DOI:
10.1039/D0RA08088H
(Paper)
RSC Adv., 2021,
11, 6049-6059
The enhancement of treatment capacity and the performance of phytoremediation system by fed batch and periodic harvesting†
Received
22nd September 2020
, Accepted 16th January 2021
First published on 2nd February 2021
Abstract
Floating macrophyte phytoremediation could be the most relevant solution to the ever-increasing finfish farm pond effluent worldwide. However, the information of Spirodela polyrhiza monoculture system in fed batch mode, with periodic harvesting and increased macrophyte density is limited. In this study, the effect of fed batch and periodic harvesting on the treatment capacity and performance of the S. polyrhiza monoculture system (with increased the macrophyte density) in fish farm wastewater were evaluated. Results showed that the system with fed batch and harvesting could treat a greater volume of wastewater, remove a higher amount of pollutants in terms of ammonia (NH3–N), phosphate (PO43−), total suspended solids (TSS) and chemical oxygen demand (COD), while meeting the effluent limits. The system with S. polyrhiza macrophyte density of 11.67 g fresh weight (FW) per L wastewater was able to decrease nitrate (NO3−–N) and nitrite (NO2−–N) to an undetected level. This study suggested that the S. polyrhiza monoculture system with fed batch, optimal harvesting and frequent sediment removal is feasible and effective in treating the fish farm wastewater, and produces biomass with superior protein content for fish feed supplement and poultry diet. The obtained data provided insights into the system reliability in wastewater treatment and ways of improvement for the system. The treated wastewater could achieve exceptional quality with minimal toxicity before discharge to receiving waters, and potentially be reused for water flow recharge, aquaculture and irrigation purposes, minimizing the pollution and ecological impacts.
1 Introduction
Aquaculture constituted 47% of the world fish production, and continues to grow faster than other major food production sectors.1 Its production depends progressively on inland aquaculture (64%), which is dominated by finfish farming (92.5%), achieving 47.5 million tonnes (year 2016).1 The production increased yearly, with 38.6 and 43.6 million tonnes in 2012 and 2014, respectively.2,3 Therefore, the continued expansion and operation of the inland finfish aquaculture site would cause increased land reclamation, effluent discharge and environmental degradation on the resource base. The fish farm wastewater is high in dissolved nutrients (nitrogen (N) and phosphorus (P)) and suspended solids,4–6 which basically originated from uneaten feed, fish faeces and excretion.5–7 Based on the estimate that water discharge is 5.5 m3 kg−1 finfish production from pond culture,8,9 the wastewater generated from the inland finfish industry alone reached up to 261.2 billion m3 worldwide in 2016. Provided that ammonia-N, nitrate-N and phosphate levels in fish farm wastewater are 26.5, 15.3 and 6.0 mg L−1, respectively,10,11 6.9, 4.0 and 1.6 million tonnes of ammonia, nitrate and phosphate were released globally into the environment.
The current practice for the wastewater is either discharge directly to waterways or go through a settlement/sedimentation pond before discharge.10 However, the issue of nutrient removal from the wastewater is still not properly addressed. Phytoremediation technology using floating macrophytes is considered the easiest approach, as treatment can be done directly by dispersing and cultivating macrophytes on the existing sedimentation ponds. It is not only effective in nutrient removal, but also potentially used as a means of resource recovery for producing value-added feedstock for fish and poultry husbandry, fertiliser and biofuel. It is the most feasible way for aquafarmers since no large capital is needed to construct the facility and treatment equipment, such as pumps, and no high recurring operation costs are required for maintenance, chemicals addition and electricity usage. Phytoremediation is self-sustaining by relying on sunlight, has minimal energy requirement, and is conceived as a low carbon footprint technology. Floating macrophytes are also easily harvested compared to microalgae. This is due to their larger size and lower density compared to water (with two distinct phases). Thus, the dewatering process that incurred high operational cost and energy consumption from microalgae separation12,13 can be omitted. Spirodela polyrhiza is chosen for the study due to its prominent nutrient uptake from synthetic14 and fish farm wastewater15 observed in previous studies, its environmental adaptability16 and economically attractive application of duckweed biomass.17
To the authors' knowledge, to date, the available research studies10,17 using Spirodela polyrhiza for the treatment of fish farm wastewater were conducted in batch system. A treatment system in batch mode may not be ideal for fish farm pond aquaculture effluent since the treatment capacity is limited and cannot cater to intermittent effluent discharge. The effluent comes from the water exchange of the pond, where a partial water exchange can be done daily from 0–4.4%,8,18,19 or when the pond gets near its carrying capacity, about 25%, whereas a full water exchange is done twice a year. Fed batch could be a more appropriate approach for the effluent compared to continuous mode, as the effluent discharge can be irregular, remediation requires a few days,15 and fed batch ensures the effluent is properly treated and reaches the limit before discharge. Periodic harvesting could become an alternative to increase the wastewater treatment performance. It not only permanently removes accumulated nutrients from the system,20 but also maintains the vigorous growth of the macrophyte colony21 in the system as older plants are removed. This leaves healthy, productive plants,20 while the crowding of macrophytes and self-shading are avoided.22 It thereby increases the efficiency of the nutrient and pollutant uptake from the wastewater. Only one study was conducted specifically to evaluate the effect of the harvesting regime on the nutrient recovery, but in swine wastewater.23 It indicated that harvesting 20% of duckweed twice a week will have higher total nitrogen (TN) and total phosphorus (TP) removals.23 Harvesting amounts of 10–25%22–24 and frequency of 1–6 days22–26 were reported. However, the harvesting amounts and frequency can be varied depending on the macrophyte species, wastewater type and nutrient level, and culture conditions, and should be determined via practical experience.22 The macrophyte density could also affect the nutrient removal from wastewater. Higher density is expected to have higher removal, but a relevant study was not found in the recent research for fish farm wastewater. Therefore, the utilisation of Spirodela polyrhiza in remediating fish farm wastewater in fed batch, with periodic harvesting scheme and increased macrophyte density is limited in the literature context, and this research aims to address these concerns. From a previous study,15 the S. polyrhiza monoculture system has the best performance among other systems in treating fish farm wastewater. In this study, fed batch and periodic harvesting were done to this S. polyrhiza monoculture system (with increased macrophytes density) to evaluate its treatment capacity and performance on fish farm wastewater. Ammonia (NH3–N), nitrate (NO3−–N), nitrite (NO2−–N), phosphate (PO43−), total suspended solids (TSS), turbidity and chemical oxygen demand (COD) removal from wastewater and corresponding changes in biomass, total carbohydrate and protein contents of the system were determined for the study. The obtained data will reveal whether fed batch mode, periodic harvesting practice and increased macrophyte density have any effect on the treatment system capacity and performance in terms of the volume of wastewater processed, amount of pollutant removed, pollutant removal efficiency and the biomass productivity. It also helps us gain insight into the reliability of the phytoremediation system after the modifications, to further improve the system, and eventually realise the commercialisation of the system in fish farm sectors.
2 Materials and methods
2.1. Source of wastewater
The wastewater was collected from a freshwater catfish farming ponds in Tanjong Piandang, northwest of the Kerian District, Perak, Malaysia. The farm covers an area of about 3 ha with 19 ponds (approximately 11
450 m2). The wastewater was taken from 4 different ponds, but were pooled together before use to limit the water quality variation due to the age of the fishes, amount of uneaten feed remained, amount of fish excretion and faeces, as well as the water exchange rate of the respective ponds. The quality of the wastewater is shown in ESI Table S1.†
2.2. Plant stock establishment
The macrophytes Spirodela polyrhiza were collected locally from a water pathway near the catfish farm. The aseptic cultures were established according to the procedure described by Ng and Chan,14 and maintained in liquid Hoagland No. 2 medium27 (ESI Table S2†) with 15 g L−1 sucrose. All cultures were then incubated at 29 ± 1 °C under a Philips TL-D 36W/865 fluorescent light (1500 lux) with a 16 h light:8 h dark photoperiod for 14 days. The propagated macrophytes were collected, washed twice with water, and then blotted with absorbent paper to remove any nutrients and sucrose from the medium before being used for the experimental setup in our phytoremediation study. This was to ensure that nutrients and sucrose were not introduced to the phytoremediation experiment.
2.3. Setup and conduct of the study
This study was carried out in a bench scale raceway pond rig system adapted from Ng and Chan,28 with dimensions of 50 cm × 25 cm × 9 cm, inside the laboratory under a controlled environment (see ESI Fig. S1(a) and (b)†). The transparent acrylic pond rig is covered with light absorbing material to prevent the excessive growth of algae. Two treatment systems were configured for the study, namely control system (without fed batch and harvesting) and FBH system (fed batch and harvesting system). Both systems were started with 12 L of fish farm wastewater and 140 g fresh weight of S. polyrhiza biomass (double the biomass/initial macrophyte density in the previous work,15) which was distributed evenly over the wastewater surface in the raceway pond. A submerged pump was used to circulate the wastewater in the raceway pond rig system, which was equipped with a RMA-34-SSV flowmeter (Dwyer Instruments, USA) at a flow rate of 50 ml min−1. The phytoremediation study was carried out at 29 ± 1 °C under Philips TL-D 36W/865 fluorescent tubes (1500 lux) with a 16 h light:8 h dark photoperiod for 16 days. A water sample (150 ml) was collected at the outlet of the pond rig every 2 days, starting at day 0 until the end of the experiment. The water samples were analysed for ammonia (NH3–N), nitrate (NO3−–N), nitrite (NO2−–N), phosphate (PO43−), chemical oxygen demand (COD), turbidity, total suspended solids (TSS) and pH. Those parameters were determined according to the standard method and protocol (see ESI Protocol S1†).
The difference between both systems was that, in the FBH system, S. polyrhiza biomasses were partially harvested and the fish farm wastewater was partly replenished with fresh wastewater on days 4, 8 and 12. Additional water collections were performed on days 4, 8 and 12. On those days, after water samples were collected, 25% surface area of S. polyrhiza covering the raceway pond was harvested. The harvested biomass was washed and blotted before being weighed. Next, 6 L of fish farm wastewater (50% of the volume) in the rig system was replaced with the fresh, new wastewater medium. The remaining S. polyrhiza (unharvested) was then ensured to be distributed equally and evenly throughout the pond surface. Another water collection was done after the old and new wastewater had been well mixed to examine the starting water quality of the fish farm wastewater after fed batch. On day 16, all biomasses of S. polyrhiza from both systems were harvested and blotted carefully with absorbent paper before being weighed for their respective fresh weights. For the FBH system, S. polyrhiza samples harvested at days 4, 8 and 12 were considered and accounted into the increment of biomass. The biomasses were then subjected to biochemical analysis to evaluate their carbohydrate and protein content.
Both systems were carried out for three replicates. The treatment capacity and performance of both systems were assessed and compared. Some of the indicators used for evaluation include the pollutant removal efficiency, total pollutant removed (ESI eqn (S1)–(S3)†), volume of wastewater treated and conformity to standard limit, depending on respective graph trends. The biomass productivity and biochemical content change for both systems were also examined.
2.4. Determination of total carbohydrate and total protein
The dried macrophyte biomass was blended into fine powder before undergoing acid hydrolysis extraction by Hoebler et al.29 0.1 g of the fine powder sample was mixed with 1.25 ml of 72% (w/w) sulphuric acid in a vial and kept at 30 °C for 30 minutes for primary hydrolysis. The mixture was then diluted with 13.5 ml of water and kept in a boiling water bath at 100 °C for 1 hour. After cooling and the addition of 3.1 ml NaOH 32% (w/v), solutions were centrifuged at 3500 × g for 15 minutes to obtain the supernatant. The carbohydrate was determined through the colorimetric method described by Dubois et al.30 using sucrose as the standard. The protein was determined through the test tube procedure of the BCA method outlined in the Thermo Scientific™ Pierce™ BCA Protein Assay Kit instructions with bovine serum albumin (BSA) being used as the standard.
2.5. Statistical analysis
The mean value and standard error were calculated for all analysed parameters. Error bars representing the standard errors were added to all plotted graphs. The statistical significance in the levels of treatment, biomass and biochemical content change in the control and FBH systems were assessed by paired t-test. For the water quality assay, each level analysed throughout the experiment was evaluated for significant differences with reference to the initial level in the control system. In the FBH system, the tests were carried out within respective terms, with respect to the initial level on that particular term. The statistical tests were performed using Minitab® version 16.2.1.
3 Results and discussion
3.1. Removal of nitrogen
The concentration of various nitrogen species, namely ammonia, nitrate and nitrite in fish farm wastewater treated by FBH, and the control systems are depicted in Fig. 1(a)–(c), respectively. The ammonia in the control system fell steeply to 9.80 ± 0.12 mg L−1 on day 2, and then to 4.55 ± 0.61 mg L−1 on day 4 with 83% removal efficiency. It dropped to an undetectable value on day 8, and maintained the level until the end of the run. The ammonia reduction was significant (p < 0.05) starting on day 2 towards the end of the study. Meanwhile, a similar trend was observed between its nitrate and nitrite levels, where both showed an almost undetected value throughout the experiment. The average nitrate and nitrite levels in the control system were determined at around 0.86 mg L−1 and 0.31 mg L−1, respectively. As for the FBH system, the ammonia level dropped sharply and significantly to 5.95 ± 0.49 mg L−1 in the end of term 1 (p < 0.05), with 75% of the removal efficiency. A significant decrement in the ammonia level was also demonstrated in terms 2, 3 and 4 (p < 0.05), whereby their levels declined to 4.05 ± 0.26 mg L−1, 1.90 ± 0.35 mg L−1 and 2.95 ± 0.03 mg L−1 on the final day of the respective terms, with removal efficiencies of 76%, 90%, and 84%, respectively. The mean ammonia removal efficiency in the FBH system during those four terms was valued at 81%. In the meantime, the profiles shown in nitrate and nitrite concentration of the FBH system were similar to each other. Both nitrate and nitrite presented an almost undetected value during term 1, and only rose slightly to levels of 0.51 ± 0.02 mg nitrate-N per L and 0.05 ± 0.01 mg nitrite-N per L in the end of term 1. During term 2, the nitrate and nitrite concentrations remained low from day 4 to day 6, but a moderate increment was noted for the next 2 days, in which both concentrations went up to 5.50 ± 0.69 mg nitrate-N per L and 3.20 ± 0.46 mg nitrite-N per L on the last day of the referred term (p < 0.05). A considerable high rise in nitrate and nitrite was found during term 3, whereby the nitrate level climbed from 1.25 ± 0.72 mg L−1 to 22.90 ± 1.44 mg L−1 (p < 0.05), while the nitrite level increased from 1.70 ± 0.29 mg L−1 to 9.50 ± 0.87 mg L−1 (p < 0.05). During term 4, nitrate rose from 2.80 ± 1.27 mg L−1 to 12.45 ± 0.66 mg L−1 (p < 0.05), whereas nitrite went up from 4.10 ± 0.52 mg L−1 to 5.50 ± 0.29 mg L−1 (p < 0.05).
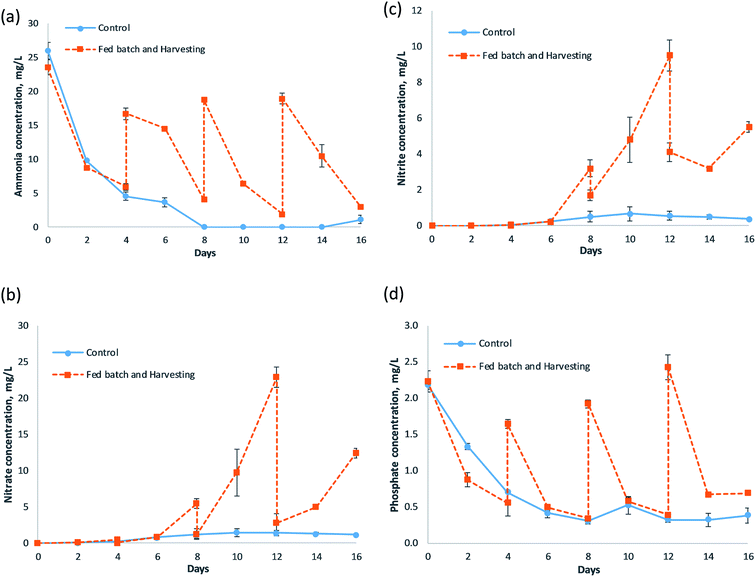 |
| Fig. 1 Concentration of (a) ammonia (NH3–N), (b) nitrate (NO3−–N), (c) nitrite (NO2−–N) and (d) phosphate (PO43−) in the fish farm wastewater in the course of 16 days of treatment by the control system and FBH system. Wastewater withdrawal and replenishment (fed batch), as well as biomass harvesting, were carried out on day 4, 8 and 12 only in the FBH system, but not in the control system. For the FBH system, its experimental period was divided into four terms, where terms 1, 2, 3 and 4 fall on days 0–4, days 4–8, days 8–12 and days 12–16, respectively. | |
For the control system, the ammonia removal remained high and as much as 83% of the ammonia could be removed in 4 days' time, and the level was rendered low for all of the times. It was primarily due to the high ammonia uptake capability of the S. polyrhiza macrophytes in the system, as discussed by Ng et al.15 Although the nutrients can be taken up passively via diffusion through the root membrane when the concentrations are sufficiently high, their active uptake system plays an important role in maximizing their uptake. The active uptake relies on the presence of specific transporter proteins on the cell membrane. As for ammonia, the roots rely on the AMT genes for the uptake of the NH4+ present in the wastewater.31 S. polyrhiza took up the ammonia and nitrate via its fronds and roots. The nitrogen constituent was then transported and accumulated in the biomass, where fluxes of ammonia and nitrate ions were detected in those parts.32,33 Nitrogen is essential to plant, as it is the elementary component of amino acids, proteins and nucleic acids, as well as chlorophyll.34 The nitrogen is crucial in the construction, growth and development of plant tissues and cells, the genetic material (e.g., DNA for plant reproduction), expressing plant traits and regulating plant cell processes, photosynthesis for carbohydrate formation, as well as giving green coloring to the plant. The nitrification process could also partly contribute to the decrement of the ammonia level in the wastewater. However, it was found that the accumulation of nitrate and nitrite in the wastewater as a result of nitrification, as in an earlier study,15 was not noticeable or remarkably observed. Instead, its nitrate and nitrite levels were almost undetected, as depicted in Fig. 1(b) and (c), in the control system. Although the same system (S. polyrhiza monoculture system) was employed for the current and previous studies,15 the macrophyte densities in both systems were different. The current system (control system) had higher macrophyte density (11.67 g FW per L wastewater) than the previous system (6.00 g FW per L wastewater), where it almost doubled the one in the previous system. Therefore, the presence of a larger amount of S. polyrhiza macrophytes allowed for a higher quantity of nitrate and nitrite to be taken up from the wastewater, and assimilated into their biomass. Thus, nitrate and nitrite in the control system could be reduced to such a low level compared to the system in the previous study. In nitrate, the NRT1 and NRT2 genes are responsible for mediating the roots in the uptake of NO3− under both low and high affinity transport systems.31 Moreover, a higher amount of ammonia removal by a greater density of macrophytes could prevent the availability of ammonia in wastewater for conversion into nitrate and nitrite via nitrification. It proved that the S. polyrhiza monoculture system with proper density coverage was able to reduce the nitrate and nitrite levels below the stringent limit and guideline, as well as further polishing the wastewater to a much lower and undetected level. The nitrate-N level was kept below the standard, 10 mg L−1,35–38 all the times and the maximum level occurred during 16 days of study. The amount of 1.45 mg L−1 nitrate-N obtained, was 86% lower than the standard, which was also much lower than the previous study. The nitrite-N concentration was also maintained below the drinking water guideline at 0.9 mg L−1 (ref. 38) and standard at 1 mg L−1 (ref. 39) throughout the study.
As for the FBH system, there were spikes in the ammonia level on days 4, 8 and 12, or specifically the beginning of terms 2, 3 and 4. This was attributed to 50% replenishment of the wastewater medium on the mentioned days, which had introduced more pollutants, e.g. ammonia, into the wastewater itself during the study. Hence, the increment in the ammonia concentration was shown on those days. In spite of that, the FBH system managed to reduce the ammonia to a very low level, which was below the statutory limits of 5 mg L−1 (ref. 35) and 10 mg L−1,40 as the average ammonia level at the end of four terms was determined at 3.71 mg L−1. It demonstrated that the system allowed the fish farm wastewater with an initial quality of around 20 mg L−1 to be sufficiently treated before discharge for each term. For term 3, the ammonia in the system could be reduced to as low as 1.90 ± 0.35 mg L−1. Furthermore, the mean ammonia removal efficiency in the FBH system for the four terms was high (81%), which was almost similar to the control system (83%) under the first 4 days of treatment. The ammonia removal remained efficient throughout the study, although the system had been routinely fed with fresh wastewater. The system did not show any sign of saturation or significant drop in ammonia removal efficiency as the days proceeded. The efficiency in the FBH system only fluctuated within 75–90%. The main causes of ammonia reduction in the system were the same as the control system. However, the periodic harvesting of the S. polyrhiza macrophyte from the system might help to remove ammonia from the wastewater since it maintained the optimal growth of the colony.21 Crowding inhibits the macrophytes' growth,22 which in turn lowered their uptake performance. The FBH system was found to have a higher total amount of ammonia removal than the control system. Throughout the 16 days study, the total amount of ammonia removed by the FBH system was 756.60 mg, which was determined to be 2.54 times greater than the control system with only 298.20 mg. A larger amount of ammonia-contaminated wastewater was also being treated by the FBH system (about 30 L) than the control system with only 12 L of wastewater. Hence, the FBH system could have 150% more treatment capacity than the control system. In the case of nitrate and nitrite for the FBH system, their levels were almost undetected from day 0 to day 6, and showed an incremental rise as the term proceeded. This could be due to a few reasons. First, more organic nitrogen was available at the rig's bottom floor due to sedimentation as fresh wastewater was added routinely. It allowed for a higher conversion into nitrate and nitrite through nitrification since ammonia was readily present from the organic nitrogen. Second, the harvesting rate of the macrophytes was higher than the growth rate of the macrophytes, leading to lower macrophyte density in the system. This would greatly reduce the removal and assimilation of nitrate and nitrite into the macrophytes' biomass. Third, the presence of nitrifying organisms from the wastewater of the previous term could accelerate the nitrification, causing the conversion into nitrate and nitrite to be faster, and thus a high increment of both levels under a short period of time. As time passed, the level of both nitrogen species increased. The nitrate level was below the most stringent limit, 10 mg L−1, for most of the time, except for the last day of terms 3 and 4, while the nitrite level was only below the drinking water standard from day 0 to day 6. It is believed that this outcome can be improved by removing the sediments (organic nitrogen) frequently, or in the end of each term, as well as reducing the harvesting rate or percent of harvested surface. The exceptional low level of nitrate and nitrite could be achieved from the frequent removal of sediment and proper density coverage of the macrophytes.
3.2. Phosphate removal
Fig. 1(d) presents the phosphate removal by FBH and control systems in the fish farm wastewater during the study. In general, both systems expressed the reduction in phosphate, but in different ways. A steep reduction was observed in the control system in the first 4 days, to 0.70 ± 0.01 mg L−1 with 68% removal efficiency, and then the phosphate was reduced considerably to 0.41 ± 0.06 mg L−1 on day 6. It later maintained the level around 0.38 mg L−1. Its phosphate reduction was significantly different from day 2 onwards (p < 0.05). For the FBH system, similarly, the phosphate was reduced sharply during term 1 to a level of 0.55 ± 0.18 mg L−1 (p < 0.05) with a removal efficiency of 75%. The rapid decrement of phosphate persisted in term 2 after the partial replenishment of wastewater on day 4 with 79% removal efficiency to 0.34 ± 0.03 mg L−1 (p < 0.05). The sharp phosphate decrement carried on in terms 3 and 4, where 80% and 71% removal efficiencies were recorded, to levels of 0.39 ± 0.02 mg L−1 (p < 0.05) and 0.69 ± 0.02 mg L−1 (p < 0.05), respectively.
The rapid phosphate reduction in both systems was attributed to the same factors, as discussed by Ng et al.,15 whereby both utilised S. polyrhiza macrophytes, which has inherent high and rapid phosphate removal capability.14 The PO43− uptake by the macrophytes and subsequent intracellular storage were demonstrated.41–43 The mass balance in the non-axenic study showed that the uptake of phosphorus by the macrophytes was the dominant mechanism of removal.43 The movement of P across the root cell requires an energy-driven phosphate transporter to move P through the membranes into the plant root cells.44 Phosphorus is a macronutrient of plants, and the major constituent in adenosine diphosphate (ADP) and adenosine triphosphate (ATP), which are crucial for energy storage and transfer in photosynthesis and respiration. It is needed for the formation of ADP and ATP, and also as the building block for nucleic acids, nucleotides, sugar phosphates and many more,45 especially during the active growth of plants (e.g., cell division and development of new tissues). It is also a component of various enzymes and proteins, RNA and DNA, where both are held by a phosphorus bond. The genetic material is vital for the proper development, functioning, growth, and reproduction in the plant. Although the phosphate removal efficiency in the control system was high, the treatment capacity was low since it is a batch system. It only can treat a fixed amount of wastewater based on the designed volume of the system. As for our rig system, the control system (batch system) could only process a 12 L volume of wastewater per study. In addition, most of the time (about 75%), the phosphate level was in the steady state, where no significant phosphate removal was found. However, when the fed batch was applied and 50% of the wastewater was being replenished every 4 days, the total amount of treated wastewater increased up to 30 L, which tremendously elevated the treatment capacity. The mean phosphate removal efficiency in the end of all terms was 76%, which was almost equivalent to its initial 4 days (term 1) removal efficiency. With larger treatment capacity, as well as high phosphate removal efficiency, demonstrated in the FBH system, the total amount of phosphate removed can be huge compared to the control system. The total amount of phosphate removed by the FBH system was 3.47 times greater than the one in control system, in which the total amount of removal was found to be 74.88 mg and 21.60 mg, respectively. Harvesting also ensured the optimum growth of the macrophytes, so that the removal of phosphate by absorption could be higher and more efficient. Moreover, the FBH system was capable of further depurating the fish farm wastewater down to 0.11 ± 0.01 mg P per L and with an average level of 0.16 mg P per L for the four terms.
Generally, the pH profile in both systems was steady throughout the experiment, with the control system fluctuating within 7.76–7.84, while the FBH system hovered between 7.64 and 7.87 (ESI Fig. S2†). These pH ranges allowed for the optimal growth of the macrophytes, thus boosting the nitrogen and phosphorus phytoremediation, and granted the occurrence of nitrification.
3.3. Profiles of the total suspended solids, turbidity and COD
The total suspended solids (TSS), turbidity and COD profiles of fish farm wastewater treated by the control and FBH systems during the 16 days of experimental period are shown in Fig. 2(a)–(c), respectively. In general, the trend of TSS, turbidity and COD in the control system were identical, where a significant decrement occurred in the first 4 days. This was followed by a slight decline in the level until the end of the study, while the TSS, turbidity and COD profiles of the FBH system were similar in that a substantial reduction took place at every term, and their levels rocketed simultaneously on days 4, 8 and 12 once the wastewater was partially replenished. As for the control system, its TSS dropped significantly to a level of 34 ± 8 mg L−1 in the first 4 days, with 61% removal efficiency. The decrement in TSS was slow thereafter. It recorded 27 ± 7 mg L−1 on day 6 (p < 0.05) and reached 16 ± 5 mg L−1 (p < 0.05) with 81% of TSS removal on the last day of the run. For turbidity, it fell considerably from an initial value of 83.45 ± 1.07 NTU to 22.05 ± 3.20 NTU on day 4 (p < 0.05, 74% removal), and then declined slightly until the end of the experiment, in which 6.03 ± 1.25 NTU was attained on day 16 (p < 0.05, 93% removal). A significant decrease of COD in the control system was observed in the first 4 days to 43 ± 8 mg L−1 (p < 0.05), with 65% removal efficiency. The COD level went down slightly from day 4 to day 16, where it achieved 24 ± 4 mg L−1 on the final day of study (p < 0.05), with a removal efficiency of 80%. As for the case in the FBH system, its TSS fell substantially to a level of 21 ± 5 mg L−1 on day 4 (p < 0.05), with 73% of removal efficiency for the term 1. A sharp TSS decrement was found in terms 2, 3 and 4, where they achieved the levels of 10 ± 0 mg L−1, 5 ± 3 mg L−1 and 11 ± 6 mg L−1 in the end of their respective terms (p < 0.05), with a removal efficiency of 91%, 95% and 90%, respectively. For turbidity, the level in the FBH system went down significantly from 78.95 ± 4.07 NTU to 12.55 ± 0.09 NTU during term 1 (p < 0.05), with 84% turbidity removal. During term 2, it started with 122.50 ± 0.29 NTU and fell rapidly to 6.96 ± 0.62 NTU (p < 0.05), which recorded 94% turbidity removal. For terms 3 and 4, they initiated with 119.00 ± 2.89 NTU and 149.50 ± 0.29 NTU, and then the levels dropped steeply to 3.31 ± 0.31 NTU and 5.76 ± 0.68 NTU on the final day of the respective terms (p < 0.05, with 97% and 96% turbidity removal, respectively). In the case of the COD level, a similar profile was found. The COD declined substantially to a level of 45 ± 2 mg L−1 during term 1 (p < 0.05), with 65% removal efficiency. A sharp COD fall was observed in terms 2, 3 and 4, to levels of 41 ± 4 mg L−1, 29 ± 2 mg L−1 and 36 ± 4 mg L−1 on the last day of the respective terms (p < 0.05), which recorded removal efficiencies of 75%, 80% and 79%, respectively.
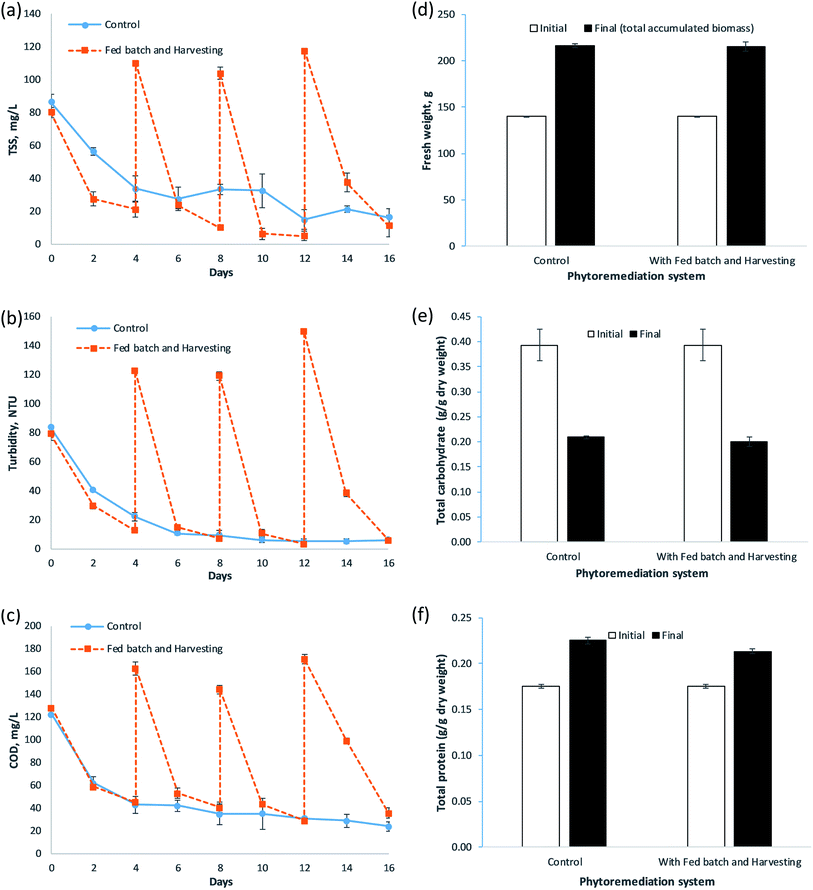 |
| Fig. 2 Water quality assay of (a) total suspended solid (TSS), (b) turbidity and (c) COD profiles in fish farm wastewater treated by the control system and FBH system, as well as their corresponding biomass change in (d) fresh weight, as well as biochemical content change in the (e) total carbohydrate and (f) total protein content of the macrophytes for 16 days of phytoremediation study. | |
The reasons for causing the TSS, turbidity and COD decrement in the control and FBH systems were identical as those discussed by Ng et al.15 It was mainly due to sedimentation of the die-off algae present in the wastewater caused by the shading effect from dense mats of macrophytes and the system itself (covered with light absorbing material). Studies from Lin et al.46 and Yeh et al.47 showed that shading reduced algae in eutrophic wastewater, and enhanced TSS and COD removal from water body. The turbidity improved after the TSS responsible for obstructing light penetration in the waters48–51 was removed. The prevention of wave formation by macrophytes52–54 and the metabolic activities of microbes resided on the macrophytes55–57 were also among the factors for the TSS, turbidity and COD decrement. For the FBH system, its routine harvesting of macrophytes might aid in lowering the TSS level in the wastewater, making the water clearer and reducing COD within. This is because the roots and fronds of the macrophytes could filter and retain some of the suspended solids and algae assemblage. Harvesting the macrophytes means removing together the suspended solids retained and attached from the wastewater. Within the 16 days study, the FBH system was able to remove 4365 mg of TSS, whereas the control system just removed 840 mg of TSS. The sum of TSS removed in the FBH system was 5.20 times the one in the control system. Although the control system attained 83% TSS removal on day 12, it treated merely 12 L of wastewater. On the other hand, the FBH system had processed 30 L of wastewater, with 87% of the mean removal efficiency throughout the terms. Aside from that, the wastewater discharged from each term were complied with Class I standard, 25 mg L−1 (ref. 58) and far less than the discharge limit, 50 mg L−1.35,40 In the case of turbidity, the FBH system was also capable of discharging a higher volume of wastewater with increased clarity. Each discharge could have an average of 93% lower turbidity value. The wastewater release can achieve quality as low as 3.31 ± 0.31 NTU too. As for COD, the control system managed to decrease the level down to 24 ± 4 mg L−1 (the lowest level so far), with 80% of the removal efficiency, but it would require 16 days to achieve such a level. Instead, the FBH system was able to reduce the COD to a level that was below the standard for discharge, 80 mg L−1 (ref. 40) and 120 mg L−1 (ref. 35), with the mean removal efficiency of 75%, which took less time (4 days, since each term lasted four days) and at a higher volume of effluent discharge. The whole amount of COD removed by the FBH and control systems for the study were 5466 mg and 1176 mg, respectively, which indicated that the FBH system had 4.65 times better removal.
3.4. Biomass and biochemical content changes
The biomass change in the fresh weight of FBH and control systems for the 16 days study is presented in Fig. 2(d). In general, both systems demonstrated almost similar growth and biomass increment. However, the final biomass in both systems was determined differently since the control system was a non-harvesting system, in which the macrophytes were only harvested at the end of the study, while the FBH system experienced an additional three times of harvesting during the study. Therefore, the final biomass in the control system represented the final harvested biomass, while the final biomass in the FBH system comprised the total accumulated biomass during the study (3 times plus final harvested biomass). Both systems started with the same fresh weight and ended with an almost identical fresh weight. The FBH system ended up with a total accumulated biomass of 215.00 ± 5.16 g, with 53% increment in fresh weight. The macrophytes' fresh weight in the control system went up to 215.95 ± 2.09 g, with 54% increment. Both systems achieved a significant difference in their biomass increment (p < 0.05).
In the case of the biochemical content, the change in the total carbohydrate content of the macrophytes in the systems at the end of the study is depicted in Fig. 2(e). It was found that the macrophytes in both systems had a decrement in total carbohydrate content. The initial total carbohydrate content of the employed macrophytes was determined to be 0.3930 ± 0.0315 g g−1 DW. For the control system, its content in the macrophytes declined to 0.2097 ± 0.0028 g g−1 DW. Meanwhile, the total intracellular carbohydrate content of the macrophytes in the FBH system fell to 0.2007 ± 0.0100 g g−1 DW. The decrement in both systems was statistically significant (p < 0.05).
Fig. 2(f) shows the total protein change of the macrophytes in the systems for the 16 days experiment. Generally, both systems exhibited similar total protein content increment. The utilised macrophytes had an initial total protein content of 0.1755 ± 0.0020 g g−1 DW. The total protein content in the control system eventually rose to 0.2251 ± 0.0030 g g−1 DW, with 28% of increment. The content in the FBH system went up to 0.2135 ± 0.0020 g g−1 DW, recording 22% increment in the total protein content. Both increments were statistically significant (p < 0.05).
The significant biomass increment in the fresh weight in both systems indicated the healthy growth of macrophytes in the systems, and the wastewater provided sufficient nutrients to support their development and growth. However, the percentage of fresh weight increment in the control system was found to be lower than the one in the S. polyrhiza monoculture system in the previous study.15 It could be due to a higher macrophyte density in the control system leading to crowding effect, which eventually limited the macrophytes' growth within the system. Plant growth can also slow down when more plants are being cultivated under the same medium (limited nutrients condition). Still, the control system showed a higher amount of fresh weight increment than the system from a previous study.15 This is because a greater quantity of macrophytes (initial culture inoculum) would tend to have a higher amount of growth. Harvesting did promote the growth of macrophytes. This deduction comes from the comparison of the systems' biomass data. Harvesting was periodically carried out in the FBH system. The fresh weight increment in the system was still similar to the control system even though the macrophytes remained would have reduced after each harvesting. The remaining macrophytes were implied to grow faster than the rate they did before harvesting in order to catch up with the fresh weight increment in the control system. Therefore, harvesting was inferred to have boosted the growth of macrophytes in the system. This was basically induced by less crowding of the macrophytes in the system.22 Hence, the harvesting rate of the macrophytes needs to be further optimised with respect to the macrophytes' growth rate to maintain the optimal macrophyte density in order to achieve its wastewater treatment goals. At the same time, it would allow greater biomass productivity of the macrophytes. The reasons for the total carbohydrate content decrement in the control and FBH systems were identical to the points presented by Ng et al.15 The macrophytes undergoing phytoremediation utilised those carbohydrates for metabolic processes, growth and producing offspring,59,60 and converted them to complex organic carbon, e.g., cellulose,60 or disseminated them into their daughter frond during multiplication.61 Furthermore, the utilised macrophytes were grown by micropropagation, with sucrose enrichment, so their initial carbohydrate content was considerably high.60,62,63 The high increment in the total protein content enhanced the macrophytes' nutritional value, which provides better quality feedstock for fish feed supplement64–66 and poultry diet.67
4 Conclusions
The FBH system was more feasible than the control system since it had higher treatment capacity with more superior treatment performance than the control system. It could treat a greater volume of wastewater, remove a higher amount of pollutants from wastewater, and meet the effluent standard limits, while maintaining the high removal efficiency throughout the terms. To be specific, the FBH system treated 30 L of wastewater and had 150% more treatment capacity, under a 4 day half wastewater replenishment, for the duration of 16 days study, compared to the system without fed batch. The amount of ammonia, phosphate, TSS and COD removed were generally higher (756.60 mg, 74.88 mg, 4365 mg and 5466 mg, respectively, with 2.54, 3.47, 5.20 and 4.65 times higher) than the control system. From the results of this study, the fed batch duration could be shortened to 3 days based on the data obtained, which may increase the treatment capacity, while maintaining low pollutant level in the discharged wastewater. The average removal efficiencies of ammonia, phosphate, TSS and COD throughout the terms in the FBH system were 81%, 76%, 87% and 75%, respectively. The summarised data of the treatment capacity and performance for the systems are shown in ESI Table S3.†
This study suggested that the S. polyrhiza monoculture system with fed batch and optimal harvesting is viable and effective in treating fish farm wastewater, as it could substantially and efficiently remove the dissolved nutrients and suspended solids within. The treated wastewater could reach beyond discharge limits, allowing the safe release to the receiving waters. It avoids pollution and reduces adverse effect towards the environment. Moreover, the treated water can be reused/recycled/recirculated for fish culture (e.g., catfish/other tolerant fish species) and agricultural purposes (e.g., irrigation of nearby paddy field). It decreases the water diversion from the ecosystems, saves potable water source and reduces wastewater discharge. The treated wastewater could also be applied for water flow recharge and augmentation to keep the streams away from impairment/drying due to water diversion, to revive and sustain the wildlife habitat in the downstream. Furthermore, the generated biomass from the system, which is high in protein content, could serve as feedstock for aquaculture, livestock and other energy-related applications.
CRediT author statement
Yin Sim Ng: methodology, validation, formal analysis, investigation, resources, data curation, writing – original draft, visualization. Derek Juinn Chieh Chan: conceptualization, resources, writing – review & editing, supervision, project administration, funding acquisition.
Conflicts of interest
There are no conflicts to declare.
Acknowledgements
This work is supported by the Long Term Research Grant Scheme (LRGS) (203/PJKIMIA/6720015 and 4411/S01) by Ministry of Higher Education, Malaysia. Yin Sim Ng acknowledges the award of USM Fellowship by Institute of Postgraduate Studies, Universiti Sains Malaysia for supporting his study and research work.
References
- FAO, The State of World Fisheries and Aquaculture 2018 – Meeting the sustainable development goals, Rome, 2018 Search PubMed.
- FAO, The State of World Fisheries and Aquaculture 2014 – Opportunities and challenges, Rome, 2014 Search PubMed.
- FAO, The State of World Fisheries and Aquaculture 2016 – Contributing to food security and nutrition for all, Rome, 2016, p. 200 Search PubMed.
- D. Miller and K. Semmens, Waste management in aquaculture, West Virginia University Extension Service Publication No. AQ02-1, USA, 2002, p. 8 Search PubMed.
- S. A. Castine, A. D. McKinnon, N. A. Paul, L. A. Trott and R. de Nys, Wastewater treatment for land-based aquaculture: improvements and value-adding alternatives in model systems from Australia, Aquac. Environ. Interact., 2013, 4, 285–300 CrossRef.
- S. A. Siddiqui, Wastewater treatment technology in aquaculture, World Aquaculture, 2003, pp. 49–52 Search PubMed.
- E. Pfeffer, The pollution of water by fish feeding, Dtsch. Tierarztl. Wochenschr., 1990, 97(7), 273–275 CAS.
- C. S. Tucker and J. A. Hargreaves, Environmental best management practices for aquaculture, John Wiley & Sons, 2009 Search PubMed.
- C. E. Boyd, C. Lim, J. Queiroz, K. Salie, L. D. Wet and A. McNevin, Best management practices for responsible aquaculture, USAID, 2008 Search PubMed.
- Y. S. Ng, N. I. S. Samsudin and D. J. C. Chan, Phytoremediation Capabilities of Spirodela polyrhiza and Salvinia molesta in Fish Farm Wastewater: A Preliminary Study, IOP Conf. Ser.: Mater. Sci. Eng., 2017, 206(1), 012084 CrossRef.
- S. C. Wuang, M. C. Khin, P. Q. D. Chua and Y. D. Luo, Use of Spirulina biomass produced from treatment of aquaculture wastewater as agricultural fertilizers, Algal Res., 2016, 15, 59–64 CrossRef.
- N. Uduman, Y. Qi, M. K. Danquah, G. M. Forde and A. Hoadley, Dewatering of microalgal cultures: a major bottleneck to algae-based fuels, J. Renewable Sustainable Energy, 2010, 2(1), 012701 CrossRef.
- D. Vandamme, Harvesting, thickening and dewatering processes, 2017 Search PubMed.
- Y. S. Ng and D. J. C. Chan, Phytoremediation capabilities of Spirodela polyrhiza, Salvinia molesta and Lemna sp. in synthetic wastewater: a comparative study, Int. J. Phytorem., 2018, 20(12), 1179–1186 CrossRef CAS.
- Y. S. Ng, Performance of Spirodela polyrhiza, Salvinia molesta and Lemna sp, in Phytoremediation of Fish Farm Wastewater, Masters thesis, Universiti Sains Malaysia, 2018 Search PubMed.
- D. An, Y. Zhou, C. Li, Q. Xiao, T. Wang, Y. Zhang, Y. Wu, Y. Li, D.-Y. Chao, J. Messing and W. Wang, Plant evolution and environmental adaptation unveiled by long-read whole-genome sequencing of Spirodela, Proc. Natl. Acad. Sci., 2019, 116(38), 18893–18899 CrossRef CAS.
- M. Ansal, A. Dhawan and V. Kaur, Duckweed based bio-remediation of village ponds: an ecologically and economically viable integrated approach for rural development through aquaculture, Livest. Res. Rural Dev., 2010, 22(7), 129 Search PubMed.
- A. M. D. d. Silva, L. d. C. Gomes and R. Roubach, Growth, yield, water and effluent quality in ponds with different management during tambaqui juvenile production, Pesqui. Agropecu. Bras., 2007, 42(5), 733–740 CrossRef.
- C. S. Tucker and J. A. Hargreaves, Biology and culture of channel catfish, Elsevier, 2004, vol. 34 Search PubMed.
- S. Iqbal, Duckweed aquaculture – Potentials, possibilities and limitations for combined wastewater treatment and animal feed production in developing countries, SANDEC Report No. 6/99, Swiss Federal Institute for Environmental Science & Technology, EAWAG, Duebendorf, Switzerland, 1999, p. 91 Search PubMed.
- M. R. Hasan and C. Rina, Use of algae and aquatic macrophytes as feed in small-scale aquaculture: a review, Food and Agriculture Organization of the United Nations (FAO), 2009 Search PubMed.
- P. Skillicorn, W. Spira and W. Journey, Duckweed aquaculture: a new aquatic farming system for developing countries, World Bank, Washington, DC, 1993 Search PubMed.
- J. Xu and G. Shen, Effects of harvest regime and water depth on nutrient recovery from swine wastewater by growing Spirodela oligorrhiza, Water Environ. Res., 2011, 83(11), 2049–2056 CrossRef CAS.
- P. Edwards, M. Hassan, C. Chao and C. Pacharaprakiti, Cultivation of duckweeds in septage-loaded earthen ponds, Bioresour. Technol., 1992, 40(2), 109–117 CrossRef CAS.
- G. Chen, Y. Fang, J. Huang, Y. Zhao, Q. Li, F. Lai, Y. Xu, X. Tian, K. He and Y. Jin, Duckweed systems for eutrophic water purification through converting wastewater nutrients to high-starch biomass: comparative evaluation of three different genera (Spirodela polyrhiza, Lemna minor and Landoltia punctata) in monoculture or polyculture, RSC Adv., 2018, 8(32), 17927–17937 RSC.
- M. Z. M. Said, D. D. Culley, L. C. Standifer, E. A. Epps, R. W. Myers and S. A. Boney, Effect of harvest rate, waste loading, and stocking density on the yield of duckweeds, in Proceedings of the World Mariculture Society, Wiley Online Library, 1979, pp. 769–780 Search PubMed.
- D. R. Hoagland and D. I. Arnon, The water-culture method for growing plants without soil, Circular. California Agricultural Experiment Station, 2nd edn, 1950, p. 347 Search PubMed.
- Y. S. Ng and D. J. C. Chan, Wastewater phytoremediation by Salvinia molesta, J. Water Process. Eng., 2017, 15, 107–115 CrossRef.
- C. Hoebler, J. L. Barry, A. David and J. Delort-Laval, Rapid acid hydrolysis of plant cell wall polysaccharides and simplified quantitative determination of their neutral monosaccharides by gas-liquid chromatography, J. Agric. Food Chem., 1989, 37(2), 360–367 CrossRef CAS.
- M. Dubois, K. A. Gilles, J. K. Hamilton, P. t. Rebers and F. Smith, Colorimetric method for determination of sugars and related substances, Anal. Chem., 1956, 28(3), 350–356 CrossRef CAS.
- N. von Wirén, A. Gojon, S. Chaillou and D. Raper, Mechanisms and Regulation of Ammonium Uptake in Higher Plants, in Plant Nitrogen, ed. P. J. Lea and J.-F. Morot-Gaudry, Springer Berlin Heidelberg, Berlin, Heidelberg, 2001, pp. 61–77 Search PubMed.
- Y. Y. Fang, O. Babourina, Z. Rengel, X. E. Yang and P. M. Pu, Ammonium and Nitrate Uptake by the Floating Plant Landoltia punctata, Ann. Bot., 2007, 99(2), 365–370 CrossRef CAS.
- Y. Y. Fang, O. Babourina, Z. Rengel, X. E. Yang and P. M. Pu, Spatial distribution of ammonium and nitrate fluxes along roots of wetland plants, Plant Sci., 2007, 173(2), 240–246 CrossRef CAS.
- E. F. George, M. A. Hall and G.-J. De Klerk, The components of plant tissue culture media I: macro-and micro-nutrients, Springer, 2008 Search PubMed.
- DOE, Environmental Quality (Sewage) Regulations 2009, in P.U.(A)432., NRE, PNMB, Malaysia, 2009 Search PubMed.
- USEPA, National Recommended Water Quality Criteria – Human Health Criteria Table, https://www.epa.gov/wqc/national-recommended-water-quality-criteria-human-health-criteria-table, June 20 Search PubMed.
- USEPA, Table of Regulated Drinking Water Contaminants, https://www.epa.gov/ground-water-and-drinking-water/table-regulated-drinking-water-contaminants#Inorganic, June 20 Search PubMed.
- WHO, Guidelines for drinking-water quality, WHO Press, Switzerland, 4th edn, 2011, p. 564 Search PubMed.
- USEPA, National Primary Drinking Water Regulations, https://www.epa.gov/ground-water-and-drinking-water/national-primary-drinking-water-regulations#Inorganic, August 20 Search PubMed.
- DOE, Environmental Quality (Industrial Effluent) Regulations 2009, in P.U.(A)434., NRE, PNMB, Malaysia, 2009 Search PubMed.
- C. I. Ullrich and A. J. Novacky, Extra- and Intracellular pH and Membrane Potential Changes Induced by K, Cl, H(2)PO(4), and NO(3) Uptake and Fusicoccin in Root Hairs of Limnobium stoloniferum, Plant Physiol., 1990, 94(4), 1561–1567 CrossRef CAS.
- R. L. Bieleski and A. Läuchli, Phosphate uptake, efflux and deficiency in the water fern, Azolla, Plant, Cell Environ., 1992, 15(6), 665–673 CrossRef CAS.
- J. B. Paterson, M. A. Camargo-Valero and A. Baker, Uncoupling growth from phosphorus uptake in Lemna: Implications for use of duckweed in wastewater remediation and P recovery in temperate climates, Food Energy Secur., 2020, 9(4), e244 Search PubMed.
- R. L. Mikkelsen and W. R. Director, A closer look at phosphorus uptake by plants. News and Views, (A regional newsletter published by the Potash & Phosphate Institute and the Potash & Phosphate Institute of Canada), 2005, p. 5051 Search PubMed.
- S. L. Tisdale, W. Nelson, J. D. Beaton and J. Havlin, Elements required in plant nutrition, Soil fertility and fertilizers, MacMillan Publ. Co., New York, 1993, pp. 48–49 Search PubMed.
- Y.-F. Lin, S.-R. Jing, D.-Y. Lee and T.-W. Wang, Removal of solids and oxygen demand from aquaculture wastewater with a constructed wetland system in the start-up phase, Water Environ. Res., 2002, 74(2), 136–141 CrossRef CAS.
- T. Yeh, T. Ke and Y. Lin, Algal growth control within natural water purification systems: macrophyte light shading effects, Water, Air, Soil Pollut., 2011, 214(1–4), 575–586 CrossRef CAS.
- M. J. Sadar, Turbidity science. Technical Information Series—Booklet no. 11, Hach Company, Loveland, CO, 1998, p. 26 Search PubMed.
- L. H. X. Daphne, H. D. Utomo and L. Z. H. Kenneth, Correlation between turbidity and total suspended solids in Singapore rivers, J. Water Sustainability, 2011, 1(3), 313–322 Search PubMed.
- Z. Amjad, The Science and Technology of Industrial Water Treatment, CRC Press, Boca Raton, FL, 2010 Search PubMed.
- Fondriest Environmental, I., Fundamentals of Environmental Measurements, http://www.fondriest.com/environmental-measurements/parameters/water-quality/turbidity-total-suspended-solids-water-clarity/, January 18 Search PubMed.
- R. Dowling and K. Stephen, The use of wetland plants in artificial wetlands in Queensland, Wetland for Water Quality Control, Townville, Australia, 1995 Search PubMed.
- M. Greenway and A. Woolley, in Changes in plant biomass and nutrient removal over 3 years in a constructed free water surface flow wetland in Cairns, 7th International conference on wetland system for water pollution control, Australia, 2000, 2000 Search PubMed.
- A. Kunihiko, Importance of aquatic macrophytes in controlling water quality of shallow lakes, in Proceedings of the 11th World Lakes Conference--Proceedings, 2006, 2006, pp. 143–147 Search PubMed.
- K. Moorhead and K. Reddy, Carbon and nitrogen transformations in wastewater during treatment with Hydrocotyle umbellata L, Aquat. Bot., 1990, 37(2), 153–161 CrossRef CAS.
- J. Vymazal, H. Brix, P. F. Cooper, R. Haberl, R. Perfler and J. Laber, Removal mechanisms and types of constructed wetlands, Constructed wetlands for wastewater treatment in Europe, 1998, pp. 17–66 Search PubMed.
- H. Brix, Gas exchange through the soil-atmosphere interphase and through dead culms of Phragmites australis in a constructed reed bed receiving domestic sewage, Water Res., 1990, 24(2), 259–266 CrossRef CAS.
- DOE, Malaysia Environmental Quality Report 2014, in NRE, Misas Advertising Sdn. Bhd., Malaysia, 2015 Search PubMed.
- T. Ohyama, T. Ikarashi, T. Matsubara and A. Baba, Behavior of carbohydrates in mother and daughter bulbs of tulips (Tulipa gesneriana), Soil Sci. Plant Nutr., 1988, 34(3), 405–415 CrossRef.
- BBC Photosynthesis – Uses of the sugar produced by photosynthesis, https://www.bbc.co.uk/education/guides/zcktw6f/revision/3, January 21 Search PubMed.
- I. Kim, Structural Differentiation of the Connective Stalk in Spirodela polyrhiza (L.) Schleiden, Appl. Microsc., 2016, 46(2), 83–88 CrossRef.
- R. Lemoine, Sucrose transporters in plants: update on function and structure, Biochim. Biophys. Acta, Biomembr., 2000, 1465(1), 246–262 CrossRef CAS.
- V. Gollagunta, J. W. Adelberg, J. Rieck and N. Rajapakse, Sucrose concentration in liquid media affects soluble carbohydrates, biomass and storage quality of micropropagated hosta, Plant Cell, Tissue Organ Cult., 2004, 77(2), 125–131 CrossRef CAS.
- E. Fasakin, A. Balogun and B. Fasuru, Use of duckweed, Spirodela polyrrhiza L. Schleiden, as a protein feedstuff in practical diets for tilapia, Oreochromis niloticus L, Aquacult. Res., 1999, 30(5), 313–318 CrossRef.
- R. Leng, J. Stambolie and R. Bell, Duckweed-a potential high-protein feed resource for domestic animals and fish, Livest. Res. Rural Dev., 1995, 7(1), 36 Search PubMed.
- M. S. Hassan and P. Edwards, Evaluation of duckweed (Lemna perpusilla and Spirodela polyrrhiza) as feed for Nile tilapia (Oreochromis niloticus), Aquaculture, 1992, 104(3–4), 315–326 CrossRef.
- A. Haustein, R. Gilman, P. Skillicorn, V. Vergara, V. Guevara and A. Gastanaduy, Duckweed, a useful strategy for feeding chickens: performance of layers fed with sewage-grown Lemnacea species, Poult. Sci., 1990, 69(11), 1835–1844 CrossRef.
Footnote |
† Electronic supplementary information (ESI) available: Additional tables, figures, equations and protocol details. See DOI: 10.1039/d0ra08088h |
|
This journal is © The Royal Society of Chemistry 2021 |
Click here to see how this site uses Cookies. View our privacy policy here.