DOI:
10.1039/C2RA20142A
(Review Article)
RSC Adv., 2012,
2, 5470-5484
Recent advances of ionic liquids in separation science and mass spectrometry
Received
24th January 2012
, Accepted 21st March 2012
First published on 23rd March 2012
Abstract
Ionic liquids (ILs) have drawn considerable interest for their use in various analytical techniques including chromatography, extractions, and mass spectrometry. This is largely due to the flexibility in tuning the physicochemical properties of ILs. There has been a significant increase in the number of publications over the last decade in which ILs have been employed as a chromatographic stationary phase, extraction solvent, or sorbent material in various preconcentration techniques. This review article highlights the recent advancements in the use of ILs in separation science including gas chromatography, high-performance liquid chromatography, capillary electrophoresis, extraction and microextraction techniques as well as mass spectrometry.
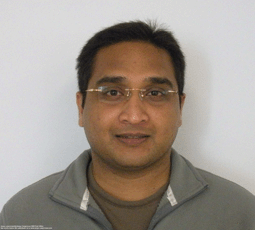 Manishkumar D. Joshi | Manishkumar D. Joshi received his B.Sc. and M.Sc. degrees in chemistry from South Gujarat University in 1999 and 2002, respectively. He obtained his M.S. degree in analytical chemistry from The University of Texas at Arlington in 2008. He joined the Ph.D. program in chemistry at The University of Toledo in 2008. His research involves the synthesis of new classes of ionic liquids and their applications in various extraction techniques. |
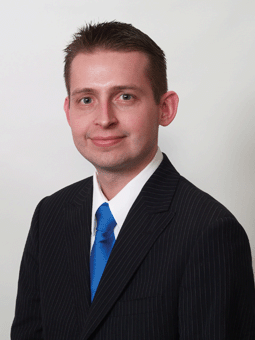 Jared L. Anderson | Jared L. Anderson obtained his B.S. degree in chemistry at South Dakota State University in 2000 and his Ph.D. in analytical chemistry at Iowa State University in 2005. He is currently a Professor of Chemistry at The University of Toledo. His research interests include the synthesis of new classes of ionic liquids and materials derived from polymeric ionic liquids, the use of ionic liquids as catalytic solvents, and the use of ionic liquids in all aspects of separation science including analytical extractions, purification, and chromatography. |
Introduction
Ionic liquids (ILs), also known as molten salts, consist of organic cations and organic/inorganic anions. According to a widely accepted definition, ILs are class of salts which are liquid below 100 °C whereas salts that are liquid at or below room temperature (i.e. 25 °C) are known as room temperature ionic liquids (RTILs). The first IL was reported by Gabriel and Weiner in 1888.1 The melting point for this ethanolammonium nitrate IL was reported to be 52–55 °C. The first RTIL, ethylammonium nitrate, was reported by Walden in 1914.2 The discovery of dialkylimidazolium chloroaluminate RTILs by Wilkes and co-workers in 1982 spearheaded the search for new classes of ILs based on organic cations.3 However, these RTILs were not highly stable in air and water due to hydrolysis of the chloroaluminate anion. Therefore, Wilkes and Zaworotko were the first to report the synthesis of air and water stable ILs with imidazolium or pyridinium cations and acetate or tetrafluoroborate anions.4 This was a significant discovery and the starting point for the current research aimed at synthesizing varying classes of RTILs and ILs as well as exploiting their unique properties. This expanded the use of ILs into different fields of research including synthesis,5 catalysis,6 electrochemistry3,7 chromatography,8 and extractions.9 The most common cations found in the literature are those of imidazolium, pyridinium, ammonium, phosphonium while common anions include bis-[(trifluoromethyl)sulfonyl]imide (NTf2−), hexafluorophosphate (PF6−), and halides, as shown in Fig. 1. There are numerous combinations of different cations and anions that are possible and it has been estimated that up to 1018 combinations exist.10
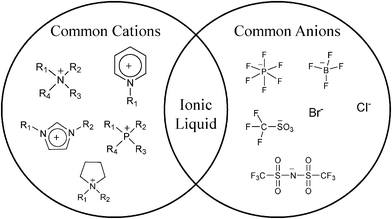 |
| Fig. 1 Common cations and anions of ionic liquids and room temperature ionic liquids. | |
ILs possess many unique physicochemical properties such as low vapour pressure, high thermal stability, high viscosity, good solvation ability, wide electrochemical windows, wide liquid range, and tunable polarity.11 These are general physicochemical properties of ILs and can vary significantly in every IL depending upon the combination of cations and anions employed. It is very common to functionalize the cation or anion to obtain desired properties. Tuning of these properties makes ILs unique compounds for applications in different areas within analytical chemistry. ILs generally possess low or negligible vapor pressure. However, there is literature precedence in which several 1-alkyl-3-methylimidazolium bis-[(trifluoromethyl)sulfonyl]imide ILs were vacuum distilled at a very low rate when the temperature was raised to 300 °C under reduced pressure of 10−4 bar.12 The high thermal stability of many ILs make them ideal candidates as stationary phases in gas chromatography (GC). The thermal stability was greatly improved by the generation of dicationic and tricationic ILs.11a,13 The thermal stability of ILs often depends on the anion present in the IL. For example, the 1-butyl-3-methylimidazolium chloride (BMIM-Cl) IL can undergo thermal degradation when the temperature is raised to 150 °C.14 The thermal stability can be significantly increased when the anion is changed to NTf2−. The knowledge of solvent properties of ILs and RTILs is necessary as they have been used as stationary phases in GC as well as solvents for synthesis and extraction. Different models have been used for evaluating solvent properties of ILs such as solvatochromic methods,15 Rohrschneider–McReynolds method,11b and the Abraham solvation parameter model.16 Among all of the aforementioned models, the Abraham solvation parameter model is widely used for characterizing ILs based on their multiple solvation interactions. Anderson et al. used it for characterization of 17 different RTILs.16a The solvation properties of different ionic liquids were reviewed by Poole et al.17 Several recent reviews that have been published relating the application of ILs in various analytical techniques.18 In this review, we focus on the use of ILs in the fields of chromatography, extractions, and mass spectrometry.
Gas chromatography (GC)
GC is widely used for the separation of small polar and nonpolar molecules. Separation of these molecules can be very challenging and often requires specially designed stationary phases capable of exhibiting high selectivity. An ideal GC stationary phase possesses high thermal stability, minimum bleeding, broad liquid temperature range, high selectivity, and high separation efficiency.11a ILs have gained popularity as stationary phases in GC due to the fact that they can be easily tailored to meet many of the aforementioned requirements. Functionalization of the cation or changing the anion can dramatically change the selectivity or separation capacity for the analyte of interest. It is also well known that ILs possess a “dual nature” separation selectivity.11 The dual nature of ILs allows them to solvate both polar molecules as well as nonpolar molecules. In GC, this provides them the capability of separating polar molecules like a polar stationary phase and nonpolar molecules like a nonpolar stationary phase. The first use of bivalent metal (Mn, Co, Ni, Cu, Zn) stearate ionic liquids as a GC stationary phase was demonstrated by Barber et al. in 1959.19 Gordon et al. showed the separation of different organic molecules using various quaternary ammonium salts with bromide, picrate and nitrate anions.20 Unfortunately, these compounds possessed narrow liquid ranges (< 140 °C) and exhibited poor separation efficiencies. After almost two decades, Pacholec et al. explored the use of the triethylammonium nitrate ionic liquid as a GC stationary phase which possessed a liquid temperature range of 40–120 °C.21 This IL was able to separate compounds, but the efficiency was poor compared to commercial Carbowax columns. The same authors demonstrated the use of the 1-ethylpyridinium bromide IL as a GC stationary phase; this IL exhibited comparable separation efficiency to commercial GC columns in addition to good thermal stability.22 Poole et al. evaluated several classes of ILs, namely, tetra-n-hexylammonium benzoate, tetra-n-heptylammonium chloride, tetra-n-butylammonium tetrafluoroborate, tri-n-butylbenzylphosphonium chloride, l-methyl-3-ethylimidazolium chloride, and sodium isovalerate as GC stationary phases.23 Despite a wide liquid temperature range (as high as 290 °C), the maximum operating temperature achieved for most of these ILs was below 160 °C. A significant improvement in the thermal stability and selectivity was achieved by Anderson and Armstrong in 2003.11a Two new ILs, namely, 1-benzyl-3-methylimidazolium trifluoromethanesulfonate and 1-(4-methoxyphenyl)-3-methylimidazolium trifluoromethanesulfonate were synthesized and studied as GC stationary phases. These new ILs exhibited good thermal stability up to 260 °C along with symmetrical peak shapes for the separation of polar and nonpolar analytes. The same authors introduced vinylimidazolium-based monocationic and dicationic ILs which were coated and immobilized on to the capillary wall to improve the wetting ability of the stationary phase.13a Huang et al. synthesized PEG-linked geminal dicationic ILs and examined them as a GC stationary phase for the separation of variety of molecules. They found good thermal stability for these robust GC stationary phases along with high selectivity and efficiency. Unsymmetrical dicationic and trigonal tricationic ILs were synthesized by Sharma et al.24 A new class of trigonal tricationic ILs were generated where different cores such as benzene, mesitylene, triethylamine and tri-(2-hexamido) ethylamine were functionalized using imidazolium and phosphonium cations.25 These tricationic ILs exhibited high thermal stability and increased operating temperatures up to 335 °C. The best selectivity and efficiency was obtained using ILs possessing the tri-(2-hexamido)ethylamine core. 1-vinyl-3-hexylimidazolium PF6−/NTf2− were grafted on to the polymethylsiloxane capillary and their separation ability was evaluated by Wei et al.26 Recently, β-cyclodextrin was modified using imidazolium moiety to generate 2,3,6-ethyl-6-(N-ethylimidazole)-β-cyclodextrin tetrafluoroborate (BF4−) and 2,3,6-ethyl-6-(N-ethylimidazole)-β-cyclodextrin (NTf2−) ILs.27 The separation of alkanes, alcohols, and aromatic positional isomers such as xylene, nitrotoluene, dichlorobenzene and cresol was achieved. Poole et al. recently extensively reviewed ionic liquids as GC stationary phases.28
RTILs were also employed in the generation of chiral stationary phases for enantiomeric separations using GC. There are two different ways to achieve chiral separations using ILs. The first approach is to synthesize chiral ionic liquids and employ them directly as the chiral stationary phase. The second method is to dissolve the chiral selector into an IL and use the mixture as the stationary phase. Ding et al. synthesized N,N-dimethylephedrinium-based ILs for the first time and separated four classes of chiral compounds, namely alcohols, sulfoxides, epoxides, and acetylated amines.8 The 1-butyl-3-methylimidazolium chloride IL was used to dissolve permethylated-β-cyclodextrin and dimethylated-β-cyclodextrin and examined for the enantiomeric separation using GC.29 High efficiencies were observed but the formation of an inclusion complex between the imidazolium cation and β-cyclodextrin resulted in limited enantioselectivity. To overcome this challenge, ionic cyclodextrins were synthesized through functionalization using imidazolium or phosphonium cations.30 These ionic cyclodextrins were dissolved in ILs which possessed bulky cations. Due to the presence of charge on the cyclodextrin and the use of bulky ILs, the inclusion complex formation was inhibited. These new GC columns exhibited very high efficiency, good thermal stability, and broad enantioselectivity. Recently, Tran et al. dissolved three different cavitands in an IL and used these mixtures as a GC stationary phase.31 Various isotopic compounds such as aromatic hydrocarbons, ethers, alcohols, and pyridine were separated. There are several other reports where ILs have been employed as a solvent to dissolve single-walled carbon nanotubes,32 fullerenes,33 and metallomesogens.34
High performance liquid chromatography (HPLC)
Ionic liquids have been incorporated in two different ways for improving chromatographic separations using HPLC. These include using the ILs as additives to the mobile phase or immobilizing them to the stationary phase. The former approach is found to be more convenient and popular. Poole et al. employed ILs as additives where a series of alkylammonium-based ionic salts were synthesized with nitrate and isocynate anions.35 Only four of these ionic salts were liquid at room temperature. These ILs were applied as additives by mixing with organic solvents, such as methanol, and used as mobile phases in HPLC. The concentration of these ILs was maintained in the range of 0.5–1.5% due to the fact that they significantly increased the viscosity of the mobile phase resulting in elevated back pressure. They also evaluated the solvent properties of these ILs and concluded that solvent selectivities were controlled by proton acceptor-donor and dispersive interactions depending on the size of cation and nature of the anion.36 ILs containing the nitrate anion act more like water, unlike ILs with the isocynate anion which act more like organic solvents. Waichigo et al. also evaluated the feasibility of using ILs as mobile phases in HPLC.37 They synthesized the ethylammonium acetate IL and prepared aqueous mobile phases where the amount of IL was varied from 20–80% by volume.38 A significant decrease in the retention of acetophenone on a C18 column was observed. Grossman et al. showed the use of 5% methylammonium formate (MAF) IL as the mobile phase additive in an aqueous mobile phase to obtain baseline separation of pyridoxine, thiamine, and nicotinamide.39 There are certain disadvantages when using ILs as additive in HPLC mobile phases. These include: high viscosity which can produce high back pressure; interference in UV detection due to the fact that many ILs possess UV chromophores; their extensive use can corrode HPLC components; and ILs can be expensive. However, literature precedence has demonstrated the utility of ILs when used in small quantities. It is well known that the surface of silica has many silanol groups which can interact strongly with positively charged analytes. Due to electrostatic interactions, charged analytes will retain longer and give tailing peaks. When ILs were used as mobile phase additives, due to the presence of cations, these negatively charged silanol groups are masked. The separation mechanism is complex when ILs are used as additives due to the fact that multiple ions compete for residual silanol groups on the silica surface. Zhang et al. separated chatecholamine by reversed phase HPLC using 1-alkyl-3-methylimidazolium and N-butyl pyridinium-based ionic liquids as additives.40 Berthod and co-workers used 1-alkyl-3-methylimidazolium tetrafluoroborate ILs as an additive in the mobile phase for HPLC and compared the ILs to triethylamine for separating β-blockers.41 They observed better separation of analytes when ILs were used as additives. The cations of ILs interact strongly with deprotonated silanol groups and alkyl chains on the cation interact further with analytes to add dispersive interactions. This dual nature of ILs in liquid chromatography was studied by Berthod et al.42 Kaliszan and co-workers studied the effect of ILs on suppressing active silanol groups and improving separation selectivity and efficiency.43 The same group also demonstrated the use of imidazolium-based tetrafluoroborate ILs as mobile phase additives.44 He et al. separated ephedrines on a C18 HPLC column using 1-alkyl-3-methylimidazolium tetrafluoroborate ILs.45 Rao et al. showed the separation of antiretroviral drugs using reversed phase HPLC where 1-butyl-3-methylimidazolium tetrafluoroborate and 1-ethyl-3-methylimidazolium tetrafluoroborate ILs were used as additives instead of the conventional triethylamine additive.46 Further, imidazolium-based ILs have been used as an additive in HPLC for the separation of amines and heterocyclic aromatic amines.47 Overall, it can be concluded that ILs are more effective additives then conventional organic additives when used in small quantities. They can provide better separation efficiency and often enhance selectivity due to their ability to undergo multiple interactions with the analytes as well as masking the acidic silanol groups on the silica surface. The use of ILs as masking agents for acidic silanol groups results in the efficient separation of basic compounds.
The shortcoming of ILs interfering during detection can be mitigated by immobilizing ILs on the surface of silica. Liu et al. first reported the generation of anion exchange media where imidazolium-based ILs were immobilized on silica.48 These IL-based columns successfully separated different analytes such as amines, nucleotides as well as anions. The columns exhibited anion exchange capability along with reverse phase characteristics. Stalcup et al. developed HPLC stationary phases by immobilizing n-butylimidazolium bromide ILs to the silica surface using an alkyl tether.49 The retention properties of this stationary phase were similar to the phenyl-based stationary phase. Recently, peptides were separated using butyl imidazolium bromide-modified silica in the isocratic mode. The separation was achieved in less than five minutes and no ion-pairing reagent was required.50 Qiu et al. generated ionic liquid-based stationary phases by modification of silica particles using 1-allyl-3-(butyl-4-sulfonate) imidazolium trifluoromethanesulfonate (TfO) and 1-allyl-3-butyl imidazolium bromide ionic liquids.51 Separation of inorganic cations and anions was achieved using water as the mobile phase. Further investigations involving these IL-based stationary phases have shown that the 1-allyl-3-(butyl-4-sulfonate) imidazolium-based stationary phase demonstrates significantly higher π–π and ion-dipole interactions and separates PAHs in reversed phase HPLC.52 The same authors demonstrated the synthesis of poly(ionic liquid)-grafted silica phase based on ionic self-assembly technology.53 Methyl orange dye was used as counter anion which exhibited very high selectivity towards shape-constrained isomers such as pyrene, triphenylene, benzo[α]anthracene, and chrysene.
Capillary electrophoresis (CE)
Due primarily to its low sample consumption and high separation efficiency, CE has emerged in recent years as a powerful analytical technique. Open tubular capillary columns possessing an inner diameter of 25–50 μm are often employed in CE separations. Due to the presence of acidic silanol groups on the surface of the capillary, an electroosmotic flow (EOF) is generated, resulting in a separation of analytes dependent on the electrophoretic mobility of the ions. Since ILs are composed of cations and anions, they serve as good electrolytes and possess the ability to control the EOF and separation of analytes. They have been employed as running buffer and capillary wall modifiers.54
Yanes et al. reported the use of 1-alkyl-3-methylimidazolium-based ILs with tetrafluoroborate or hexafluorophosphate anions as buffer modifiers for the separation of polyphenol compounds obtained from grape seed extracts.55 They found better separation using the 1-ethyl-3-methylimidazolium BF4− IL compared to 1-butyl-3-methylimidazolium BF4− IL. ILs have also been employed as additives for the separation of carboxylates,56 proteins,57 and bioactive flavone derivatives.58 Recently, 1-ethyl-3-methylimidazolim chloride (EMImCl), 1-butyl-3-methylimidazolim chloride (BMImCl), 1-ethyl-2,3-dimethylimidazolium ethyl sulfate (EdiMImEtSO4), 1-ethyl-3-methyl imidazolium octylsulfate (EMImOctSO4) and 1-dodecyl-3-methylimidazolium chloride (C12MImCl) were used as modifiers for the separation of uncharged sucrose, D-glucose, D-fructose, and D-ribose using CE.59
The separation of hydrophobic analytes is a challenge as they are often sparingly soluble in commonly employed aqueous buffers. This challenge can be circumvented using nonaqueous CE, in which organic solvents are used as the running buffer. Due to the high solubility of ILs in organic solvents, they have been also exploited as modifiers in nonaqueous CE. Vaher et al. used 1-alkyl-3-imidazolium-based ILs for the separation of polyphenol compounds in acetonitrile.56 The same authors demonstrated the use of imidazolium-based ILs for the separation of different dyes in nonaqueous CE.60 Mwongela and co-workers reported the separation of chiral as well as achiral analytes using polymeric surfactants dissolved in imidazolium-based ILs.61 Chiral ILs based on choline derivatives (R)(−)1-Hydroxy-N,N,N-trimethylbutan-2-aminium and (R)(−)2-Hydroxy-N,N,N-trimethyl-1-phenylethanaminium (PhChol) paired with the NTf2− anion were also employed with cyclodextrin to achieve chiral separation of anti-inflammatory drugs.62
Besides using ILs as running buffer modifiers, efforts have been made to generate covalently modified capillaries. Qin et al. modified the capillary surface by immobilizing the imidazolium-based ionic liquid to separate metabolites in human serum.63 Due to the IL modification, the EOF was reversed while enhanced resolution was achieved. The same authors demonstrated the separation of mono and bivalent metal ions using 1-ethyl-3-methylimidazolium hydroxide IL as running buffer modifier.54a Imidazolium-based ILs and zwitterionic salts were immobilized on the silica capillary and the separation of inorganic ions and alkylphosphonic acids/monoesters was enhanced significantly.64
Extractions
Due to the structural tunability of ILs, they can be designed to preconcentrate analytes of interest from the aqueous phase and phase separate from water. ILs have been applied in a number of sample preparation methods including liquid–liquid extraction (LLE), single drop microextraction (SDME), dispersive liquid–liquid extraction (DLLME), and solid phase microextraction (SPME). A schematic representation of the different extraction techniques using ILs as the solid or liquid extraction phase is shown in Fig. 2.
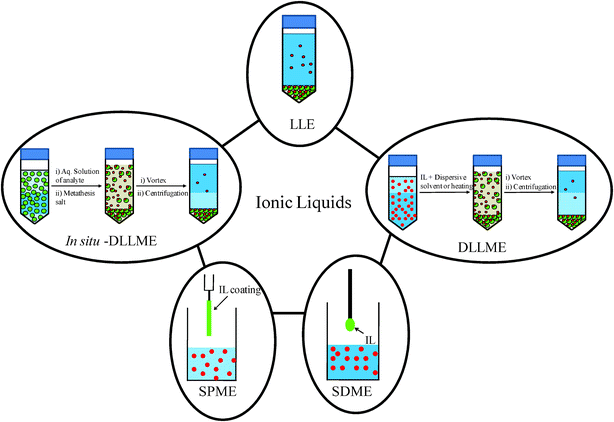 |
| Fig. 2 Different extraction and microextraction techniques using ionic liquids as the solid or liquid extraction phase. | |
Liquid–liquid extraction
Liquid–liquid extraction (LLE) is the most widely employed extraction technique often used to extract analytes from an aqueous phase. Organic solvents such as hexane, chloroform, and ethers are often used as the extraction phase. These solvents are highly combustible, often toxic, and volatile in nature. There is a need for new extraction media which can replace conventional solvents thereby making the approach more environmentally friendly. Huddleston and co-workers first evaluated the 1-butyl-3-methylimidazolium hexafluorophosphate ([BMIM] [PF6]) ionic liquid as a novel extraction solvent for nineteen different compounds including acids, alcohols and bases as well as benzene and its derivatives in an attempt to understand the partitioning behavior of these analytes.65 A comparison of Pil–w, the partition coefficient of analyte between IL and water, and Po–w, the partition coefficient of analyte between octanol and water, was conducted. In follow up work, the same authors demonstrated change in the partitioning behavior of thymol blue dye based on the charge on the molecule as the pH is varied.66 At low pH, the thymol blue dye exists as zwitterions and partitions to the IL phase. At higher pH, thymol blue exists in a monoanion form and partitions to the aqueous phase. They observed higher partitioning of uncharged analytes to the IL phase compared to charged molecules. Carda-Broch and co-workers also investigated the partitioning behavior of 38 different compounds in a [BMIM] [PF6]/water system.14 It was concluded that compounds with acidic functional groups partitioned more to the water phase and less to the IL phase; amines however, exhibited a reversed trend. Neutral or zwitterionic compounds exhibited similar trends in both systems.
Dai et al. demonstrated the use of imidazolium-based ILs with PF6− and NTf2− anions as extraction solvents for metal ions using crown ethers as extractants.67 Large distribution coefficients for strontium nitrate were obtained. In another study, N-alkyl aza-18-crown-6 was synthesized and compared with dicyclohexano-18-crown-6 for the extraction of different metal ions such as Sr2+, Na+, K+, and Cs+.68 It was found that N-alkyl aza-18-crown-6 exhibited higher selectivity for bivalent ion Sr2+ as opposed to dicyclohexano-18-crown-6 which exhibited high selectivity for K+. Also, the selectivity of ions varied based on the alkyl chain present on the imidazolium-based NTf2− ILs. In another study, the extraction of different metal ions (Na+, K+, Sr+ and Cs+) was achieved from the aqueous phase using solutions containing calix[4]arene-bis(tert-octylbenzo-crown-6) in N-alkyl-N-methylimidazolium bromide and N-alkyl-N-methylimidazolium NTf2−.69 Higher distribution coefficients of metal ions were obtained using imidazolium cations with short alkyl chain substituents but limited the solubility of calix[4]arene-bis(tert-octylbenzo-crown-6). 1-alkyl-3-methylimidazolium hexafluorophosphate ILs were used in place of chloroform to dissolve calix[4]arene for the extraction of silver ions.70 Extraction of lanthanides and bivalent metal ions was achieved using 1-butyl-3-methylimidazolium bis-[(trifluoromethyl)sulfonyl]imide as the extraction solvent and 2- thenoyltrifluoroacetone and 18-crown-6 as extractants.71 Extraction of Zn(II) from a hydrochloric acid solution was achieved by the trihexyl(tetradecyl)phosphonium chloride IL using a polymer membrane.72 In a recent study, 1-ethyl-3-(4-sulfobutyl)imidazolium bis-[(trifluoromethyl)sulfonyl]imide was used as extractant and catalyst for the desulfurization of dibenzothiophene (DBT) in octane.73 Wei et al. utilized BMIM-PF6 for the extraction of gold nanoparticles and gold nanorods of desired size and shape without the use of a thiol capping agent.74 Recently, Bica and co-workers exploited three different ILs, namely, 1-butyl-3-methylimidazolium chloride, 1-allyl-3-methylimidazolium chloride, and 1-ethyl-3-methylimidazolium acetate to dissolve orange peels for the extraction of the essential oil limonene.75 These aforementioned ILs are also known to dissolve various types of biomass such as cellulose, chitin, and wood. ILs were also exploited for the extraction of biomolecules including proteins and DNA. Wang et al. for the first time showed the extraction of double-stranded DNA using the IL [BMIM][PF6].76 The extraction efficiency of DNA was increased as the length of an alkyl chain on the imidazolium cation was increased. The use of ILs for the extraction of DNA avoids the use of the existing phenol/chloroform method and circumvents the denaturation of DNA. Further use of ILs in the extraction of proteins has been reported.77 Lu et al. performed a thermodynamic study on the partitioning behaviour of cytochrome c in a biphasic system consisting of IL and water.78
Recently, microwave-assisted extractions (MAE) of various biological samples have gained momentum. The use of ILs as the extractant in MAE increases the extraction efficiency and decreases the extraction time. Due to their high thermal stability and negligible vapor pressure, some ILs may be more environmentally benign than organic solvents. Yansheng et al. demonstrated the use of two different ILs N, N-dimethyl-N-(2-hydroxyethoxyethyl)ammonium propionate (DMHEEAP) and N, N-dimethyl(cyanoethyl)ammonium propionate (DMCEAP) in MAE for the extraction of lactones from traditional Chinese herbs.79 Extraction equilibrium was achieved in 1 and 5 mins using DMHEEAP and DMCEAP, respectively. Various imidazolium-based ILs have been employed in the MAE of essential oils,80 alkaloids,81 polyphenolic compounds,82 and podophyllotoxin83 from different medicinal plants or fruits. Pino et al. demonstrated the use of 1-hexadecyl-3-methylimidazolium bromide IL aggregates for the MAE of PAHs from certified harbour sediment84 and toasted cereals.85 Gao et al. achieved MAE of phenylurea and triazine herbicides from milk.86
Liquid phase microextraction (LPME)
The conventional LLE method is tedious, time consuming, and uses high volumes of solvent. Therefore, a driving force in sample preparation is the development of methods that are fast, cheap, and compatible with current separation methods such as HPLC and GC. Miniaturized sample preparation techniques based on LLE, often referred to as liquid phase microextraction (LPME), emerged during the 1990s. In LPME, the analyte is extracted from a large volume of the aqueous sample to the extractant phase, also called the acceptor phase. LPME was first introduced by Liu and Dasgupta in 1996.87 This new liquid phase microextraction technique, called drop-in-drop, was successfully employed for the extraction of sodium dodecyl sulphate from water using chloroform. A small volume of chloroform (1.3 μL) was used as the water immiscible phase and immersed into the flowing aqueous drop to extract the analyte.87 Since the early report, several other microextraction techniques including single drop microextraction (SDME) and hollow fiber microextraction techniques have been developed. He et al. developed dynamic LPME in which 2 μL of organic solvent was used to extract chlorobenzenes from water using a microsyringe.88 A very thin film was coated on the wall of the syringe when organic solvent was withdrawn. This was followed by the withdrawal of an aqueous sample solution which allowed analytes to partition to and preconcentrate into the organic film. The higher enrichment factors and shorter extraction times were observed for dynamic LPME compared to static LPME. However, poor reproducibility was reported for dynamic LPME compared to static LPME due to the lack of automation for sampling. Overall, LPME possesses many advantages including rapid extraction, utilizes low volumes of the extraction phase, has no carry over effect, and is highly cost effective. As designer solvents, ILs possess favorable properties such as high viscosity, tuneable hydrophobicity, and nonvolatility which make them ideal candidates for LPME.89 Further, 1-hexyl-3-methylimidazolium tris(pentafluoroethyl)trifluorophosphate ([HMIM][FAP]) was employed in IL-based hollow fiber supported liquid phase microextraction (HF-LPME) of UV filters by Ge et al.90 Similarly, 1-octyl-3-mythylimidazolium hexafluorophosphate IL has been applied in HF-LPME for the extraction of sulfonamides from environmental samples.91
Single drop microextraction (SDME)
In SDME, a very small volume (microlitre) of the extraction solvent is used to preconcentrate analytes of interest from the aqueous phase. It can be performed in two different modes, namely direct-immersion SDME and headspace SDME. Jeannot and Cantwell demonstrated the extraction of 4-methylacetophenone into an 8 μL microdroplet of octane which was hung from a Teflon rod and immersed into the aqueous phase. The drop was then withdrawn into the Teflon rod from the aqueous phase and injected to GC using a microsyringe.92 The disadvantage of this approach is that extraction and analysis must be performed separately. This problem was addressed by the same group through the introduction of a microsyringe capable of accommodating the droplet.93 A droplet of n-octane with a volume of 1 μL was suspended into an aqueous solution from the tip of a microsyringe to perform the extraction of malathion, 4-methylacetophenone, 4-nitrotoluene, and progesterone.
The analysis of volatile and semivolatile compounds can be performed using headspace SDME, allowing this technique to emerge as an inexpensive, convenient, and precise sample preparation method. Theis et al. reported a headspace SDME method using 1-octanol as an extractant for the preconcentration of benzene, toluene, ethylbenzene, and o-xylene (BTEX).94 The biggest challenge with headspace-SDME lies with the stability of the microdroplet on the tip of the syringe. The choice of the extraction solvent is also limited due to the low viscosity, low surface tension, and high volatility of many organic solvents.
Many of the aforementioned limitations can be overcome by employing ILs as extraction solvents in SDME. The first use of an IL solvent in HS-SDME was demonstrated by Liu et al.89 Three different ILs, namely 1-butyl-3-methylimidazolium hexafluorophosphate (BMIM-PF6), 1-hexyl-3-methylimidazolium hexafluorophosphate (HMIM-PF6), 1-octyl-3-methylimidazolium hexafluorophosphate (OMIM-PF6), were employed. The extraction of polycyclic aromatic hydrocarbons (PAHs) was achieved using a microdroplet volume of 3 μL. Compared to 1-octanol, higher enrichment factors were obtained due to the larger drop size of the IL which can be sustained on the tip of the syringe for longer periods of time. Due to their desirable microdroplet stability, imidazolium-based ILs possessing the PF6− anion were often used as the extraction solvent for SDME. However, it is now known that the PF6− anion is hydrolytically unstable and is not conducive for use during long extraction times. In an effort to overcome these limitations, Yao et al. explored a new class of ILs possessing the tris(pentafluoroethyl)trifluorophosphate (FAP) anion paired with imidazolium, phosphonium, and pyrrolidinium cations.95 Due to higher hydrophobicity and enhanced hydrolytic stability, microdroplet volumes of 10 μL were employed and allowed for high enrichment factors of PAHs. Highest extraction efficiencies were achieved using the trihexyl(tetradecyl)phosphonium FAP and 1-hexyl-3-methylimidazolium FAP ILs for PAHs and small polar molecules, respectively. Aguilera-Herrador and co-workers developed a removable interface to couple IL-SDME directly to GC-MS facilitating the introduction of analytes to the GC column while preventing IL from entering the column.96 After extraction, the analytes were desorbed from the IL microdroplet using high temperature and transferred to the GC column. Several other applications involving ILs in SDME are listed in Table 1.
Table 1 Applications using single drop microextraction with various IL-based extraction solvents. Abbreviations used in the table are as follows: [CnMIM][PF6] (1-alkyl-3-methylimidazolium hexafluorophosphate), [C6MIM][NTf2] (1-hexyl-3-methylimidazolium bis-[(trifluoromethyl)sulfonyl]imide, [HMIM][FAP] (1-hexyl-3-methylimidazolium tris(pentafluoroethyl)trifluorophosphate), [PH3T] [FAP] (trihexyl(tetradecyl)phosphonium tris(pentafluoroethyl)trifluorophosphate), [BMPL] [FAP] (1-butyl-1-methylpyrrolidinium tris(pentafluoroethyl)trifluorophosphate), [HNH2MPL] [FAP] (1-(6-amino-hexyl)-1-methylpyrrolidinium tris(pentafluoroethyl)trifluorophosphate), MALDI-TOF (Matrix assisted laser desorption ionization-Time-of-flight), ETAAS (Electrothermal atomic absorption spectrometry), CE (Capillary electrophoresis), ICP-MS (Inductively coupled plasma mass spectrometry), GC-MS (Gas chromatography mass spectrometry), GC-FID (Gas chromatography flame ionization detection), HPLC-PDA (High performance liquid chromatography-photo diode array), HPLC-DAD (High performance liquid chromatography-diode array detection)
Ionic Liquid |
Analyte/Matrix |
Analysis method |
LOD |
Ref. |
[C6MIM][NTf2] |
2,4,6-trichloroanisole/water and wine |
Ion mobility spectrometry |
0.2 ng L−1 |
135
|
[C4MIM][PF6] |
Pathogenic bacteria/water |
MALDI-TOF |
106 cfu mL−1 |
136
|
[C4MIM][PF6] |
Methylcyclopentadienyl-manganese tricarbonyl (MMT)/Gasoline & water |
ETAAS |
10.0 ng L−1 |
137
|
[C4MIM][PF6] |
Copper/Food & water |
UV-VIS |
0.15 μg L−1 |
138
|
[CnMIM][PF6] (n = 4, 6, 8) |
A toxic alkaloid—colchicines/water |
CE-UV |
0.25 μg mL−1 |
139
|
[C6MIM][PF6] |
Aromatic amines (2-nitroaniline, 3-chloroaniline, 4-bromoaniline)/water |
LC-UV |
1–2.5 μg L−1 |
140
|
[C6MIM][PF6] |
Co(II) ions as an ammonium pyrrolidinedithiocarbamate (APDC) complex/water |
ETAAS |
0.04 μg L−1 |
141
|
tetradecyl(trihexyl)phosphonium chloride (CYPHOS IL 101) |
Hg species/Sea water, fish tissues, hair and wine |
ETAAS |
10 ng L−1 |
142
|
[C8MIM][PF6] |
Carbonyl compounds/water |
HPLC-UV |
0.04–2.03 ng mL−1 |
143
|
[CnMIM][PF6] (n = 4, 6, 8) |
Phenols/water |
CE-UV |
<0.05 μg mL−1 |
144
|
[C4MIM][PF6] |
Tributyltin (TBT) and triphenyltin (TPhT)/water |
HPLC-Fluorimetry |
0.62 μg L−1 |
145
|
[C6MIM][PF6] |
UV filters/water |
HPLC-UV |
0.06–3.0 μg L−1 |
146
|
Tetradecyl(trihexyl)phosphonium chloride (CYPHOS IL 101) |
Lead/water |
ETAAS |
3.2 ng L−1 |
147
|
[C4MIM][PF6] |
Lead/water & food sample |
ETAAS |
0.015 μg L−1 |
148
|
[C8MIM][PF6] |
Trihalomethanes/water |
GC-ion mobility spectrometry |
0.1–0.9 ng mL−1 |
149
|
[HMIM] [FAP], [PH3T] [FAP], [BMPL] [FAP] [HNH2MPL] [FAP] |
PAHs/water |
HPLC-UV |
0.03–1.2 μg L−1 |
95
|
[C8MIM][PF6] |
Chlorobenzene derivatives/waste water sample |
GC-FID |
0.1–0.5 μg L−1 |
150
|
[C4MIM][PF6], [C6MIM][PF6] |
Hg species (MeHg+, EtHg+, PhHg+ and Hg2+)/water |
HPLC-PDA |
1.0–22.8 μg L−1 |
151
|
[C6MIM][PF6] |
Chlorobenzenes/water |
GC-MS |
1 and 4 ng L−1 |
152
|
[C4MIM][PF6] |
Manganese/water |
ETAAS |
0.024 μg L−1 |
153
|
[C4MIM][PF6] |
Co, Hg and Pb/water & human serum |
ICP-MS |
1.5, 9.8 and 6.7 pg mL−1 |
154
|
[C8MIM][PF6] |
Trihalomethanes (THM) (chloroform, bromodichloromethane, dibromochloromethane, bromoform) |
GC-MS |
0.5–0.9 μg L−1 |
155
|
[C4MIM][PF6] [C8MIM][PF6] |
Phenols/water |
GC-FID |
0.1–0.4 ng mL−1 |
156
|
[C8MIM][PF6] |
Benzene, toluene, ethylbenzene and xylenes isomers (BTEX)/water |
GC-MS |
20–91 ng L−1 |
157
|
[C4MIM][PF6] |
Aromatic amines (1-naphthylamine, N,N-dimethylaniline, diphenylamine)/water |
HPLC-UV |
0.09–0.38 μg L−1 |
158
|
[C4MIM][PF6] |
Dichloromethane, p-xylene, n-undecane/water |
GC-MS |
5.6, 15.6, 7.9 ng mL−1 |
96
|
[C4MIM][PF6], [C6MIM][PF6] |
Benzophenone-3/Urine samples |
HPLC-DAD |
1.3 ng mL−1 |
159
|
[C4MIM][PF6] |
Chlorobenzenes/water samples |
HPLC-PDA |
0.102–0.203 μg L−1 |
160
|
[C4MIM][PF6] |
Phenols (2,4-dichlorophenol, 2-naphthol, 2-nitrophenol, and 4-chlorophenol) |
HPLC-UV |
0.3–0.5 μg L−1 |
161
|
[CnMIM][PF6] (n = 4, 6, 8) |
PAHs/water |
HPLC-DAD |
— |
89
|
Dispersive liquid–liquid microextraction (DLLME)
Dispersive liquid–liquid microextraction (DLLME) was introduced by Rezaee and co-workers in 2006.97 The fundamental principle of DLLME involves the addition of a hydrophobic extraction solvent and dispersive solvent to an aqueous sample. This leads to the formation of a cloudy solution. Upon centrifugation, a biphasic system is established. DLLME provides high enrichment factors due to the fact that the dispersive solvent results in the formation of microdroplets which increase the contact area of the extraction solvent.
Zhou et al. introduced temperature-controlled IL-DLLME for the extraction of pyrethroid pesticides from water.98 Heat was applied to an optimized temperature of 70 °C for complete dissolution of the [C6MIM][PF6] IL to enhance partitioning of the analyte to the IL phase. The IL was then phase separated by cooling and centrifuging the sample. The same authors replaced heat with ultrasonication in order to make dissolution more efficient.99 Liu et al. removed the temperature dependence and used different organic solvents as dispersive solvent.100 The [C6MIM][PF6] IL was used as the extraction solvent with acetone, methanol, and acetonitrile used as the dispersive solvents. Four heterocyclic insecticides, namely, fipronil, chlorfenapyr, buprofezin, and hexythiazox were selected as model compounds for validating this new method. In effort to automate DLLME, Valcárcel and co-workers used a 10 mL syringe to avoid the centrifugation step.101
In situ solvent formation microextraction (ISFME) based on ILs was developed by Shemirani and co-workers.102 In this method, sodium hexafluorophosphate was added as an ion pairing reagent to the sample solution containing the 1-hexyl-3-methylimidazolium tetrafluoroborate IL. A cloudy solution of the 1-hexyl-3-methylimidazolium hexafluorophosphate IL resulted followed by phase separation. A similar approach was introduced at nearly the same time by Yao and Anderson.103 This approach involves the dissolution of a hydrophilic IL in the water phase containing the analytes of interest. A metathesis salt is then added resulting in the generation of a hydrophobic ionic liquid phase via in situ metathesis reaction. The simultaneous extraction and metathesis reaction forming the IL-based extraction phase greatly decreases the extraction time as well as provides higher enrichment factors compared to the traditional IL DLLME and direct immersion single-drop microextraction methods. The same approach was used by Joshi et al. for the extraction of boron from water using glucaminium-based ionic liquids.104Table 3 describes the use of different ILs in a variety of applications using DLLME.
Table 2 Applications using solid-phase microextraction with IL or PIL-based sorbent coatings. Abbreviations used in the table are as follows: [CnMIM][PF6] (1-alkyl-3-methylimidazolium hexafluorophosphate), [C6MIM][BF4] (1-hexyl-3-methylimidazolium tetrafluoroborate), [C4MIM][OH] (1-butyl-3-methylimidazolium hydroxide), ([ViHIM] [PF6]) (1-vinyl-3-hexylimidazolium hexafluorophosphate), poly([VBHDIM][NTf2]) (poly(1-(4-vinylbenzyl)-3-hexadecylimidazolium bis[(trifluoromethyl)sulfonyl]imide), poly ([ViHIM][Cl]) (poly(1-vinyl-3-hexylimidazolium chloride), [AMIM][PF6] (1-allyl-3-methylimidazolium hexafluorophosphate), [AMIM][NTf2] (1-allyl-3-methylimidazolium bis-[(trifluoromethyl)sulfonyl]imide), Poly([VHIM][NTf2]) (poly(1-vinyl-3-hexylimidazolium) bis-[(trifluoromethyl)sulfonyl]imide), poly([VHIM] [taurate]) (poly(1-vinyl-3-hexylimidazolium taurate)), 1-hexadecyl-3-methylimidazolium bromide (HDMIm-Br), 1-hexadecyl-3-butylimidazolium bromide (HDBIm-Br), and 1,3-didodecylimidazolium bromide (DDDDIm-Br), (1-methyl-3-octylimidazolium trifluoromethanesulfonate [C8mim][TfO], 1-benzyl-3-methylimidazolium trifluoromethanesulfonate [Bemim][TfO], 1-methyl-3-phenylpropylimidazolium trifluoromethanesulfonate [Phpromim][TfO], 1-hexadecyl-3-methylimidazolium bromide (HDMIm-Br), 1-hexadecyl-3-butylimidazolium bromide (HDBIm-Br), 1,3-didodecylimidazolium bromide (DDDDIm-Br), GC-TCD (Gas chromatography-thermal conductivity detector), GC-ECD (Gas chromatography-electron capture detector), GC-FID (Gas chromatography-flame ionization detector), HS-SPME (Head space-solid phase microextraction), HF-SPME (Hollow fiber-solid phase microextraction), DI-SPME (Direct immersion-solid phase microextraction)
Ionic Liquid |
Mode of Extraction |
Analyte/Matrix |
Analysis method |
LOD |
Ref. |
[C4MIM][OH] |
HF-SPME |
Diazinon, fenitrothion, malathion, fenvalerate, phosalone and tridemorph/water & hair sample |
HPLC-DAD |
0.003–0.095 ng mL−1 |
162
|
Poly([ViHIM] [PF6] |
HS/DI-SPME |
Benzene, toluene, ethylbenzene and xylenes (BTEX), phenols and PAHs |
GC-FID |
0.02–0.05 μg L−1 |
163
|
poly([VBHDIM][NTf2]), poly ([ViHIM][Cl]) |
HS-SPME |
PAHs/Complex coffee aroma samples |
GC-MS |
— |
164
|
[C4MIM] [PF6] |
HS-SPME |
Pesticides/water |
GC-ECD |
0.12–0.31 ng L−1 |
165
|
[1-allyl-3-(6′-oxo-benzo-15-crown-5 hexyl) imidazolium] [PF6] |
HS/DI-SPME |
Alcohols, phthalate esters, phenolic environmental estrogens, fatty acids and aromatic amines/Water |
GC-FID |
— |
166
|
[C4MIM][BF4], [C8MIM] [BF4], [C8MIM] [PF6], [C2MIM] [EtSO4] |
HS-SPME |
Methyl tert-butyl ether (MTBE)/gasoline sample |
GC-FID |
0.09 μg L−1 |
167
|
Poly ([ViHIM][Cl]) |
HS-SPME |
Alcohols & volatile fatty acids (VFAs)/water |
GC-FID |
0.02–7.5 μg L−1 |
168
|
Poly([ViHIM] [Cl]), Poly([Vi HIM][NTf2]), Poly([ViHDIM][Cl]), Poly([Vi HDIM][NTf2]) |
HS-SPME |
PAHs/water |
GC-FID |
0.1–129 μg L−1 |
169
|
[C4MIM][BF4] |
HS-SPME |
Benzene derivatives/water |
GC-FID |
9.3–48.1 ng L−1 |
170
|
[1-methyl-3-(3-trimethoxysilyl propyl)] [NTf2] |
HS-SPME |
Methyl-tert-butyl ether (MTBE)/gasoline sample |
GC-FID |
0.1 μg L−1 |
110
|
[Bis-hydroxy ethyl imidazoliu m triethoxyethylene] [NTf2]/ [TfO] |
HS-SPME |
Low mol. wt. alcohols, acetone, ethyl acetate, and acetonitrile |
GC-FID |
0.06–0.08 μg L−1 |
171
|
[AMIM][PF6], [AMIM][NTf2] |
HS-SPME |
Phenolic environmental estrogens (PEEs)/water |
GC-FID |
0.003–0.125 μg L−1 |
112
|
Poly ([VBHDIM] [NTf2]) |
DI-SPME |
14 endocrine disruptors (ECDs), PAHs, alkylphenols, and parabens/water |
GC-FID |
9–7 ng L−1 |
172
|
poly([VBHD IM][NTf2]) |
HS-SPME |
12 PAHs/water |
GC-FID |
0.003–6.0 μg L−1 |
173
|
Poly([VHIM] [NTf2]), [poly([VHIM] [taurate]) |
HS-SPME |
CO2 / flue gas |
GC-TCD/FID |
— |
174
|
Poly([ViHDIM][NTf2]) |
DI-SPME |
PAHs and substituted phenols |
GC-MS |
0.005–4.4 ng mL−1 |
175
|
Poly([VHIM] [NTf2]), Poly([VHIM][taurate]) |
HS-SPME |
CO2 |
GC-TCD/FID |
— |
114
|
Poly[ViHDIM] [NTf2] |
HS-SPME |
High mol. wt. aliphatic hydrocarbons and fatty acid methyl esters |
GC-TCD/FID |
0.3–0.6 mg kg−1 |
113
|
[C4MIM][PF6], [C8MIM][PF6] |
HS-SPME |
PAHs/Mosquito incense coil |
GC-FID |
— |
109
|
[EeMIM][NTf2] |
HS-SPME |
Methamphetamine (MAP) and amphetamine (AP)/Human urine |
GC-MS/FID |
0.1–0.5 μg L−1 |
176
|
[C16MIM][Br], [C16BIM][Br], [DDDIM][Br] |
DI-SPME |
Aromatic analytes/water |
HPLC-UV |
— |
177
|
[C4MIM][BF4] |
DI-SPME |
Heterocyclic arom. Amines/food matrices |
HPLC-fluorescence |
0.3–75 ng L−1 |
178
|
[C16MIM][Br], [C16BIM][Br], [DDDDIM][Br] |
HS-SPME |
Polycyclic arom. hydrocarbons (PAHs) |
GC-MS |
— |
179
|
Poly([ViHIM][NTf2]), Poly([ViDDIM][NTf2]), Poly([ViHDIM][NTf2]) |
HS-SPME |
Esters/water |
GC-FID |
2.5–50 μg L−1 |
106
|
[PhproMIM]/ [C8MIM]/ [BeMIM]/[TfO] |
HS-SPME |
PAHs /water |
GC-MS |
5 pg mL−1 |
108
|
[C8MIM][PF6] |
HS-SPME |
benzene, toluene, ethylbenzene, and xylenes (BTEX)/paints |
GC-FID |
56–271 μg g−1 |
107
|
Solid-phase microextraction (SPME)
Since its introduction in the early 1990s by Pawliszyn and co-workers, solid-phase microextraction (SPME) has become a very popular technique in sample preparation.105 SPME consists of a fiber which is coated with a sorbent extraction material, which can be liquid or solid. The fiber is exposed to the matrix by either immersing it into the sample or by sampling the headspace above the matrix. Analytes are then extracted and preconcentrated into the sorbent material followed by thermal desorption in the GC inlet. SPME is simple, robust, inexpensive, and possesses high sensitivity. Since sampling, preconcentration, and desorption of analytes is consolidated into a single step, the approach is quick and often simple to automate. However, the limited number of commercially available sorbent coatings for SPME hinders the selectivity and sensitivity for a variety of classes of analytes. ILs have proven to be a good alternative sorbent material due to their tunable physicochemical properties.106
The first IL-based disposable sorbent coating was introduced by Liu et al. in 2005.107 BTEX compounds in paint were extracted using 1-octyl-3-methylimidazolium hexafluorophosphate ([OMIM][PF6]) and analyzed using GC. Although the IL coating was removed after each extraction by washing the fiber with organic solvent, the higher limits of detection were observed and compared to the commercial polydimethylsiloxane (PDMS) fiber coating. Hsieh et al. improved the stability of the coating by incorporating nafion on the SPME fiber.108 Three different ILs, namely, [OMIM][TfO], [BMIM][TfO] and 1-methyl-3-phenylpropylimidazolium trifluoromethanesulfonate were employed for the extraction of PAHs from rain and ground water. An even film of IL coating was achieved on nafion pretreated fibers compared to a drop-like coated layer on bare silica fiber. In both cases, the fibers must be replenished with a new coating after each extraction. The loading of IL coating on the fiber was further increased to achieve the lower limits of detection by etching a fused-silica fiber using an ammonium hydrogen difluoride solution.109 [BMIM][PF6] and [OMIM][PF6] were coated on this etched fused silica fiber as sorbent coatings for the extraction of PAHs. The extraction efficiency increased significantly for the etched fiber due to the increased IL loading on the etched fiber compared to nafion coated and bare fused silica fibers.
Physically coated IL-based coatings often flow off the fiber when elevated desorption temperatures are used. An approach to overcome this problem is to chemically bond the IL on the surface of the fiber. Amini and co-workers synthesized 1-methyl-3-(3-trimethoxysilyl propyl) imidazolium bis-[(trifluoromethyl)sulfonyl]imide IL and immobilized it on a fused silica fiber by immersing the silica fiber in the IL solution followed by refluxing for 24 h.110 This chemically modified fiber provided high thermal stability (220 °C) and good extraction efficiencies for methyl tert-butyl ether in gasoline.
Sol–gel hybrid organic-inorganic SPME sorbent coatings were developed by Malik and co-workers using two different ILs, namely, trihexyltetradecylphosphonium tetrafluoroborate and N-butyl-4-methylpyridinium tetrafluoroborate and used as porogens.111 Two types of hydroxyl terminated polymers Si-OH terminated polymer (PDMS and BMPO) as well as C–OH terminated polymer (PEG and polyTHF) were developed for in-tube SPME. The extraction efficiency for the IL-mediated PDMS and BMPO sorbent material was found to be 28 times higher than the sorbent material generated without IL. The reverse trend was observed for PEG and polyTHF sol–gel materials. The high viscosity of ILs and lower sol–gel reactivity of C–OH compared to Si–OH was likely responsible for these results. Liu and co-workers synthesized allyl functionalized ILs, namely 1-allyl-3-methylimidazolium hexafluorophosphate and 1-allyl-3-methylimidazolium bis-[(trifluoromethyl)sulfonyl]imide, and chemically bonded these compounds to the fused silica fiber using sol–gel chemistry to generate hybrid organic–inorganic sorbent materials.112 These new sorbent coatings possess many advantageous properties such as a porous surface structure, high thermal stability, wide range of pH stability, strong solvent resistance, and good coating preparation reproducibility. Due to strong electrostatic interactions, hydrogen bonding, and π–π interactions, they possess high selectivity and sensitivity towards environmental polar phenolic estrogens as well as aromatic amines.
Although IL-coatings have proven to be good sorbent materials for extracting many analytes of interest, they also possess a number of limitations. It was observed that ILs are prone to flow off the fiber at higher desorption temperatures and elevated desorption times. In some cases, the IL can bleed from the fiber and contaminate the liner requiring frequent inspection of the inlet. Due to the fact that the fiber has to be re-coated, fiber-to-fiber reproducibility and calibration studies can be a challenge. In an attempt to overcome the aforementioned challenges, our research group introduced polymeric ionic liquids (PILs) as a novel class of sorbent coatings for SPME.106 In the initial study, three PIL-based sorbent coatings, namely, poly(1-vinyl-3-hexylimidazolium NTf2−), poly(1-vinyl-3-dodecylimidazolium NTf2−) and poly(1-vinyl-3-hexadecylimidazolium NTf2−) were synthesized and examined in the extraction of esters in wines. Scanning electron micrographs demonstrated that reusable films (∼12–18 μm) could be achieved using the PIL coatings. These PIL-based coatings showed exceptional thermal stability and fiber lifetimes (over 100 extractions) with acceptable precision. It was also demonstrated that the PIL-based coatings could be used at elevated temperatures to analyze hydrocarbons and fatty acid methyl esters using static headspace SPME.113 Two different PILs, namely, poly(1-vinyl-3-hexylimidazolium bis[(trifluoromethyl)sulfonyl]imide (poly(1-VHIM-NTf2) and poly(1-vinyl-3-hexylimidazolium) taurate (poly(VHIM-taurate) were exploited as sorbent coatings for the selective extraction of CO2.114 The PIL-based fibers, despite their tunable smaller film thickness, exhibited similar sensitivity and superior extraction-to-extraction reproducibility compared to the commercial PDMS and Carboxen fibers. The poly(1-VHIM-taurate) fiber exhibited exceptional capability to retain CO2 under different storage conditions. Dicationic ILs and styrene-based PILs have also been chemically bonded on to the fused-silica fiber and compared with commercial fibers such as the polyacrylate fiber.115Table 2 presents ILs and PILs that have been used in SPME for the extraction of various analytes.
Table 3 Applications employing different ionic liquids in dispersive liquid–liquid microextraction. Abbreviations used in the table are as follows: [CnMIM][PF6] (1-alkyl-3-methylimidazolium hexafluorophosphate), [HNH2MPL][FAP] (1-(6-amino-hexyl)-1-methylpyrrolidiniumtris(pentafluoroethyl) trifluorophosphate), [D(i-C8)IM] [PF6] (1,3-diisooctylimidazolium hexafluorophosphate), [Hpy][PF6] (1-Hexylpyridinium hexafluorophosphate), [(i-C8)MIM] [PF6] (1-isooctyl-3-methylimidazolium hexafluorophosphate), HPLC-LIFD (High performance liquid chromatography-laser-induced fluorescence detector), LC-ESI-MS (Liquid chromatography-electrospray ionization mass spectrometry)
Ionic Liquid |
Mode of Extraction |
Analyte/Matrix |
Analysis method |
LOD |
Ref. |
[C4MIM][PF6], [C6MIM][PF6], [C8MIM][PF6] |
DLLME |
Celastrol/urine |
HPLC-PDA |
1.6 μg L−1 |
180
|
[C6MIM][Cl] |
In situ DLLME |
Insecticides (methoxyfenozide, tetrachlorvinphos, thiamethoxam, and diafenthiuron)/water |
HPLC |
0.98 and 2.54 μg L−1 |
181
|
Glucaminium-based ILs |
In situ DLLME |
Boron/water |
HPLC-UV |
— |
104
|
[HMIM][PF6] |
DLLME |
Isoproturon, monolinuron, linuron, and diuron/water |
HPLC |
0.10–0.24 μg L−1 |
182
|
[C4MIM][PF6], [C6MIM][PF6] |
DLLME |
Ochratoxin A (OTA)/wine |
HPLC-LIFD |
5.2 ng L−1 |
183
|
[C6MIM][PF6] |
DLLME |
Pesticides (carbendazim/benomyl, thiabendazole, fuberidazole, carbaryl, triazophos, 2-aminobenzimidazole, metabolite of carbendazim and 1-naphthol, metabolite of carbaryl)/soil |
HPLC-Fluorescence |
low ng g−1 |
184
|
[C6MIM][PF6] |
DLLME |
Pyrrolidinedithiocarbamate chelate/water |
Flame atomic absorption spectroscopy |
1.5 μg L−1 |
185
|
[C4MIM][PF6] |
DLLME |
Aromatic amine/water |
HPLC-UV |
0.015–0.026 ng mL−1 |
186
|
[C2MIM][BF4], [C8MIM][PF6] |
DLLME |
Hexabromocyclododecane diastereomers (α-, β- and γ-HBCD)/water |
LC-ESI-MS |
0.12–0.22 μg L−1 |
187
|
[HNH2MPL] [FAP] |
In situ DLLME |
Pollutants/water |
HPLC-UV |
0.1 to 55.1 μg L−1 |
188
|
[C4MIM][PF6], [C8MIM][PF6] |
DLLME |
4 benzophenone-type UV filters/water |
HPLC-UV |
1.9–6.4 ng mL−1 |
189
|
[C6MIM][PF6] |
DLLME |
Antioxidants (Irganox 1010, Irganox 1076, Irgafos 168)/water |
HPLC-UV |
5.0–10.0 ng mL−1 |
190
|
[C4MIM][PF6] |
DLLME |
Chlorobenzenes/water |
HPLC-DAD |
0.05–0.1 μg L−1 |
191
|
[D(i-C8)IM] [PF6] |
DLLME |
2,2,2-trichloro-1,1-bis(4-chlorophenyl)ethanol (dicofol) and its degrdn. Products/water |
GC-MS |
3–8 ng L−1 |
192
|
[C4MIM][PF6], [C6MIM][PF6] |
DLLME |
Triazines/honey |
HPLC-DAD |
5.31–8.59 μg kg−1 |
193
|
[Hpy][PF6] |
DLLME |
Aluminum/water, fruit juice, food |
Stopped-flow spectrofluorometry |
0.05 μg L−1 |
194
|
[C8MIM][PF6] |
DLLME |
Parathion, phoxim, phorate and chlorpyrifos/water |
HPLC-UV |
0.1–5.0 μg L−1 |
195
|
[C6MIM][PF6] |
DLLME |
Thiophanate-Me, carbofuran, carbaryl, tebuconazole, iprodione, oxyfluorfen, hexythiazox, and fenazaquin/fruits |
HPLC-DAD |
0.651–5.44 μg kg−1 |
196
|
[C4MIM][Cl] |
DLLME |
Aromatic compounds/water |
HPLC-UV |
0.02–0.3 μg L−1 |
103
|
[C6MIM][PF6] |
DLLME |
Organophosphorus pesticides/water |
HPLC-UV |
0.17–0.29 ng mL−1 |
197
|
[C6MIM][PF6] |
DLLME |
Pyrethroid pesticides/water |
HPLC-UV |
0.28–0.6 μg L−1 |
98
|
[(i-C8)MIM] [PF6] |
DLLME |
Dicofol and DDT/water |
GC-MS |
1.3 ng L−1 |
198
|
[C6MIM][PF6] |
DLLME |
Dichlorodiphenyltrichloroethane (DDT) and its main metabolites/water |
HPLC-UV |
0.24–0.45 ng mL−1 |
199
|
[C4MIM][BF4], [C6MIM][PF6] |
DLLME |
Triclosan and triclocarban bactericides/water |
HPLC-MS |
0.04–0.58 μg L−1 |
200
|
[C4MIM][Cl] |
In situ DLLME |
Endocrine disrupting phenols/water |
HPLC-UV |
0.01–0.087 μg mL−1 |
201
|
[CnMIM][PF6] (n = 4, 6, 8) |
DLLME |
Emodin and its metabolites/urine samples |
HPLC-MS/MS |
0.1–1 ng mL−1 |
202
|
Mass spectrometry
Matrix-assisted laser desorption ionization (MALDI) and electrospray ionization (ESI) are two of the most widely used mass spectrometry techniques. Both are soft ionization techniques used for the analysis of various analytes ranging from small organic molecules, peptides, biomolecules, and polymers. The application of ILs as MALDI matrices and ion pairing reagents in ESI has shown promising results. The development and progress of ILs in the field of mass spectrometry are highlighted in the subsequent two sections.
Matrix assisted laser desorption ionization (MALDI)
MALDI is a soft ionization technique used widely for the analysis of biomolecules and polymers. To perform MALDI, a sample is prepared by dissolving the analyte of interest along with the matrix in a volatile organic solvent. This solution is then spotted onto a MALDI plate and the solvent is allowed to evaporate. Using traditional solid matrices, an analyte often co-crystallizes with the matrix as the solvent evaporates. The sample is then subjected to laser irradiation. The matrix is the most important component in the sample preparation step and is present in high amount compared to the analyte. During laser irradiation, the matrix absorbs the laser energy and prevents fragmentation of analytes while also promoting ionization of the analyte. An ideal matrix should possess chromophores allowing it to absorb laser energy, high stability under vacuum, negligible volatility, ability to dissolve and co-crystallize with the analyte, and promote ionization of the analyte. Most MALDI matrices are small, polar organic molecules and are analyte specific in that a matrix optimal for one type of analyte may not be optimal for another group of analytes. Solid matrices such as α-cyano-4-hydroxycinnamic acid (CHCA) and 2,5-dihydroxybenzoic acid (DHB) are very common.116 However, solid matrices often result in a heterogeneous sample in which different amounts of analyte are found at different spots resulting in poor spot-to-spot reproducibility. This problem can be overcome using liquid matrices. There is literature precedence for the use of liquid matrices. Liquid matrices produce homogenous samples along with good reproducibility but often suffer from extensive adduct formation, peak broadening, high volatility, and low sensitivities.117
ILs possess many desirable characteristics for use in MALDI including high stability under vacuum, formation of a homogenous sample, and often contain UV chromophores, and good ionization efficiencies. Armstrong et al. exploited the unique properties of RTILs for the first time as matrices in MALDI.118 A series of imidazolium and ammonium-based RTILs were synthesized with acidic anions generated from sinapinic acid. The performance of these RTILs was tested using peptides, proteins, and polyethylene glycol. A comparison was made with traditional solid matrices. The newly developed ILs easily dissolved biomolecules and polymers and generated homogenous and uniform films on the MALDI plate. This provided better reproducibility and eliminated the necessity to find the ‘sweet spot’ on the plate. It was also found that protic IL matrices provided much higher signal intensities compared to aprotic IL matrices. The same group developed second generation ammonium cation-based ILs with ferulate, cinnamate, and sinapinate anions as the MALDI matrices.119 These ILs were applied in the analysis of peptides, proteins, and carbohydrates and exhibited wider mass ranges (<1000 Da to >270
000 Da) and better signal-to-noise (S/N) ratios compared to conventional solid matrices. Tholey and co-workers demonstrated the use of 2,5-dihydroxybenzoic acid-based ILs with pyridinium or n-butylamine as cations for the analysis of phosphopeptides where 1% phosphoric acid (by volume) was used as the matrix additive.120 Li et al. showed the use of 1-methylimidazolium α-cyano-4-hydroxycinnamate ILs in the MALDI imaging of gangliosides in mouse brain.121 Recently, Serrano et al. synthesized two new ILs, namely, N,N-diisopropylethylammonium 3-oxocoumarate and N,N-diisopropylethylammonium dihydroxymonooxoacetophenoate, and demonstrated their use in the analysis of aliphatic biodegradable photoluminescent polymers.122 Lovejoy et al. recently showed the use of tetraalkylphosphonium-based ILs with conventional matrices (DHB, CHCA and feluric acid) as anions as an extraction media and matrix for the detection of different dyes.123 The use of two new IL matrices based on the combination of 2-(4-hydroxyphenylazo)benzoic acid with 1,1,3,3-tetramethylguanidine and spermine were evaluated for the analysis of heparin oligosaccharides by Przybylski et al.124 Berthod et al. reported two new IL matrices, namely, N,N-diisopropylethylammonium α-cyano-4-hydroxycinnamate (DEA-CHCA) and N,N-diisopropylethylammonium indoleacrylate (DEA-IAA) for the analysis of polar biodegradable polymers.125 These authors found that DEA-CHCA showed the mass of the intact polymer and was superior to the DEA-IAA IL. DNA oligomer masses were determined using ionic matrices prepared from different basic cations and acidic anions.126 It was found that ionic matrices gave better results than conventional matrices. Ionic matrices composed of 3-hydroxypicolinic acid as the anion with different basic cations yielded good results for the analysis of DNA oligomers. Laremore et al. demonstrated the use of 1,1,3,3-tetramethylguanidinium α-cyano-4-hydroxycinnamic acid IL matrix for the detection of sulfated oligosaccharides.127 The IL matrix suppressed the loss of the sulfate group and the detected intact sulfated oligosaccharides were at a concentration of 10 picomol or higher. Fukuyama et al. modified this ionic matrix by replacing α-cyano-4-hydroxy cinnamic acid with p-coumaric acid to suppress the fragmentation of oligosaccharides in addition to lowering the detection limit to 1 femtomole.128 Mank et al. synthesized the new IL 2,4-dihydroxybenzoic acid butylamine and compared it to a conventional matrix as well as with two other IL matrices, α-cyano-4-hydroxycinnamic acid butylamine and 3,5-dimethoxycinnamic acid triethylamine.129 The best results were found with the 2,4-dihydroxybenzoic acid butylamine IL matrix in the detection of biomolecules. Li at al. evaluated IL matrices for the detection of phospholipids and achieved good sensitivity.130
Ionic liquids as ion pairing reagents in electrospray ionization mass spectrometry (ESI-MS)
Electrospray ionization is a very simple, sensitive, and convenient method for the detection of various analytes. However, the sensitivity is often low in the negative ion mode compared to positive ion mode with commonly used solvents such as methanol and water due to corona discharge, poor spray stability, and a tendency for arcing to occur. Also, ions that possess low molecular weight are often difficult to detect. Significant improvement in the detection of anions has been attained using ILs as ion pairing reagents.131 This has made detection of anions possible in the positive ion mode which provides much lower detection limits. In the first report of using ILs as ion pairing reagents, Martinelango et al. demonstrated the utility of dicationic ILs for the detection of perchlorate (ClO4−) in water.132 This work was followed by examining 23 different dicationic ILs for the detection of nitrate, iodide, cyanate, monochloroacetate, benzenesulfonate, and perfluoro-octanoate.131 The detection limits for each anion varied from the low ppb (μg L−1) to ppt (ng L−1) levels. Soukup-Hein and co-workers also explored tricationic ILs for the detection of doubly charged anions in the positive mode of ESI.133 These dicationic and tricationic ILs were also employed for the detection of bisphosphonate drugs.134 Better detection limits were found using dicationic ILs compared to tricationic ILs. Overall, ILs can serve as ion pairing reagents in the detection of anions and dianions with improved limits of detection. In addition, the ability to pair with di- or trications varies dramatically for each anion or dianion.
Future outlook
The design and synthesis of new ILs and RTILs will continue to attract the interest of separation scientists to these unique solvents. The continual development and introduction of commercial GC stationary phases based on ILs will highlight the advantages they possess in their unique separation selectivity. Application of ILs and RTILs in solid and liquid phase microextraction has opened up a new avenue for achieving highly selective and efficient extractions due to their tunable properties. In SPME, there are currently limited sorbent coatings available which may hinder the use of SPME in some analyses. The introduction of ILs and PILs as SPME coatings has tremendous promise in achieving the selectivities required for broad and targeted classes of analytes. The generation of reproducible sorbent coatings is one major challenge needing addressed. The development of new polar coatings as well as thermally stable ILs and PILs can extend the use of SPME for the analysis of many polar analytes by lowering the limit of detection. The design and application of ILs for the selective extraction of analytes in all solid or liquid phase extraction techniques will be an area of immense interest. The successful implementation of ILs as MALDI matrices has provided versatile matrices with high shot-to-shot reproducibility and lower detection limits. New IL-based ion pairing reagents will most likely soon be commercially available to aid in the part-per-trillion level detection of ions using ESI-MS. Overall, ILs have emerged as unique and versatile solvents and materials with a number of advantageous properties and will continue to gain tremendous attention from the separations and mass spectrometry communities.
References
- S. Gabriel and J. Weiner, Ber. Dtsch. Chem. Ges., 1888, 21, 2669–2679 CrossRef.
- P. Von Walden, Bull. Acad. Imp. Sci. St. Petersburg, 1914, 8, 405–422 Search PubMed.
- J. S. Wilkes, J. A. Levisky, R. A. Wilson and C. L. Hussey, Inorg. Chem., 1982, 21, 1263–1264 CrossRef CAS.
- J. S. Wilkes and M. J. Zaworotko, J. Chem. Soc., Chem. Commun., 1992, 965–967 RSC.
- T. Welton, Chem. Rev., 1999, 99, 2071–2084 CrossRef CAS.
- T. Welton, Coord. Chem. Rev., 2004, 248, 2459–2477 CrossRef CAS.
- D. Wei and A. Ivaska, Anal. Chim. Acta, 2008, 607, 126–135 CrossRef CAS.
- J. Ding, T. Welton and D. W. Armstrong, Anal. Chem., 2004, 76, 6819–6822 CrossRef CAS.
- J. G. Huddleston and R. D. Rogers, Chem. Commun., 1998, 1765–1766 RSC.
- K. R. Seddon, A. Stark and M. J. Torres, Pure Appl. Chem., 2000, 72, 2275–2287 CrossRef CAS.
-
(a) J. L. Anderson and D. W. Armstrong, Anal. Chem., 2003, 75, 4851–4858 CrossRef CAS;
(b) D. W. Armstrong, L. He and Y.-S. Liu, Anal. Chem., 1999, 71, 3873–3876 CrossRef CAS.
- M. J. Earle, J. M. S. S. Esperança, M. A. Gilea, J. N. C. Lopes, L. P. N. Rebelo, J. W. Magee, K. R. Seddon and J. A. Widegren, Nature, 2006, 439, 831–834 CrossRef CAS.
-
(a) J. L. Anderson and D. W. Armstrong, Anal. Chem., 2005, 77, 6453–6462 CrossRef CAS;
(b) T. Payagala, Y. Zhang, E. Wanigasekara, K. Huang, Z. S. Breitbach, P. S. Sharma, L. M. Sidisky and D. W. Armstrong, Anal. Chem., 2008, 81, 160–173 CrossRef.
- S. Carda-Broch, A. Berthod and D. W. Armstrong, Anal. Bioanal. Chem., 2003, 375, 191–199 CAS.
-
(a) M. J. Muldoon, C. M. Gordon and I. R. Dunkin, J. Chem. Soc., Perkin Trans. 2, 2001, 433–435 RSC;
(b) A. J. Carmichael and K. R. Seddon, J. Phys. Org. Chem., 2000, 13, 591–595 CrossRef CAS.
-
(a) J. L. Anderson, J. Ding, T. Welton and D. W. Armstrong, J. Am. Chem. Soc., 2002, 124, 14247–14254 CrossRef CAS;
(b) M. H. Abraham, Chem. Soc. Rev., 1993, 22, 73–83 RSC.
- C. F. Poole, J. Chromatogr., A, 2004, 1037, 49–82 CrossRef CAS.
-
(a) J. L. Anderson, D. W. Armstrong and G. T. Wei, Anal. Chem., 2006, 78, 2892–2902 CrossRef;
(b) R. J. Soukup-Hein, M. M. Warnke and D. W. Armstrong, Annu. Rev. Anal. Chem., 2009, 2, 145–168 CrossRef CAS;
(c) P. Sun and D. W. Armstrong, Anal. Chim. Acta, 2010, 661, 1–16 CrossRef CAS;
(d) A. Berthod, M. Ruiz-Angel and S. Carda-Broch, J. Chromatogr., A, 2008, 1184, 6–18 CrossRef CAS;
(e) X. Han and D. W. Armstrong, Acc. Chem. Res., 2007, 40, 1079–1086 CrossRef CAS.
- D. Barber, C. Phillips, G. Tusa and A. Verdin, J. Chem. Soc., 1959, 18–24 RSC.
- J. E. Gordon, J. E. Selwyn and R. L. Thorne, J. Org. Chem., 1966, 31, 1925–1930 CrossRef CAS.
- F. Pacholec, H. T. Butler and C. F. Poole, Anal. Chem., 1982, 54, 1938–1941 CrossRef CAS.
- F. Pacholec and C. F. Poole, Chromatographia, 1983, 17, 370–374 CAS.
- C. F. Poole, H. T. Butler, M. E. Coddens, S. C. Dhanesar and F. Pacholec, J. Chromatogr., A, 1984, 289, 299–320 CrossRef CAS.
-
(a) P. S. Sharma, T. Payagala, E. Wanigasekara, A. B. Wijeratne, J. Huang and D. W. Armstrong, Chem. Mater., 2008, 20, 4182–4184 CrossRef CAS;
(b) T. Payagala, J. Huang, Z. S. Breitbach, P. S. Sharma and D. W. Armstrong, Chem. Mater., 2007, 19, 5848–5850 CrossRef CAS.
- T. Payagala, Y. Zhang, E. Wanigasekara, K. Huang, Z. S. Breitbach, P. S. Sharma, L. M. Sidisky and D. W. Armstrong, Anal. Chem., 2009, 81, 160–173 CrossRef CAS.
- Q. Wei, M. Qi, H. Yang and R. Fu, Chromatographia, 2011, 74, 717–724 CAS.
- L. X. Zhang, T. Liu and A. Q. Luo, Adv. Mater. Res., 2011, 317, 1936–1939 CrossRef.
- C. F. Poole and S. K. Poole, J. Sep. Sci., 2011, 34, 888–900 CrossRef CAS.
- A. Berthod, L. He and D. Armstrong, Chromatographia, 2001, 53, 63–68 CAS.
- K. Huang, X. Zhang and D. W. Armstrong, J. Chromatogr., A, 2010, 1217, 5261–5273 CrossRef CAS.
- C. D. Tran, I. Mejac, J. Rebek Jr and R. J. Hooley, Anal. Chem., 2008, 81, 1244–1254 CrossRef.
- L. M. Yuan, C. X. Ren, L. Li, P. Ai, Z. H. Yan, M. Zi and Z. Y. Li, Anal. Chem., 2006, 78, 6384–6390 CrossRef CAS.
- C. D. Tran and S. Challa, Analyst, 2008, 133, 455–464 RSC.
- K. P. Huang, T. K. Misra, G. R. Wang, B. Y. Huang and C. Y. Liu, J. Chromatogr., A, 2008, 1215, 177–184 CrossRef CAS.
- C. F. Poole, B. R. Kersten, S. S. J. Ho, M. E. Coddens and K. G. Furton, J. Chromatogr., A, 1986, 352, 407–425 CrossRef CAS.
- P. H. Shetty, P. J. Youngberg, B. R. Kersten and C. F. Poole, J. Chromatogr., A, 1987, 411, 61–79 CrossRef CAS.
-
(a) M. M. Waichigo, B. M. Hunter, T. L. Riechel and N. D. Danielson, J. Liq. Chromatogr. Relat. Technol., 2007, 30, 165–184 CrossRef CAS;
(b) M. M. Waichigo and N. D. Danielson, J. Chromatogr. Sci., 2006, 44, 607–614 CAS.
- M. Waichigo, T. Riechel and N. Danielson, Chromatographia, 2005, 61, 17–23 CAS.
- S. Grossman and N. D. Danielson, J. Chromatogr., A, 2009, 1216, 3578–3586 CrossRef CAS.
- W. Zhang, L. He, Y. Gu, X. Liu and S. Jiang, Anal. Lett., 2003, 36, 827–838 CrossRef CAS.
- M. Ruiz-Angel, S. Carda-Broch and A. Berthod, J. Chromatogr., A, 2006, 1119, 202–208 CrossRef CAS.
- A. Berthod, M. J. Ruiz-Angel and S. Huguet, Anal. Chem., 2005, 77, 4071–4080 CrossRef CAS.
- M. P. Marszall, T. Baczek and R. Kaliszan, Anal. Chim. Acta, 2005, 547, 172–178 CrossRef CAS.
- R. Kaliszan, M. P. Marsza, M. Jan Markuszewski, T. Bczek and J. Pernak, J. Chromatogr., A, 2004, 1030, 263–271 CrossRef CAS.
- L. He, W. Zhang, L. Zhao, X. Liu and S. Jiang, J. Chromatogr., A, 2003, 1007, 39–45 CrossRef CAS.
- R. Nageswara Rao, B. Ramachandra and R. Mastan Vali, J. Sep. Sci., 2011, 34, 500–507 CrossRef.
-
(a) A. Martín-Calero, V. Pino, J. H. Ayala, V. González and A. M. Afonso, Talanta, 2009, 79, 590–597 CrossRef;
(b) A. Martín-Calero, G. Tejral, J. H. Ayala, V. González and A. M. Afonso, J. Sep. Sci., 2010, 33, 182–190 CrossRef;
(c) X. Xiaohua, Z. Liang, L. Xia and J. Shengxiang, Anal. Chim. Acta, 2004, 519, 207–211 CrossRef.
-
(a) L. Shu Juan, Z. Feng and X. Xiao Hua, Chin. Chem. Lett., 2004, 15, 1060–1062 Search PubMed;
(b) H. Qiu, S. Jiang and X. Liu, J. Chromatogr., A, 2006, 1103, 265–270 CrossRef CAS;
(c) H. Qiu, S. Jiang, X. Liu and L. Zhao, J. Chromatogr., A, 2006, 1116, 46–50 CrossRef CAS.
- Y. Sun, B. Cabovska, C. Evans, T. Ridgway and A. Stalcup, Anal. Bioanal. Chem., 2005, 382, 728–734 CrossRef CAS.
- K. Chitta, D. Van Meter and A. Stalcup, Anal. Bioanal. Chem., 2010, 396, 775–781 CrossRef CAS.
- H. Qiu, L. Wang, X. Liu and S. Jiang, Analyst, 2008, 134, 460–465 RSC.
- H. Qiu, M. Takafuji, X. Liu, S. Jiang and H. Ihara, J. Chromatogr., A, 2010, 1217, 5190–5196 CrossRef CAS.
- H. Qiu, M. Takafuji, T. Sawada, X. Liu, S. Jiang and H. Ihara, Chem. Commun., 2010, 46, 8740–8742 RSC.
-
(a) W. Qin, H. Wei and S. F. Y. Li, J. Chromatogr., A, 2003, 985, 447–454 CrossRef CAS;
(b) M. Vaher, M. Koel and M. Kaljurand, Electrophoresis, 2002, 23, 426–430 CrossRef CAS.
- E. G. Yanes, S. R. Gratz, M. J. Baldwin, S. E. Robison and A. M. Stalcup, Anal. Chem., 2001, 73, 3838–3844 CrossRef CAS.
- M. Vaher, M. Koel and M. Kaljurand, J. Chromatogr., A, 2002, 979, 27–32 CrossRef CAS.
-
(a) T. F. Jiang, Y. L. Gu, B. Liang, J. B. Li, Y. P. Shi and Q. Y. Ou, Anal. Chim. Acta, 2003, 479, 249–254 CrossRef CAS;
(b) X. Wu, W. Wei, Q. Su, L. Xu and G. Chen, Electrophoresis, 2008, 29, 2356–2362 CrossRef CAS.
- S. Qi, Y. Li, Y. Deng, Y. Cheng, X. Chen and Z. Hu, J. Chromatogr., A, 2006, 1109, 300–306 CrossRef CAS.
- M. Vaher, M. Koel, J. Kazarjan and M. Kaljurand, Electrophoresis, 2011, 32, 1068–1073 CrossRef CAS.
- M. Vaher, M. Koel and M. Kaljurand, Chromatographia, 2001, 53, 302–306 Search PubMed.
- S. M. Mwongela, A. Numan, N. L. Gill, R. A. Agbaria and I. M. Warner, Anal. Chem., 2003, 75, 6089–6096 CrossRef CAS.
- Y. Francois, A. Varenne, E. Juillerat, D. Villemin and P. Gareil, J. Chromatogr., A, 2007, 1155, 134–141 CrossRef CAS.
- W. Qin and S. F. Y. Li, Electrophoresis, 2002, 23, 4110–4116 CrossRef CAS.
- M. Borissova, M. Vaher, M. Koel and M. Kaljurand, J. Chromatogr., A, 2007, 1160, 320–325 CrossRef CAS.
- J. G. Huddleston and R. D. Rogers, Chem. Commun., 1998, 1765–1766 RSC.
- A. E. Visser, R. P. Swatloski and R. D. Rogers, Green Chem., 2000, 2, 1–4 RSC.
- S. Dai, Y. Ju and C. Barnes, J. Chem. Soc., Dalton Trans., 1999, 1201–1202 RSC.
- H. Luo, S. Dai and P. V. Bonnesen, Anal. Chem., 2004, 76, 2773–2779 CrossRef CAS.
- H. Luo, S. Dai, P. V. Bonnesen, A. Buchanan III, J. D. Holbrey, N. J. Bridges and R. D. Rogers, Anal. Chem., 2004, 76, 3078–3083 CrossRef CAS.
- K. Shimojo and M. Goto, Anal. Chem., 2004, 76, 5039–5044 CrossRef CAS.
-
(a) N. Hirayama, H. Okamura, K. Kidani and H. Imura, Anal. Sci., 2008, 24, 697–699 CrossRef CAS;
(b) K. Kidani, N. Hirayama and H. Imura, Anal. Sci., 2008, 24, 1251–1254 CrossRef CAS.
- D. Kogelnig, A. Regelsberger, A. Stojanovic, F. Jirsa, R. Krachler and B. K. Keppler, Monatshefte für Chemie/Chemical Monthly, 2011, 1–4 Search PubMed.
- C. Yansheng, L. Changping, J. Qingzhu, L. Qingshan, Y. Peifang, L. Xiumei and U. Welz-Biermann, Green Chem., 2011, 13, 1224–1229 RSC.
- G. T. Wei, Z. Yang, C. Y. Lee, H. Y. Yang and C. R. C. Wang, J. Am. Chem. Soc., 2004, 126, 5036–5037 CrossRef CAS.
- K. Bica, P. Gaertner and R. D. Rogers, Green Chem., 2011, 13, 1997–1999 RSC.
- J. H. Wang, D. H. Cheng, X. W. Chen, Z. Du and Z. L. Fang, Anal. Chem., 2007, 79, 620–625 CrossRef CAS.
-
(a) K. Shimojo, N. Kamiya, F. Tani, H. Naganawa, Y. Naruta and M. Goto, Anal. Chem., 2006, 78, 7735–7742 CrossRef CAS;
(b) Y. Pei, J. Wang, K. Wu, X. Xuan and X. Lu, Sep. Purif. Technol., 2009, 64, 288–295 CrossRef CAS.
- Y. Lu, W. Lu, W. Wang, Q. Guo and Y. Yang, Talanta, 2011, 85, 1621–1626 CrossRef CAS.
- C. Yansheng, Z. Zhida, L. Changping, L. Qingshan, Y. Peifang and U. Welz-Biermann, Green Chem., 2011, 13, 666–670 RSC.
- Y. Zhai, S. Sun, Z. Wang, J. Cheng, Y. Sun, L. Wang, Y. Zhang, H. Zhang and A. Yu, J. Sep. Sci., 2009, 32, 3544–3549 CrossRef CAS.
- Y. Lu, W. Ma, R. Hu, X. Dai and Y. Pan, J. Chromatogr., A, 2008, 1208, 42–46 CrossRef CAS.
- F. Y. Du, X. H. Xiao, X. J. Luo and G. K. Li, Talanta, 2009, 78, 1177–1184 CrossRef CAS.
- Y. Yuan, Y. Wang, R. Xu, M. Huang and H. Zeng, Analyst, 2011, 136, 2294–2305 RSC.
- V. Pino, J. L. Anderson, J. H. Ayala, V. González and A. M. Afonso, J. Chromatogr., A, 2008, 1182, 145–152 CrossRef CAS.
- M. German-Hernandez, V. Pino, J. L. Anderson and A. M. Afonso, Talanta, 2011, 85, 1199–1206 CrossRef CAS.
- S. Gao, J. You, X. Zheng, Y. Wang, R. Ren, R. Zhang, Y. Bai and H. Zhang, Talanta, 2010, 82, 1371–1377 CrossRef CAS.
- H. Liu and P. K. Dasgupta, Anal. Chem., 1996, 68, 1817–1821 CrossRef CAS.
- Y. He and H. Lee, Anal. Chem., 1997, 69, 4634–4640 CrossRef CAS.
- J. Liu, G. Jiang, Y. Chi, Y. Cai, Q. Zhou and J. T. Hu, Anal. Chem., 2003, 75, 5870–5876 CrossRef CAS.
- D. Ge and H. K. Lee, J. Chromatogr., A, 2012, 1229, 1–5 CrossRef CAS.
- Y. Tao, J. F. Liu, X. L. Hu, H. C. Li, T. Wang and G. B. Jiang, J. Chromatogr., A, 2009, 1216, 6259–6266 CrossRef CAS.
- M. A. Jeannot and F. F. Cantwell, Anal. Chem., 1996, 68, 2236–2240 CrossRef CAS.
- M. A. Jeannot and F. F. Cantwell, Anal. Chem., 1997, 69, 235–239 CrossRef CAS.
- A. L. Theis, A. J. Waldack, S. M. Hansen and M. A. Jeannot, Anal. Chem., 2001, 73, 5651–5654 CrossRef CAS.
- C. Yao, W. R. Pitner and J. L. Anderson, Anal. Chem., 2009, 81, 5054–5063 CrossRef CAS.
- E. Aguilera-Herrador, R. Lucena, S. Cardenas and M. Valcarcel, Anal. Chem., 2008, 80, 793–800 CrossRef CAS.
- M. Rezaee, Y. Assadi, M. R. Milani Hosseini, E. Aghaee, F. Ahmadi and S. Berijani, J. Chromatogr., A, 2006, 1116, 1–9 CrossRef CAS.
- Q. Zhou, H. Bai, G. Xie and J. Xiao, J. Chromatogr., A, 2008, 1177, 43–49 CrossRef CAS.
- Q. Zhou, X. Zhang and J. Xiao, J. Chromatogr., A, 2009, 1216, 4361–4365 CrossRef CAS.
- Y. Liu, E. Zhao, W. Zhu, H. Gao and Z. Zhou, J. Chromatogr., A, 2009, 1216, 885–891 CrossRef CAS.
- M. Cruz-Vera, R. Lucena, S. Cárdenas and M. Valcárcel, J. Chromatogr., A, 2009, 1216, 6459–6465 CrossRef CAS.
- M. Baghdadi and F. Shemirani, Anal. Chim. Acta, 2009, 634, 186–191 CrossRef CAS.
- C. Yao and J. L. Anderson, Anal. Bioanal. Chem., 2009, 395, 1491–1502 CrossRef CAS.
- M. D. Joshi, G. Chalumot, Y.-w. Kim and J. L. Anderson, Chem. Commun., 2012, 48, 1410–1412 RSC.
- C. L. Arthur and J. Pawliszyn, Anal. Chem., 1990, 62, 2145–2148 CrossRef CAS.
- F. Zhao, Y. Meng and J. L. Anderson, J. Chromatogr., A, 2008, 1208, 1–9 CrossRef CAS.
- J. Liu, N. Li, G. Jiang, J. A. Jonsson and M. Wen, J. Chromatogr., A, 2005, 1066, 27–32 CrossRef CAS.
- Y. N. Hsieh, P. C. Huang, I. Sun, T. J. Whang, C. Y. Hsu, H. H. Huang and C. H. Kuei, Anal. Chim. Acta, 2006, 557, 321–328 CrossRef CAS.
- K. P. Huang, G. R. Wang, B. Y. Huang and C. Y. Liu, Anal. Chim. Acta, 2009, 645, 42–47 CrossRef CAS.
- R. Amini, A. Rouhollahi, M. Adibi and A. Mehdinia, J. Chromatogr., A, 2010, 1218, 130–136 CrossRef.
- A. M. Shearrow, G. A. Harris, L. Fang, P. K. Sekhar, L. T. Nguyen, E. B. Turner, S. Bhansali and A. Malik, J. Chromatogr., A, 2009, 1216, 5449–5458 CrossRef CAS.
- M. Liu, X. Zhou, Y. Chen, H. Liu, X. Feng, G. Qiu, F. Liu and Z. Zeng, Anal. Chim. Acta, 2010, 683, 96–106 CrossRef CAS.
- Y. Meng, V. Pino and J. L. Anderson, Anal. Chem., 2009, 81, 7107–7112 CrossRef CAS.
- Q. Zhao, J. C. Wajert and J. L. Anderson, Anal. Chem., 2009, 82, 707–713 CrossRef.
- E. Wanigasekara, S. Perera, J. A. Crank, L. Sidisky, R. Shirey, A. Berthod and D. W. Armstrong, Anal. Bioanal. Chem., 2010, 396, 511–524 CrossRef CAS.
- J. Zhang, R. Knochenmuss, E. Stevenson and R. Zenobi, Int. J. Mass Spectrom., 2002, 213, 237–250 CrossRef CAS.
- A. Tholey and E. Heinzle, Anal. Bioanal. Chem., 2006, 386, 24–37 CrossRef CAS.
- D. W. Armstrong, L. K. Zhang, L. He and M. L. Gross, Anal. Chem., 2001, 73, 3679–3686 CrossRef CAS.
- J. A. Crank and D. W. Armstrong, J. Am. Soc. Mass Spectrom., 2009, 20, 1790–1800 CrossRef CAS.
- A. Tholey, Rapid Commun. Mass Spectrom., 2006, 20, 1761–1768 CrossRef CAS.
- K. Chan, P. Lanthier, X. Liu, J. K. Sandhu, D. Stanimirovic and J. Li, Anal. Chim. Acta, 2009, 639, 57–61 CrossRef CAS.
- C. A. Serrano, Y. Zhang, J. Yang and K. A. Schug, Rapid Commun. Mass Spectrom., 2011, 25, 1152–1158 CrossRef CAS.
- K. S. Lovejoy, G. M. Purdy, S. Iyer, T. C. Sanchez, A. Robertson, A. T. Koppisch and R. E. Del Sesto, Anal. Chem., 2011, 83, 2921–2930 CrossRef CAS.
- C. Przybylski, F. Gonnet, D. Bonnaffé, Y. Hersant, H. Lortat-Jacob and R. Daniel, Glycobiology, 2010, 20, 224 CrossRef CAS.
- A. Berthod, J. A. Crank, K. L. Rundlett and D. W. Armstrong, Rapid Commun. Mass Spectrom., 2009, 23, 3409–3422 CrossRef CAS.
- S. Carda-Broch, A. Berthod and D. W. Armstrong, Rapid Commun. Mass Spectrom., 2003, 17, 553–560 CrossRef CAS.
- T. N. Laremore, F. Zhang and R. J. Linhardt, Anal. Chem., 2007, 79, 1604–1610 CrossRef CAS.
- Y. Fukuyama, S. Nakaya, Y. Yamazaki and K. Tanaka, Anal. Chem., 2008, 80, 2171–2179 CrossRef CAS.
- M. Mank, B. Stahl and G. Boehm, Anal. Chem., 2004, 76, 2938–2950 CrossRef CAS.
- Y. L. Li, M. L. Gross and F. F. Hsu, J. Am. Soc. Mass Spectrom., 2005, 16, 679–682 CrossRef CAS.
- J. W. Remsburg, R. J. Soukup-Hein, J. A. Crank, Z. S. Breitbach, T. Payagala and D. W. Armstrong, J. Am. Soc. Mass Spectrom., 2008, 19, 261–269 CrossRef CAS.
- P. K. Martinelango, J. L. Anderson, P. K. Dasgupta, D. W. Armstrong, R. S. Al-Horr and R. W. Slingsby, Anal. Chem., 2005, 77, 4829–4835 CrossRef CAS.
- R. J. Soukup-Hein, J. W. Remsburg, Z. S. Breitbach, P. S. Sharma, T. Payagala, E. Wanigasekara, J. Huang and D. W. Armstrong, Anal. Chem., 2008, 80, 2612–2616 CrossRef CAS.
- M. M. Warnke, Z. S. Breitbach, E. Dodbiba, J. A. Crank, T. Payagala, P. Sharma, E. Wanigasekara, X. Zhang and D. W. Armstrong, Anal. Chim. Acta, 2009, 633, 232–237 CrossRef CAS.
- I. Márquez-Sillero, S. Cárdenas and M. Valcárcel, J. Chromatogr., A, 2011, 1218, 7574–7580 CrossRef.
- F. Ahmad and H. F. Wu, Analyst, 2011, 136, 4020–4027 RSC.
- M. Rahmani and M. Kaykhaii, Microchim. Acta, 2011, 174, 413–419 CrossRef CAS.
- X. Wen, Q. Deng and J. Guo, Spectrochim. Acta, Part A, 2011, 79, 1941–1945 CrossRef CAS.
- Q. Wang, H. Qiu, J. Li, H. Han, X. Liu and S. Jiang, J. Sep. Sci., 2011, 35, 594–600 CrossRef CAS.
- A. Sarafraz-Yazdi and F. Mofazzeli, Chromatographia, 2010, 72, 867–873 CAS.
- M. Amjadi, J. L. Manzoori and J. Abulhassani, Journal of AOAC International, 2010, 93, 985–991 CAS.
- E. M. Martinis and R. G. Wuilloud, J. Anal. At. Spectrom., 2010, 25, 1432–1439 RSC.
- Q. Liu, Y. Liu and S. Chen, J. Sep. Sci., 2010, 33, 2376–2382 CrossRef CAS.
- Q. Wang, H. Qiu, J. Li, X. Liu and S. Jiang, J. Chromatogr., A, 2010, 1217, 5434–5439 CrossRef CAS.
- H. Sheikhloie, M. Saber-Tehrani, P. Abrumand-Azar and S. Waqif-Husain, Acta Chromatogr., 2009, 21, 577–589 CrossRef CAS.
- L. Vidal, A. Chisvert, A. Canals and A. Salvador, Talanta, 2010, 81, 549–555 CrossRef CAS.
- E. M. Martinis, P. Bertón, J. C. Altamirano, U. Hakala and R. G. Wuilloud, Talanta, 2010, 80, 2034–2040 CrossRef CAS.
- J. L. Manzoori, M. Amjadi and J. Abulhassani, Anal. Chim. Acta, 2009, 644, 48–52 CrossRef CAS.
- E. Aguilera-Herrador, R. Lucena, S. Cárdenas and M. Valcárcel, J. Chromatogr., A, 2009, 1216, 5580–5587 CrossRef CAS.
- F. Zhao, S. Lu, W. Du and B. Zeng, Microchim. Acta, 2009, 165, 29–33 CrossRef CAS.
- F. Pena-Pereira, I. Lavilla, C. Bendicho, L. Vidal and A. Canals, Talanta, 2009, 78, 537–541 CrossRef CAS.
- A. Chisvert, I. P. Román, L. Vidal and A. Canals, J. Chromatogr., A, 2009, 1216, 1290–1295 CrossRef CAS.
- J. L. Manzoori, M. Amjadi and J. Abulhassani, Talanta, 2009, 77, 1539–1544 CrossRef CAS.
- L. Xia, X. Li, Y. Wu, B. Hu and R. Chen, Spectrochim. Acta, Part B, 2008, 63, 1290–1296 CrossRef.
- E. Aguilera-Herrador, R. Lucena, S. Cárdenas and M. Valcárcel, J. Chromatogr., A, 2008, 1209, 76–82 CrossRef CAS.
- F. Q. Zhao, J. Li and B. Z. Zeng, J. Sep. Sci., 2008, 31, 3045–3049 CrossRef CAS.
- E. Aguilera-Herrador, R. Lucena, S. Cárdenas and M. Valcárcel, J. Chromatogr., A, 2008, 1201, 106–111 CrossRef CAS.
- Q. Zhou and C. Ye, Microchim. Acta, 2008, 162, 153–159 CrossRef CAS.
- L. Vidal, A. Chisvert, A. Canals and A. Salvador, J. Chromatogr., A, 2007, 1174, 95–103 CrossRef CAS.
- L. Vidal, E. Psillakis, C. E. Domini, N. Grané, F. Marken and A. Canals, Anal. Chim. Acta, 2007, 584, 189–195 CrossRef CAS.
- Q. X. Zhou, J. P. Xiao, C. L. Ye and X. M. Wang, Chin. Chem. Lett., 2006, 17, 1073 CAS.
- M. Ebrahimi, Z. Es'haghi, F. Samadi and M. S. Hosseini, J. Chromatogr., A, 2011, 1218, 8313–8321 CrossRef CAS.
- J. Feng, M. Sun, L. Xu, J. Li, X. Liu and S. Jiang, J. Chromatogr., A, 2011, 1218, 7758–7764 CrossRef CAS.
- J. López-Darias, J. L. Anderson, V. Pino and A. M. Afonso, Anal. Bioanal. Chem., 2011, 401, 1–12 CrossRef.
- Z. Gao, W. Li, B. Liu, F. Liang, H. He, S. Yang and C. Sun, J. Chromatogr., A, 2011, 1218, 6285–6291 CrossRef CAS.
- X. Zhou, P. Xie, J. Wang, B. Zhang, M. Liu, H. Liu and X. Feng, J. Chromatogr., A, 2011, 1218, 3571–3580 CrossRef CAS.
- R. Amini, A. Rouhollahi, M. Adibi and A. Mehdinia, Talanta, 2011, 84, 1–6 CrossRef CAS.
- Y. Meng, V. Pino and J. L. Anderson, Anal. Chim. Acta, 2010, 687, 141–149 CrossRef.
- C. M. Graham, Y. Meng, T. Ho and J. L. Anderson, J. Sep. Sci., 2011, 34, 340–346 CrossRef CAS.
- F. Zhao, M. Wang, Y. Ma and B. Zeng, J. Chromatogr., A, 2010, 1218, 387–391 CrossRef.
- S. Carda-Broch, M. J. Ruiz-Angel, D. W. Armstrong and A. Berthod, Sep. Sci. Technol., 2010, 45, 2322–2328 CrossRef CAS.
- J. López-Darias, V. Pino, Y. Meng, J. L. Anderson and A. M. Afonso, J. Chromatogr., A, 2010, 1217, 7189–7197 CrossRef.
- Y. Meng and J. L. Anderson, J. Chromatogr., A, 2010, 1217, 6143–6152 CrossRef CAS.
- Q. Zhao and J. L. Anderson, J. Chromatogr., A, 2010, 1217, 4517–4522 CrossRef CAS.
- J. López-Darias, V. Pino, J. L. Anderson, C. M. Graham and A. M. Afonso, J. Chromatogr., A, 2010, 1217, 1236–1243 CrossRef.
- Y. He, J. Pohl, R. Engel, L. Rothman and M. Thomas, J. Chromatogr., A, 2009, 1216, 4824–4830 CrossRef CAS.
- C. Yao, V. Pino and J. L. Anderson, J. Chromatogr., A, 2009, 1216, 948–955 CrossRef CAS.
- A. Martín-Calero, J. H. Ayala, V. González and A. M. Afonso, Anal. Bioanal. Chem., 2009, 394, 937–946 CrossRef.
- L. Guerra-Abreu, V. Pino, J. L. Anderson and A. M. Afonso, J. Chromatogr., A, 2008, 1214, 23–29 CrossRef CAS.
- J. N. Sun, Y. P. Shi and J. Chen, J. Chromatogr., B: Anal. Technol. Biomed. Life Sci., 2011, 879, 3429–3433 CrossRef CAS.
- S. Li, H. Gao, J. Zhang, Y. Li, B. Peng and Z. Zhou, J. Sep. Sci., 2011, 34, 3178–3185 CrossRef CAS.
- S. Wang, C. Liu, F. Liu and L. Ren, Curr. Anal. Chem., 2011, 7, 357–364 CrossRef CAS.
- N. Arroyo-Manzanares, A. M. García-Campaña and L. Gámiz-Gracia, Anal. Bioanal. Chem., 2011, 401, 2987–2994 CrossRef CAS.
- M. Asensio-Ramos, J. Hernandez-Borges, T. M. Borges-Miquel and M. A. Rodriguez-Delgado, J. Chromatogr., A, 2010, 1218, 4808–4816 CrossRef.
- M. Soylak and E. Yilmaz, Desalination, 2011, 275, 297–301 CrossRef CAS.
- D. Han, H. Yan and K. H. Row, J. Sep. Sci., 2011, 34, 1184–1189 CrossRef CAS.
- R. S. Zhao, X. Wang, L. L. Zhang, S. S. Wang and J. P. Yuan, Anal. Methods, 2011, 3, 831–836 RSC.
- C. Yao, T. Li, P. Twu, W. R. Pitner and J. L. Anderson, J. Chromatogr., A, 2011, 1218, 1556–1566 CrossRef CAS.
- L. Ye, J. Liu, X. Yang, Y. Peng and L. Xu, J. Sep. Sci., 2011, 34, 700–706 CrossRef CAS.
- H. R. Sobhi, A. Kashtiaray, H. Farahani and M. R. Farahani, J. Sep. Sci., 2011, 34, 77–82 CrossRef CAS.
- F. Kamarei, H. Ebrahimzadeh and Y. Yamini, Talanta, 2010, 83, 36–41 CrossRef CAS.
- Z. Li, Y. Fang, P. Chen, Z. Wang, L. Wang, G. Ren and Y. Huang, Int. J. Environ. Anal. Chem., 2010, 90, 856–868 CrossRef CAS.
- Y. Wang, J. You, R. Ren, Y. Xiao, S. Gao, H. Zhang and A. Yu, J. Chromatogr., A, 2010, 1217, 4241–4246 CrossRef CAS.
- H. Abdolmohammad-Zadeh and G. Sadeghi, Talanta, 2010, 81, 778–785 CrossRef CAS.
- L. He, X. Luo, H. Xie, C. Wang, X. Jiang and K. Lu, Anal. Chim. Acta, 2009, 655, 52–59 CrossRef CAS.
- L. M. Ravelo-Pérez, J. Hernández-Borges, A. V. Herrera-Herrera and M. Á. Rodríguez-Delgado, Anal. Bioanal. Chem., 2009, 395, 2387–2395 CrossRef.
- Q. Zhou, H. Bai, G. Xie and J. Xiao, J. Chromatogr., A, 2008, 1188, 148–153 CrossRef CAS.
- L. Zaijun, C. Peipei, Y. Cuiping, F. Yinjun, W. Zhongyun, L. Ming and S. Haixia, Curr. Anal. Chem., 2009, 5, 324–329 CrossRef.
- H. Bai, Q. Zhou, G. Xie and J. Xiao, Anal. Chim. Acta, 2009, 651, 64–68 CrossRef CAS.
- R. S. Zhao, X. Wang, J. Sun, S. S. Wang, J. P. Yuan and X. K. Wang, Anal. Bioanal. Chem., 2010, 397, 1627–1633 CrossRef CAS.
- J. López-Darias, V. Pino, J. H. Ayala and A. M. Afonso, Microchim. Acta, 2011, 174, 213–222 CrossRef.
- J. Tian, X. Chen and X. Bai, J. Sep. Sci., 2012, 35, 145–152 CrossRef CAS.
|
This journal is © The Royal Society of Chemistry 2012 |
Click here to see how this site uses Cookies. View our privacy policy here.