Legionella pneumophila occurrence in reduced-occupancy buildings in 11 cities during the COVID-19 pandemic†
Received
20th April 2023
, Accepted 18th July 2023
First published on 18th August 2023
Abstract
In spring 2020, numerous buildings were closed or operated at reduced occupancies to slow the spread of COVID-19. An unintended consequence of these social distancing measures was a reduction in water demand in many buildings. Concerns arose that contaminants associated with water stagnation, such as Legionella pneumophila, could become prevalent. To investigate the potential public health risk associated with L. pneumophila, samples from 26 reduced-occupancy buildings in 11 cities in the United States, Canada, and Switzerland were analyzed for L. pneumophila using liquid culture (Legiolert, n = 258) and DNA-based methods (qPCR/ddPCR, n = 138). L. pneumophila culture-positivity was largely associated with five buildings, each of which had specific design or operational deficiencies commonly associated with L. pneumophila occurrence. Samples from buildings with free chlorine residual disinfection had higher culture-positivity (37%) than samples from buildings with chloramine (1%). Additionally, 78% of culture-positive samples occurred when the disinfectant residual was ≤0.1 mg L−1 Cl2 and only three free chlorine samples were culture-positive when the disinfectant residual was >0.2 mg L−1 as Cl2. Although overall sample positivity using culture- and DNA-based methods was equivalent (34% vs. 35%), there was disagreement between the methods in 13% of samples (n = 18 of 138). Few buildings reported any water management activities, and L. pneumophila concentrations in flushed samples were occasionally greater than in first-draw samples. This study provides insight into how building plumbing characteristics and water management practices contribute to L. pneumophila occurrence during low water use periods and can inform targeted prevention and mitigation efforts.
Water impact
Low building water usage can lead to water quality deterioration, but it was unclear how the widespread, extended occupancy reductions during the COVID-19 pandemic would influence Legionella pneumophila occurrence. In this study, L. pneumophila was measured in 26 buildings across three countries, concluding that building water system design issues and free chlorine systems were linked to higher L. pneumophila positivity.
|
1. Introduction
Closures of non-residential buildings to limit the spread of COVID-19 resulted in water demand reductions in many public, industrial, educational, and commercial buildings.1–5 While short periods of reduced water use (e.g., nights, weekends, school breaks) are common in certain buildings,6 many buildings were closed or at reduced occupancy for several months during the COVID-19 pandemic.7–10 Reduced water use is associated with decreased disinfectant residual levels, equilibration of hot and cold water temperatures with building temperature, and potential for increased concentrations of contaminants such as disinfection byproducts, metals, and opportunistic pathogens.11–21 The bacterium Legionella pneumophila, the primary causative agent of Legionnaires' disease and Pontiac fever, was of particular concern because its occurrence has been associated with low water use,22–26 and disease incidence is rising around the world.27–30 While there are other species of Legionella that cause human infections, L. pneumophila is responsible for the majority of legionellosis illnesses with an identified causative agent.31 Though previous studies have linked low water use to L. pneumophila occurrence, few studies have investigated its occurrence during extended periods of widespread reduced water use, such as during the onset of the COVID-19 pandemic.22,26,32–34
The quantification of L. pneumophila in potable water systems has traditionally been performed using plate culture methods. However, alternative methods such as the IDEXX Legiolert® kit, a liquid culture method that yields results in most probable number (MPN) per volume, has become more widely used due to its ease of use and reported specificity for L. pneumophila.35–41 DNA-based methods (e.g., quantitative polymerase chain reaction [qPCR] and droplet digital polymerase chain reaction [ddPCR]) have also become more common due to the fast turnaround time, the possibility to detect cells in the viable but non-culturable state, and the potential for increased specificity, as compared to culture-based methods.42–44 However, comparisons of results obtained with different methods are generally lacking in the current scientific literature.30,43L. pneumophila monitoring is typically not conducted outside healthcare settings unless the location is associated with disease incidence. This lack of broad surveillance and method comparability has limited our understanding of typical L. pneumophila concentrations in commercial and public building plumbing, the impacts of water demand patterns on L. pneumophila occurrence, and the effectiveness of various interventions for L. pneumophila mitigation.34
Investigations into the impact of COVID-19 pandemic-related building closures have reported that building water quality overall was negatively impacted.45–53 However, studies have reported mixed results with respect to L. pneumophila, including no or low detection of L. pneumophila,45,50,52 no change in the occurrence of L. pneumophila as water use returned to normal levels,46 a small increase (2×) in Legionella spp. relative abundance after two months of reduced water use,48 and widespread detection of L. pneumophila that increased during closure.47 Each of these studies focused on individual cities, buildings, or regions, with none conducting sampling across distribution systems in different regions. Regional differences such as climate, source water, and system operation (e.g., water age, pH, residual disinfectant type) may also influence L. pneumophila occurrence in drinking water distribution systems.36,54 For example, a residual disinfectant in drinking water distribution systems is required in most public water systems in the United States and Canada, but not in other countries, such as Switzerland.55
The objective of this study was to characterize the occurrence of L. pneumophila in drinking water collected from large, non-residential buildings at reduced occupancy due to the COVID-19 pandemic across 11 cities and three countries (United States, Canada, and Switzerland) using both culture-based (Legiolert) and DNA-based (qPCR or ddPCR) methods. Physicochemical water quality parameters, building characteristics, and details of water management practices were collected to identify factors that contributed to L. pneumophila occurrence. Sampling was initiated as a rapid response to assess a potential public health concern, conducted across multiple academic institutions. Site-specific investigations were conducted at several of the sampling locations, the results of which are reported elsewhere.51–53,56,57 This study presents a cross-sectional analysis of potential L. pneumophila exposure risks in buildings with diverse uses, plumbing configurations, operation, and climate regions.
2. Methods
2.1 Sampling locations
Samples were collected (n = 258) from 26 buildings that were either closed or operating at reduced capacity in 11 cities (“sites”) in the United States, Canada, and Switzerland between April and December 2020 (ESI† Fig. S1). All buildings were connected to underground municipal drinking water distribution systems and received water with free chlorine (free chlorine buildings) or monochloramine (chloramine buildings) as the secondary disinfectant, except the building in Switzerland (CH-1), which received water without a secondary disinfectant (“no residual” building). All buildings from the same site were supplied by the same distribution system. Source waters for municipal systems were either surface water (five sites), groundwater (two sites), or mixed groundwater and surface water (four sites). Buildings were large, multi-story, recreational, educational, office, commercial, or mixed-use (e.g., research labs and offices) facilities (Table 1). Samples were collected from showers (n = 101), manual faucets (n = 139), electronic (automatic) faucets (n = 13), and bottle-filling stations and drinking fountains (n = 5). Samples included hot water (n = 114), cold water (n = 43), or “mixed” water (n = 101; hot and cold water mixed prior to the fixture). Samples included 203 first-draw and 55 flushed samples. The results for these samples are presented together except where noted.
Table 1 Summary of sampling locations and building characteristics
Disinfectant type |
Site |
Bldg. |
Source water |
Building characteristics |
Shutdown conditions |
Sampling totals |
Use type |
Number of floors |
Approx. number of fixturesf |
Approx. year built (renovation date) |
Primary plumbing material |
Approx. occupancy during shutdown |
Days reduced occupancy at sampling |
Number of fixtures sampled |
Approx. percent of fixtures sampled |
Number of samples |
Sites: IN: Indiana, USA; OH: Ohio, USA; AZ: Arizona, USA; PA: Pennsylvania, USA; WV: West Virginia, USA; QC: Québec, Canada; MI: Michigan, USA; VA: Virginia, USA; MA: Massachusetts, USA; CA: California, USA; CH: Switzerland. NR: not reported. Use types: Mixed1 – office, classroom, and commercial; Mixed2 – office and laboratory. Source water types – G: groundwater, S: surface water. Primary plumbing materials – C: copper, PVC: polyvinyl chloride, GS: galvanized steel, SS: stainless steel. Water softening at building water inlet, varied hot water setpoint. Laboratory-scale shower rig, no water use during closure. Aerator removed prior to sampling. Suboptimal hot water setpoint of 45 °C with two days per week at 60 °C. Green building. Approximate number of fixtures includes only the following potable fixtures: faucets in bathrooms and kitchens, drinking water fountains and bottle filling stations, and showers. |
Free chlorine |
IN |
IN-1 |
G |
Multifamily housing |
4 |
23 |
1930 |
GS |
0% |
138–174 |
3 |
13% |
4 |
IN-2 |
Commercial |
10 |
215 |
1962 |
C |
0% |
120 |
4 |
2% |
4 |
IN-3 |
Commercial |
4 |
114 |
2002 |
C |
0% |
122 |
2 |
2% |
2 |
IN-4 |
Mixed1 |
5 |
193 |
1951 |
C |
NR |
122 |
2 |
1% |
2 |
OH |
OH-1 |
G |
Education |
2 |
255 |
2012 |
C |
0% |
148–191 |
4 |
2% |
4 |
AZa |
AZ-1e |
S & G |
Mixed2 |
7 |
48 |
2018 |
C |
30–50% |
162 |
7 |
15% |
7 |
PAb |
PA-1 |
S & G |
Mixed2 |
15 |
9 |
1971 (2019) |
C & PVC |
5% |
125 |
9 |
100% |
18 |
WV |
WV-1 |
S |
Mixed1 |
5 |
75 |
2015 |
PVC |
5% |
147 |
3 |
4% |
5 |
WV-2 |
Dorm |
9 |
660 |
1966 |
NR |
2% |
147 |
6 |
1% |
9 |
WV-3 |
Dorm |
3 |
315 |
1918 |
NR |
0% |
147 |
4 |
1% |
7 |
WV-4 |
Dorm |
10 |
550 |
1966 |
C |
0% |
147 |
6 |
1% |
9 |
QC |
QC-1 |
S |
Mixed2 |
7 |
160 |
1959 (1989) |
C |
2% |
62 |
21 |
13% |
21 |
QC-2 |
Mixed2 |
8 |
128 |
2005 |
C |
2% |
53 |
12 |
9% |
12 |
QC-3 |
Sport complex |
5 |
290 |
1974 (2007) |
C |
0% |
269 |
23 |
8% |
23 |
Monochloramine |
MI |
MI-1 |
S & G |
Recreation |
3 |
95 |
1956–1978 |
C & GS |
25% |
160 |
6 |
6% |
6 |
MI-2 |
Recreation |
2 |
37 |
1976 (2018) |
C |
25% |
163 |
7 |
19% |
7 |
MI-3 |
Recreation |
6 |
74 |
1928 (2016) |
C |
25% |
165 |
6 |
8% |
6 |
VA |
VA-1e |
S |
Mixed2 |
3 |
71 |
2005 |
C |
15% |
132 |
5 |
7% |
10 |
VA-2 |
Mixed2 |
2 |
25 |
2007 |
C |
5% |
134 |
4 |
16% |
8 |
MAc |
MA-1 |
S |
Mixed2 |
5 |
31 |
1963 |
NR |
5% |
74 |
2 |
6% |
4 |
MA-2 |
Mixed2 |
4 |
22 |
1984 |
NR |
5% |
74 |
2 |
9% |
4 |
MA-3 |
Mixed2 |
6 |
47 |
2017 |
NR |
5% |
74 |
2 |
4% |
4 |
CA |
CA-1 |
S |
Dorm |
4 |
90 |
1990 |
C |
0–4% |
106 |
8 |
9% |
8 |
CA-2 |
Dorm |
3 |
45 |
1940 |
C |
0–2% |
106 |
6 |
13% |
6 |
CA-3 |
Dorm |
3 |
57 |
1950 |
C |
0–2% |
106 |
6 |
11% |
6 |
None |
CHd |
CH-1 |
S & G |
Mixed2 |
8 |
>200 |
1970s |
SS |
<5% |
46 |
41 |
≤20% |
62 |
Relevant building information was collected from owners and maintenance staff, including estimated building occupancy levels, building plumbing characteristics, and details of water management plans and practices prior to, during, and after building water shutdowns (Tables 1 and S1†). Building-level monthly water usage data were obtained for nine buildings (Fig. S2†). Building occupancy levels were used as a proxy for water consumption because metered flow data were not available for every building. At Site PA, samples were collected from laboratory-scale shower rigs supplied by building water. The building plumbing at Site CH was known to contain L. pneumophila prior to 2020. Permission was obtained prior to sample collection and results of testing were communicated to building owners. While the investigations at each site followed the same overall study design, there were variations in fixture flushing and analysis methods due to the collaborative nature of the sampling campaign.
2.2 Sample collection
First-draw samples (n = 203) were collected from every fixture sampled. Afterwards, flushed samples (n = 55) were collected at a subset of locations, with flush times ranging from one to 30 minutes. Samples were collected without removing or sanitizing aerators/showerheads (except at Site MA), representing what users would experience upon opening fixtures. For both first-draw and flushed samples, 1 to 1.5 L of water was collected in a sterile container. Samples were immediately split onsite for physicochemical (100 mL), culture-based (100 mL; IDEXX Laboratories Inc., catalog number WV120SBST-20), and DNA-based (0.8 L to 1.1 L) analyses and processed the same day. Residual disinfectant was quenched using sodium thiosulfate in subsamples used for culture-based and DNA-based analyses. Sample totals by analysis method and site are provided in Table S2.† Sampling controls are described in Table S3.†
2.3 Physicochemical analyses
Chlorine species were measured using the N,N-diethyl-p-phenylenediamine (DPD) colorimetric method and portable spectrophotometers (Hach, Loveland, CO, USA) with limits of detection (LOD) ranging from 0.02 to 0.05 mg L−1 as Cl2. Chlorine residuals are presented as total chlorine for all sites except for Site WV, where only free chlorine was measured (Table S4†). Site-specific details of additional physicochemical methods (including pH and temperature) are included in Table S4.† Physicochemical parameters were not recorded for samples collected at Sites CH and OH, and most samples from Site IN.
2.4 Culture-based methods
Culturable L. pneumophila was quantified using Legiolert according to manufacturer instructions for 100 mL potable water samples (IDEXX Laboratories, Inc., Westbrook, ME, USA, catalog number WLGT-20). The LOD was 10 MPN L−1, and the upper limit of quantification (ULOQ) was 22
726 MPN L−1. Positive and negative controls were included per manufacturer guidelines (Table S3†). Additional confirmation of positive wells was not performed.
2.5 DNA concentration and extraction
DNA was filter-concentrated onto membrane filters with pore sizes of either 0.2 or 0.4 μm on the day of sampling (Table S5†). Filters were frozen at either −80 °C or −20 °C until extraction. Samples were extracted using the methods described in Table S5.† Filtration and extraction controls are described in Table S3.†
2.6 qPCR and ddPCR
Of the 258 samples analyzed for culturable L. pneumophila, L. pneumophila was quantified using PCR-based methods in 138 samples (n = 120 by qPCR and n = 18 by ddPCR) using previously optimized assays that were validated by the individual laboratories (referred to as Laboratories A to E; Tables S6 and S7†). DNA extracts from Sites CA, WV, IN, and OH were shipped overnight on dry ice to Laboratory A for qPCR analysis (Table S7†). Laboratories A, B, and E analyzed samples using qPCR with an assay targeting a single-copy macrophage infectivity potentiator (mip) gene.58 Laboratory D, which analyzed samples from Site QC, used the proprietary iQ-Check® Quanti L. pneumophila real-time PCR quantification kit (Bio-Rad, Hercules, CA, USA, catalog number 3578103). Laboratory C analyzed samples from Site PA using ddPCR and a different mip gene assay, which was modified for use without a probe (Table S6†).59 Additional qPCR and ddPCR details are included in Tables S6 and S7.† Samples from Sites CH and MA were not analyzed for L. pneumophila gene targets.
All laboratories followed the Minimum Information for Publication of Quantitative Real-Time PCR Experiments (MIQE) and Environmental Microbiology Minimum Information (EMMI) guidelines.60,61 For qPCR, all samples, standards, and controls were analyzed using triplicate reactions on each plate, except for Laboratory D, which used duplicate reactions (Table S7†). Serial dilutions of synthetic DNA consisting of the 79 base pair (bp) amplicon with 30 bp neutral adaptors on each end (gBlock, Integrated DNA Technologies [IDT], Coralville, IA, USA; Table S6†),58 ranging from 108 to 100 gene copies per reaction (gc per reaction) served as the standards for qPCR in all laboratories except Laboratory D, which used the proprietary standards included in the Bio-Rad kit. Efficiencies between 80% and 115% and R2 values of at least 0.98 were required for all qPCR plates containing positive samples (Table S8†). Any plate that did not meet these criteria was re-run. For ddPCR, serial dilutions of synthetic DNA (gBlock, IDT)59 were included on each plate, as were no-template controls (Table S7†). No-template controls were either negative or amplified at Cq values less than the LOD for each group.
The qPCR/ddPCR LOD and lower limit of quantification (LLOQ) were determined in each laboratory independently using serial dilutions of standards with at least 21 replicates across at least three plates, at concentrations ranging from 102 to 100 gc per reaction (Table S9†), except for Laboratory D which followed kit instructions. The qPCR/ddPCR LOD was defined as the lowest concentration at which at least 95% of the standard replicates were detected,60 resulting in LODs ranging from 21 to 1 × 105 gene copies per liter (gc L−1; 1 to 100 gc per reaction) across laboratories. The LLOQ was defined as the lowest concentration where the coefficient of variation was less than 25%,62 resulting in LLOQs ranging from 3.5 × 102 to 2 × 105 gc L−1 (6.1 to 100 gc per reaction) across laboratories. Details of the conversion of LOD and LLOQ to gc L−1 are provided in eqn (S1).† Details of inhibition testing at each laboratory are provided in Text S1.†
Cross-laboratory validation of quantitative standards was performed using a single gBlock that was sent to all laboratories using the Nazarian et al. assay (Fig. S3†).58
2.7 Data analysis
Data analyses were performed using R (version 4.1.1) and RStudio (version 1.4.1717) using a custom R pipeline that included the packages tidyverse, lubridate, and readxl.63,64 Because data were left-censored, imputation was performed, replacing results (physicochemical, qPCR/ddPCR, and Legiolert) less than the LOD with one half the LOD for plotting, calculating summary statistics, and non-parametric hypothesis testing. Legiolert results that were above the ULOQ were set at 30
000 MPN L−1 for non-parametric analysis and visualization. For qPCR/ddPCR data, values between the LOD and LLOQ were replaced with the average of the LOD and LLOQ to assign identical rank for non-parametric analyses. Samples were considered positive by qPCR/ddPCR if gene copy concentrations were above the LOD and quantifiable if concentrations were above the LLOQ. As data were non-normal (Shapiro–Wilk's, R function shapiro.test, p < 0.01), hypothesis testing was conducted using the non-parametric two-sample Wilcoxon rank sum test (“Mann–Whitney”, R function wilcox.test) with a significance threshold of 0.05. The R package stats was used to calculate medians. Rank correlations were calculated using Kendall's tau-b using cor.test, which is well-suited for nonparametric, left-censored data.65 Correlation analysis and principal component analysis (PCA) were performed using the R packages vegan, Hmisc, GGally, forcats, corrplot, devtools, and ggbiplot. The binomial generalized linear mixed-effects model used to investigate the relationship between L. pneumophila culture positivity, physicochemical parameters, and building characteristics was performed using glmer, with the input consisting of 112 samples from ten buildings. The equation used for model generation is provided in eqn (S2).† Figures were generated in R using the additional packages ggPlot2, cowplot, scales, lubridate, repr, sf, rnaturalearth, maps, viridis, ggrepel, and ggnewscale. The full R pipeline and associated data are available on GitHub (https://github.com/kathdowd/Dowdell_and_Healy_2023).
3. Results and discussion
3.1
L. pneumophila occurrence
3.1.1
L. pneumophila detection was building-specific.
The overall L. pneumophila sample positivity was 34% (n = 88 of 258) by Legiolert and 35% (n = 48 of 138) by qPCR/ddPCR. However, L. pneumophila detection was limited to a few specific buildings and was heterogeneous among buildings within the same distribution system. Only seven of the 26 buildings yielded Legiolert-positive samples (IN-1, AZ-1, PA-1, QC-1, QC-3, MI-3, and CH-1; Fig. 1A), and the vast majority of positive samples (98%, n = 86 of 88) occurred in five buildings: four free chlorine buildings (IN-1, AZ-1, PA-1, QC-3) and the no residual building (CH-1). In these five buildings, the percentages of Legiolert-positive samples ranged from 65% to 100% (4–62 samples collected), and median L. pneumophila concentrations in Legiolert-positive samples ranged from 20 to >22
726 MPN L−1. The highest concentrations of culturable L. pneumophila were observed in Buildings AZ-1 (median: >22
726 MPN L−1) and QC-3 (median: 1198 MPN L−1), both of which yielded at least one sample above the ULOQ (>22
726 MPN L−1). Similar results were observed using qPCR/ddPCR: seven buildings had at least one positive sample (AZ-1, PA-1, QC-3, WV-1, WV-2, MI-1, CA-2), three of which (AZ-1, PA-1, QC-3) were also positive by Legiolert. The median positive sample concentrations in qPCR/ddPCR-positive buildings ranged from 158 to 3.1 × 106 gc L−1, with the highest concentrations (>106 gc L−1) occurring in Buildings WV-1, WV-2, and QC-3 (Fig. S4†).
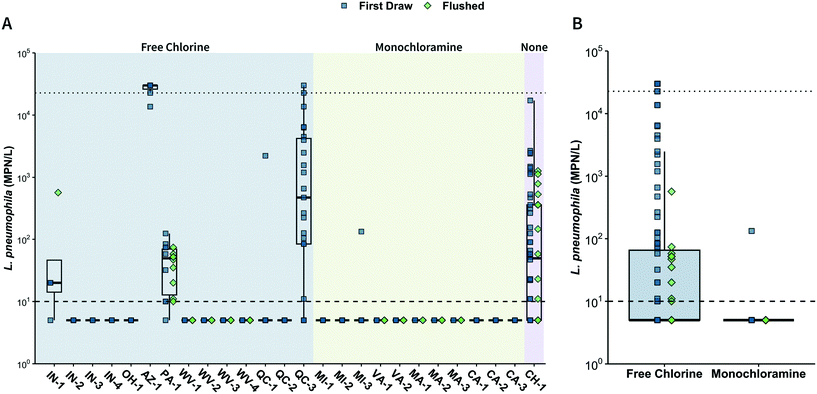 |
| Fig. 1 Culturable Legionella pneumophila concentrations (MPN L−1) by (A) building and (B) secondary disinfectant type. Marker color represents flush condition, where blue circles are first-draw samples and green circles are flushed samples. The dashed horizontal line indicates the LOD (10 MPN L−1) and the dotted horizontal line indicates the ULOQ (22 726 MPN L−1). Results below the LOD are plotted at one-half the LOD (5 MPN L−1). Results above the ULOQ are plotted as 30 000 MPN L−1. | |
There was heterogeneity in L. pneumophila occurrence among different buildings within the same distribution system. For instance, at Sites IN and QC, only one building yielded multiple Legiolert-positive samples while other buildings yielded either one or zero positive samples. Buildings that were positive for L. pneumophila tended to have multiple positive fixtures, which has also been commonly reported in the literature.66–68 For example, though growth trends for L. pneumophila and Legionella spp. cannot be directly compared, a 2019 survey reported that buildings with Legionella spp. detection in the centralized hot water systems had an average distal positivity of 83% and had significantly higher average concentrations of Legionella spp. compared to buildings without Legionella spp. in the centralized hot water system.69 In that same study, the majority of systems with at least one positive sample showed Legionella spp. positivity rates of at least 30% at distal sites. It should be noted that distal site positivity does not necessarily predict incidence of Legionnaires' disease, which also depends on factors such as exposure route and population susceptibility.70,71
3.1.2 Building-specific features may have shaped L. pneumophila positivity.
The buildings with multiple Legiolert-positive samples had identifiable building plumbing operational and/or design deficiencies that did not align with industry recommended practices. Building IN-1 (Legiolert positivity: 75% [n = 3 of 4]; qPCR positivity not measured) is a large, single-family home converted to a multi-family residence with an oversized water heater (100-gal heater with 115-gal insulated holding tank). Building AZ-1 (Legiolert positivity: 100% [n = 7 of 7]; qPCR positivity: 100% [n = 7 of 7]) uses a whole-building water softening system that treats water upon entry into the building. Samples collected from the water entry point revealed that the inlet water was Legiolert-negative and contained a chlorine residual of 0.59 mg L−1 as Cl2,53 whereas the sample from the water storage tank immediately after the softener was positive for L. pneumophila by both Legiolert (10
616 MPN L−1) and qPCR (650 gc L−1) and had a low chlorine residual (0.05 mg L−1 as Cl2). These results indicate that residual disinfectant loss was occurring in the water softener and storage tank. Previous investigations in this building found that the softener was oversized for building water demand, leading to high water residence times in the softener.72,73 Additionally, AZ-1 is a green building (LEED platinum-certified) equipped with low-flow fixtures. Buildings with low-flow water fixtures have previously been linked to high water residence times and, in certain cases, L. pneumophila occurrence.12,72,73 Building PA-1 (Legiolert positivity: 94% [n = 17 of 18]; ddPCR positivity: 67% [n = 12 of 18]) is a laboratory-scale experimental shower rig consisting of three water heaters, each of which supplies three showers. The water heaters were operated at 49 °C and thermostatic mixing valves limited water temperature to 40 °C at the fixtures. After four months of no water use (while still maintaining the 49 °C heater set point), the median L. pneumophila concentration in first-draw samples was 66 MPN L−1. Building QC-3 (Legiolert positivity: 83% [n = 19 of 23]; qPCR positivity: 91% [n = 21 of 23]) is a large sports building with a complex hot water system, which includes four water heaters in series. Issues identified included that a portion of the returned hot water was mixed with the hot water exiting the water heaters, resulting in a decrease of the distributed hot water temperature to below 60 °C; a single thermostatic mixing valve for many showerheads (>20) created large volumes of tepid water; and the third water heater was found to be off but still connected to the system, resulting in cooling of hot water to 30 °C.51 Building CH-1 (Legiolert positivity: 65% [n = 40 of 62]; qPCR positivity: not measured) is a mixed-use building that was historically culture-positive for L. pneumophila and fluctuates the water heater set point between 45 °C (5 days per week) to 60 °C (2 days per week) to save energy.56 Building CH-1 also reported significant heat losses in the primary recirculating line and passive recirculation on individual floor loops, leading to suboptimal temperatures for control of L. pneumophila.
3.1.3
L. pneumophila occurrence varied by secondary disinfectant type.
Five free chlorine buildings yielded multiple Legiolert-positive samples, whereas only a single sample was Legiolert-positive from the chloramine buildings. Thus, the positive buildings strongly influenced the overall sample positivity by secondary disinfectant residual, resulting in free chlorine samples having a substantially higher Legiolert-positivity (37%, n = 47 of 127) than samples collected from chloramine buildings (1.4%, n = 1 of 69, Fig. 1B). Culturable L. pneumophila results were also significantly higher in free chlorine samples compared to chloramine samples (p < 0.01). The median L. pneumophila concentration in the Legiolert-positive samples from the free chlorine buildings was 126 MPN L−1. The concentration measured in the single Legiolert-positive sample from the chloramine buildings was 133 MPN L−1. Similar trends were observed in qPCR/ddPCR-positive samples, where positivity was 52% in free chlorine building samples (n = 45 of 86) and 6% in chloramine building samples (n = 3 of 52, Fig. S5†). Median concentrations in qPCR/ddPCR-positive samples were 5.3 × 103 gc L−1 (n = 45) in the free chlorine buildings and 1.1 × 105 gc L−1 (n = 3) in the chloramine buildings.
Drinking water systems using chloramine for secondary disinfection have previously been reported to have lower L. pneumophila occurrence and concentrations compared to free chlorine,15,68,74–76 even in buildings with reduced occupancy.45 This study supports these findings, as only one sample collected from chloramine systems was culture-positive for L. pneumophila. The low rate of L. pneumophila occurrence in chloramine systems was maintained despite 41% of the samples containing little to no chlorine residual (≤0.1 mg L−1 as Cl2; n = 28 of 69). One reason for lower L. pneumophila occurrence in chloramine systems may be that monochloramine has been reported to better penetrate biofilms,77–80 which could result in enhanced inactivation of L. pneumophila within drinking water system biofilms. Other possible explanations for lower L. pneumophila occurrence in chloramine systems include that chloramine can be more stable in building water systems compared to free chlorine, though the presence of nitrification can reduce chloramine stability.77 Chloramine may also more efficiently inactivate L. pneumophila and its amoebal hosts compared to free chlorine, and one study proposed that chloramine may trigger encystment of amoebae, preventing L. pneumophila infection.81–83 Regardless of the mechanism, these results suggest that drinking water systems that use chloramine for secondary disinfection may better control L. pneumophila. However, most buildings cannot choose which residual disinfectant is supplied in the water, and there are other concerns with monochloramine that should be considered when evaluating secondary disinfectants, such as the potential for nitrification, formation of unregulated disinfection byproducts, and growth of other opportunistic pathogens.84–86
While samples obtained from the no residual building (CH-1) exhibited the highest Legiolert-positivity (65%, n = 40 of 62), this building was previously reported to contain L. pneumophila (i.e., when water demand was typical).87 The few previous studies that have examined L. pneumophila in distribution systems without a disinfectant residual generally report low prevalence of L. pneumophila,59,88–91 though there have been reports of L. pneumophila occurrence in some building hot water systems.92,93 A study conducted prior to the pandemic reported low occurrence of L. pneumophila in Dutch buildings, which were required to flush weekly.89 Additional studies are needed to evaluate the impact of low water use resulting from the COVID-19 pandemic on buildings in distribution systems without residual disinfection.
3.2 Impacts of physicochemical parameters and flushing
3.2.1 Secondary disinfectant concentration influenced presence and concentrations of L. pneumophila in systems with residual disinfection.
Despite reduced occupancies in the buildings and the limited use of water quality mitigation measures (Tables 1 and S1†), 69% (n = 101 of 146) of the first-draw samples analyzed for chlorine (from systems with residual disinfection) contained a detectable chlorine residual (≥0.02 or ≥0.05 mg L−1 as Cl2) but the median concentrations were low (free chlorine buildings: <0.05 mg L−1 as Cl2; chloramine buildings: 0.13 mg L−1 as Cl2; Fig. S6A†). First-draw samples with detectable chlorine residuals were observed in buildings regardless of reported occupancy or preventative maintenance practices. Detectable chlorine in first-draw samples may be a result of water age being lower than expected due to water demand from leaks, maintenance activities, air conditioning systems or cooling towers, and/or operation of treatment systems (e.g., water softeners that automatically regenerate). Though occupancy was low, recent use of fixtures may also have contributed to the presence of residuals, since access to study fixtures was not restricted at all sites prior to sampling. Flushed samples tended to have higher median residuals (free chlorine: 0.05 mg L−1 as Cl2; chloramine: 0.88 mg L−1 as Cl2; Fig. S6A†). The finding that many samples contained measurable disinfectant residuals differs from other investigations of COVID-19-related building closures, which have reported that measurable disinfectant residuals were lacking in most samples.45,49 This difference in findings is likely linked to tap use prior to sampling.
L. pneumophila positivity in disinfected samples generally decreased with increasing disinfectant residual (Fig. 2A, S7 and S8†). In free chlorine buildings, approximately 80% (78%, n = 35 of 45 by Legiolert; 82%, n = 37 of 45 by qPCR/ddPCR) of L. pneumophila positive samples occurred when the chlorine residual was ≤0.1 mg L−1 as Cl2 (Fig. S7C and D†). Only three free chlorine samples were Legiolert-positive with chlorine residuals above 0.2 mg L−1 as Cl2, and the highest chlorine residual in the Legiolert-positive samples was 0.4 mg L−1 as Cl2. The single Legiolert-positive sample from a chloramine building had a chlorine residual below the detection limit (<0.05 mg L−1 as Cl2). However, the other 27 chloramine samples that contained little to no chlorine residual (≤0.1 mg L−1 as Cl2) were Legiolert-negative.
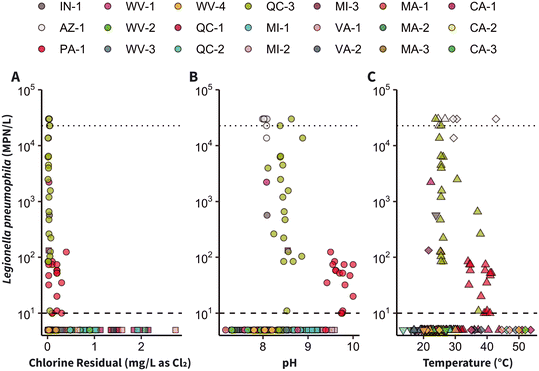 |
| Fig. 2 Physicochemical parameters and culturable L. pneumophila (MPN L−1) for samples by building. A) Chlorine residual (mg L−1 as Cl2), B) pH, and C) temperature. For A and B, marker shape represents secondary disinfectant type, with circles being free chlorine and squares being monochloramine. For C, marker shape shows the water temperature type, with upside down triangles being cold water samples, diamonds being hot water samples, and triangles being hot and cold water mixed. The dashed line shows the LOD. The dotted line shows the ULOQ. Buildings CH-1, IN-2, IN-3, IN-4, and OH-1 are excluded because physicochemical parameters were not recorded. | |
The question of what concentrations of residual disinfectant are required to control L. pneumophila in building water systems is one of pressing importance for public health. In this study, only 7% (n = 3 of 45) of samples collected from free chlorine systems that contained free chlorine concentrations above 0.2 mg L−1 as Cl2 were culture-positive for L. pneumophila. This finding is similar to the results from other studies, which have suggested that free chlorine concentrations ranging from 0.1 to 0.4 mg L−1 as Cl2 may control Legionella spp. or L. pneumophila in distribution systems and building plumbing.30,94–96 However, at least one study has reported no relationship between building water system free chlorine concentration and Legionella spp. occurrence.69 While higher concentrations of chloramine have been found to decrease the occurrence of L. pneumophila, the concentration required for prevention of L. pneumophila varies in the literature.30,75,94,97,98 The relationship between L. pneumophila occurrence and chloramine concentrations could not be characterized in this study due to the low overall positivity in chloramine systems. Additional studies are needed to elucidate the factors that influence the relationships between residual disinfectants and L. pneumophila to identify concentrations that may prevent occurrence.
3.2.2 Sample temperature and pH were not associated with L. pneumophila culture-positivity.
Of the samples where temperature was measured (n = 182), first-draw sample (n = 146) water temperatures were similar to ambient building temperatures regardless of their source (hot, cold, or mixed), with an overall median of 25 °C (Fig. S6C†). Only 19% (n = 4 of 21) of cold water first-draw samples were below 20 °C (median: 23 °C), and only 5% (n = 2 of 39) of hot water first-draw samples were above 50 °C (median: 22 °C, Fig. 2C). In paired first-draw and flushed samples where temperature was recorded (n = 28 pairs), flushing significantly changed the water temperature, reducing the cold water temperatures and increasing hot and mixed water temperatures (p < 0.05, Fig. S9†).
Overall, 46 samples with corresponding temperature measurements were Legiolert-positive, including one cold water sample, five hot water samples, and 40 mixed water samples. Among these samples, temperatures ranged from 22 °C to 43 °C (median: 29 °C), which is within the suitable growth range for L. pneumophila (20 °C to 45 °C).67,99,100 Within these samples there was no apparent trend between temperature and L. pneumophila occurrence or concentration (Fig. 2C). Culture-negative samples occurred at all temperatures measured (n = 136, 14 °C to 54 °C) and sample temperature types (hot, cold, and mixed). The majority of Legiolert-negative samples (82%, n = 111 of 136) were also at temperatures within the L. pneumophila suitable growth range. In samples analyzed using qPCR/ddPCR for which temperature was recorded (n = 130), the temperature in the positive samples (n = 48) ranged from 20 °C to 43 °C and included only hot (n = 10) and mixed (n = 38) water samples. In qPCR/ddPCR-negative samples (n = 82), temperatures ranged from 17 °C to 52 °C (Fig. S10†). Concentrations of L. pneumophila above 1 × 106 gc L−1 occurred in three samples, which were all at approximately room temperature (20 °C to 26 °C). These samples included two hot water samples from Buildings WV-1 and WV-2 and one mixed water sample from Building QC-3.
It is recommended that hot water temperatures be at least 60 °C in water heaters and 55 °C at distal points to control L. pneumophila.30,101 In this study, only four of the 52 hot water samples were at temperatures of at least 50 °C, and none were at or above the recommended temperature of 55 °C. Additionally, the median first-draw hot water temperature was 22 °C. L. pneumophila was not detected in samples with temperatures above 43 °C. The temperature range of L. pneumophila positive samples (20 °C to 43 °C) is in alignment with previous studies, which reported that temperatures between 20 °C and 40 °C were associated with increased L. pneumophila occurrence.96,102,103 However, observed water temperature at distal fixtures may not always be helpful in predicting L. pneumophila concentrations because distal sampling locations acclimate to ambient temperature.69,95,104 Therefore, approaches that incorporate whole-building temperature profiling may be more effective for assessing the influence of hot water temperature on L. pneumophila.99 Intermittent heating, and other energy-saving methods have previously been linked to lower water qualities due to decreased hot water temperatures in building plumbing.105,106 Consistent with this, two buildings with multiple culture-positive samples (CH-1 and AZ-1) practiced hot water temperature fluctuation for energy savings.
Additional physicochemical parameters, including pH (Fig. 2B and S11†), dissolved oxygen (Fig. S12†), and electrical conductivity (Fig. S13†) were measured in a subset of samples (Tables S4 and S10†). Sample pH in chloramine buildings (median: 9.1, n = 69) was higher than the pH of samples from free chlorine buildings (median: 8.2, n = 112; Fig. 2B and S6B†), most likely because higher pH is a strategy to reduce nitrification, which can lead to diminished chloramine residual, nitrate/nitrite formation, and reductions in pH and dissolved oxygen.107 pH values in Legiolert-positive samples ranged from 8.0 to 10.0. Though the median pH was higher in Legiolert-positive samples (8.6, n = 46) than in Legiolert-negative samples (8.3, n = 135), pH tended to co-vary by building. In paired samples where pH was measured (n = 27), pH was significantly different between first-draw and flushed samples (p < 0.05). pH may influence the occurrence of L. pneumophila in distribution systems and building water,108 though one laboratory study reported that L. pneumophila can grow in water with pH values ranging from 5.5 to 9.2.103 A 2021 cooling tower study suggested that high pH (9.6) may be a technique for L. pneumophila control, though the authors noted this may be related to a reduction in protozoan hosts at high pH.109 Ultimately, additional research is needed to investigate the pH sensitivity of L. pneumophila alone and within amoebae.
3.2.3 Fixture flushing did not consistently reduce L. pneumophila occurrence.
The impact of flushing during sampling on L. pneumophila was investigated using paired samples from 48 fixtures (n = 96 samples from 12 buildings). While flushing (for two to 30 minutes, median: five minutes) tended to increase disinfectant residual (Fig. S14†) and shift water temperatures toward cold influent and hot water set points (Fig. S9†), the impact of flushing on Legiolert-positivity varied by fixture: 24 pairs were Legiolert-negative in both first-draw and flushed sample, 18 were positive in both, three fixtures went from negative to positive (PA-1 and CH-1), and three fixtures went from positive to negative with flushing (CH-1; Fig. S15†). With qPCR/ddPCR, nine fixtures were negative in first-draw and flushed samples, three fixtures were positive in both samples (PA-1), four fixtures went from negative to positive (PA-1 and WV-1), and four went from positive to negative (in PA-1 and WV-2; Fig. S16†). When only considering Legiolert-positive pairs (n = 18 pairs from three buildings [PA-1, CH-1, and IN-1]) flushing did not significantly change the concentration of culturable L. pneumophila (median decrease of 27 MPN L−1 with flushing, p = 0.24). However, at individual sampling locations, flushing was also observed to increase L. pneumophila concentrations as much as 549 MPN L−1 (IN-1) and decrease concentrations by as much as 1.6 × 104 MPN L−1 (CH-1). In the three qPCR/ddPCR-positive sample pairs (PA-1), the impact of flushing on L. pneumophila gene copies was similarly mixed (L. pneumophila concentrations increased in one fixture and decreased in two).
Most guidance on L. pneumophila prevention and building recommissioning recommends flushing to introduce fresh water from the distribution system into building plumbing.110–115 Recommendations typically include volume- or water-quality-based flushing, usually of outlets at specific locations (e.g., dead-ends) or the full building water system.110,111,116 Previous studies have reported that flushing that is not performed systematically or that does not consider building plumbing characteristics may be ineffective for improving building water quality.117,118 In practice, a variety of flushing strategies may be employed, which was observed in the reported water management practices of the study buildings. Only two buildings (MI-1 and MI-2) of the 17 buildings for which additional information was provided reported regularly flushing building plumbing while occupancy was restricted (Table S1†). Of these buildings, MI-1 tended to have higher first-draw disinfectant residuals (median: 0.8 mg L−1 as Cl2, n = 6) than the disinfectant residuals in first-draw samples from other chloramine buildings (median: 0.09 mg L−1 as Cl2, n = 48). Interestingly, samples from MI-1 were among the only samples from chloramine buildings where L. pneumophila was detected by qPCR, though the results were below the LLOQ (Fig. S4†). None of the samples from MI-2 (n = 7) contained measurable concentrations of disinfectant. While water management practices varied by building, only one (OH-1, of n = 21 respondents) reported having a formal building water management plan that predated the pandemic and one (CH-1) reported a pre-existing informal management plan. Though the effectiveness of flushing for reducing L. pneumophila concentrations was mixed, this study was not designed to specifically investigate flushing strategies and additional work is needed to determine optimal flushing methods after extended stagnation.
3.2.4 Fixture type was not associated with differences in L. pneumophila occurrence.
Showers and other fixtures that generate droplets and aerosols are of particular concern for opportunistic pathogen respiratory infections because inhalation is the primary route of exposure.119–121 Among showers and manual faucets, the primary fixture types in this study, overall culture-positivity was similar (showers: 38%, n = 38 of 101; manual faucets: 34%, n = 47 of 139). By qPCR/ddPCR, showers were 45% positive (n = 38 of 84) and manual faucets were 11% positive (n = 4 of 36). However, the distribution of fixture types sampled was not uniform across buildings. Positivity in electronic faucets (n = 13 samples across four buildings) was 23% by Legiolert (n = 3, AZ-1) and 46% by qPCR (n = 6, AZ-1 and WV-1). Electronic faucets and other water-saving fixtures have been previously linked to Legionella spp. occurrence.12,14,105,122,123
3.2.5 Source water type was not associated with differences in L. pneumophila occurrence.
Source water type is another factor that might relate to L. pneumophila occurrence.124 This study included 16 samples from buildings receiving treated groundwater (n = 5 buildings, Sites IN and OH), 136 samples from buildings receiving treated surface water (n = 15 buildings, Sites WV, QC, VA, MA, and CA), and 106 samples from buildings receiving mixed groundwater and surface water (n = 6 buildings, Sites AZ, PA, MI, and CH). The sites using groundwater received water with free chlorine as the secondary disinfectant. The surface water and mixed source water sites included systems using free chlorine and chloramine. Median concentrations of culturable L. pneumophila by source water type were below the method LOD (<5 MPN L−1) for groundwater and surface water and 23 MPN L−1 for mixed source water (Fig. S17†). Nineteen percent (n = 3 of 16) of samples from groundwater systems and 15% of surface water samples (n = 20 of 136) were culture-positive for L. pneumophila, while culture positivity was 61% (n = 65 of 106) in mixed source water samples. Among surface water samples from free chlorine systems (n = 86), which included samples from Sites QC and WV, the median concentration was below the LOD (<10 MPN L−1). For mixed water samples from free chlorine systems (n = 25), which included samples from Sites AZ and PA, the median was 58 MPN L−1. Municipal drinking water systems using groundwater have previously been reported to have lower concentrations of Legionella spp.59 and to be less associated with legionellosis outbreaks compared to those using surface water sources.124 In contrast to previous studies, samples from groundwater and surface water systems in this study contained similar concentrations of L. pneumophila, with mixed source water having the highest L. pneumophila positivity. However, as this study was not designed to specifically test the influence of source water, differences between the groups, such as sample size, disinfectant type, and building-specific characteristics, prevented a more thorough evaluation of the role of source water type in L. pneumophila occurrence. Findings from this study and others support the need for additional investigation of the potential influence of source water type on L. pneumophila in drinking water systems.
3.2.6 Combined effects of quantitative physicochemical and building parameters in L. pneumophila occurrence.
To investigate the impact of building characteristics and physicochemical parameters on L. pneumophila culture-positivity in free chlorine buildings, a binomial generalized linear mixed model (glmm) was developed. The model was developed using free chlorine samples from 10 buildings where associated physicochemical measurements and building characteristics were available (n = 112) because disinfectant type has been previously shown to influence L. pneumophila occurrence.68,74,125 The model included the physicochemical parameters pH, temperature, and free chlorine concentration and the building-specific parameter building age as fixed effects; these parameters were chosen based on measured factors previously reported to influence Legionella spp. occurrence.66,89,126–128 To account for clustering by building, building identity was included in the model as a random effect. This analysis showed that none of the parameters were significantly associated with L. pneumophila positivity (p > 0.05, Table S11†). While it was observed that culture positivity was significantly associated with lower disinfectant residuals overall, when looking at the relationship between culture positivity and disinfectant residual within specific buildings, this trend was not observed, likely due to building-specific factors, as discussed in section 3.1.2. Though no parameters met the significance threshold, pH was the model input with the greatest effect on the probability of L. pneumophila-positivity (p = 0.14, odds ratio: 10.9; see eqn (S2)† for equation). A principal component analysis (PCA) was also used to investigate the impact of multiple parameters on L. pneumophila occurrence. L. pneumophila positivity again could not be fully explained by the quantitative variables included, but most Legiolert-positive samples clustered in a region representing lower chlorine concentrations (Fig. S18†).
3.3
L. pneumophila quantification with Legiolert versus qPCR/ddPCR
L. pneumophila was quantified in samples from 17 buildings using qPCR (n = 120) and one building using ddPCR (PA-1; n = 18). Of these samples, 35% (n = 48 of 138) were positive by qPCR/ddPCR and 34% (n = 88 of 258) were positive using Legiolert, with an 87% (n = 120 of 138) agreement between qPCR/ddPCR and Legiolert (positive by both methods: n = 37; negative by both methods: n = 83). The 13% (n = 18 of 138) of samples where there was disagreement between the two methods included 11 samples (8%) that were positive only by qPCR/ddPCR (PA-1, QC-3, WV-1, WV-2, MI-1, CA-2) and seven samples (5%) that were positive only by Legiolert (PA-1 and MI-3). The samples that were only positive by Legiolert exhibited low concentrations (median: 32 MPN L−1), and all but one (n = 6 of 7) were collected from PA-1 and analyzed using ddPCR, indicating that the ddPCR LOD and/or DNA extraction recovery efficiency may have impeded detection. Samples positive only by Legiolert (5% of samples) could have been false positives, which have been observed with rates ranging from 3–4% in previous studies.129–131 However, because confirmation testing of positive wells was not performed, the extent to which these samples were false positives is unclear. The majority of samples that were positive only by qPCR/ddPCR (n = 9 of 11) were quantifiable (above the LLOQ), spanning the full range of L. pneumophila concentrations (102 to 106 gc L−1, Fig. 3A), indicating the presence of dead or non-culturable L. pneumophila.
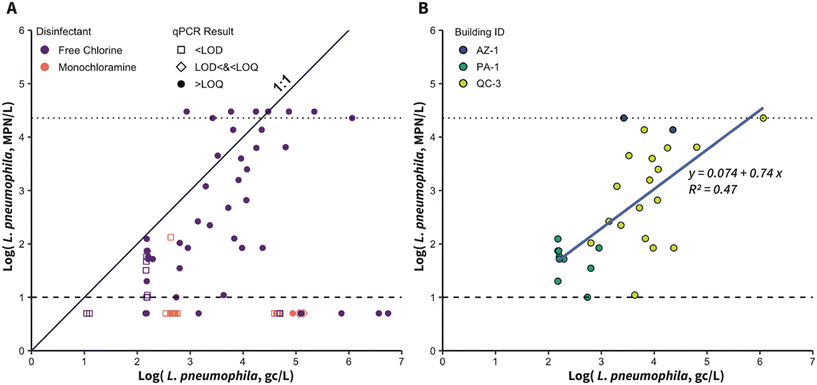 |
| Fig. 3 Culturable L. pneumophila results (log(MPN L−1)) versus qPCR/ddPCR results (log(gc L−1)). A) Results for all samples analyzed by both Legiolert and qPCR/ddPCR (n = 138). The solid black diagonal line has a slope of 1 for reference. Samples that were below the LOD were plotted at one half the LOD (open squares). qPCR/ddPCR samples between the LOD and LLOQ were plotted as the average of the LOD and LLOQ (open diamonds). Samples above the LLOQ were plotted as filled circles. Results above the Legiolert ULOQ were plotted at 30000 MPN L−1. B) Results for the subset of samples that were quantifiable by both methods (n = 31). The blue line is the linear regression. The dashed horizontal line indicates the Legiolert LOD (10 MPN L−1) and the dotted horizontal line indicates the Legiolert ULOQ (22 726 MPN L−1). | |
Samples positive and quantifiable by both methods had a median 0.72
log more L. pneumophila measured by qPCR/ddPCR compared to Legiolert (n = 31, Fig. 3B). However, seven samples that were positive by both methods yielded higher results with Legiolert than qPCR/ddPCR (median difference: 0.32
log). Linear regression analysis of the quantifiable samples resulted in a line with a slope of 0.74 and y-intercept of 0.07 (R2 = 0.47, Fig. 3B). This analysis was used to estimate the relationship between qPCR/ddPCR and Legiolert, but, as shown in Fig. 3B, this relationship may be influenced by location-specific characteristics. Among all samples analyzed by qPCR and Legiolert, there was a negative correlation (Kendall's tau = −0.277, p < 0.001, n = 138), likely due to the way non-quantifiable values were substituted. When only the quantifiable data are considered, the correlation is stronger and positive (Kendall's tau = 0.493, p < 0.001, n = 31).
Previous studies have reported higher Legionella spp. percent positivity by qPCR than culture-based methods, attributing this difference to higher sensitivity of DNA-based methods and the inclusion of cells that are non-viable and/or non-culturable.132–134 However, the relatively high LODs of some of the laboratories (100 gc per reaction, translating to up to 105 gc L−1; Table S9†), as well as losses during concentration and extraction, may have prevented the detection of low concentrations of L. pneumophila DNA in some samples. The higher concentrations observed with qPCR/ddPCR compared to Legiolert agree with previous studies, which have reported that qPCR results were 0.5 to 3
log higher than the corresponding culture-based results.43,94,133 The correlations between quantifiable samples (Kendall's tau = 0.493; linear regression slope = 0.74; Fig. 3B) were similar to those reported in previous studies comparing qPCR to culture-based methods.134,135
Action levels for L. pneumophila are primarily based on plate culture methods, such as the International Organization for Standardization (ISO) method 11
731, which yield results in colony forming units (CFU) per volume.30 However, liquid culture and DNA-based methods like those used in this study are increasingly being used for environmental monitoring due to their ease of use and reduced time to results.42–44L. pneumophila action levels that have been proposed by various publications and organizations vary widely: risk-based limits vary from 14.4 CFU L−1 (disability adjusted life year, 10−6 DALY target) to 1410 CFU L−1 (10−4 annual probability of infection target) in non-healthcare showers and from 12.3 CFU L−1 to 4670 CFU L−1 for total building water fixture exposures;136 1000 CFU L−1 is recommended by the European Working Group for Legionella Infections (EWGLI);137 and health outcome-based levels are as high as 50
000 CFU L−1.30 To place MPN and gene copy results in a health context given existing standards, they must be converted to approximate CFU. Previous studies have found MPN to be equivalent to approximately 1.2 times CFU concentrations.129,138 Using this approximation, the number of samples in the current study that would exceed action thresholds ranged from 33 samples from five buildings using the adjusted Hamilton et al. 14.4 CFU L−1 threshold,136 25 samples from four buildings if using the adjusted EWGLI 1000 CFU L−1 threshold,137 and up to three samples (samples above the Legiolert ULOQ) when using the adjusted 50
000 CFU L−1 threshold.30 It should be noted, however, that none of the buildings included in the study were associated with any legionellosis infections. As suggested by others, a combination of routine monitoring using DNA-based methods to monitor trends and focused investigations with culture-based methods when issues arise may be ideal for balancing cost and public health goals.30 Additional studies that compare the performance of these methods in environmental matrices and realistic building management scenarios and relate the results to health outcomes and risk assessment are needed to bridge the gap between results and action limits.
3.4 Limitations and future work
Although this study represents the most expansive drinking water sampling campaign conducted during the COVID-19 pandemic, the study sample size is limited and, therefore, cannot represent conditions in all non-residential buildings. Additional limitations of this study include that most sites were not monitored for L. pneumophila prior to COVID-19 pandemic building closures, which prevented investigation of how L. pneumophila concentrations changed over time with reduced water use in buildings. Differences in sample analysis methods between laboratories may also influence results, particularly the use of different DNA extraction methods, which have been shown to influence recovery of total DNA.139–141 Detailed water use data were also only available for nine of the buildings. Also, other water quality issues that can result from low water use, such as occurrence of other opportunistic pathogens, metals, and disinfection byproducts, were not assessed in this study.45,46,49,142 Additional work is needed to fully characterize the impact of reduced water use periods on building water quality and inform how buildings can prevent or react to reduced water use.
4. Conclusions
Significant attention was paid by researchers to the potential public health threat posed by exposure to L. pneumophila in building water systems during COVID-19 pandemic-related building closures.24,26 This study found that in 20% of the large, low occupancy buildings sampled, building water contained significant concentrations of culturable L. pneumophila (>102 MPN L−1). However, building water samples from the majority of sites did not contain substantial concentrations of L. pneumophila. This study is unique in that it included data from geographically and structurally diverse buildings in three countries. Results from smaller studies that have investigated the impact of the COVID-19 pandemic on L. pneumophila in building water have ranged from widespread contamination47 to little to no occurrence.45 This study reflects the range of results reported in the literature, as studies focused on only one or a few of the selected sites would yield vastly different results. Similar to previous reports, the choice of secondary disinfectant was the factor most strongly associated with sample positivity, with the overwhelming majority of L. pneumophila positive samples collected from buildings receiving drinking water disinfected with free chlorine compared to monochloramine. Heterogeneity was also observed between buildings within the same free chlorine distribution systems, indicating that building-specific factors, in addition to secondary disinfectant selection, impacted which buildings contained substantial concentrations of L. pneumophila. While the impact of COVID-19 pandemic building closures on legionellosis rates remains unclear, this study highlights that large buildings that receive chlorinated water and have building plumbing characteristics or management practices that are not in-line with best practices may be particularly vulnerable to L. pneumophila occurrence during low flow periods.143–145
Author contributions
WJR, KJP, EG, AJW, LR, CRP, MP, AP, KLN, KAH, and SJH: conceptualization and funding acquisition. KSD, HGH, SJ, MGC, SP, YS, CL, LCK, SV and LH: sample collection and analysis. KSD, HGH, and WJR: data compilation, analysis, and manuscript preparation. All authors: review and editing.
Conflicts of interest
IDEXX Laboratories, Inc. provided 140 free Legiolert tests and additional kits at a discount for this study. IDEXX Laboratories, Inc. did not contribute to the design, analysis, or writing of this manuscript. The interpretation of results and opinions presented in the manuscript are those of the authors and do not necessarily reflect those of IDEXX Laboratories, Inc.
Acknowledgements
The authors would like to thank building owners and managers that responded to questions and provided facility access and acknowledge IDEXX Laboratories, Inc. for providing free and discounted Legiolert kits. The authors appreciate Aliya Ehde, Kirk Olsen, Benjamin Gincley, Umang Chauhan, Irmarie Cotto, and Katherine Vilardi for their water sampling assistance and Amy Pruden, Marc Edwards, and Frederik Hammes for their review of the manuscript. K. S. D. was supported by the National Science Foundation (NSF) Graduate Research Fellowship under Grant No. DGE-1256260, the University of Michigan Blue Sky Grant, and the University of Michigan Rackham Predoctoral Fellowship. H. G. H. was supported by the NSF Graduate Research Fellowship under Grant No. DGE-1752814. S. J. was supported by Arizona State University engineering startup funds. S. P. was supported by a University of Pittsburgh Central Development Research award. W. J. R. was supported by Swiss Federal Institute of Aquatic Science and Technology (EAWAG) discretionary funding. C. R. P., A. J. W., and C. L. were supported by NSF Grants No. CBET-2039498 and CBET-2027049. C. R. P. was supported by the Purdue University Lilian Gilbreth Postdoctoral Fellowship. S. V. and L. H. were supported by NSF Grants No. CBET-2029850 and CBET-1749530. Water Research Foundation Project 4721 additionally supported the development of qPCR/ddPCR methods applied in this study.
References
- M. Poch, M. Garrido-Baserba, L. Corominas, A. Perelló-Moragues, H. Monclús, M. Cermerón-Romero, N. Melitas, S. C. Jiang and D. Rosso, When the fourth water and digital revolution encountered COVID-19, Sci. Total Environ., 2020, 744, 140980, DOI:10.1016/j.scitotenv.2020.140980.
- N. B. Irwin, S. J. McCoy and I. K. McDonough, Water in the time of corona(virus): The effect of stay-at-home orders on water demand in the desert, J. Environ. Econ. Manage., 2021, 109, 102491, DOI:10.1016/j.jeem.2021.102491.
-
American Water Works Association, The financial impact of the COVID-19 crisis on U.S. drinking water utilities, 2020, https://news.awwa.org/AWWA-AMWA-COVID Search PubMed.
- T. Menneer, Z. Qi, T. Taylor, C. Paterson, G. Tu, L. R. Elliott, K. Morrissey and M. Mueller, Changes in domestic energy and water usage during the UK COVID-19 lockdown using high-resolution temporal data, Int. J. Environ. Res. Public Health, 2021, 18, 6818, DOI:10.3390/ijerph18136818.
- D. Li, R. A. Engel, X. Ma, E. Porse, J. D. Kaplan, S. A. Margulis and D. P. Lettenmaier, Stay-at-home orders during the COVID-19 pandemic reduced urban water use, Environ. Sci. Technol. Lett., 2021, 8, 431–436, DOI:10.1021/acs.estlett.0c00979.
- Y. Lambrinidou, S. Triantafyllidou and M. Edwards, Failing our children: Lead in U.S. school drinking water, New Solut., 2010, 20, 25–47, DOI:10.2190/NS.022010eov.
- L. A. Spearing, H. R. Tiedmann, L. Sela, Z. Nagy, J. A. Kaminsky, L. E. Katz, K. A. Kinney, M. J. Kirisits and K. M. Faust, Human–infrastructure interactions during the COVID-19 pandemic: Understanding water and electricity demand profiles at the building level, ACS ES&T Water, 2021, 1, 2327–2338, DOI:10.1021/acsestwater.1c00176.
- R. Richard and T. H. Boyer, Pre- and post-flushing of three schools in Arizona due to COVID-19 shutdown, AWWA Water Sci., 2021, 3(5) DOI:10.1002/aws2.1239.
- E. Z. Berglund, S. Buchberger, M. Cunha, K. M. Faust, M. Giacomoni, E. Goharian, Y. Kleiner, J. Lee, A. Ostfeld, F. Pasha, J. E. Pesantez, J. Saldarriaga, E. Shafiee, L. Spearing, J. E. Van Zyl and Y. C. E. Yang, Effects of the COVID-19 pandemic on water utility operations and vulnerability, J. Water Resour. Plan. Manag., 2022, 148(6), 04022027, DOI:10.1061/(ASCE)WR.1943-5452.0001560.
- E. Montagnino, C. R. Proctor, K. Ra, C. Ley, Y. Noh, K. Vigil, T. G. Aw, S. Dasika and A. J. Whelton, Over the weekend: Water stagnation and contaminant exceedances in a green office building, PLOS Water, 2022, 1, e0000006, DOI:10.1371/journal.pwat.0000006.
-
National Research Council, Drinking water distribution systems: Assessing and reducing risks, The National Academies Press, Washington, DC, 2006, DOI:10.17226/11728.
- W. J. Rhoads, A. Pruden and M. A. Edwards, Survey of green building water systems reveals elevated water age and water quality concerns, Environ. Sci.: Water Res. Technol., 2016, 2, 164–173, 10.1039/C5EW00221D.
- P. Lipphaus, F. Hammes, S. Kötzsch, J. Green, S. Gillespie and A. Nocker, Microbiological tap water profile of a medium-sized building and effect of water stagnation, Environ. Technol., 2014, 35, 620–628, DOI:10.1080/09593330.2013.839748.
- C. J. Ley, C. R. Proctor, G. Singh, K. Ra, Y. Noh, T. Odimayomi, M. Salehi, R. Julien, J. Mitchell, A. P. Nejadhashemi, A. J. Whelton and T. G. Aw, Drinking water microbiology in a water-efficient building: stagnation,
seasonality, and physicochemical effects on opportunistic pathogen and total bacteria proliferation, Environ. Sci.: Water Res. Technol., 2020, 6, 2902–2913, 10.1039/D0EW00334D.
- H. Wang, S. Masters, Y. Hong, J. Stallings, J. O. Falkinham, M. A. Edwards and A. Pruden, Effect of disinfectant, water age, and pipe material on occurrence and persistence of Legionella, mycobacteria, Pseudomonas aeruginosa, and two amoebas, Environ. Sci. Technol., 2012, 46, 11566–11574, DOI:10.1021/es303212a.
- S.-J. Haig, N. Kotlarz, J. J. LiPuma and L. Raskin, A high-throughput approach for identification of nontuberculous mycobacteria in drinking water reveals relationship between water age and Mycobacterium avium, mBio, 2018, 9, e02354-17, DOI:10.1128/mBio.02354-17.
- S.-J. Haig, N. Kotlarz, L. M. Kalikin, T. Chen, S. Guikema, J. J. LiPuma and L. Raskin, Emerging investigator series: bacterial opportunistic pathogen gene markers in municipal drinking water are associated with distribution system and household plumbing characteristics, Environ. Sci.: Water Res. Technol., 2020, 6(11), 3032–3043, 10.1039/D0EW00723D.
- D. L. Tolofari, T. Bartrand, S. V. Masters, M. Duarte Batista, C. N. Haas, M. Olson and P. L. Gurian, Influence of hot water temperature and use patterns on microbial water quality in building plumbing systems, Environ. Eng. Sci., 2022, 39(4), 309–319, DOI:10.1089/ees.2021.0272.
- C. Nguyen, C. Elfland and M. Edwards, Impact of advanced water conservation features and new copper pipe on rapid chloramine decay and microbial regrowth, Water Res., 2012, 46, 611–621, DOI:10.1016/j.watres.2011.11.006.
- C. Elfland, P. Scardina and M. Edwards, Lead-contaminated water from brass plumbing devices in new buildings, J. - Am. Water Works Assoc., 2010, 102, 66–76, DOI:10.1002/j.1551-8833.2010.tb11340.x.
- E. Bédard, C. Laferrière, E. Déziel and M. Prévost, Impact of stagnation and sampling volume on water microbial quality monitoring in large buildings, PLoS One, 2018, 13(6), e0199429, DOI:10.1371/journal.pone.0199429.
- L. E. Garrison, J. M. Kunz, L. A. Cooley, M. R. Moore, C. Lucas, S. Schrag, J. Sarisky and C. G. Whitney, Vital signs: deficiencies in environmental control identified in outbreaks of Legionnaires’ disease—North America, 2000–2014, Am. J. Transplant., 2016, 16(10), 3049–3058, DOI:10.1111/ajt.14024.
- H. Kanamori, D. J. Weber and W. A. Rutala, Healthcare outbreaks associated with a water reservoir and infection prevention strategies, Clin. Infect. Dis., 2016, 62, 1423–1435 CrossRef PubMed.
- C. R. Proctor, W. J. Rhoads, T. Keane, M. Salehi, K. Hamilton, K. J. Pieper, D. M. Cwiertny, M. Prévost and A. J. Whelton, Considerations for large building water quality after extended stagnation, AWWA Water Sci., 2020, e1186, DOI:10.1002/aws2.1186.
- R. Dey and N. J. Ashbolt,
Legionella infection during and after the COVID-19 Pandemic, ACS ES&T Water, 2021, 1(1), 13–14, DOI:10.1021/acsestwater.0c00151.
- W. J. Rhoads and F. Hammes, Growth of Legionella during COVID-19 lockdown stagnation, Environ. Sci.: Water Res. Technol., 2021, 7(1), 10–15, 10.1039/D0EW00819B.
- J. Beauté and on behalf of the European Legionnaires’ Disease Surveillance Network, Legionnaires’ disease in Europe, 2011 to 2015, Eurosurveillance, 2017, 22(27) DOI:10.2807/1560-7917.ES.2017.22.27.30566.
- S. L. Greco, C. Drudge, R. Fernandes, J. Kim and R. Copes, Estimates of healthcare utilisation and deaths from waterborne pathogen exposure in Ontario, Canada, Epidemiol. Infect., 2020, 148, e70, DOI:10.1017/S0950268820000631.
- S. A. Collier, L. Deng, E. A. Adam, K. M. Benedict, E. M. Beshearse, A. J. Blackstock, B. B. Bruce, G. Derado, C. Edens, K. E. Fullerton, J. W. Gargano, A. L. Geissler, A. J. Hall, A. H. Havelaar, V. R. Hill, R. M. Hoekstra, S. C. Reddy, E. Scallan, E. K. Stokes, J. S. Yoder and M. J. Beach, Estimate of burden and direct healthcare cost of infectious waterborne disease in the United States, Emerging Infect. Dis., 2021, 27(1), 140–149, DOI:10.3201/eid2701.190676.
-
National Academies of Sciences, Engineering, and Medicine, Management of Legionella in Water Systems, National Academies Press, Washington, D.C., 2020, https://nap.nationalacademies.org/catalog/25474/management-of-legionella-in-water-systems Search PubMed.
- N. Mérault, C. Rusniok, S. Jarraud, L. Gomez-Valero, C. Cazalet, M. Marin, E. Brachet, P. Aegerter, J. L. Gaillard, J. Etienne, J. L. Herrmann, DELPH-I Study Group, C. Lawrence and C. Buchrieser, Specific real-time PCR for simultaneous detection and identification of Legionella pneumophila Serogroup 1 in water and clinical samples, Appl. Environ. Microbiol., 2011, 77(5), 1708–1717, DOI:10.1128/AEM.02261-10.
- C. A. Ciesielski, M. J. Blaser and W. L. Wang, Role of stagnation and obstruction of water flow in isolation of Legionella pneumophila from hospital plumbing, Appl. Environ. Microbiol., 1984, 48(5), 984–987, DOI:10.1128/aem.48.5.984-987.1984.
- M. A. Nisar, K. E. Ross, M. H. Brown, R. Bentham and H. Whiley, Water stagnation and flow obstruction reduces the quality of potable water and increases the risk of Legionelloses, Front. Environ. Sci., 2020, 8, 13, DOI:10.3389/fenvs.2020.611611.
- C. Proctor, E. Garner, K. A. Hamilton, N. J. Ashbolt, L. J. Caverly, J. O. Falkinham, C. N. Haas, M. Prevost, D. R. Prevots, A. Pruden, L. Raskin, J. Stout and S.-J. Haig, Tenets of a holistic approach to drinking water-associated pathogen research, management, and communication, Water Res., 2022, 211, 117997, DOI:10.1016/j.watres.2021.117997.
- J. Checa, I. Carbonell, N. Manero and I. Martí, Comparative study of Legiolert with ISO 11731-1998 standard method-conclusions from a public health laboratory, J. Microbiol. Methods, 2021, 186, 106242, DOI:10.1016/j.mimet.2021.106242.
- M. LeChevallier, Monitoring distribution systems for Legionella pneumophila using Legiolert, AWWA Water Sci., 2019, 1(1), e1122, DOI:10.1002/aws2.1122.
- D. P. Sartory, K. Spies, B. Lange, S. Schneider and B. Langer, Evaluation of a most probable number method for the enumeration of Legionella pneumophila from potable and related water samples, Lett. Appl. Microbiol., 2017, 64(4), 271–275, DOI:10.1111/lam.12719.
- R. Petrisek and J. Hall, Evaluation of a most probable number method for the enumeration of Legionella pneumophila from North American potable and nonpotable water samples, J. Water Health, 2018, 16(1), 25–33, DOI:10.2166/wh.2017.118.
- M. M. Rech, B. M. Swalla and J. K. Dobranic, Evaluation of Legiolert for quantification of Legionella pneumophila from non-potable water, Curr. Microbiol., 2018, 75(10), 1282–1289, DOI:10.1007/s00284-018-1522-0.
- I. Barrette, Comparison of Legiolert and a conventional culture method for detection of Legionella pneumophila from cooling towers in Québec, J. AOAC Int., 2019, 102(4), 1235–1240, DOI:10.5740/jaoacint.18-0245.
- H. Inoue, M. Baba and S. Tayama, Evaluation of Legiolert for quantification of Legionella pneumophila from bath water samples, Biocontrol Sci., 2020, 25(3), 179–182, DOI:10.4265/bio.25.179.
- H. Wang, E. Bédard, M. Prévost, A. K. Camper, V. R. Hill and A. Pruden, Methodological approaches for monitoring opportunistic pathogens in premise plumbing: A review, Water Res., 2017, 117, 68–86, DOI:10.1016/j.watres.2017.03.046.
- H. Whiley and M. Taylor,
Legionella detection by culture and qPCR: Comparing apples and oranges, Crit. Rev. Microbiol., 2016, 42(1), 65–74, DOI:10.3109/1040841X.2014.885930.
- T. G. Aw and J. B. Rose, Detection of pathogens in water: from phylochips to qPCR to pyrosequencing, Curr. Opin. Biotechnol., 2012, 23(3), 422–430, DOI:10.1016/j.copbio.2011.11.016.
- R. M. Hozalski, T. M. LaPara, X. Zhao, T. Kim, M. B. Waak, T. Burch and M. McCarty, Flushing of stagnant premise water systems after the COVID-19 shutdown can reduce infection risk by Legionella and Mycobacterium spp, Environ. Sci. Technol., 2020, 54(24), 15914–15924, DOI:10.1021/acs.est.0c06357.
- C. Ye, X. Xian, R. Bao, Y. Zhang, M. Feng, W. Lin and X. Yu, Recovery of microbiological quality of long-term stagnant tap water in university buildings during the COVID-19 pandemic, Sci. Total Environ., 2022, 806(Part 2), 150616, DOI:10.1016/j.scitotenv.2021.150616.
- O. De Giglio, G. Diella, M. Lopuzzo, F. Triggiano, C. Calia, C. Pousis, F. Fasano, G. Caggiano, G. Calabrese, V. Rafaschieri, F. Carpagnano, M. Carlucci, L. Gesualdo, M. L. Ricci, M. Scaturro, M. C. Rota, L. Bonadonna, L. Lucentini and M. T. Montagna, Impact of lockdown on the microbiological status of the hospital water network during COVID-19 pandemic, Environ. Res., 2020, 191, 110231, DOI:10.1016/j.envres.2020.110231.
- J. Liang, C. S. Swanson, L. Wang and Q. He, Impact of building closures during the COVID-19 pandemic on Legionella infection risks, Am. J. Infect. Control, 2021, 49(12), 1564–1566, DOI:10.1016/j.ajic.2021.09.008.
- M. Salehi, D. DeSimone, K. Aghilinasrollahabadi and T. Ahamed, A case study on tap water quality in large buildings recommissioned after extended closure due to the COVID-19 pandemic, Environ. Sci.: Water Res. Technol., 2021, 7, 1996–2009, 10.1039/D1EW00428J.
- T. G. Aw, L. Scott, K. Jordan, K. Ra, C. Ley and A. J. Whelton, Prevalence of opportunistic pathogens in a school building plumbing during periods of low water use and a transition to normal use, Int. J. Hyg. Environ. Health, 2022, 241, 113945, DOI:10.1016/j.ijheh.2022.113945.
- M. Grimard-Conea, E. Deshommes, E. Doré and M. Prévost, Impact of recommissioning flushing on Legionella pneumophila in a large building during the COVID-19 pandemic, Front. Water, 2022, 4, 959689, DOI:10.3389/frwa.2022.959689.
- H. Greenwald, L. C. Kennedy, A. Ehde, Y. Duan, C. I. Olivares, R. Kantor and K. L. Nelson, Is flushing necessary during building closures? A study of water quality and bacterial communities during extended reductions in building occupancy, Front. Water, 2022, 4, 958523, DOI:10.3389/frwa.2022.958523.
- S. Joshi, R. Richard, C. Levya, J. C. Harrison, D. Saetta, N. Sharma, L. Crane, N. Mushro, L. Dieter, G. V. Morgan, A. Heida, B. Welco, T. H. Boyer, P. Westerhoff and K. A. Hamilton, Pinpointing drivers of widespread colonization of Legionella pneumophila in a green building: Roles of water softener system, expansion tank, and reduced occupancy, Front. Water, 2023, 4, 966223, DOI:10.3389/frwa.2022.966223.
- R. Sakamoto, Legionnaire’s disease, weather and climate, Bull. W. H. O., 2015, 93(6), 435–436, DOI:10.2471/BLT.14.142299.
- F. Hammes, C. Berger, O. Köster and T. Egli, Assessing biological stability of drinking water without disinfectant residuals in a full-scale water supply system, J. Water Supply: Res. Technol.--AQUA, 2010, 59(1), 31–40, DOI:10.2166/aqua.2010.052.
- W. J. Rhoads, M. Sindelar, C. Margot, N. Graf and F. Hammes, Variable Legionella response to building occupancy patterns and precautionary flushing, Microorganisms, 2022, 10(3), 555, DOI:10.3390/microorganisms10030555.
- S. Vosloo, L. Huo, U. Chauhan, I. Cotto, B. Gincley, K. J. Vilardi, B. Yoon, K. Bian, M. Gabrielli, K. J. Pieper and A. J. Pinto, Gradual recovery of building plumbing-associated microbial communities after extended periods of altered water demand during the COVID-19 pandemic, Environ. Sci. Technol., 2023, 57(8), 3248–3259, DOI:10.1021/acs.est.2c07333.
- E. J. Nazarian, D. J. Bopp, A. Saylors, R. J. Limberger and K. A. Musser, Design and implementation of a protocol for the detection of Legionella in clinical and environmental samples, Diagn. Microbiol. Infect. Dis., 2008, 62(2), 125–132, DOI:10.1016/j.diagmicrobio.2008.05.004.
- B. A. Wullings, G. Bakker and D. van Der Kooij, Concentration and diversity of uncultured Legionella spp. in two unchlorinated drinking water supplies with different concentrations of natural organic matter, Appl. Environ. Microbiol., 2011, 77(2), 634–641, DOI:10.1128/AEM.01215-10.
- S. A. Bustin, V. Benes, J. A. Garson, J. Hellemans, J. Huggett, M. Kubista, R. Mueller, T. Nolan, M. W. Pfaffl, G. L. Shipley, J. Vandesompele and C. T. Wittwer, The MIQE Guidelines: Minimum Information for Publication of Quantitative Real-Time PCR Experiments, Clin. Chem., 2009, 55(4), 611–622, DOI:10.1373/clinchem.2008.112797.
- M. A. Borchardt, A. B. Boehm, M. Salit, S. K. Spencer, K. R. Wigginton and R. T. Noble, The Environmental Microbiology Minimum Information (EMMI) Guidelines: qPCR and dPCR Quality and Reporting for Environmental Microbiology, Environ. Sci. Technol., 2021, 55(15), 10210–10223, DOI:10.1021/acs.est.1c01767.
- A. Forootan, R. Sjöback, J. Björkman, B. Sjögreen, L. Linz and M. Kubista, Methods to determine limit of detection and limit of quantification in quantitative real-time PCR (qPCR), Biomol. Detect. Quantif., 2017, 12, 1–6, DOI:10.1016/j.bdq.2017.04.001.
-
R Core Team, R: A language and environment for statistical computing, R Foundation for Statistical Computing, Vienna, Austria, 2021, https://www.R-project.org/ Search PubMed.
-
RStudio Team, RStudio: Integrated development for R, RStudio, PBC, Boston, MA, 2020, https://www.rstudio.com/ Search PubMed.
- M. D. Wood, N. A. Beresford and D. Copplestone, Limit of detection values in data analysis: Do they matter?, Radioprotection, 2011, 46(6), S85–S90, DOI:10.1051/radiopro/20116728s.
- E. Leoni, G. De Luca, P. P. Legnani, R. Sacchetti, S. Stampi and F. Zanetti,
Legionella waterline colonization: detection of Legionella species in domestic, hotel and hospital hot water systems, J. Appl. Microbiol., 2005, 98(2), 373–379, DOI:10.1111/j.1365-2672.2004.02458.x.
- M. Alary and J. R. Joly, Factors contributing to the contamination of hospital water distribution systems by Legionellae, J. Infect. Dis., 1992, 165(3), 565–569, DOI:10.1093/infdis/165.3.565.
- B. Flannery, L. B. Gelling, D. J. Vugia, J. M. Weintraub, J. J. Salerno, M. J. Conroy, V. A. Stevens, C. E. Rose, M. R. Moore, B. S. Fields and R. E. Besser, Reducing Legionella colonization of water systems with monochloramine, Emerging Infect. Dis., 2006, 12(4), 588–596, DOI:10.3201/eid1204.051101.
- D. Pierre, J. L. Baron, X. Ma, F. P. Sidari, M. M. Wagener and J. E. Stout, Water quality as a predictor of Legionella positivity of building water systems, Pathogens, 2019, 8(4), 295, DOI:10.3390/pathogens8040295.
- J. G. Allen, T. A. Myatt, D. L. MacIntosh, J. F. Ludwig, T. Minegishi, J. H. Stewart, B. F. Connors, M. P. Grant and J. F. McCarthy, Assessing risk of health care–acquired Legionnaires’ disease from environmental sampling: The limits of using a strict percent positivity approach, Am. J. Infect. Control, 2012, 40(10), 917–921, DOI:10.1016/j.ajic.2012.01.013.
- A. Demirjian, C. E. Lucas, L. E. Garrison, N. A. Kozak-Muiznieks, S. States, E. W. Brown, J. M. Wortham, A. Beaudoin, M. L. Casey, C. Marriott, A. M. Ludwig, A. F. Sonel, R. R. Muder and L. A. Hicks, The importance of clinical surveillance in detecting Legionnaires’ disease outbreaks: A large outbreak in a hospital with a Legionella disinfection system—Pennsylvania, 2011–2012, Clin. Infect. Dis., 2015, 60(11), 1596–1602, DOI:10.1093/cid/civ153.
- R. Richard, K. A. Hamilton, P. Westerhoff and T. H. Boyer, Physical, chemical, and microbiological water quality variation between city and building and within multistory building, ACS ES&T Water, 2021, 1(6), 1369–1379, DOI:10.1021/acsestwater.0c00240.
- R. Richard, K. A. Hamilton, P. Westerhoff and T. H. Boyer, Tracking copper, chlorine, and occupancy in a new, multi-story, institutional green building, Environ. Sci.: Water Res. Technol., 2020, 6, 1672–1680, 10.1039/D0EW00105H.
- J. L. Kool, J. C. Carpenter and B. S. Fields, Effect of monochloramine disinfection of municipal drinking water on risk of nosocomial Legionnaires’ disease, Lancet, 1999, 353(9149), 272–277, DOI:10.1016/S0140-6736(98)06394-6.
- D. A. Lytle, S. Pfaller, C. Muhlen, I. Struewing, S. Triantafyllidou, C. White, S. Hayes, D. King and J. Lu, A comprehensive evaluation of monochloramine disinfection on water quality, Legionella and other important microorganisms in a hospital, Water Res., 2021, 189, 116656, DOI:10.1016/j.watres.2020.116656.
- M. R. Moore, M. Pryor, B. Fields, C. Lucas, M. Phelan and R. E. Besser, Introduction of monochloramine into a municipal water system: Impact on colonization of buildings by Legionella spp, Appl. Environ. Microbiol., 2006, 72(1), 378–383, DOI:10.1128/AEM.72.1.378-383.2006.
- M. W. LeChevallier, C. D. Cawthon and R. G. Lee, Inactivation of biofilm bacteria, Appl. Environ. Microbiol., 1988, 54(10), 2492–2499, DOI:10.1128/aem.54.10.2492-2499.1988.
- M. M. Samrakandi, C. Roques and G. Michel, Influence of trophic conditions on exopolysaccharide production: bacterial biofilm susceptibility to chlorine and monochloramine, Can. J. Microbiol., 1997, 43(8), 751–758, DOI:10.1139/m97-108.
- C. Chen, T. Griebe and W. G. Characklis, Biocide action of monochloramine on biofilm systems of Pseudomonas aeruginosa, Biofouling, 1993, 7(1), 1–17, DOI:10.1080/08927019309386240.
- W. H. Lee, D. G. Wahman, P. L. Bishop and J. G. Pressman, Free chlorine and monochloramine application to nitrifying biofilm: Comparison of biofilm penetration, activity, and viability, Environ. Sci. Technol., 2011, 45(4), 1412–1419, DOI:10.1021/es1035305.
- M. Dupuy, S. Mazoua, F. Berne, C. Bodet, N. Garrec, P. Herbelin, F. Ménard-Szczebara, S. Oberti, M. H. Rodier, S. Soreau, F. Wallet and Y. Héchard, Efficiency of water disinfectants against Legionella pneumophila and Acanthamoeba, Water Res., 2011, 45(3), 1087–1094, DOI:10.1016/j.watres.2010.10.025.
- M. Dupuy, F. Berne, P. Herbelin, M. Binet, N. Berthelot, M.-H. Rodier, S. Soreau and Y. Héchard, Sensitivity of free-living amoeba trophozoites and cysts to water disinfectants, Int. J. Hyg. Environ. Health, 2014, 217(2–3), 335–339, DOI:10.1016/j.ijheh.2013.07.007.
- W. J. Johnson, P. K. Jjemba, Z. Bukhari and M. W. LeChevallier, Occurrence of Legionella in nonpotable reclaimed water, J. AWWA, 2018, 110(3), 15–27, DOI:10.5942/jawwa.2018.110.0021.
- C. Nguyen, C. Elfland and M. Edwards, Impact of advanced water conservation features and new copper pipe on rapid chloramine decay and microbial regrowth, Water Res., 2012, 46(3), 611–621, DOI:10.1016/j.watres.2011.11.006.
- B. Casini, A. Buzzigoli, M. L. Cristina, A. M. Spagnolo, P. D. Giudice, S. Brusaferro, A. Poscia, U. Moscato, P. Valentini, A. Baggiani and G. Privitera, Long-term effects of hospital water network disinfection on Legionella and other waterborne bacteria in an Italian university hospital, Infect. Control Hosp. Epidemiol., 2014, 35(3), 293–299, DOI:10.1086/675280.
- S. Pfaller, D. King, J. H. Mistry, M. Alexander, G. Abulikemu, J. G. Pressman, D. G. Wahman and M. J. Donohue, Chloramine concentrations within distribution systems and their effect on heterotrophic bacteria, mycobacterial species, and disinfection byproducts, Water Res., 2021, 205, 117689, DOI:10.1016/j.watres.2021.117689.
- C. Margot, W. J. Rhoads, N. Graf, F. Rölli and F. Hammes, Légionelles et eau potable. Une étude de cas de contrôle par la temperature, Aqua Gas, 2021, 101(9), 78–83 Search PubMed.
- P. W. J. J. van der Wielen and D. van der Kooij, Nontuberculous mycobacteria, fungi, and opportunistic pathogens in unchlorinated drinking water in the Netherlands, Appl. Environ. Microbiol., 2013, 79(3), 825–834, DOI:10.1128/AEM.02748-12.
- W. van der Lugt, S. M. Euser, J. P. Bruin, J. W. den Boer and E. P. F. Yzerman, Wide-scale study of 206 buildings in the Netherlands from 2011 to 2015 to determine the effect of drinking water management plans on the presence of Legionella spp, Water Res., 2019, 161, 581–589, DOI:10.1016/j.watres.2019.06.043.
- M. B. Waak, T. M. LaPara, C. Hallé and R. M. Hozalski, Occurrence of Legionella spp. in water-Main biofilms from two drinking water distribution systems, Environ. Sci. Technol., 2018, 52(14), 7630–7639, DOI:10.1021/acs.est.8b01170.
- B. M. W. Diederen, C. M. A. de Jong, I. Aarts, M. F. Peeters and A. van der Zee, Molecular evidence for the ubiquitous presence of Legionella species in Dutch tap water installations, J. Water Health, 2007, 5(3), 375–383, DOI:10.2166/wh.2007.033.
- D. G. Groothuis, H. R. Veenendaal and H. L. Dijkstra, Influence of temperature on the number of Legionella pneumophila in hot water systems, J. Appl. Bacteriol., 1985, 59(6), 529–536, DOI:10.1111/j.1365-2672.1985.tb03356.x.
- S. M. Euser, J. P. Bruin, W. van der Hoek, W. A. Schop and J. W. den Boer, Wellness centres: an important but overlooked source of Legionnaires’ disease. Eight years of source investigation in the Netherlands, 1 August 2002 to 1 August 2010, Eurosurveillance, 2012, 17(8), 20097, DOI:10.2807/ese.17.08.20097-en.
- M. J. Donohue, Quantification of Legionella pneumophila by qPCR and culture in tap water with different concentrations of residual disinfectants and heterotrophic bacteria, Sci. Total Environ., 2021, 774, 145142, DOI:10.1016/j.scitotenv.2021.145142.
- W. J. Rhoads, T. N. Bradley, A. Mantha, L. Buttling, T. Keane, A. Pruden and M. A. Edwards, Residential water heater cleaning and occurrence of Legionella in Flint, MI, Water Res., 2020, 171, 115439, DOI:10.1016/j.watres.2019.115439.
- M. W. LeChevallier, Occurrence of culturable Legionella pneumophila in drinking water distribution systems, AWWA Water Sci., 2019, 1(3), e1139, DOI:10.1002/aws2.1139.
- I. Marchesi, S. Paduano, G. Frezza, L. Sircana, E. Vecchi, P. Zuccarello, G. Oliveri Conti, M. Ferrante, P. Borella and A. Bargellini, Safety and effectiveness of monochloramine treatment for disinfecting hospital water networks, Int. J. Environ. Res. Public Health, 2020, 17(17), 6116, DOI:10.3390/ijerph17176116.
- L. Huo, L. Pan, R. Chen, B. Shi, H. Wang and S. He, Effects of disinfectants and particles on the occurrence of different microorganisms in drinking water distribution systems, Environ. Sci.: Water Res. Technol., 2021, 7, 983–992, 10.1039/D0EW01119C.
- E. Bédard, S. Fey, D. Charron, C. Lalancette, P. Cantin, P. Dolcé, C. Laferrière, E. Déziel and M. Prévost, Temperature diagnostic to identify high risk areas and optimize Legionella pneumophila surveillance in hot water distribution systems, Water Res., 2015, 71, 244–256, DOI:10.1016/j.watres.2015.01.006.
- W. J. Rhoads, P. Ji, A. Pruden and M. A. Edwards, Water heater temperature set point and water use patterns influence Legionella pneumophila and associated microorganisms at the tap, Microbiome, 2015, 3(1), 67, DOI:10.1186/s40168-015-0134-1.
- J. Darelid, S. Löfgren and B.-E. Malmvall, Control of nosocomial Legionnaires’ disease by keeping the circulating hot water temperature above 55°C: Experience from a 10-year surveillance programme in a district general hospital, J. Hosp. Infect., 2002, 50(3), 213–219, DOI:10.1053/jhin.2002.1185.
- J. Lu, H. Buse, I. Struewing, A. Zhao, D. Lytle and N. Ashbolt, Annual variations and effects of temperature on Legionella spp. and other potential opportunistic pathogens in a bathroom, Environ. Sci. Pollut. Res., 2017, 24(3), 2326–2336, DOI:10.1007/s11356-016-7921-5.
- R. M. Wadowsky, R. Wolford, A. M. McNamara and R. B. Yee, Effect of temperature, pH, and oxygen level on the multiplication of naturally occurring Legionella pneumophila in potable water, Appl. Environ. Microbiol., 1985, 49(5), 1197–1205, DOI:10.1128/aem.49.5.1197-1205.1985.
- D. Hayes-Phillips, R. Bentham, K. Ross and H. Whiley, Factors influencing Legionella contamination of domestic household showers, Pathogens, 2019, 8(1), 27, DOI:10.3390/pathogens8010027.
- E. Bédard, S. Lévesque, P. Martin, L. Pinsonneault, K. Paranjape, C. Lalancette, C.-É. Dolcé, M. Villion, L. Valiquette, S. P. Faucher and M. Prévost, Energy conservation and the promotion of Legionella pneumophila growth: The probable role of heat exchangers in a nosocomial outbreak, Infect. Control Hosp. Epidemiol., 2016, 37(12), 1475–1480, DOI:10.1017/ice.2016.205.
- S. Bernander, K. Jacobson, J. H. Helbig, P. C. Luck and M. Lundholm, A hospital-associated outbreak of Legionnaires’ disease caused by Legionella pneumophila Serogroup 1 is characterized by stable genetic fingerprinting but variable monoclonal antibody patterns, J. Clin. Microbiol., 2003, 41(6), 2503–2508, DOI:10.1128/JCM.41.6.2503-2508.2003.
-
United States Environmental Protection Agency, Nitrification, 2002, https://www.epa.gov/sites/default/files/2015-09/documents/nitrification_1.pdf Search PubMed.
- R. L. Martin, O. Strom, Y. Song, D. Mena-Aguilar, W. J. Rhoads, A. Pruden and M. A. Edwards, Copper pipe, lack of corrosion control, and very low pH may have influenced the trajectory of the Flint Legionnaires’ disease outbreak, ACS ES&T Water, 2022, 2(8), 1440–1450, DOI:10.1021/acsestwater.2c00182.
- I. S. M. Pinel, P. M. Hankinson, D. H. Moed, L. J. Wyseure, J. S. Vrouwenvelder and M. C. M. van Loosdrecht, Efficient cooling tower operation at alkaline pH for the control of Legionella pneumophila and other pathogenic genera, Water Res., 2021, 197, 117047, DOI:10.1016/j.watres.2021.117047.
-
United States Environmental Protection Agency, Maintaining or restoring water quality in buildings with low or no use, 2020, https://www.epa.gov/sites/default/files/2020-05/documents/final_maintaining_building_water_quality_5.6.20-v2.pdf Search PubMed.
-
ASHRAE, ASHRAE Guideline 12-2020: Managing the risk of Legionellosis associated with building water systems, 2020, https://www.techstreet.com/ashrae/standards/guideline-12-2020-managing-the-risk-of-legionellosis-associated-with-building-water-systems?product_id=2111422 Search PubMed.
-
ASHRAE, Standard 188-2018 -- Legionellosis: Risk management for building water systems, 2018, https://www.ashrae.org/technical-resources/bookstore/ansi-ashrae-standard-188-2018-legionellosis-risk-management-for-building-water-systems Search PubMed.
- R. Singh, K. A. Hamilton, M. Rasheduzzaman, Z. Yang, S. Kar, A. Fasnacht, S. V. Masters and P. L. Gurian, Managing water quality in premise plumbing: Subject matter experts’ perspectives and a systematic review of guidance documents, Water, 2020, 12(2), 347, DOI:10.3390/w12020347.
-
United States Centers for Disease Control and Prevention, Toolkit for controlling Legionella in common sources of exposure, United States Centers for Disease Control and Prevention, 2021, https://www.cdc.gov/legionella/wmp/control-toolkit/index.html Search PubMed.
-
United States Centers for Disease Control and Prevention, Developing a water management program to reduce Legionella growth & spread in buildings - a practical guide to implementing industry standards; version 1.1, 2021, https://www.cdc.gov/legionella/downloads/toolkit.pdf Search PubMed.
-
United States Centers for Disease Control and Prevention, Reopening buildings after prolonged shutdown or reduced operation, 2021, https://www.cdc.gov/nceh/ehs/water/legionella/building-water-system.html?CDC_AA_refVal=https%3A%2F%2Fwww.cdc.gov%2Fcoronavirus%2F2019-ncov%2Fphp%2Fbuilding-water-system.html Search PubMed.
- K. S. Casteloes, R. H. Brazeau and A. J. Whelton, Decontaminating chemically contaminated residential premise plumbing systems by flushing, Environ. Sci.: Water Res. Technol., 2015, 1, 787–799, 10.1039/C5EW00118H.
- K. Ra, T. Odimayomi, C. Ley, T. G. Aw, J. B. Rose and A. J. Whelton, Finding building water quality challenges in a 7 year old green school: implications for building design, sampling, and remediation, Environ. Sci.: Water Res. Technol., 2020, 6, 2691–2703, 10.1039/D0EW00520G.
- A. J. Prussin, D. O. Schwake and L. C. Marr, Ten questions concerning the aerosolization and transmission of Legionella in the built environment, Build. Environ., 2017, 123, 684–695, DOI:10.1016/j.buildenv.2017.06.024.
- D. W. Fraser, Legionellosis: evidence of airborne transmission, Ann. N. Y. Acad. Sci., 1980, 353, 61–66, DOI:10.1111/j.1749-6632.1980.tb18906.x.
- B. A. Cunha, A. Burillo and E. Bouza, Legionnaires’ disease, Lancet, 2016, 387(10016), 376–385, DOI:10.1016/S0140-6736(15)60078-2.
- E. R. M. Sydnor, G. Bova, A. Gimburg, S. E. Cosgrove, T. M. Perl and L. L. Maragakis, Electronic-eye faucets: Legionella species contamination in healthcare settings, Infect. Control Hosp. Epidemiol., 2012, 33(3), 235–240, DOI:10.1086/664047.
-
A. Pruden, M. A. Edwards and J. O. Falkinham, State of the science and research needs for opportunistic pathogens in premise plumbing, Water Research Foundation, 2013, https://www.waterrf.org/research/projects/state-science-and-research-needs-opportunistic-pathogens-premise-plumbing Search PubMed.
- H. Holsinger, N. Tucker, S. Regli, K. Studer, V. A. Roberts, S. Collier, E. Hannapel, C. Edens, J. S. Yoder and K. Rotert, Characterization of reported legionellosis outbreaks associated with buildings served by public drinking water systems: United States, 2001–2017, J. Water Health, 2022, 20(4), 702–711, DOI:10.2166/wh.2022.002.
- M. J. Donohue, S. Vesper, J. Mistry and J. M. Donohue, Impact of chlorine and chloramine on the detection and quantification of Legionella pneumophila and Mycobacterium species, Appl. Environ. Microbiol., 2019, 85(24), e01942-19, DOI:10.1128/AEM.01942-19.
- V. Mouchtouri, E. Velonakis, A. Tsakalof, C. Kapoula, G. Goutziana, A. Vatopoulos, J. Kremastinou and C. Hadjichristodoulou, Risk factors for contamination of hotel water distribution systems by Legionella species, Appl. Environ. Microbiol., 2007, 73(5), 1489–1492, DOI:10.1128/AEM.02191-06.
- P. Borella, M. T. Montagna, S. Stampi, G. Stancanelli, V. Romano-Spica, M. Triassi, I. Marchesi, A. Bargellini, D. Tatò, C. Napoli, F. Zanetti, E. Leoni, M. Moro, S. Scaltriti, G. Ribera D’Alcalà, R. Santarpia and S. Boccia,
Legionella contamination in hot water of Italian hotels, Appl. Environ. Microbiol., 2005, 71(10), 5805–5813, DOI:10.1128/AEM.71.10.5805-5813.2005.
- M. J. Donohue, D. King, S. Pfaller and J. H. Mistry, The sporadic nature of Legionella pneumophila, Legionella pneumophila Sg1 and Mycobacterium avium occurrence within residences and office buildings across 36 states in the United States, J. Appl. Microbiol., 2019, 126, 1568–1579, DOI:10.1111/jam.14196.
- L. A. Boczek, M. Tang, C. Formal, D. Lytle and H. Ryu, Comparison of two culture methods for the enumeration of Legionella pneumophila from potable water samples, J. Water Health, 2021, 19(3), 468–477, DOI:10.2166/wh.2021.051.
- M. Hirsh, J. L. Baron, S. Mietzner, J. D. Rihs and J. E. Stout, Cross-reactivity of the IDEXX Legiolert method with other Gram-negative bacteria and waterborne pathogens leads to false-positive assay results, Lett. Appl. Microbiol., 2021, 72(6), 750–756, DOI:10.1111/lam.13469.
- S. N. Monteiro, A. M. Robalo and R. J. Santos, Evaluation of Legiolert™ for the detection of Legionella pneumophila and comparison with spread-plate culture and qPCR methods, Curr. Microbiol., 2021, 78(5), 1792–1797, DOI:10.1007/s00284-021-02436-6.
- S. Ditommaso, E. Ricciardi, M. Giacomuzzi, S. R. Arauco Rivera, A. Ceccarelli and C. M. Zotti, Overestimation of the Legionella spp. load in environmental samples by quantitative real-time PCR: pretreatment with propidium monoazide as a tool for the assessment of an association between Legionella concentration and sanitary risk, Diagn. Microbiol. Infect. Dis., 2014, 80(4), 260–266, DOI:10.1016/j.diagmicrobio.2014.09.010.
- J. V. Lee, S. Lai, M. Exner, J. Lenz, V. Gaia, S. Casati, P. Hartemann, C. Lück, B. Pangon, M. L. Ricci, M. Scaturro, S. Fontana, M. Sabria, I. Sánchez, S. Assaf and S. Surman-Lee, An international trial of quantitative PCR for monitoring Legionella in artificial water systems, J. Appl. Microbiol., 2011, 110(4), 1032–1044, DOI:10.1111/j.1365-2672.2011.04957.x.
- S. Collins, D. Stevenson, J. Walker and A. Bennett, Evaluation of Legionella real-time PCR against traditional culture for routine and public health testing of water samples, J. Appl. Microbiol., 2017, 122(6), 1692–1703, DOI:10.1111/jam.13461.
- N. Wellinghausen, C. Frost and R. Marre, Detection of legionellae in hospital water samples by quantitative real-time LightCycler PCR, Appl. Environ. Microbiol., 2001, 67(9), 3985–3993, DOI:10.1128/AEM.67.9.3985-3993.2001.
- K. A. Hamilton, M. T. Hamilton, W. Johnson, P. Jjemba, Z. Bukhari, M. LeChevallier, C. N. Haas and P. L. Gurian, Risk-based critical concentrations of Legionella pneumophila for indoor residential water uses, Environ. Sci. Technol., 2019, 53(8), 4528–4541, DOI:10.1021/acs.est.8b03000.
-
European Working Group for Legionella Infections, ESGLI European technical guidelines for the prevention, control and investigation of infections caused by Legionella species, European Guidelines Working Group, 2017, https://www.escmid.org/fileadmin/src/media/PDFs/3Research_Projects/ESGLI/ESGLI_European_Technical_Guidelines_for_the_Prevention_Control_and_Investigation_of_Infections_Caused_by_Legionella_species_June_2017.pdf Search PubMed.
- K. Spies, S. Pleischl, B. Lange, B. Langer, I. Hübner, L. Jurzik, K. Luden and M. Exner, Comparison of the Legiolert™/Quanti-Tray® MPN test for the enumeration of Legionella pneumophila from potable water samples with the German regulatory requirements methods ISO 11731-2 and ISO 11731, Int. J. Hyg. Environ. Health, 2018, 221(7), 1047–1053, DOI:10.1016/j.ijheh.2018.07.006.
- S. Claassen, E. Du Toit, M. Kaba, C. Moodley, H. J. Zar and M. P. Nicol, A comparison of the efficiency of five different commercial DNA extraction kits for extraction of DNA from faecal samples, J. Microbiol. Methods, 2013, 94(2), 103–110, DOI:10.1016/j.mimet.2013.05.008.
- M. Albertsen, S. M. Karst, A. S. Ziegler, R. H. Kirkegaard and P. H. Nielsen, Back to basics – The influence of DNA extraction and primer choice on phylogenetic analysis of activated sludge communities, PLoS One, 2015, 10(7), e0132783, DOI:10.1371/journal.pone.0132783.
-
K. S. Dowdell, L. Raskin, T. Olson, S.-J. Haig, D. Dai, M. Edwards and A. Pruden, Methods for detecting and differentiating Opportunistic Premise Plumbing Pathogens (OPPPs) to determine efficacy of control and treatment technologies, Water Research Foundation, Denver, CO, 2022, https://www.waterrf.org/resource/methods-detecting-and-differentiating-opportunistic-premise-plumbing-pathogens-oppps Search PubMed.
- G. G. Clark, W. Pan, D. E. Giammar and T. H. Nguyen, Influence of point-of-use filters and stagnation on water quality at a preschool and under laboratory conditions, Water Res., 2022, 211, 118034, DOI:10.1016/j.watres.2021.118034.
-
Centers for Disease Control and Prevention, National notifiable diseases surveillance system, weekly tables of infectious disease data, https://www.cdc.gov/nndss/data-statistics/index.html Search PubMed.
-
Wisconsin Department of Health Services, Legionellosis (Legionnaires' disease and Pontiac fever), 2021, https://www.dhs.wisconsin.gov/disease/legionellosis.htm Search PubMed.
-
Schweizerische Eidgenossenschaft Eidgenössisches Departement des Innern EDI, BAG-Bulletin Woche 30/2021 - Informationsmagazin für medizinische Fachpersonen und Medienschaffende, Bern, Switzerland, 2021, https://www.bag.admin.ch/dam/bag/de/dokumente/cc/Kampagnen/Bulletin/2021/bu-30-21.pdf.download.pdf/BU_30_21_DE.pdf Search PubMed.
Footnotes |
† Electronic supplementary information (ESI) available. See DOI: https://doi.org/10.1039/d3ew00278k |
‡ Co-first authors. |
§ Current affiliation: Black and Veatch, Kansas City, MO 64114, USA. |
|
This journal is © The Royal Society of Chemistry 2023 |
Click here to see how this site uses Cookies. View our privacy policy here.