz, CDCl3) δ 7.06 (3H, m, ArH), 6.97 (1H, d, J = 8.9 Hz, –CH
CH–), 6.89 (1H, d, J = 8.9 Hz, –CH
CH–), 6.76 (2H, s, H-3, 5), 3.97 (3H, s, –OCH3), 3.93 (3H, s, –OCH3), 3.90 (6H, s, 2 × –OCH3), 2.37 (3H, s, –COCH3); 13C-NMR (75 MHz, CDCl3) δ 168.7, 151.9, 148.3, 135.8, 130.4, 129.5, 128.5, 122.1, 111.6, 110.7, 106.0, 105.4, 56.0, 55.8, 55.7, 20.5; ESI-MS m/z: 359.1 [M + H]+; 4-[(E)-3,4-dimethoxystyryl]-2,6-dimethoxyphenyl acetate: 1H-NMR (300 MHz, CDCl3) δ 6.87 (1H, dd, J = 1.8, 8.1 Hz, H-6′), 6.84 (1H, d, J = 1.8 Hz, H-2′), 6.78 (1H, d, J = 8.1 Hz, H-5′), 6.57 (2H, s, H-3, 5), 6.54 (1H, d, J = 12.0 Hz, –CH
CH–), 6.49 (1H, d, J = 12.0 Hz, –CH
CH–), 3.88 (3H, s, –OCH3), 3.69 (6H, s, 2 × –OCH3), 3.68 (3H, s, –OCH3), 2.34 (3H, s, –COCH3); 13C-NMR (75 MHz, CDCl3) δ 168.9, 152.2, 149.1, 149.0, 136.0, 130.1, 128.8, 126.6, 122.0, 111.2, 108.7, 102.9, 56.1, 56.0, 55.9, 20.5; ESI-MS m/z: 359.1 [M + H]+.
4.1.2. 4-(3,4-Dimethoxyphenethyl)-2,6-dimethoxyphenyl acetate (CTX-0).
The mixture of stilbenes (1 g, 2.79 mmol) was reduced by treatment with catalytic amounts of 5% Pd–C over H2 (4 atm) in ethanol (5 mL) to quantitatively afford 8 with bibenzyl skeleton. White crystal, mp 124–125 °C; IR vmax (KBr, cm−1): 3422, 2924, 1760, 1603, 1517; 1H-NMR (300 MHz, CDCl3) δ 6.82 (1H, d, J = 8.1 Hz, H-5′), 6.75 (1H, dd, J = 1.8, 8.1 Hz, H-6′), 6.67 (1H, d, J = 1.8 Hz, H-2′), 6.41 (2H, s, H-3, 5), 3.88 (3H, s, –OCH3), 3.86 (3H, s, –OCH3), 3.80 (6H, s, 2 × –OCH3), 2.88 (4H, s, –CH2CH2–), 2.86 (3H, s, –COCH3); 13C-NMR (75 MHz, CDCl3) δ 168.9, 151.7, 148.6, 147.2, 141.2, 140.1, 134.0, 120.2, 111.8, 111.1, 105.0, 56.0, 55.8, 55.7, 38.6, 37.4, 20.4; ESI-MS m/z: 361.1 [M + H]+; HPLC purity: 99%, tR = 2.13 min.
4.1.3. Chrysotoxine (CTX).
CTX-0 (1 g, 2.78 mmol) was first dissolved in methanol (5 mL), and then catalytic amount of MeONa was added and stirred for 30 min in the room temperature. 5% HCl was added to adjust pH = 7. After concentration under vacuum, the residue was extracted with DCM (10 mL × 3), dried with Na2SO4 and then purified by fast chromatography (PE/acetone 5
:
1) to get a light yellow solid 0.88 g, 99.8% yield. Mp 98–99 °C; IR vmax (KBr, cm−1): 3462, 3133, 2933, 1609, 1517; 1H-NMR (300 MHz, CDCl3) δ 6.81 (1H, d, J = 8.1 Hz, H-5′), 6.72 (1H, dd, J = 1.8, 8.1 Hz, H-6′), 6.68 (1H, d, J = 1.8 Hz, H-2′), 6.38 (2H, s, H-3, 5), 5.39 (1H, s, –OH), 3.88 (6H, s, 2 × –OCH3), 3.87 (6H, s, 2 × –OCH3), 2.85 (4H, s, –CH2CH2–); 13C-NMR (75 MHz, CDCl3) δ 146.9, 146.3, 140.4, 133.8, 132.3, 127.8, 119.9, 111.4, 110.7, 104.6, 55.7, 55.4, 55.3, 37.9, 37.3; ESI-MS m/z: 319.1 [M + H]+, 336.1 [M + NH4]+; HPLC purity: 100%, tR = 1.71 min.
4.1.4. General procedure for preparation of 4-O-alkyl substituted CTX ester derivatives (1a–1f).
To a mixture of CTX (30 mg, 0.094 mmol), EDC·HCl and catalytic amount of DMAP in DCM (5 mL), corresponding short-chain carboxylic acid was added. The reaction mixture was stirred for 12–20 h in room temperature. After complete reaction, the solvent was evaporated under reduced pressure. The residue was purified on a fast chromatography using the mixture of PE/EtOAc (20
:
1–8
:
1) as eluent, by which we obtained the corresponding 4-O-modified CTX derivatives.
4.1.4.1. 4-(3,4-Dimethoxyphenethyl)-2,6-dimethoxyphenyl propionate (1a).
Yield 63.7%; white solid, mp 101–102 °C; IR vmax (KBr, cm−1): 3422, 2922, 1758, 1604, 1515; 1H-NMR (300 MHz, CDCl3) δ 6.82 (1H, d, J = 8.1 Hz, H-5′), 6.74 (1H, dd, J = 1.8, 8.1 Hz, H-6′), 6.67 (1H, d, J = 1.8 Hz, H-2′), 6.40 (2H, s, H-3, 5), 3.88 (3H, s, –OCH3), 3.87 (3H, s, –OCH3), 3.79 (6H, s, 2 × –OCH3), 2.88 (4H, s, –CH2CH2–), 2.66 (2H, q, J = 7.8 Hz, –COCH2–), 1.29 (3H, t, J = 7.5 Hz, –CH3); 13C-NMR (75 MHz, CDCl3) δ 172.0, 151.4, 148.3, 146.8, 139.6, 133.7, 119.8, 111.4, 110.7, 104.7, 55.6, 55.5, 55.3, 38.2, 37.0, 26.7, 8.8; ESI-MS m/z: 392.3 [M + NH4]+, 375.2 [M + H]+; HPLC purity: 100%, tR = 2.22 min.
4.1.4.2. 4-(3,4-Dimethoxyphenethyl)-2,6-dimethoxyphenyl butyrate (1b).
Yield 42.1%; yellow powder; mp 60–61 °C; IR vmax (KBr, cm−1): 3446, 2953, 1758, 1603, 1508; 1H-NMR (300 MHz, CDCl3) δ 6.82 (1H, d, J = 8.1 Hz, H-5′), 6.74 (1H, dd, J = 1.8, 8.1 Hz, H-6′), 6.67 (1H, d, J = 1.8 Hz, H-2′), 6.40 (2H, s, H-3, 5), 3.88 (3H, s, –OCH3), 3.86 (3H, s, –OCH3), 3.79 (6H, s, 2 × –OCH3), 2.88 (4H, s, –CH2CH2–), 2.61 (2H, t, J = 7.2 Hz, –COCH2–), 1.82 (2H, m, J = 7.2 Hz, –CH2–), 1.07 (3H, t, J = 7.2 Hz, –CH3); 13C-NMR (75 MHz, CDCl3) δ 171.1, 151.4, 148.5, 139.6, 133.7, 119.8, 114.5, 111.4, 110.7, 104.7, 55.6, 55.5, 55.3, 38.2, 37.0, 35.3, 18.2, 13.1; ESI-MS m/z: 406.3 [M + NH4]+, 389.2 [M + H]+; HPLC purity: 98%, tR = 2.44 min.
4.1.4.3. 4-(3,4-Dimethoxyphenethyl)-2,6-dimethoxyphenyl isobutyrate (1c).
Yield 49.2%; yellow powder, mp 80–81 °C; IR vmax (KBr, cm−1): 3433, 2932, 1760, 1601, 1509, 1117; 1H-NMR (300 MHz, CDCl3) δ 6.81 (1H, d, J = 8.1 Hz, H-5′), 6.74 (1H, dd, J = 1.8, 8.1 Hz, H-6′), 6.67 (1H, d, J = 1.8 Hz, H-2′), 6.40 (2H, s, H-3, 5), 3.88 (3H, s, –OCH3), 3.87 (3H, s, –OCH3), 3.78 (6H, s, 2 × –OCH3), 2.89 (1H, m, –COCH–) 2.88 (4H, s, –CH2CH2–), 1.34 (6H, d, J = 6.9 Hz, 2 × –CH3); 13C-NMR (75 MHz, CDCl3) δ 174.7, 151.4, 148.5, 146.8, 139.4, 133.7, 119.8, 114.5, 111.4, 110.7, 104.8, 55.6, 55.5, 55.3, 38.2, 37.0, 33.4, 18.6; ESI-MS m/z: 406.3 [M + NH4]+, 389.2 [M + H]+; HPLC purity: 100%, tR = 2.47 min.
4.1.4.4. 4-(3,4-Dimethoxyphenethyl)-2,6-dimethoxyphenyl pentanoate (1d).
Yield 63.3%; white crystal; mp 77–78 °C; IR vmax (KBr, cm−1): 3448, 2956, 1741, 1601, 1509, 1128; 1H-NMR (300 MHz, CDCl3) δ 6.82 (1H, d, J = 8.1 Hz, H-5′), 6.74 (1H, dd, J = 1.8, 8.1 Hz, H-6′), 6.67 (1H, d, J = 1.8 Hz, H-2′), 6.40 (2H, s, H-3, 5), 3.88 (3H, s, –OCH3), 3.86 (3H, s, –OCH3), 3.78 (6H, s, 2 × –OCH3), 2.88 (4H, s, –CH2CH2–), 2.62 (2H, t, J = 7.2 Hz, –COCH2–), 1.78 (2H, m, J = 7.2 Hz, –CH2–), 1.48 (2H, m, –CH2–), 0.98 (3H, t, J = 7.2 Hz, –CH3); 13C-NMR (75 MHz, CDCl3) δ 181.1, 151.4, 148.3, 146.8, 139.6, 134.9, 119.8, 111.4, 110.7, 104.7, 55.6, 55.5, 55.3, 38.2, 37.0, 33.1, 26.7, 21.7, 13.3; ESI-MS m/z: 420.3 [M + NH4]+, 403.2 [M + H]+; HPLC purity: 100%, tR = 2.70 min.
4.1.4.5. 4-(3,4-Dimethoxyphenethyl)-2,6-dimethoxyphenyl 3-methylbutanoate (1e).
Yield 60.6%; white crystal; mp 102–103 °C; IR vmax (KBr, cm−1): 3422, 2923, 1758, 1607, 1506, 1124; 1H-NMR (300 MHz, CDCl3) δ 6.82 (1H, d, J = 8.1 Hz, H-5′), 6.74 (1H, dd, J = 1.8, 8.1 Hz, H-6′), 6.67 (1H, d, J = 1.8 Hz, H-2′), 6.40 (2H, s, H-3, 5), 3.88 (3H, s, –OCH3), 3.86 (3H, s, –OCH3), 3.78 (6H, s, 2 × –OCH3), 2.88 (4H, s, –CH2CH2–), 2.49 (2H, d, J = 7.2 Hz, –COCH2–), 2.29 (1H, m, –CH–), 1.09 (6H, d, J = 6.6 Hz, 2 × –CH3); 13C-NMR (75 MHz, CDCl3) δ 170.5, 151.4, 148.2, 146.8, 139.6, 133.65, 119.8, 111.4, 110.7, 104.7, 55.5, 55.4, 55.3, 42.5, 38.2, 37.0, 25.6, 21.8; ESI-MS m/z: 420.3 [M + NH4]+, 403.2 [M + H]+; HPLC purity: 100%, tR = 2.69 min.
4.1.4.6. 4-(3,4-Dimethoxyphenethyl)-2,6-dimethoxyphenyl 2-propylpentanoate (1f).
Yield 48.7%; white crystal; mp 63–64 °C; IR vmax (KBr, cm−1): 3417, 2914, 1758, 1607, 1513, 1129; 1H-NMR (300 MHz, CDCl3) δ 6.81 (1H, d, J = 8.1 Hz, H-5′), 6.73 (1H, dd, J = 1.8, 8.1 Hz, H-6′), 6.66 (1H, d, J = 1.8 Hz, H-2′), 6.38 (2H, s, H-3, 5), 3.88 (3H, s, –OCH3), 3.86 (3H, s, –OCH3), 3.76 (6H, s, 2 × –OCH3), 2.87 (4H, s, –CH2CH2–), 2.67 (1H, m, –COCH–), 1.80 (2H, m, –CH2), 1.56 (2H, m, –CH2), 1.52 (4H, m, 2 × –CH2–), 0.97 (6H, t, J = 8.1 Hz, 2 × –CH3); 13C-NMR (75 MHz, CDCl3) δ 173.8, 151.4, 148.3, 146.8, 139.5, 133.7, 119.8, 111.4, 110.7, 104.7, 55.5, 55.4, 55.3, 44.8, 38.2, 37.0, 34.4, 19.9, 13.6; ESI-MS m/z: 462.3 [M + NH4]+, 445.2 [M + H]+; HPLC purity: 96%, tR = 2.02 min.
4.1.5. General procedure for preparation of 4-O-cyclicsubstituted CTX ester derivatives (2a–2e).
Corresponding cyclic-substituted acid was reacted in SOCl2 (3 mL) under reflux for 2 h. After concentration under vacuum, chloride powder was obtained for the next procedure. To a mixture of CTX (30 mg, 0.094 mmol) and corresponding chloride in acetone (5 mL), Et3N (200 µL) was dropped slowly under ice bath. The solution refluxed for 4 h and then was evaporated under reduced pressure. Water was added in the residue, and DCM (10 mL × 3) was used for extract. Organic layer was dried with Na2SO4 and then was evaporated to get the crude target. Silica gel chromatography was applied for purification with elution of PE/EtOAc (20
:
1–3
:
1).
4.1.5.1. 4-(3,4-Dimethoxyphenethyl)-2,6-dimethoxyphenyl benzoate (2a).
Yield 90.3%; white crystal; mp 136–137 °C; IR vmax (KBr, cm−1): 3448, 2935, 1741, 1601, 1509, 1130; 1H-NMR (300 MHz, CDCl3) δ 8.26, 7.61, 7.52 (5H, m, ArH), 6.83 (1H, d, J = 8.1 Hz, H-5′), 6.76 (1H, dd, J = 1.8, 8.1 Hz, H-6′), 6.70 (1H, d, J = 1.8 Hz, H-2′), 6.46 (2H, s, H-3, 5), 3.89 (6H, s, 2 × –OCH3), 3.79 (6H, s, 2 × –OCH3), 2.91 (4H, s, –CH2CH2–); 13C-NMR (75 MHz, CDCl3) δ 184.1, 151.6, 148.2, 145.7, 139.8, 133.7, 132.8, 131.2, 129.9, 127.9, 119.8, 111.4, 110.7, 104.8, 55.7, 55.5, 55.4, 38.3, 37.1; ESI-MS m/z: 440.3 [M + NH4]+, 423.2 [M + H]+; HPLC purity: 99%, tR = 2.53 min.
4.1.5.2. 4-(3,4-Dimethoxyphenethyl)-2,6-dimethoxyphenyl 4-chlorobenzoate (2b).
Yield 87.2%; light yellow powder; mp 130–131 °C; IR vmax (KBr, cm−1): 3447, 2933, 1743, 1601, 1508, 1127; 1H-NMR (300 MHz, CDCl3) δ 8.19 (2H, d, J = 8.4 Hz, ArH), 7.49 (2H, d, J = 8.4 Hz, ArH), 6.83 (1H, d, J = 8.1 Hz, H-5′), 6.76 (1H, dd, J = 1.8, 8.1 Hz, H-6′), 6.70 (1H, d, J = 1.8 Hz, H-2′), 6.45 (2H, s, H-3, 5), 3.91 (6H, s, 2 × –OCH3), 3.82 (6H, s, 2 × –OCH3), 2.91 (4H, s, –CH2CH2–); 13C-NMR (75 MHz, CDCl3) δ 187.3, 151.5, 149.5, 147.0, 140.0, 135.9, 133.7, 131.3, 128.4, 128.3, 119.8, 111.4, 110.7, 104.7, 55.6, 55.5, 55.4, 42.8, 38.3, 37.0, 33.5; ESI-MS m/z: 474.2 [M + NH4]+, 457.1 [M + H]+; HPLC purity: 95%, tR = 3.06 min.
4.1.5.3. 4-(3,4-Dimethoxyphenethyl)-2,6-dimethoxyphenyl 3-phenylpropanoate (2c).
Yield 85.7%; light yellow powder; mp 65–66 °C; IR vmax (KBr, cm−1): 3423, 2923, 1759, 1605, 1508, 1127; 1H-NMR (300 MHz, CDCl3) δ 7.31 (5H, m, ArH), 6.82 (1H, d, J = 8.1 Hz, H-5′), 6.74 (1H, dd, J = 1.8, 8.1 Hz, H-6′), 6.67 (1H, d, J = 1.8 Hz, H-2′), 6.40 (2H, s, H-3, 5), 3.88 (3H, s, –OCH3), 3.86 (3H, s, –OCH3), 3.76 (6H, s, 2 × –OCH3), 3.12 (2H, t, J = 6.9 Hz, –CH2–), 2.95 (2H, t, J = 6.9 Hz, –CH2–), 2.87 (4H, s, –CH2CH2–); 13C-NMR (75 MHz, CDCl3) δ 170.4, 151.3, 148.5, 146.8, 140.0, 139.7, 133.6, 128.0, 127.9, 125.7, 119.8, 111.4, 110.7, 104.7, 55.6, 55.5, 55.3, 38.3, 37.0, 34.9, 30.5; ESI-MS m/z: 468.2 [M + NH4]+, 451.1 [M + H]+; HPLC purity: 99%, tR = 2.66 min.
4.1.5.4. 4-(3,4-Dimethoxyphenethyl)-2,6-dimethoxyphenyl picolinate (2d).
Yield 72.7%; yellow powder; mp 132–133 °C; IR vmax (KBr, cm−1): 3131, 2944, 1757, 1601, 1514; 1H-NMR (300 MHz, CDCl3) δ 8.89, 8.32, 7.95, 7.57 (4H, pyridine), 6.83 (1H, d, J = 8.1 Hz, H-5′), 6.75 (1H, dd, J = 1.8, 8.1 Hz, H-6′), 6.69 (1H, d, J = 1.8 Hz, H-2′), 6.44 (2H, s, H-3, 5), 3.89 (3H, s, –OCH3), 3.88 (3H, s, –OCH3), 3.78 (6H, s, 2 × –OCH3), 2.91 (4H, s, –CH2CH2–); 13C-NMR (75 MHz, CDCl3) δ 180.1, 151.9, 150.1, 148.9, 146.0, 140.4, 137.0, 134.2, 127.2, 125.9, 120.3, 111.9, 111.2, 105.1, 56.1, 55.9, 55.8, 38.8, 37.5; ESI-MS m/z: 424.1 [M + H]+; HPLC purity: 99%, tR = 2.00 min.
4.1.5.5. 4-(3,4-Dimethoxyphenethyl)-2,6-dimethoxyphenyl isonicotinate (2e).
Yield 87.7%; white crystal; mp 118–119 °C; IR vmax (KBr, cm−1): 3131, 2932, 1735, 1601, 1514; 1H-NMR (300 MHz, CDCl3) δ 8.86 (2H, d, J = 5.7 Hz, pyridine), 8.06 (2H, d, J = 5.7 Hz, pyridine), 6.83 (1H, d, J = 8.1 Hz, H-5′), 6.75 (1H, dd, J = 1.8, 8.1 Hz, H-6′), 6.69 (1H, d, J = 1.8 Hz, H-2′), 6.45 (2H, s, H-3, 5), 3.89 (3H, s, –OCH3), 3.88 (3H, s, –OCH3), 3.78 (6H, s, 2 × –OCH3), 2.91 (4H, s, –CH2CH2–); 13C-NMR (75 MHz, CDCl3) δ 181.5, 155.4, 151.8, 150.6, 141.1, 136.8, 134.1, 123.7, 120.3, 111.9, 111.2, 105.2, 56.1, 56.0, 55.9, 38.8, 37.5; ESI-MS m/z: 424.1 [M + H]+, 406.1 [M − OH]+; HPLC purity: 98%, tR = 2.42 min.
4.1.6. General procedure for preparation of 4-O-alkyl and 4-O-cyclic substituted CTX ether derivatives (3a–3j).
CTX (30 mg, 0.094 mmol) and corresponding bromo-substituted hydrocarbons were under reflux in DMF (3 mL) for 6 h. To the solution EtOAc (30 mL) was added, and water was used to wash DMF for 5 times. The organic layer was dried with Na2SO4 and the solvent was removed under reduced pressure. Silica gel chromatography was applied for purification with elution of PE/EtOAc (20
:
1–10
:
1).
4.1.6.1. 5-(3,4-Dimethoxyphenethyl)-2-ethoxy-1,3-dimethoxybenzene (3a).
Yield 52.9%; yellow crystal, mp 68–69 °C; IR vmax (KBr, cm−1): 3132, 2957, 1589, 1515; 1H-NMR (300 MHz, CDCl3) δ 6.82 (1H, d, J = 8.1 Hz, H-5′), 6.74 (1H, d, J = 1.8, 8.1 Hz, H-6′), 6.68 (1H, d, J = 1.8 Hz, H-2′), 6.38 (2H, s, H-3, 5), 4.04 (2H, q, J = 7.2 Hz, –OCH2–), 3.88 (3H, s, –OCH3), 3.86 (3H, s, –OCH3), 3.83 (6H, s, 2 × –OCH3), 2.87 (4H, s, –CH2CH2–), 1.37 (3H, t, J = 7.2 Hz, –CH3); 13C-NMR (75 MHz, CDCl3) δ 153.3, 148.7, 146.9, 137.2, 134.3, 120.3, 115.1, 111.9, 111.1, 105.6, 74.2, 56.1, 55.9, 55.8, 38.6, 37.6, 31.1; ESI-MS m/z: 347 [M + H]+, HPLC purity: 99%, tR = 2.38 min.
4.1.6.2. 5-(3,4-Dimethoxyphenethyl)-1,3-dimethoxy-2-propoxybenzene (3b).
Yield 61.8%; light yellow oil; IR vmax (KBr, cm−1): 3000, 2958, 2935, 1589, 1513; 1H-NMR (300 MHz, CDCl3) δ 6.81 (1H, d, J = 8.1 Hz, H-5′), 6.73 (1H, d, J = 1.8, 8.1 Hz, H-6′), 6.68 (1H, d, J = 1.8 Hz, H-2′), 6.38 (2H, s, H-3, 5), 3.92 (2H, t, J = 6.9 Hz, –OCH2–), 3.88 (3H, s, –OCH3), 3.86 (3H, s, –OCH3), 3.82 (6H, s, 2 × –OCH3), 2.86 (4H, s, –CH2CH2–), 1.78 (2H, m, –CH2–), 1.01 (3H, t, J = 7.5 Hz, –CH3); 13C-NMR (75 MHz, CDCl3) δ 153.3, 148.7, 146.4, 137.2, 134.3, 120.3, 115.1, 111.9, 111.1, 105.6, 75.1, 56.1, 55.9, 55.8, 38.6, 37.6, 23.3, 10.4; ESI-MS m/z: 378 [M + NH4]+, 361 [M + H]+; HPLC purity: 97%, tR = 2.70 min.
4.1.6.3. 2-Butoxy-5-(3,4-dimethoxyphenethyl)-1,3-dimethoxybenzene (3c).
Yield 65.2%; yellow oil; IR vmax (KBr, cm−1): 3132, 2955, 1581, 1514; 1H-NMR (300 MHz, CDCl3) δ 6.81 (1H, d, J = 8.1 Hz, H-5′), 6.73 (1H, d, J = 1.8, 8.1 Hz, H-6′), 6.68 (1H, d, J = 1.8 Hz, H-2′), 6.37 (2H, s, H-3, 5), 3.95 (2H, t, J = 6.9 Hz, –OCH2–), 3.88 (3H, s, –OCH3), 3.86 (3H, s, –OCH3), 3.82 (6H, s, 2 × –OCH3), 2.86 (4H, s, –CH2CH2–), 1.76 (2H, m, –CH2–), 1.49 (2H, m, –CH2–), 0.97 (3H, t, J = 6.5 Hz, –CH3); 13C-NMR (75 MHz, CDCl3) δ 153.3, 148.7, 147.2, 137.2, 134.3, 120.3, 111.9, 111.2, 105.6, 73.2, 56.1, 56.0, 55.8, 38.6, 37.6, 32.2, 19.1, 13.9; ESI-MS m/z: 392.2 [M + NH4]+, 375.2 [M + H]+; HPLC purity: 99%, tR = 3.06 min.
4.1.6.4. 5-(3,4-Dimethoxyphenethyl)-2-isobutoxy-1,3-dimethoxybenzene (3d).
Yield 49.8%; yellow oil; IR vmax (KBr, cm−1): 3132, 2935, 1588, 1514; 1H-NMR (300 MHz, CDCl3) δ 6.81 (1H, d, J = 8.1 Hz, H-5′), 6.73 (1H, d, J = 1.8, 8.1 Hz, H-6′), 6.68 (1H, d, J = 1.8 Hz, H-2′), 6.37 (2H, s, H-3, 5), 3.88 (3H, s, –OCH3), 3.86 (3H, s, –OCH3), 3.82 (6H, s, 2 × –OCH3), 3.71 (2H, d, J = 6.9 Hz, –OCH2–), 2.86 (4H, s, –CH2CH2–), 2.07 (1H, m, –CH–), 1.03 (6H, d, 2 × –CH3); 13C-NMR (75 MHz, CDCl3) δ 153.2, 148.8, 147.3, 137.1, 134.3, 120.3, 111.9, 111.1, 105.9, 80.2, 56.2, 56.0, 55.8, 38.5, 37.6, 29.0, 19.2; ESI-MS m/z: 392.2 [M + NH4]+, 375.2 [M + H]+; HPLC purity: 100%, tR = 3.21 min.
4.1.6.5. 2-(sec-Butoxy)-5-(3,4-dimethoxyphenethyl)-1,3-dimethoxybenzene (3e).
Yield 42.7%; yellow oil; IR vmax (KBr, cm−1): 3132, 2955, 1584, 1515; 1H-NMR (300 MHz, CDCl3) δ 6.81 (1H, d, J = 8.1 Hz, H-5′), 6.73 (1H, d, J = 1.8, 8.1 Hz, H-6′), 6.67 (1H, d, J = 1.8 Hz, H-2′), 6.37 (2H, s, H-3, 5), 4.11 (1H, m, –CH–), 3.88 (3H, s, –OCH3), 3.85 (3H, s, –OCH3), 3.80 (6H, s, 2 × –OCH3), 2.85 (4H, s, –CH2CH2–), 1.80–161 (2H, m, –OCH2–), 1.23 (3H, d, J = 6.3 Hz, –CH3), 0.99 (3H, t, J = 7.5 Hz, –CH3); 13C-NMR (75 MHz, CDCl3) δ 153.5, 148.7, 142.1, 136.9, 129.3, 120.3, 115.0, 105.6, 80.1, 56.0, 55.9, 55.8, 38.6, 37.6, 29.5, 19.5, 10.0; ESI-MS m/z: 392.2 [M + NH4]+, 375.2 [M + H]+; HPLC purity: 98%, tR = 3.06 min.
4.1.6.6. 5-(3,4-Dimethoxyphenethyl)-2-(isopentyloxy)-1,3-dimethoxybenzene (3f).
Yield 44.9%; yellow oil; IR vmax (KBr, cm−1): 3132, 2924, 1589, 1518; 1H-NMR (300 MHz, CDCl3) δ 6.81 (1H, d, J = 8.1 Hz, H-5′), 6.73 (1H, d, J = 1.8, 8.1 Hz, H-6′), 6.68 (1H, d, J = 1.8 Hz, H-2′), 6.37 (2H, s, H-3, 5), 3.97 (2H, t, J = 6.9 Hz, –OCH2–), 3.88 (3H, s, –OCH3), 3.86 (3H, s, –OCH3), 3.82 (6H, s, 2 × –OCH3), 2.86 (4H, s, –CH2CH2–), 1.89 (1H, m, –CH–), 1.67 (2H, t, J = 6.9 Hz, –CH2–), 0.96 (6H, d, J = 6.6 Hz, 2 × –CH3); 13C-NMR (75 MHz, CDCl3) δ 153.3, 148.7, 147.2, 137.2, 134.3, 120.3, 111.9, 111.1, 105.6, 71.9, 56.1, 55.9, 55.8, 38.9, 38.6, 37.6, 24.8, 22.6; ESI-MS m/z: 406.2 [M + NH4]+, 389.2 [M + H]+; HPLC purity: 100%, tR = 3.47 min.
4.1.6.7. 5-(3,4-Dimethoxyphenethyl)-1,3-dimethoxy-2-(pentan-2-yloxy)benzene (3g).
Yield 37.4%; yellow oil; IR vmax (KBr, cm−1): 3133, 2935, 1587, 1517; 1H-NMR (300 MHz, CDCl3) δ 6.81 (1H, d, J = 8.1 Hz, H-5′), 6.73 (1H, d, J = 1.8, 8.1 Hz, H-6′), 6.68 (1H, d, J = 1.8 Hz, H-2′), 6.36 (2H, s, H-3, 5), 4.19 (1H, m, –OCH–), 3.88 (3H, s, –OCH3), 3.85 (3H, s, –OCH3), 3.80 (6H, s, 2 × –OCH3), 2.86 (4H, brs, –CH2CH2–), 1.76, 1.51 (4H, m, –CH2CH2–), 1.22 (2H, t, J = 6.3 Hz, –CH2–), 0.95 (3H, t, J = 6.9 Hz, –CH3); 13C-NMR (75 MHz, CDCl3) δ 153.5, 148.6, 147.2, 136.9, 134.4, 120.3, 111.9, 111.1, 105.5, 78.5, 56.0, 55.9, 55.8, 39.1, 38.6, 37.6, 20.0, 18.8, 14.2; ESI-MS m/z: 406.2 [M + NH4]+, 389.2 [M + H]+; HPLC purity: 99%, tR = 3.53 min.
4.1.6.8. 5-(3,4-Dimethoxyphenethyl)-2-[(2-ethylhexyl)oxy]-1,3-dimethoxybenzene (3h).
Yield 39.4%; yellow oil; IR vmax (KBr, cm−1): 3133, 2958, 1637, 1513; 1H-NMR (300 MHz, CDCl3) δ 6.81 (1H, d, J = 8.1 Hz, H-5′), 6.72 (1H, dd, J = 1.8, 8.1 Hz, H-6′), 6.68 (1H, d, J = 1.8 Hz, H-2′), 6.36 (2H, s, H-3, 5), 3.88 (3H, s, –OCH3), 3.85 (3H, s, –OCH3), 3.82 (2H, d, J = 6.9 Hz, –OCH2–), 3.80 (6H, s, 2 × –OCH3), 2.86 (4H, brs, –CH2CH2–), 1.40–1.72 (9H, m), 0.95 (6H, t, J = 6.9 Hz, 2 × –CH3); 13C-NMR (75 MHz, CDCl3) δ 153.5, 149.6, 146.9, 137.2, 134.5, 120.3, 111.9, 111.1, 105.8, 75.8, 56.1, 55.9, 55.8, 40.2, 38.5, 37.6, 30.2, 29.0, 23.5, 23.2, 14.2, 10.9; ESI-MS m/z: 448.2 [M + NH4]+, 431.2 [M + H]+; HPLC purity: 98%, tR = 6.65 min.
4.1.6.9. 2-(Cyclopentyloxy)-5-(3,4-dimethoxyphenethyl)-1,3-dimethoxybenzene (3i).
Yield 25.8%; yellow crystal, 51–52 °C; IR vmax (KBr, cm−1): 3416, 2361, 1637, 1420, 1231, 1122; 1H-NMR (300 MHz, CDCl3) δ 6.81 (1H, d, J = 8.1 Hz, H-5′), 6.73 (1H, d, J = 1.8, 8.1 Hz, H-6′), 6.67 (1H, d, J = 1.8 Hz, H-2′), 6.37 (2H, s, H-3, 5), 4.76 (1H, t, J = 5.4 Hz, –OCH–), 3.88 (3H, s, –OCH3), 3.85 (3H, s, –OCH3), 3.80 (6H, s, 2 × –OCH3), 2.86 (4H, brs, –CH2CH2–), 1.91, 1.67, 1.58 (8H, m); 13C-NMR (75 MHz, CDCl3) δ 153.8, 149.7, 148.5, 138.3, 137.2, 134.5, 120.3, 111.9, 111.1, 105.7, 84.3, 56.1, 55.9, 55.8, 38.6, 37.5, 32.7, 23.7; ESI-MS m/z: 404.2 [M + NH4]+, 387.2 [M + H]+; HPLC purity: 98%, tR = 3.22 min.
4.1.6.10. 2-(Benzyloxy)-5-(3,4-dimethoxyphenethyl)-1,3-dimethoxybenzene (3j).
Yield 96.8%; yellow crystal, 77–78 °C; IR vmax (KBr, cm−1): 3416, 2937, 1589, 1514, 1128, 1028; 1H-NMR (300 MHz, CDCl3) δ 7.52–7.31 (5H, m, ArH), 6.81 (1H, d, J = 8.1 Hz, H-5′), 6.71 (1H, d, J = 1.8, 8.1 Hz, H-6′), 6.67 (1H, d, J = 1.8 Hz, H-2′), 6.37 (2H, s, H-3, 5), 5.00 (2H, s, –CH2–), 3.88 (3H, s, –OCH3), 3.86 (3H, s, –OCH3), 3.81 (6H, s, 2 × –OCH3), 2.87 (4H, s, –CH2CH2–); 13C-NMR (75 MHz, CDCl3) δ 153.3, 148.8, 147.3, 138.0, 137.6, 134.3, 128.5, 128.1, 127.8, 120.4, 111.9, 111.2, 105.6, 75.1, 56.1, 56.0, 55.8, 38.6, 37.6; ESI-MS m/z:426.2 [M + NH4]+, 409.2 [M + H]+; HPLC purity: 100%, tR = 3.53 min.
4.2. Pharmacology
4.2.1. Cell culture.
Aβ25–35 (Sigma) was dissolved in deionized distilled water to obtain a stock solution (1 mM). After aggregation induced by incubating at 37 °C for 4 days,44 the stock solution was stored at −20 °C.
The PC12 cells were purchased from the Institute of Biochemistry and Cell Biology (Shanghai, China) and cultured in Dulbecco's Modified Eagle's Medium (DMEM) supplemented with penicillin (100 U mL−1), streptomycin (100 µg mL−1), and 10% (vol/vol) fetal bovine serum (FBS) at 37 °C in an atmosphere containing 5% CO2. DMEM and FBS were obtained from Gibco (GrandIsland, NY). The other reagents were purchased from Beyotime Institute of Biotechnology (Shanghai, China).
For the experimental studies, the PC12 cells were subcultured in 96 or 6-well plates at a density of 1.5 × 104 or 2 × 105 cells per well and allowed to adhere and grow. When cells reached the required confluence, they were placed in serum-free medium and pretreated with different concentrations of test compounds for 0.5 h,45 and then Aβ25–35 (10 µM) was added to the medium for the corresponding time.
4.2.2. Cell viability assay.
The neuroprotective effects of test compounds on Aβ25–35-induced PC12 cells were determined by the MTT assay.46 After the treatments with test compounds and Aβ25–35, MTT (0.5 mg mL−1, final concentration) was added and incubated for 2 hours at 37 °C. Formazan crystals were dissolved by DMSO, and absorbance was measured at a wavelength of 570 nm by a 1500 microplate reader (Thermo Fisher Scientific Co.). Cell viability was expressed as a percentage of the non-treated control. Values were represented as the mean ± SD of 3 independent experiments in triplicate. The significance of differences between groups was assessed by a one-way analysis of variance (ANOVA).
4.2.3. Inhibition evaluation of self-induced Aβ1–42 aggregation.
Thioflavin T (ThT) fluorescence assay34 was used to assess the ability of four chrysotoxine derivatives to inhibit the self-induced Aβ1–42 aggregation with resveratrol and curcumin as reference compounds. Aβ1–42 (Anaspec Inc) was dissolved in HFIP (1 mg mL−1) and incubated for 24 h at room temperature. Then it was stored at −80 °C after solvent was evaporated. Solutions of test compounds were prepared in DMSO at 25 mM for storage and diluted with phosphate buffer solution (pH 7.4) before use.
For the inhibition of self-induced aggregation assay, 20 µL Aβ1–42 (25 µM, final concentration) was incubated with 20 µL of test compounds (25 µM, final concentration) in 50 mM phosphate buffer solution (pH 7.4) at 37 °C for 24 h. To minimize evaporation effect the wells were sealed by a transparent heat-resistant plastic film. After incubation, 160 µL of 5 µM thioflavin T (TCI (Shanghai) Development Co., Ltd.) in 50 mM glycine–NaOH buffer (pH 8.5) was added. Fluorescence was measured on a Varioskan Flash Multimode Reader (Thermo Scientific) with excitation and emission wavelengths at 446 nm and 490 nm, respectively. The fluorescence intensities were compared and the percent inhibition due to the presence of the inhibitor was calculated by the following formula: 100 − (IFi/IFc × 100), where IFi and IFc were the fluorescence intensities obtained for Aβ1–42 in the presence and in the absence of inhibitor, respectively. Each assay was run in triplicate.
4.2.4. Inhibition evaluation of Cu2+-induced Aβ1–42 aggregation.
To investigate the ability of the 4 active compounds to inhibit Cu2+-induced Aβ1–42 aggregation, a ThT-binding assay was used similar as before.35 Resveratrol and curcumin were chosen as reference compounds. Aβ1–42 was diluted in 20 mM HEPES (pH 6.6) with 150 mM NaCl. The mixture of the peptide (10 µL, 25 µM, final concentration) with or without copper (10 µL, 25 µM, final concentration) and the test compound (10 µL, 25 µM, final concentration) was incubated at 37 °C for 24 h. 30 µL of the sample was diluted to a final volume of 200 µL with 50 mM glycine–NaOH buffer (pH 8.0) containing thioflavin T (5 µM). The detection method was the same as that of self-induced Aβ aggregation experiment.
4.2.5. Metal chelating effect.
The study of metal chelation was performed in methanol at 298 K using UV-vis spectrophotometer (SHIMADZU UV-2450PC) with wavelength ranging from 200 to 500 nm.36,47 The difference UV-vis spectra due to complex formation was obtained by numerical subtraction of the spectra of the metal alone and the compound alone (at the same concentration used in the mixture) from the spectra of the mixture. A fixed amount of compound 2a (final concentration, 25 µM) was mixed with growing amounts of copper ion or aluminum ion (final concentration, 2–50 µM), and the difference UV-vis spectra was tested to investigate the ratio of ligand/metal in the complex.
4.2.6. Western blot analysis on Aβ-induced tau protein hyperphosphorylation.
After PC12 cells were lysed with RIPA buffer, total protein concentration was estimated by Bradford assay. Total cell lysates (20 µg) were resolved by 10% SDS-PAGE and transferred to nitrocellulose membrane. Membranes were incubated in a blocking buffer (PBS, 5% non-fat milk) for 1 h at 20 °C. After overnight incubation (4 °C) with primary antibody (tau 5 at 1
:
1500; p-tau S396 at 1
:
500; S199/202 at 1
:
500), the blots were washed in Tween 20-TBS (TBST) (0.02% Tween 20, 100 mM Tris, pH 7.5, 150 nM NaCl) for 20 min and then incubated with secondary antibody for 1 h at room temperature. The blots were washed in TBST for 20 min and incubated with ECL chemiluminescent reagent (Thermo Scientific) for 3 min. Quantification of pixel intensity was done with Image-Pro Plus 6.0 software. β-Actin was used as a control to normalize tau protein.
4.2.7. Docking study of compound 2a with Aβ1–42.
Molecular modeling calculations and docking studies were performed using Molecular Operating Environment (MOE) software version 2009.10 (Chemical Computing Group, Montreal, Canada). The X-ray crystal structure of Aβ1–42 (PDB 1IYT) used in the docking study was obtained from the Protein Data Bank (http://www.rcsb.org). Heteroatoms and water molecules in the PDB file were removed at the beginning, and all hydrogen atoms were added to the protein. Amber99 force field was assigned to the enzyme and the partial charges were calculated with the same force field. Protonated states of the enzyme at pH 7.4 were obtained by following the Protonate 3D protocol in which all configurations were set as default. Compound 2a was drawn in MOE with all hydrogen atoms added. During the docking procedure, pose of compound 2a was initially generated by Triangle Matcher method, and scored with London dG function. 30 poses of the compound were dedicated to the next refinement procedure. All poses were fine-tuned with the force field refinement scheme. The best 10 poses of molecules were retained and scored. After docking, the geometry of resulting complex was studied using the MOE's pose viewer utility.
4.2.8.
In vitro blood-brain barrier permeability assay.
The BBB penetration of compounds was evaluated using the parallel artificial membrane permeability assay (PAMPA) described by Di et al.43 Commercial drugs, dodecane and DMSO were purchased from Sigma and Aladdin. Porcine brain lipid (PBL) was obtained from Avanti Polar Lipids. The donor microplate (96-well filter plate, PVDF membrane, pore size of 0.45 µm) and acceptor microplate were purchased from Millipore. The 96-well UV plate (COSTAR®) was obtained from Corning Incorporated. The acceptor 96-well microplate was filled with 300 µL of PBS/EtOH (7
:
3), and the filter membrane was impregnated with 4 µL of PBL in dodecane (20 mg mL−1). Compounds were dissolved in DMSO at 5 mg mL−1 and diluted 50-fold in PBS/EtOH (7
:
3) to a final concentration of 100 µg mL−1. Then, 200 µL of the solution was added to the donor wells. The acceptor filter plate was carefully placed on the donor plate to form a sandwich, and the plates were incubated undisturbed for 18 h at 25 °C. After incubation, the donor plate was carefully removed, and the concentration of the compounds in the acceptor wells was determined using a UV plate reader (Flexstation® 3). Each sample was analyzed at five wavelengths in four wells and in at least three independent runs. Pe was calculated by the following formula: Pe = −Vd × [Va(Vd + Va)A × t] × ln(1 − drugacceptor/drugequilibrium), where Vd is the volume of the donor well, Va is the volume of the acceptor well, A is the filter area, t is the permeation time, drugacceptor is the absorbance obtained in the acceptor well, and drugequilibrium is the theoretical equilibrium absorbance. The results are given as the mean ± SD. In the experiment, 10 quality control standards with known BBB permeabilities were included to validate the analysis set. A plot of the experimental data versus the literature values gave a strong linear correlation: Pe(exp.) = 1.054Pe(bibl.) + 0.5582 (R2 = 0.987). From this equation and the limit established by Di et al. (Pe(lit.) = 4.0 × 10−6 cm s−1) for BBB permeation, we concluded that compounds with a permeability greater than 4.8 × 10−6 cm s−1 can cross the BBB.
Acknowledgements
This research work was financially supported by the National Natural Science Foundation of China (No. 81373956, No. 81274064, No. 81502950 and No. 81573557), Seed Funding Program for Basic Research from HKU (201111159043), the Priority Academic Program Development of Jiangsu Higher Education Institutions, the Fundamental Research Funds for the Central Universities (2015ZD010), Huahai Graduate Innovation Fund (No. CX14B-007HH), College Students Innovation Project for the R&D of Novel Drugs (J1030830), National Students Innovation and Entrepreneurship Training Program (G15073).
References
- H. W. Querfurth and F. M. LaFerla, N. Engl. J. Med., 2010, 362, 329–344 CrossRef CAS PubMed.
- World Alzheimer Report 2015, http://www.alz.co.uk/research/world-report-2015, assessed August 2015.
- G. Pepeu and M. G. Giovannini, Curr. Alzheimer Res., 2009, 6, 86–96 CrossRef CAS PubMed.
- B. Reisberg, R. Doody, A. Stöffler, F. Schmitt, S. Ferris and H. J. Möbius, N. Engl. J. Med., 2003, 348, 1333–1341 CrossRef CAS PubMed.
- K. A. Bates, G. Verdile, Q. X. Li, D. Ames, P. Hudson, C. L. Masters and R. N. Martins, Mol. Psychiatry, 2008, 14, 469–486 CrossRef PubMed.
- A. Boutajangout and T. Wisniewski, Gerontology, 2014, 60, 381–385 CrossRef CAS PubMed.
- A. I. Bush and R. E. Tanzi, Neurotherapeutics, 2008, 5, 421–432 CrossRef CAS PubMed.
- M. A. Ansari and S. W. Scheff, J. Neuropathol. Exp. Neurol., 2010, 69, 155 CrossRef CAS PubMed.
- P. T. Francis, A. M. Palmer, M. Snape and G. K. Wilcock, J. Neurol., Neurosurg. Psychiatry, 1999, 66, 137–147 CrossRef CAS.
- J. Hardy, J. Neurochem., 2009, 110, 1129–1134 CrossRef CAS PubMed.
- M. Jucker and L. C. Walker, Ann. Neurol., 2011, 70, 532–540 CrossRef CAS PubMed.
- M. Hernández-Rodríguez, J. Correa-Basurto, M. I. Nicolás-Vázquez, R. Miranda-Ruvalcaba, C. G. Benítez-Cardoza, A. A. Reséndiz-Albor, J. V. Méndez-Méndez and M. C. Rosales-Hernández, PLoS One, 2015, 10, e0130263 Search PubMed.
- O. Crescenzi, S. Tomaselli, R. Guerrini, S. Salvadori, A. M. D'Ursi, P. A. Temussi and D. Picone, Eur. J. Biochem., 2002, 269, 5642–5648 CrossRef CAS PubMed.
- S. Paul, S. Planque and Y. Nishiyama, Rejuvenation Res., 2010, 13, 179–187 CrossRef CAS PubMed.
- L. Huang, C. Lu, Y. Sun, F. Mao, Z. Luo, T. Su, H. Jiang, W. Shan and X. Li, J. Med. Chem., 2012, 55, 8483–8492 CrossRef CAS PubMed.
- J. Hardy and D. J. Selkoe, Science, 2002, 297, 353–356 CrossRef CAS PubMed.
- T. A. Dineen, K. Chen, A. C. Cheng, K. Derakhchan, O. Epstein, J. Esmay, D. Hickman, C. E. Kreiman, I. E. Marx, R. C. Wahl, P. H. Wen, M. M. Weiss, D. A. Whittington, S. Wood, R. T. Fremeau, R. D. White and V. F. Patel, J. Med. Chem., 2014, 57, 9811–9831 CrossRef CAS PubMed.
- S. Lovestone, C. H. Reynolds, D. Latimer, D. R. Davis, B. H. Anderton, J. M. Gallo, D. Hanger, S. Mulot and B. Marquardt, Curr. Biol., 1994, 4, 1077–1086 CrossRef CAS PubMed.
- A. M. Palmer, Trends Pharmacol. Sci., 2011, 32, 141–147 CrossRef CAS PubMed.
- L. E. Scott and C. Orvig, Chem. Rev., 2009, 109, 4885–4910 CrossRef CAS PubMed.
- S. Boopathi and P. Kolandaivel, RSC Adv., 2014, 4, 38951 RSC.
- D. E. Clark, J. Pharm. Sci., 1999, 88, 815–821 CrossRef CAS PubMed.
- W. M. Pardridge, Alzheimer's Dementia, 2009, 5, 427–432 CrossRef PubMed.
- M. Ono, Y. Ito, C. Masuoka, H. Koga and T. Nohara, Food Sci. Technol. Int., 1995, 1, 115–120 CrossRef CAS.
- J. X. Song, S. C. W. Sze, T. B. Ng, C. K. F. Lee, G. P. H. Leung, P. C. Shaw, Y. Tong and Y. B. Zhang, J. Ethnopharmacol., 2012, 139, 698–711 CrossRef CAS PubMed.
- J. J. Fan, L. Guan, Z. Q. Kou, F. Feng, Y. B. Zhang and W. Y. Liu, J. Chromatogr. B, 2014, 967, 57–62 CrossRef CAS PubMed.
- J. X. Song, P. C. Shaw, C. W. Sze, Y. Tong, X. S. Yao, T. B. Ng and Y. B. Zhang, Neurochem. Int., 2010, 57, 676–689 CrossRef CAS PubMed.
- J. X. Song, P. C. Shaw, N. S. Wong, C. W. Sze, X. S. Yao, C. W. Tang, Y. Tong and Y. B. Zhang, Neurosci. Lett., 2012, 521, 76–81 CrossRef CAS PubMed.
- A. A. Reinke and J. E. Gestwicki, Chem. Biol. Drug Des., 2007, 70, 206–215 CAS.
- Y. Hernández-Romero, J. I. Rojas, R. Castillo, A. Rojas and R. Mata, J. Nat. Prod., 2004, 67, 160–167 CrossRef PubMed.
- Y. W. Lee, D. H. Kim, S. J. Jeon, S. J. Park, J. M. Kim, J. M. Jung, H. E. Lee, S. G. Bae, H. K. Oh, K. H. Ho Son and J. H. Ryu, Eur. J. Pharmacol., 2013, 704, 70–77 CrossRef CAS PubMed.
- A. Diaz, D. Limon, R. Chávez, E. Zenteno and J. Guevara, J. Alzheimer's Dis., 2012, 30, 505–522 CAS.
- Y. G. Kaminsky, M. W. Marlatt, M. A. Smith and E. A. Kosenko, Exp. Neurol., 2010, 221, 26–37 CrossRef CAS PubMed.
- N. Jiang, X. B. Wang, Z. R. Li, S. Y. Li, S. S. Xie, M. Huang and L. Y. Kong, RSC Adv., 2015, 5, 14242–14255 RSC.
- N. Jiang, S. Y. Li, S. S. Xie, Z. R. Li, K. D. G. Wang, X. B. Wang and L. Y. Kong, Eur. J. Med. Chem., 2014, 87, 540–551 CrossRef CAS PubMed.
- S. Y. Li, X. B. Wang and L. Y. Kong, Eur. J. Med. Chem., 2014, 71, 36–45 CrossRef CAS PubMed.
- M. G. Spillantini and M. Goedert, Trends Neurosci., 1998, 21, 428–433 CrossRef CAS PubMed.
- C. Ballatore, V. M. Y. Lee and J. Q. Trojanowski, Nat. Rev. Neurosci., 2007, 8, 663–672 CrossRef CAS PubMed.
- A. A. Reinke and J. E. Gestwicki, Chem. Biol. Drug Des., 2007, 70, 206–215 CAS.
- W. Pardridge, Neurotherapeutics, 2005, 2, 3–14 CrossRef PubMed.
- C. A. Lipinski, Drug Discovery Today, 2004, 1, 337–341 CrossRef CAS PubMed.
- D. E. Clark, J. Pharm. Sci., 1999, 88, 815–821 CrossRef CAS PubMed.
- L. Di, E. H. Kerns, K. Fan, O. J. McConnell and G. T. Carter, Eur. J. Med. Chem., 2003, 38, 223–232 CrossRef CAS PubMed.
- Y. F. Xian, Z. X. Lin, Q. Q. Mao, S. P. Ip, Z. R. Su and X. P. Lai, Cell. Mol. Neurobiol., 2012, 32, 353–360 CrossRef CAS PubMed.
- G. Li, R. Ma, C. Huang, Q. Tang, Q. Fu, H. Liu, B. Hu and J. Xiang, Neurosci. Lett., 2008, 442, 143–147 CrossRef CAS PubMed.
- D. L. Chen, P. Zhang, L. Lin, O. Shuai, H. M. Zhang, S. H. Liu and J. Y. Wang, Cell. Mol. Neurobiol., 2013, 33, 837–850 CrossRef CAS PubMed.
- W. Huang, D. Lv, H. Yu, R. Sheng, S. C. Kim, P. Wu, K. Luo, J. Li and Y. Hu, Bioorg. Med. Chem., 2010, 18, 5610–5615 CrossRef CAS PubMed.
Footnote |
† Electronic supplementary information (ESI) available. See DOI: 10.1039/c5ra21313d |
|
This journal is © The Royal Society of Chemistry 2016 |
Click here to see how this site uses Cookies. View our privacy policy here.