Multiple barriers for micropollutants in nutrient recovery from centrate – combining membrane bioreactor and electrodialysis†
Received
25th January 2024
, Accepted 22nd May 2024
First published on 24th June 2024
Abstract
Centrate from digested sludge dewatering holds promise for nutrient recovery, but concerns about organic and inorganic contaminants must be addressed. This study investigates the effectiveness of a two-stage system in retaining organic micropollutants, metals, and metalloids during recovery of a multi-nutrient solution from centrate. In combination, the lab-scale membrane bioreactor (MBR) and electrodialysis (ED) effectively reduced contaminant loads by >90% for 21 of the monitored 22 organic micropollutants and for six of nine metals and metalloids. The combined process demonstrated resilience to fluctuations in the MBR stage, with a temporary 87% decrease in MBR removal efficiency for carbamazepine translating to only 6% decrease after the ED. Despite this robust performance, individual compounds such as valsartan acid or benzotriazole were detected at around 10–20 μg L−1 in the recovered nutrient solution. Zn was present at around 400 μg L−1 with the highest concentrations of monitored metals. Still, all metals ranged at least one order of magnitude below recommended values for wastewater reuse. Therefore, the risk associated with contaminant uptake into hydroponically cultivated produce is considered low, given the high retention in the system and the necessary dilution of the multi-nutrient solution before its application as fertilizer. This study demonstrates the effective removal of contaminants by the combination of MBR and ED for nutrient recovery from centrate, achieving a fit-for-purpose quality of the derived multi-nutrient solution.
Water impact
Reclaiming nutrients from centrate, a by-product of digested sludge dewatering, could contribute to sustainable resource management. Achieving fit-for-purpose quality of the recovered nutrient solution for hydroponic use requires effective retention of metals and organic contaminants. Our study demonstrates the combined membrane bioreactor and electrodialysis system's robust contaminant removal, showcasing it as a suitable technology for nutrient recovery.
|
Introduction
Wastewater has been used as a nutrient source in agricultural production for many years,1 and reuse of treated and untreated wastewater is being practiced in most arid countries around the globe to reduce freshwater withdrawal for agriculture.1 The use of soilless cultivation systems, such as hydroponics, can be beneficial due to their enhanced water2 and nutrient efficiency.3 However, complex nutritional media are required for successful hydroponic cultivation, containing both macronutrients and essential micronutrients.4 Anaerobic digestates have been proven to work as sole nutrient sources for hydroponics, as they often contain complex mixtures of micronutrients that are solubilized during the anaerobic process.5 In the context of wastewater treatment, increased attention has been given to the liquid residues of dewatering of digested sewage sludge in wastewater treatment plants (WWTPs), often referred to as centrate, sludge liquor, process water or reject water. This wastewater stream has a high concentration of ammonia-nitrogen and is commonly recycled to the influent of the WWTP, increasing the nitrogen load to be removed during biological treatment.6
However, high concentrations of organic micropollutants as well as metals and metalloids in centrate, which may exceed the concentrations found in the influent of a WWTP,7 impede the reuse e.g. for nutrient recovery.6 Furthermore, high ammonium concentrations present in anaerobic effluents may be toxic to plants.5 Moreover, hydroponics has high requirements for the purity of the nutrient solution, as in soilless cultivation contaminants are in direct contact with the plant root, without a soil buffer that has been shown to bind or to allow degradation of contaminants. Lastly, microbial contamination remaining in the nutrient solution may also pose a threat in hydroponics.5 Particularly, in closed-loop hydroponic systems, the nutrient solution is recycled favoring further enrichment of microbial contamination.5 Therefore, appropriate treatment steps are required to achieve fit-for-purpose quality of the hydroponic nutrient solution recovered from centrate.
The membrane bioreactor (MBR) represents a well-established technology in advanced biological wastewater treatment that has been shown to enhance the removal of organic micropollutants.8,9 Higher solid retention times and therefore high sludge ages can be achieved compared to activated sludge systems.9 Thus, slow-growing microorganisms, e.g. ammonia-oxidizing bacteria (AOB), can be enriched in MBRs.10 Beneficially, the membrane filtration removes microbial contamination.11 Cultivation using MBR-nitrified digestate as a hydroponic growth media has been shown to achieve similar yields to a control medium.12 Furthermore, the positive impact of nitrification on the co-metabolic degradation of organic micropollutants has been discussed extensively.13,14 Moreover, the removal of heavy metals such as Cu and Cr is enhanced in MBRs.8
However, some recalcitrant compounds, e.g. carbamazepine, cannot be completely eliminated in aerated MBR systems,15 and also biological transformation products remain in the MBR permeate as they are formed during biological processes.16 Therefore, further treatment is necessary.
Beyond improving the average quality of the MBR permeate the use of an additional treatment stage can provide improved removal stability following the multi-barrier principle which is applied in drinking water treatment.17 One disadvantage of advanced membrane technologies such as reverse osmosis or nanofiltration that have been successfully used for the removal of organic and inorganic contaminants from MBR effluent, is that they also remove the macronutrients such as nitrate or potassium.18 While tailored NF membranes have been shown to allow for simultaneous recovery of nutrients and retention of micropollutants from synthetic wastewater, their application in real wastewater is yet to be demonstrated.19
Electrodialysis (ED) is a technology that has received increasing attention in recent years for the selective recovery of nutrients and metals from wastewater.20 Moreover, it has been used in industry since the 1950s, mostly in desalination of potable water.21 In ED, anions and cations are transferred from a feed (also referred to as diluate) through ion-exchange membranes to a receiving stream (or concentrate) by use of an electric field.21 Important operational parameters such as electric current density or flow velocity can be modified to improve selectivity of the process.21 Indeed, ED has been successfully applied for the simultaneous extraction of nutrients and removal of micropollutants from centrate.20 While overall removal of organic micropollutants is >90%, attempts to optimize operational parameters (electric current density, feed pH) for enhanced retention proved ineffective.22 Furthermore, ED is faced with challenges related to wastewater reuse, as it is not suitable for removal of particulate or colloidal suspended material.21 Still, it was shown that ED has the capacity to fully disinfect a microbially contaminated feed at high current densities.23 However, it suffers heavily from deposition of organic material (fouling) on the anion exchange membrane and effects of inorganic scaling on the cation exchange membranes at high feed concentrations.24 Using the MBR prior to the ED helps to reduce both organic loads as well as particulate content and is therefore beneficial for ED operation.
In this study, we investigate the efficiency of contaminant removal of a combined MBR and ED treatment system on a laboratory scale during recovery of a multi-nutrient solution from centrate. Specifically, we focus on the efficiency and stability of removal of organic micropollutants, metals, and metalloids. Finally, we analyze the concentrations of these contaminants in the recovered multi-nutrient solution to evaluate its suitability for hydroponic cultivation, regarding the content of organic micropollutants, metals, and metalloids.
Material & methods
The combination of membrane bioreactor (MBR) and electrodialysis (ED) is titled NEWtrient® recovery process and was developed at Fraunhofer UMSICHT. It is envisioned to be part of a holistic transformation of wastewater treatment plants (WWTP) to NEWtrient® Centers for sustainable resource management.
The two-stage lab-scale setup for nutrient recovery consisted of a MBR alongside an ED. It was designed and operated at Fraunhofer UMSICHT. Centrate, the water residue from digested sludge dewatering served as the initial input for the nutrient recovery process. The centrate was sourced from two local municipal WWTPs. During the first phase of the experiment, the centrate was sourced from WWTP Bochum-Oelbach (130
000 p.e.), which treats wastewater with considerable industrial contribution. During the second phase, centrate was obtained from the WWTP Dinslaken (63
000 p.e.), which serves a predominantly residential sewer catchment. In both cases, the centrate was obtained monthly and stored at ambient temperature in high-density polyethylene (HDPE) containers until processing for nutrient recovery.
The MBR was operated in semi-batch mode. It received a batch-wise influx of raw centrate from the storage container, while the permeate was withdraw continuously. The ED was operated in batches. During the process, every 10 liters of centrate yielded 10 liters of MBR permeate, which was further processed into 1.5–2.0 liters of ED concentrate which is the multi-nutrient solution.
Setup and operation
Membrane bioreactor.
Fig. 1 shows a schematic of the lab-scale setup of the two-stage nutrient recovery system. A 5 liter glass vessel served as the bioreactor, housing probes for monitoring pH, temperature (T), and dissolved oxygen concentration (DO). A gas flow control was used to maintain DO concentration above 2–3 mg L−1, thereby preventing any limitation on the nitrification process. Continuous agitation of the reactor contents was achieved through a combination of gas bubbles and an overhead stirrer. Centrate was intermittently introduced into the reactor once the filling level dropped below a predetermined threshold. The MBR permeate was produced by filtration of the reactor's mixed liquor with four sidestream polymeric ultra-filtration modules (Type MO P1U(0.5 m)-I8LE, Berghof Membrane Technology GmbH, Eningen, Germany). The mixed liquor was continuously recirculated through the sidestream filtration channel at of 50 L h−1 by a peristaltic pump (630 L, Watson-Marlow Fluid Technology Solutions, Falmouth, UK).
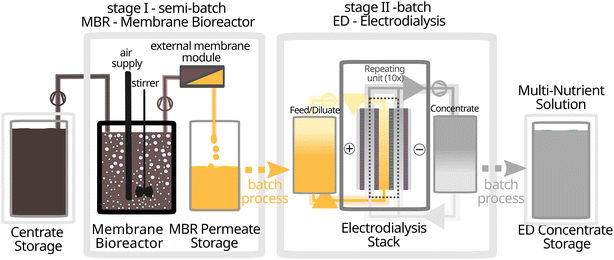 |
| Fig. 1 Experimental setup of the two-stage nutrient recovery system with storage of centrate as well as the final ED concentrate at room temperature. | |
The MBR permeate was collected in HDPE containers and stored at room temperature until subsequent treatment with the electrodialysis. Average operational parameters are provided in Table 1.
Table 1 Operational parameters of the membrane bioreactor
|
Average values |
HRT [h] |
∼70 |
SRT [d] |
209–221 |
Flux [mL min−1] |
2.4–4.8 |
TSS g L−1 |
2.9–4.0 |
Volatile suspended solids VSS [%] |
62–87 |
DO [mg L−1] |
2–3 |
pH |
6–7 |
Electrodialysis unit.
The MBR permeate underwent treatment using a laboratory-scale electrodialysis system (ED 64002, PCCell GmbH, Hausweiler, Germany) comprising 10 pairs of cation exchange membranes (CEM) and anion exchange membranes (AEM). The CEM (PC SK-ED 64002, PCCell GmbH) was a sulfonic acid-based ion exchange membrane with an approximate water content of 9%. The AEM (PC SA-ED 64002, PCCell GmbH) employed an ion exchange membrane with quaternary amine functional groups and a water content of about 14%. The total effective membrane area was 1280 cm2 (64 cm2 per membrane). Both the AEM and CEM measured 100 to 120 μm in thickness. Spacers made of polypropylene (PP) were utilized to maintain a channel thickness of 450 μm. To ensure durability, robust CEM end-membranes (PC MTE ED 64002/64004 MT6644) based on sulfonic acid were deployed in the electrolyte chambers at the electrodes. The anode and cathode in the endplates of the membrane stack consisted of wire meshes made of Pt/Ir-coated titanium and V4A steel, respectively. The electric current was driven by a programmable power supply (9181B, B&K Precision Corporation, Yorba Linda, USA). Electrical conductivity (SE-204, Knick, Berlin, Germany) and pH (Inpro 4260/PT1000, Mettler-Toledo GmbH, Gießen, Germany) were continuously monitored in the diluate and concentrate fluid circuits.
For processing in the ED setup, the MBR permeate was used as starting diluate and deionized water as the starting concentrate. The system was operated at a current density of about 3.1 mA cm−2 (31 A m−2), with 250 mM K2SO4 as electrolyte. Diluate and concentrate streams were continuously recirculated through the membrane stack until the electrical conductivity (EC) of concentrate and diluate reached a ratio of 5. As the concentration of nutrient ions present is proportional to the EC both in the diluate and concentrate, the EC ratio also corresponds to a concentration factor of the nutrients.
Sampling procedure.
Centrate samples were obtained approximately once per week from the storage container when the feed supply to the MBR was restocked. Due to the high particulate content of centrate, thorough mixing was necessary before sampling. Each permeate tank was treated in batch in the ED. After completion of each batch the ED concentrate samples were obtained. Samples were stored frozen at −20 °C until further pre-treatment.
Sample preparation and instrumental analysis.
Centrate, MBR permeate, and ED concentrate were thawed and filtered (0.45 μm, regenerated cellulose) at UFZ directly before analysis. Experiments to determine filter recovery showed acceptable recoveries >95% for most organic micropollutants (Table S1†) as well as metals and metalloids (Table S2†). Prior to LC–MS/MS analysis, samples were diluted 1
:
10 in Milli-Q water and spiked with 10 μL mL−1 of a labeled internal standard mix. Samples for ICP-MS analysis were diluted 1
:
10 in HNO3 (1%) before analysis.
For the determination of organic micropollutants, samples were analyzed via LC-ESI-MS/MS using an Agilent 1260 Infinity series HPLC system (Agilent Technologies, Waldbronn, Germany). An Atlantis T3 column (2.1 × 100 mm, Waters Corp., Milford, USA) equipped with a SecurityGuard C18 guard column (4 × 2.0 mm; Phenomenex) was utilized, in conjunction with a QTRAP 5500 mass spectrometer (Sciex, Darmstadt, Germany). Chromatographic separation parameters were adopted from Kahl et al., 2017 (ref. 25) and are outlined in Table S4.† Mass spectrometry parameters are detailed in Table S4.† Electrospray ionization operated in both positive and negative modes. Analytes were identified using scheduled multiple reaction monitoring (sMRM) based on two mass transitions and retention time. Available isotopically labeled standards were used to compensate for matrix effects for compounds specified in Table S5.†
For trace elemental analysis of heavy metals and selected metalloids, an iCAP Qs ICP-MS system (Thermo Scientific, Waltham, USA) was employed. Concentrations of more prevalent elements were determined by ICP-AES using an ICP-720-ES system (Varian Inc, Palo Alto, USA).
Choice of micropollutants
Organic micropollutants.
In this study, 22 micropollutants, that were identified in centrate,7 were monitored throughout the nutrient recovery process. A detailed list of the examined compounds can be found in Table S6.† We note that, data for melamine was excluded due to its observed leaching from the ultra-filtration membranes utilized in the membrane bioreactor, leading to non-representative results. The majority of the investigated compounds possess a low molecular weight (<300 Da) and exhibit hydrophilic characteristics (Log
D(pH=7) < 2). These properties are positively associated with the potential for plant uptake,26 which may occur when a recuperated nutrient solution is employed in plant cultivation. Chemical database access, structure-based property calculation, search, and reporting were facilitated using JChem for Office (Excel) version 21.1.0.787, 2021, developed by ChemAxon (https://www.chemaxon.com).
Metals and metalloids.
A wide range of metals and metalloids was analyzed, encompassing those specified in the WHO guidelines for safe wastewater reuse.27 Additionally, the study encompassed elements outlined in the preparatory study to the EU regulation for wastewater reuse,28 which aligns with directives from the EU water framework, as well as regulations concerning foodstuffs “EU Regulation on Foodstuffs” (2006)29 and the application of sewage sludge in agriculture “EU Directive Sewage Sludge in Agriculture” (1986).30
Calculation of elimination.
The load of organic micropollutants, metals and metalloids in the dissolved phase was used to calculate the elimination in the individual treatment stages of MBR and ED (eqn (1)). |  | (1) |
From eqn (1) a negative elimination may be derived. This is found when the dissolved output load of an individual treatment stage is higher than its dissolved input load. Negative elimination may be observed, e.g. when micropollutants are formed during biological processes or desorption from the particulate matter leads to an increased dissolved concentration at the output of the treatment stage.
Results & discussion
Characteristics of MBR input
Centrate from wastewater treatment plants (WWTPs) was used as input for the two-stage nutrient recovery system. The centrate was obtained from the dewatering of digested sludge by centrifuging. The nutrient recovery system consisting of a membrane bioreactor (MBR) and an electrodialysis (ED) was operated in two phases that were approximately a year apart. Centrate of different sources was used in each phase: in the first phase, the centrate (centrate I) was obtained from a WWTP treating wastewater with a considerable industrial contribution, while in the second, centrate (centrate II) originated from a WWTP serving a predominantly residential area. Of the 22 micropollutants studied, the highest median concentrations were determined for the antihypertonic valsartan at around 31 μg L−1, followed by the corrosion inhibitor benzotriazole (20 μg L−1) (Fig. 2).
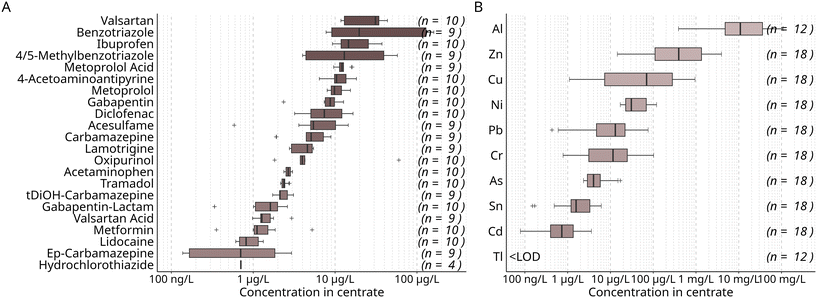 |
| Fig. 2 Median concentration of organic micropollutants (A) and metals and metalloids (B) in the centrate used as feed for the nutrient recovery process aggregated over the study period. Samples were obtained directly prior to treatment in the MBR (n = number of detects in 10 (A) and 18 (B) samples). Concentrations distinguished for phases I and II are shown in Table S7.† | |
Several compounds were found at significantly higher concentrations in centrate I (Table S7†). In centrate I, benzotriazole and 4/5-methylbenzotriazole were found at median concentrations of 130 μg L−1 and 40 μg L−1, compared to 8 μg L−1 and 4 μg L−1 in centrate II, respectively. Still, median concentrations found in centrate in this study (Fig. 2) were considerably lower than those found in a previous study in centrate from a WWTP treating mostly household waters, but also street run-off,7 which indicates that the concentrations of organic micropollutants in centrate vary depending on many factors, such as the characteristics of the sewer catchment and the operation of the dewatering process. For example, it was observed that higher dosing of flocculant before centrifugation resulted in higher dissolved micropollutant concentrations in centrate7 Consequently, considering source-dependent differences in centrate quality, the dewatering process may be modified to achieve lower micropollutant concentrations, if feasible.
Elimination of organic micropollutants in MBR + ED
Overall elimination of organic micropollutants in the two-stage nutrient recovery process was >90% for 21 of the investigated 22 organic micropollutants (Fig. 3). Complete removal was observed for ibuprofen, acetaminophen, valsartan, 4-acetoaminoantipyrine as well as hydrochlorothiazide. The small and polar compounds metformin, benzotriazole, and 4/5-methylbenzotriazole still showed an average removal above 95%. They also exhibited strong fluctuations (>50%) in the study period (Fig. 3). The lowest removal (74 ± 21%) was found for lidocaine. Moreover, the effectiveness of removal in the individual stages of the nutrient recovery process was compound-specific: some compounds were completely eliminated in the MBR stage, while for most other compounds the subsequent ED stage was required to achieve a total removal of >90% (Fig. 3).
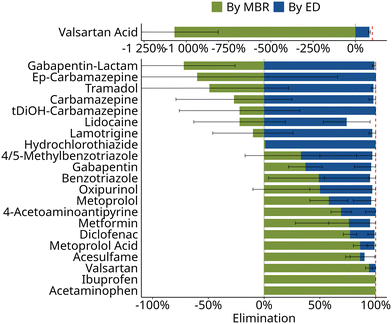 |
| Fig. 3 Calculated elimination in the membrane bioreactor and retention in the electrodialysis of organic micropollutants aggregated across phase I and phase II. For individual compounds elimination, distinguished in both phases, is shown in Fig. 4. The dashed line indicates 100% elimination. The calculation of elimination is based on eqn (1). | |
Membrane bioreactor.
In the MBR stage, seven of the investigated micropollutants showed a removal of >75%. Complete removal, however, was observed only for the analgesic acetaminophen and the anti-inflammatory drug ibuprofen (Fig. 3). Literature shows that elimination in MBR systems is compound-specific and bio-transformation as well as sorption to the sludge biomass are its main drivers.31 Sorption to or rejection by the UF membranes plays only a minor role.32 For the rather hydrophilic micropollutants considered in this study (Log
D(pH=6) < 3) the contribution of adsorption to the retention is expected to be less relevant.33 For organic cations, adsorption to the negatively charged sludge flocs can play a role in MBR systems.34 However, removal in the MBR stage was observed for only one of the cations, metoprolol, but not for lamotrigine and lidocaine (Fig. 3). Therefore, the removal observed in the MBR stage in this study is, likely, primarily due to biological transformation.
The microbial degradation of micropollutants is reported to occur either via catabolic or co-metabolic processes.35 Compared to conventional activated sludge systems, MBRs can enhance the removal of micropollutants with medium bio-biodegradability.9 When comparing micropollutant removal in the MBR in this study to literature data, it is important to consider the influence of nitrifying conditions in the system. It has been shown that the transformation of compounds with medium bio-degradability such as diclofenac can be improved through co-metabolic degradation by nitrifiers36 due to the expression of enzymes of low substrate-specificity that can degrade a broad spectrum of micropollutants.37 Indeed, complete nitrification was achieved in the present study (Table S8†) and simultaneously 80% of diclofenac is removed in the MBR (Fig. 3), while removal in conventional activated sludge systems does not exceed 15%.33 The importance of co-metabolic transformation under nitrifying conditions has also been demonstrated for benzotriazole in mixed culture studies and lab reactor studies with around 40% removal under nitrifying conditions.37 Studies in pilot scale and full-scale have reported on average 60% (ref. 38) and 25% (ref. 39) removal of benzotriazole at SRTs of 26 to 105 days and 35 to 40 days, respectively. These removal rates are consistent with the results obtained here, where benzotriazole removal in the MBR stage reached 50% (Fig. 3).
Complete removal of ibuprofen and acetaminophen in the MBR stage was observed. Both compounds are highly bio-degradable and well removed in MBR systems.16,36 Additionally, ibuprofen is completely co-metabolically transformed by AOB36 which might have played a role in this study.
Not all compounds were removed similarly well in the MBR stage. For instance, carbamazepine showed negative median removal rate. This agrees well with previous studies showing that removal of carbamazepine through the combination of sorption and bio-transformation did not exceed 10%,33 independent of nitrifying co-metabolism.36 Increased dissolved concentrations of carbamazepine which were partly observed, may be explained by changing sludge floc characteristics during nitrifying conditions in the MBR stage which lead to desorption of the low amount of initially adsorbed carbamazepine.40
After the MBR stage, the median concentration of organic micropollutants in the permeate was <5 μg L−1 for all studied compounds but candesartan (16 μg L−1) and valsartan acid (17 μg L−1) (Table S7†). While concentrations were reduced for most compounds, concentrations of biological transformation products such as valsartan acid, gabapentin-lactam, or ep-carbamazepine increased in the MBR, making a subsequent treatment step necessary.
Electrodialysis.
The ED is the final treatment stage of the nutrient recovery system. It showed >90% rejection for 20 of the investigated compounds (Fig. 3 and Table S9†). The retention in the ED stage was below 90% only for three compounds: valsartan acid (83 ± 6%), metformin (78 ± 4%), and lidocaine (74 ± 21%).
In principle, in ED only small ions should be capable of migrating to the concentrate in response to the applied electric field. Accordingly, small ionic micropollutants such as valsartan acid, metformin, and lidocaine showed retention rates lower than 90% during ED, which was the lowest of all compounds investigated. While literature data on these three compounds is scarce, one previous study confirmed lower retention; and even showed that transport of metformin may reach up to 60% of the (unhindered) transport of inorganic salts through the membrane in ED treatment of a synthetic wastewater.22 Moreover, in this study, retention of metoprolol was also incomplete at 90%, similar to previous studies.20 However, as long as inorganic salts are present in the feed, which take the role of charge carriers, they will be preferentially transported compared to organic ions.41 Consequently, in this study, the ED stage proved suitable to recover e.g. nitrate or potassium while retaining most of the less preferentially transported micropollutants.
Neutral molecules, on the other hand, should largely remain in the diluate, since they can only be transported across ion exchange membranes by passive processes such as diffusion or electro-convective water transport,42 whose contribution to transport is minor compared to the active electro-migration.43 Moreover, sorption onto the membrane surface by either hydrophobic or electrostatic interaction has been shown to enhance the retention of neutral compounds.41 Accordingly, in this study the neutral compounds carbamazepine and benzotriazole are well retained in ED at retention rates of 98% and 91%, respectively (Fig. 3 and Table S9†). High retention of carbamazepine was also reported in lab-scale ED treatment of synthetic spiked centrate.20,22
Fluctuations in removal and complementarity of barriers
Fig. 3 shows that only after the second treatment stage stable and near-complete retention of organic micropollutants is achieved: the two stages act together as complementary barriers. For some compounds high standard deviations are observed, which mostly resulted from variations in the elimination performance of the MBR stage (Fig. 3). Between phase I and II of the study, the MBR required the complete re-inoculation with nitrifying sludge. Additionally, the source of the centrate was changed. Even after the stabilization of the biological nitrification process (Table S8†), a weaker elimination of carbamazepine (ΔElimination,MBR = −87%) and benzotriazole (ΔElimination,MBR = −74%) in the MBR stage was observed in phase II compared to phase I. However, the total removal in the two-stage system (ΔElimination,Total) remained stable (change of −6% and −4% for carbamazepine and benzotriazole, respectively) as the ED stage was able to compensate for the reduced MBR elimination (Fig. 4). Conversely, for the ionic compound lidocaine, total removal was significantly decreased (from 77% in phase I to 52% in phase II) as the ED was only partly able to compensate for the weaker performance of the MBR (ΔElimination,MBR = −70%). This suggests that the transport of neutral compounds in the ED was independent of the feed concentration, unlike for ions where increasing feed concentrations yielded higher effluent concentrations. Therefore, the ED acted as a complementary barrier for neutral compounds, while reduced removal of ionic compounds in the MBR stage could not be fully compensated in the ED.
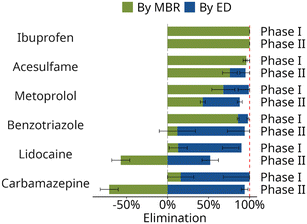 |
| Fig. 4 Calculated elimination in the membrane bioreactor and the electrodialysis of organic micropollutants with strong differences between phase I and phase II, plus ibuprofen. All data can be found in Fig. S1.† | |
Elimination of heavy metals in MBR + ED
The two-stage treatment system also retained metals and metalloids effectively: for six of the nine elements, retention was >90% (Fig. 5), with 99% retention of Al, Cu, and Pb. The lowest overall retention in the combined system was observed for zinc with 71%.
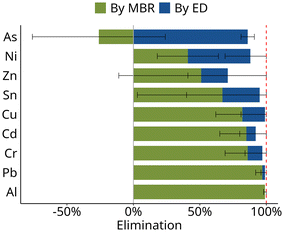 |
| Fig. 5 Calculated elimination in the membrane bioreactor and retention in the electrodialysis of heavy metals and metalloids aggregated across phase I and phase II. The dashed line indicates 100% elimination. | |
Membrane bioreactor.
In the MBR stage removal of >90% for Cd, Cr, Cu, Pb; of >40% for Ni, Zn; and <0% for As correspond well or even exceeds removal reported in literature, especially for Pb for which 40% removal has been reported previously.8 In this study, complete removal was observed, which is consistent with the 96% removal reported by.44
High removal was observed especially for metals and metalloids that have been reported to partition to the sludge phase such as Al or Pb (Fig. 5). Consequently, high mixed liquor suspended solids concentration improves removal of metals in the MBR.8
Lower retention in the MBR is expected for dissolved metal ions, as their hydration shells are usually smaller than the pore size (10–20 nm) of UF membranes used in MBR processes.45 Indeed, for Ni and Zn only 40% and 50% retention were observed in the MBR in this study. Interestingly, the presence of organic material in the MBR has been shown to increase the retention of those metals, as they are more likely rejected by the membrane pores when chelated or bound in a metal–organo-complex.46
The concentration of arsenic, the only metalloid monitored, increased in the MBR. This suggests that it was solubilized during the oxidative nitrification in the MBR. Studies have shown that As(III) – arsenite – is oxidized to As(V) – arsenate – during biological nitrification.47 While arsenite, which occurs ins neutral form as As(OH)3, may still be adsorbed to the sludge matrix, oxidation to As(V) which occurs as an anion will lead to stronger partitioning to the liquid phase and thus higher dissolved concentrations after the MBR. However, as speciation analysis was not performed in this study this explanation remains speculative.
In summary, metals that partition to the liquid phase, such as Ni, As, and Zn, are retained in the MBR to a smaller extent compared to those that adsorb on the biomass (Fig. 5). Thus, for removal of more soluble metals and metalloids, the electrodialysis is needed as a second treatment stage after the MBR.
Electrodialysis.
The retention of metals and metalloids in the ED stage showed a heterogeneous picture. While Cu and Cr were retained completely and retention of As was as high as 85% in the ED, less than 50% of the incoming Cd or Zn were retained in the ED stage in this study (Fig. 5).
In literature, studies on metal and metalloids in ED are often related to the recovery of metals from highly concentrated wastewater, e.g. from electroplating or mining residues.48 Near-complete removal rates (recovery = transfer into the concentrate stream = removal) are reported for most of metals including all that were investigated in this study.48 Specifically, recovery rates >90% for Cd, Cr, Cu, Fe, Ni, and Zn are reported.48 Moreover, the recovery of As from metallurgical effluent has been reported to reach >90%.49 Only few studies report suboptimal (>70%) recovery e.g. for Ni in ED.50
Without exception, the retention of metals and metalloids in the ED treatment of this study was lower compared to values reported in literature. Two factors may explain this lower retention: flow rates reported for most metal recovery processes are between 0.005 to 7 L h−1 and electric current densities ranged from 0.2 to 20 mA cm−2,48 while the ED system used here was operated at higher diluate and concentrate recirculation rates (20 to 60 L h−1) and also, the electric current density (3.1 mA cm−2) used in this study, was in the lower range compared to most metal recovery processes. Furthermore, median concentrations of all but two metals were below 100 μg L−1 in the centrate (Fig. 2B), and thus significantly lower than those found in metallurgical wastewaters (>5 mg L−1 for Cd, Ni, Cr, and As >50 mg L−1 or Zn, and Cu >100 mg L−1).50 Indeed, low metal concentrations in the feed have been shown to result in lower retention in ED and optimal recovery was predicted only for feed concentrations of 500 mg L−1.48
Quality of the multi-nutrient solution derived from ED
The ED concentrate is the final product of the two-stage process. As a multi-nutrient solution, it is the basis for a liquid fertilizer for hydroponic applications. In the concentrate, 18 of the 22 monitored organic micropollutants were found to be reduced to median concentrations ≤1 μg L−1 (Fig. 6). Only four compounds – valsartan acid, metoprolol, acetaminophen, and benzotriazole – significantly exceeded 1 μg L−1, with valsartan acid reaching a median concentration of 11 μg L−1 (Fig. 6).
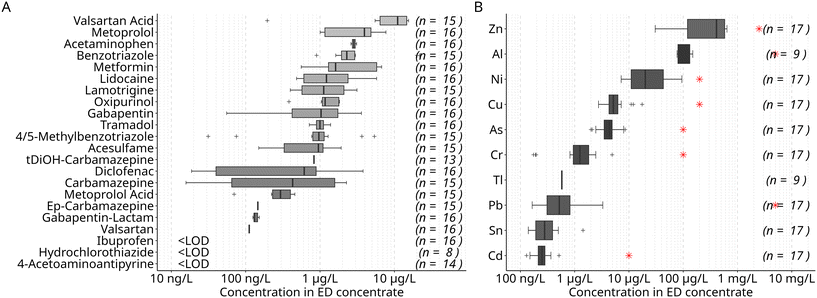 |
| Fig. 6 Concentration of organic micropollutants (A) and metals and metalloids (B) in the electrodialysis concentrate derived as multi-nutrient solution by the nutrient recovery process (n = number of detects in 16 (A) and 17 (B) samples). Red crosses indicate the maximum concentration of metals and metalloids recommended for wastewater reuse.27 | |
We note that concentrations of some individual micropollutants varied significantly across concentrate batches. For instance, benzotriazole, whose median concentration was 2 μg L−1, was found at concentrations as high as 20 μg L−1 in some batches. As mentioned above, specific process parameters such as flow rate, applied current density, or water transfer rate in the ED influence elimination, which, in turn, may result in varying micropollutant concentrations in ED concentrate.
Since 2020 the reuse of treated wastewater for agricultural purposes has been regulated by the ref. 51. However, as threshold values for the concentration of organic pollutants have not yet been determined, a risk-assessment approach is therefore required to verify the suitability of the ED concentrate as a liquid fertilizer. It is important to consider that in its intended application in hydroponic systems, the ED concentrate will be diluted to be used as a hydroponic nutrient solution. The choice of dilution factor depends on the plant-specific optimal composition and is determined based on the concentrations of macronutrients (i.e., P, N, and K) and their ratio. Dilution factors of 100 to 1000 have been applied in experiments using the ED concentrate produced in this study (unpublished work). Therefore, most monitored micropollutants were present at concentrations around 1 ng L−1 in the nutrient solution that will come into direct contact with the plants.
Uptake of micropollutants into plants irrigated with wastewater has been studied extensively in field studies.52 While in soil-based cultivation the soil may act as an additional sink for micropollutants, no such barrier is present in hydroponic systems. Enhanced uptake of diclofenac has been observed in tomato plants grown in soilless systems.53 Micropollutant uptake may result in a health risk, e.g. carbamazepine uptake may result in metabolization in the plant tissue to the geno-toxic 10,11-epoxicarbamazepine.54 However, using the TTC approach55 to estimate the associated risk, Riemenschneider et al., 2016 (ref. 52) estimated >300 ng gdry weight−1 of carbamazepine in edible parts of lettuce to be no cause of concern. These results suggest that, at the concentrations found in the ED concentrate, organic micropollutants should similarly not be problematic.
Metals and metalloids were present in the ED concentrate in concentrations ranging over several orders of magnitude. While the lowest median concentrations in ED concentrate were found for Tl, Cd, Sn (<100 ng L−1), the highest was found for Zn at around 400 μg L−1. Interestingly, Al, which was almost completely retained in the MBR stage (99%), was still present at concentrations of around 100 μg L−1 in the ED concentrate (Fig. 6). Due to its presence in flocculants, high concentrations (around 100 mg L−1) are present in the centrate used in the experiments reported here, which leads to elevated concentrations also in the ED concentrate.
However, concentrations of metals and metalloids in the ED concentrate are well below the recommended maximum concentration in wastewater used for fertilizing plants as defined by WHO (Fig. 6).27 The median concentrations in centrate were about two orders of magnitude lower than the maximum level, except for Zn with only one order of magnitude difference. Cu and Al concentrations did exceed the recommended maximum in individual batches of centrate, but, when considering the dilution of the ED concentrate prior to its use as hydroponic nutrient solution, all batches of ED concentrate comply with the recommended maximum concentrations. Therefore, no risk should be associated with metal uptake in plants from this nutrient solution.
To verify the compliance of the studied treatment technology with current EU legislation on minimum requirements for water reuse, the safety of the applied water reclamation technology should be investigated on an individual case basis. Therefore, to ensure safety of use of the multi-nutrient solution in hydroponic cultivation, further studies into this nutrient recovery system should focus on the potential uptake of micropollutants into edible parts of the cultivated plant tissue to verify the possibility of risk-free consumption.
Conclusion
In this study, we showed that the combination of MBR and ED is an effective system for the safe recovery of nutrients from centrate, achieving >90% of removal for 21 out of the 22 investigated organic micropollutants and for six out of nine metals and metalloids. Fluctuations in the removal by the MBR were compensated near completely by the removal performance of ED for neutral and, to a lesser extent, ionic compounds. In the recovered multi-nutrient solution, median concentrations of organic micropollutants did not exceed 10–20 μg L−1. While Zn was present at around 400 μg L−1 in the multi-nutrient solution, other metals and metalloids were present at <100 μg L−1, thereby remaining safely under the maximum recommended values for agricultural wastewater reuse.
Therefore, the combination of MBR and ED appears to be a suitable combination for safe nutrient recovery from centrate, as it can consistently achieve high removal rates for micropollutants, metals and metalloids. The combination of removal mechanisms at play in the MBR (sorption, bio-degradation) and ED (size-exclusion, retention of neutral compounds) are suited to retain a broad spectrum of micropollutants. The ED proved to be a suitable last stage for the treatment system, as it does not require the addition of chemicals, and does not lead to formation of transformation products. The quality of recovered multi-nutrient solution is likely to be appropriate fit-for-purpose for the intended use as hydroponic nutrient solution, as it will be diluted prior to its use, further reducing the risk of remaining micropollutants, metals and metalloids.
Data availability
Data will be made available on request.
Author contributions
Conceptualization: Anna Hendrike Hofmann, Victor Takazi Katayama, Paul Genz, Thorsten Reemtsma. Methodology: Anna Hendrike Hofmann, Victor Takazi Katayama, Paul Genz. Formal analysis: related to operation of MBR & ED: Anna Hendrike Hofmann, Victor Takazi Katayama. Fate of organic and inorganic contaminants in MBR & ED: Paul Genz. Investigation: related to operation of MBR & ED: Victor Takazi Katayama, Anna Hendrike Hofmann. Fate of organic and inorganic contaminants in MBR & ED: Paul Genz. Resources: related to operation of MBR & ED: Victor Takazi Katayama, Anna Hendrike Hofmann. Fate of organic and inorganic contaminants in MBR & ED: Thorsten Reemtsma. Data curation: Anna Hendrike Hofmann, Victor Takazi Katayama, Paul Genz. Writing – original draft: Paul Genz. Writing – review & editing: Anna Hendrike Hofmann, Victor Takazi Katayama, Paul Genz, Thorsten Reemtsma. Visualization: Paul Genz. Supervision: Thorsten Reemtsma, Victor Takazi Katayama. Project administration: Thorsten Reemtsma. Funding acquisition: Thorsten Reemtsma.
Conflicts of interest
There are no conflicts to declare.
Acknowledgements
This study was financed in part by the German Ministry for Education and Research (BMBF) through the project “Entwicklung eines nachhaltigen Kultivierungssystems für Nahrungsmittel resilienter Metropolregionen SUSKULT” (FKz: 031B0728A/M). We are grateful to Madlen Zeuschner, Karsten Marien and Daniel Kolb (UFZ) for the analysis by ICP-MS, ICP-OES, IC and photometry.
References
- Water reuse: an international survey of current practice, issues and needs, in Scientific and technical report No 20, ed. B. Jiménez, IWA Publishing, London, 2008, p. 628 Search PubMed
.
- G. Barbosa, F. Gadelha, N. Kublik, A. Proctor, L. Reichelm and E. Weissinger,
et al. Comparison of Land, Water, and Energy Requirements of Lettuce Grown Using Hydroponic vs. Conventional Agricultural Methods, Int. J. Environ. Res. Public Health, 2015, 12(6), 6879–6891 CrossRef PubMed
.
- P. N. Carvalho, Y. Zhang, T. Lyu, C. A. Arias, K. Bester and H. Brix, Methodologies for the analysis of pesticides and pharmaceuticals in sediments and plant tissue, Anal. Methods, 2018, 10(30), 3791–3803 RSC
.
-
Hydroponics – A Standard Methodology for Plant Biological Researches [Internet], ed. T. Asao, InTech, 2012 [cited 2023 Oct 27], Available from: http://www.intechopen.com/books/hydroponics-a-standard-methodology-for-plant-biological-researches (accessed: 2024-04-19) Search PubMed
.
- D. Ronga, L. Setti, C. Salvarani, R. De Leo, E. Bedin and A. Pulvirenti,
et al. Effects of solid and liquid digestate for hydroponic baby leaf lettuce (Lactuca sativa L.) cultivation, Sci. Hortic., 2019, 244, 172–181 CrossRef CAS
.
- C. Karmann, A. Mágrová, P. Jeníček, J. Bartáček and V. Kouba, Advances in nitrogen removal and recovery technologies from reject water: Economic and environmental perspectives, Bioresour. Technol., 2024, 391, 129888 CrossRef CAS PubMed
.
- P. Genz and T. Reemtsma, Polar Micropollutants and Metals in Centrate from Dewatered Sewage Sludge Intended for Reuse in Soilless Horticulture, ACS ES&T Water, 2022, 2(12), 2548–2557 Search PubMed
.
- D. Bolzonella, F. Fatone, S. Di Fabio and F. Cecchi, Application of membrane bioreactor technology for wastewater treatment and reuse in the Mediterranean region: Focusing on removal efficiency of non-conventional pollutants, J. Environ. Manage., 2010, 91(12), 2424–2431 CrossRef CAS PubMed
.
- S. Weiss and T. Reemtsma, Membrane bioreactors for municipal wastewater treatment – a viable option to reduce the amount of polar pollutants discharged into surface waters?, Water Res., 2008, 42(14), 3837–3847 CrossRef CAS
.
- A. Kruglova, A. Mikola, A. Gonzalez-Martinez and R. Vahala, Effect of sulfadiazine and trimethoprim on activated sludge performance and microbial community dynamics in laboratory-scale membrane bioreactors and sequencing batch reactors at 8 °C, Biotechnol. Prog., 2019, 35(1), e2708 CrossRef
.
- T. Melin, B. Jefferson, D. Bixio, C. Thoeye, W. De Wilde and J. De Koning,
et al. Membrane bioreactor technology for wastewater treatment and reuse, Desalination, 2006, 187(1–3), 271–282 CrossRef CAS
.
- O. P. Lind, M. Hultberg, K. J. Bergstrand, H. Larsson-Jönsson, S. Caspersen and H. Asp, Biogas Digestate in Vegetable Hydroponic Production: pH Dynamics and pH Management by Controlled Nitrification, Waste Biomass Valoriz., 2021, 12(1), 123–133 CrossRef
.
- K. Fenner and Y. Men, Comment on “Role of Ammonia Oxidation in Organic Micropollutant Transformation during Wastewater Treatment”: Overlooked Evidence to the Contrary, Environ. Sci. Technol., 2021, 55(17), 12128–12129 CrossRef CAS PubMed
.
- Q. Su, A. R. Schittich, M. M. Jensen, H. Ng and B. F. Smets, Role of Ammonia Oxidation in Organic Micropollutant Transformation during Wastewater Treatment: Insights from Molecular, Cellular, and Community Level Observations, Environ. Sci. Technol., 2021, 55(4), 2173–2188 CrossRef CAS
.
- F. I. Hai, X. Li, W. E. Price and L. D. Nghiem, Removal of carbamazepine and sulfamethoxazole by MBR under anoxic and aerobic conditions, Bioresour. Technol., 2011, 102(22), 10386–10390 CrossRef CAS
.
- J. Quintana, S. Weiss and T. Reemtsma, Pathways and metabolites of microbial degradation of selected acidic pharmaceutical and their occurrence in municipal wastewater treated by a membrane bioreactor, Water Res., 2005, 39(12), 2654–2664 CrossRef CAS
.
- E. L. Marron, W. A. Mitch, U. Von Gunten and D. L. Sedlak, A Tale of Two Treatments: The Multiple Barrier Approach to Removing
Chemical Contaminants During Potable Water Reuse, Acc. Chem. Res., 2019, 52(3), 615–622 CrossRef CAS
.
- M. Racar, D. Dolar, K. Karadakić, N. Čavarović, N. Glumac and D. Ašperger,
et al. Challenges of municipal wastewater reclamation for irrigation by MBR and NF/RO: Physico-chemical and microbiological parameters, and emerging contaminants, Sci. Total Environ., 2020, 722, 137959 CrossRef CAS PubMed
.
- Y. Zhao, X. Tong and Y. Chen, Fit-for-Purpose Design of Nanofiltration Membranes for Simultaneous Nutrient Recovery and Micropollutant Removal, Environ. Sci. Technol., 2021, 55(5), 3352–3361 CrossRef CAS PubMed
.
- K. Arola, A. Ward, M. Mänttäri, M. Kallioinen and D. Batstone, Transport of pharmaceuticals during electrodialysis treatment of wastewater, Water Res., 2019, 161, 496–504 CrossRef CAS
.
-
L. Gurreri, A. Cipollina, A. Tamburini and G. Micale, Electrodialysis for wastewater treament—Part I: Fundamentals and municipal effluents, in Current Trends and Future Developments on (Bio-) Membranes [Internet], Elsevier, 2020 [cited 2023 Aug 9], pp. 141–192, Available from: https://linkinghub.elsevier.com/retrieve/pii/B9780128168233000071 Search PubMed
.
- P. Genz, V. T. Katayama and T. Reemtsma, Retention of Organic Micropollutants in Nutrient Recovery from Centrate by Electrodialysis–Influence of Feed pH and Current Density, ACS ES&T Water, 2023, 3(12), 4066–4073 Search PubMed
.
- T. Sato, M. Akiba, T. Suzuki and H. Ohya, Disinfection of River Water Using an Electrodialytic Disinfection System, Nippon Kaisui Gakkaishi, 1997, 3, 113–118 Search PubMed
.
- M. A. Andreeva, V. V. Gil, N. D. Pismenskaya, L. Dammak, N. A. Kononenko and C. Larchet,
et al. Mitigation of membrane scaling in electrodialysis by electroconvection enhancement, pH adjustment and pulsed electric field application, J. Membr. Sci., 2018, 549, 129–140 CrossRef CAS
.
- S. Kahl, J. Nivala, M. van Afferden, R. A. Müller and T. Reemtsma, Effect of design and operational conditions on the performance of subsurface flow treatment wetlands: Emerging organic contaminants as indicators, Water Res., 2017, 125, 490–500 CrossRef CAS PubMed
.
- M. Lamshoeft, Z. Gao, H. Resseler, C. Schriever, R. Sur and P. Sweeney,
et al. Evaluation of a novel test design to determine uptake of chemicals by plant roots, Sci. Total Environ., 2018, 613–614, 10–19 CrossRef CAS PubMed
.
-
WHO Guidelines for the safe use of wastewater, excreta and greywater, World Health Organization, Geneva, 2006, p. 182 Search PubMed
.
-
L. Alcalde-Sanz and B. M. Gawlik, Minimum quality requirements for water reuse in agricultural irrigation and aquifer recharge: Towards a legal instrument on water reuse at EU level, Publications Office of the European Union, 2017 Search PubMed
.
-
EU Regulation on Foodstuffs [Internet], (EC) No 1881/2006 Dec 19, 2006, Available from: https://eur-lex.europa.eu/LexUriServ/LexUriServ.do?uri=OJ:L:2006:364:0005:0024:EN:PDF (accessed: 2024-04-19) Search PubMed.
-
EU Directive Sewage Sludge in Agriculture [Internet], 86/278/EEC Jun 12, 1986, Available from: https://eur-lex.europa.eu/legal-content/EN/TXT/PDF/?uri=CELEX:31986L0278&from=EN (accessed: 2024-04-19) Search PubMed.
- N. Tadkaew, F. I. Hai, J. A. McDonald, S. J. Khan and L. D. Nghiem, Removal of trace organics by MBR treatment: The role of molecular properties, Water Res., 2011, 45(8), 2439–2451 CrossRef CAS
.
- A. Vona, F. Di Martino, J. Garcia-Ivars, Y. Picó, J. A. Mendoza-Roca and M. I. Iborra-Clar, Comparison of different removal techniques for selected pharmaceuticals, J. Water Process Eng., 2015, 5, 48–57 CrossRef
.
- H. Fan, J. Li, L. Zhang and L. Feng, Contribution of sludge adsorption and biodegradation to the removal of five pharmaceuticals in a submerged membrane bioreactor, Biochem. Eng. J., 2014, 88, 101–107 CrossRef CAS
.
- T. Alvarino, S. Suarez, J. Lema and F. Omil, Understanding the sorption and biotransformation of organic micropollutants in innovative biological wastewater treatment technologies, Sci. Total Environ., 2018, 615, 297–306 CrossRef CAS
.
- B. Tiwari, B. Sellamuthu, Y. Ouarda, P. Drogui, R. D. Tyagi and G. Buelna, Review on fate and mechanism of removal of pharmaceutical pollutants from wastewater using biological approach, Bioresour. Technol., 2017, 224, 1–12 CrossRef CAS PubMed
.
- S. K. Maeng, B. G. Choi, K. T. Lee and K. G. Song, Influences of solid retention time, nitrification and microbial activity on the attenuation of pharmaceuticals and estrogens in membrane bioreactors, Water Res., 2013, 47(9), 3151–3162 CrossRef CAS PubMed
.
- R. Trejo-Castillo, E. G. El Kassis, F. Cuervo-López and A. C. Texier, Cometabolic biotransformation of benzotriazole in nitrifying batch cultures, Chemosphere, 2021, 270, 129461 CrossRef CAS
.
- S. Weiss, J. Jakobs and T. Reemtsma, Discharge of Three Benzotriazole Corrosion Inhibitors with Municipal Wastewater and Improvements by Membrane Bioreactor Treatment and Ozonation†, Environ. Sci. Technol., 2006, 40(23), 7193–7199 CrossRef CAS PubMed
.
- B. Herzog, H. Lemmer, B. Helmreich, H. Horn and E. Müller, Monitoring benzotriazoles: a 1 year study on concentrations and removal efficiencies in three different wastewater treatment plants, Water Sci. Technol., 2014, 69(4), 710–717 CrossRef CAS PubMed
.
- N. Dorival-García, A. Zafra-Gómez, A. Navalón, J. González-López, E. Hontoria and J. L. Vílchez, Removal and degradation characteristics of quinolone antibiotics in laboratory-scale activated sludge reactors under aerobic, nitrifying and anoxic conditions, J. Environ. Manage., 2013, 120, 75–83 CrossRef PubMed
.
- M. Vanoppen, A. F. A. M. Bakelants, D. Gaublomme, K. V. K. M. Schoutteten, J. V. Bussche and L. Vanhaecke,
et al. Properties Governing the Transport of Trace Organic Contaminants through Ion-Exchange Membranes, Environ. Sci. Technol., 2015, 49(1), 489–497 CrossRef CAS PubMed
.
- L. Han, S. Galier and H. Roux-de Balmann, Transfer of neutral organic solutes during desalination by electrodialysis: Influence of the salt composition, J. Membr. Sci., 2016, 511, 207–218 CrossRef CAS
.
- S. Ozkul, J. J. Van Daal, N. J. M. Kuipers, R. J. M. Bisselink, H. Bruning and J. E. Dykstra,
et al. Transport mechanisms in electrodialysis: The effect on selective ion transport in multi-ionic solutions, J. Membr. Sci., 2023, 665, 121114 CrossRef CAS
.
- S. Malamis, E. Katsou, K. Takopoulos, P. Demetriou and M. Loizidou, Assessment of metal removal, biomass activity and RO concentrate treatment in an MBR–RO system, J. Hazard. Mater., 2012, 209–210, 1–8 CrossRef CAS PubMed
.
- D. Q. Cao, X. Wang, Q. H. Wang, X. M. Fang, J. Y. Jin and X. D. Hao,
et al. Removal of heavy metal ions by ultrafiltration with recovery of extracellular polymer substances from excess sludge, J. Membr. Sci., 2020, 606, 118103 CrossRef CAS
.
- R. Gao, S. Mosquera-Romero, E. Ntagia, X. Wang, K. Rabaey and L. Bonin, Review—Electrochemical Separation of Organic and Inorganic Contaminants in Wastewater, J. Electrochem. Soc., 2022, 169(3), 033505 CrossRef CAS
.
- S. Papirio, G. Zou, A. Ylinen, F. Di Capua, F. Pirozzi and J. A. Puhakka, Effect of arsenic on nitrification of simulated mining water, Bioresour. Technol., 2014, 164, 149–154 CrossRef CAS PubMed
.
- J. M. A. Juve, F. M. S. Christensen, Y. Wang and Z. Wei, Electrodialysis for metal removal and recovery: A review, Chem. Eng. J., 2022, 435, 134857 CrossRef
.
- K. J. Min, J. H. Kim and K. Y. Park, Characteristics of heavy metal separation and determination of limiting current density in a pilot-scale electrodialysis process for plating wastewater treatment, Sci. Total Environ., 2021, 757, 143762 CrossRef CAS PubMed
.
- Y. Liu, X. Ke, H. Zhu, R. Chen, X. Chen and X. Zheng,
et al. Treatment of raffinate generated via copper ore hydrometallurgical processing using a bipolar membrane electrodialysis system, Chem. Eng. J., 2020, 382, 122956 CrossRef CAS
.
-
Regulation (EU) 2020/741 on minimum requirements for water reuse, 2020, Available from: https://eur-lex.europa.eu/legal-content/EN/TXT/PDF/?uri=CELEX:32020R0741 (accessed: 2024-04-19) Search PubMed.
- C. Riemenschneider, M. Al-Raggad, M. Moeder, B. Seiwert, E. Salameh and T. Reemtsma, Pharmaceuticals, Their Metabolites, and Other Polar Pollutants in Field-Grown Vegetables Irrigated with Treated Municipal Wastewater, J. Agric. Food Chem., 2016, 64(29), 5784–5792 CrossRef CAS
.
- A. Kovačič, E. Andreasidou, A. Brus, A. Vehar, D. Potočnik and M. J. Hudobivnik,
et al. Contaminant uptake in wastewater irrigated tomatoes, J. Hazard. Mater., 2023, 448, 130964 CrossRef
.
- C. Riemenschneider, B. Seiwert, M. Moeder, D. Schwarz and T. Reemtsma, Extensive Transformation of the Pharmaceutical Carbamazepine Following Uptake into Intact Tomato Plants, Environ. Sci. Technol., 2017, 51(11), 6100–6109 CrossRef CAS
.
- R. Kroes, J. Kleiner and A. Renwick, The Threshold of Toxicological Concern Concept in Risk Assessment, Toxicol. Sci., 2005, 86, 226–230 CrossRef CAS PubMed
.
|
This journal is © The Royal Society of Chemistry 2024 |
Click here to see how this site uses Cookies. View our privacy policy here.