DOI:
10.1039/D1TC04207F
(Paper)
J. Mater. Chem. C, 2022,
10, 2616-2622
Diketopyrrolopyrrole-based conjugated polymers synthesized by direct arylation polycondensation for anisole-processed high mobility organic thin-film transistors†
Received
5th September 2021
, Accepted 7th October 2021
First published on 8th October 2021
Abstract
Two conjugated polymers (CPs) Fu-F and Fu-Cl that are soluble in a green solvent anisole were successfully synthesized via direct arylation polycondensation (DArP) using a furan-flanked diketopyrrolopyrrole (DPP) derivative (FDPP-Br) as the C–Br monomer and (E)-1,2-bis(3,4-difluorothien-2-yl)ethene (4FTVT) or (E)-1,2-bis(3,4-dichlorothien-2-yl)ethene (4ClTVT) as the C–H monomer. With anisole as the processing solvent and polyethylenimine ethoxylated (PEIE) as the electrode modification layer, n-type organic thin-film transistors (OTFTs) of the polymers were fabricated via bar-coating. The electron mobility (μe) of the devices based on Fu-Cl was only in the magnitude of 10−2 cm2 V−1 s−1, owing to the unfavoured fine granular film morphology. In contrast, Fu-F films showed fibre-like morphology with the polymer chains aligned along the bar-coating direction, which is favourable for the charge transport in OTFTs. Therefore, Fu-F delivered much better device performance with the μe of up to 2.79 cm2 V−1 s−1. Our study demonstrates that green solvent processed high mobility CPs can be synthesized by DArP, an emerging eco-friendly protocol for the synthesis of CPs.
1. Introduction
Conjugated polymer (CP)-based organic thin-film transistors (OTFTs) have received a lot of attention due to their intrinsic advantages of solution processability and mechanical flexibility.1–8 Significant progress in material exploration and device engineering has pushed the performance of OTFTs of up to the commercialization threshold.9–16 To date, halogenated solvents, such as chlorobenzene, o-dichlorobenzene (o-DCB), chloroform and so on, have usually been used for the fabrication of high performance OTFTs. These solvents suffer from serious health and environmental issues.17–21 Therefore, the quest for high mobility CPs processible with green solvents is a matter of intense research.17,18,22,23
Diketopyrrolopyrrole (DPP)-based CPs represent one class of high mobility CPs that have been investigated the most in the past years.24–26 In the above context, some DPP-based high mobility CPs processible with non-halogenated solvents, such as toluene, xylene, tetralin and tetrahydrofuran (THF), were also reported.27–38 Lowering the regularity is generally adopted as an effective method to enhance the solubility of CPs.27–29,39 For instance, Li et al. reported toluene-processed DPP-based high mobility CPs with decreased regioregularity.28 Kwon et al. also demonstrated a DPP-based random copolymer for tetralin-processed OTFTs with a hole mobility (μh) of up to 6.05 cm2 V−1 s−1.29 DPP-based CPs soluble in non-chlorinated solvents such as toluene, THF and hexane have also been demonstrated by incorporating furan into conjugated backbones.39–41 Recently, we found that the solubility of DPP-based CPs could be remarkably enhanced by enriching the density of alkyl side chains, and accordingly poly(diketopyrrolopyrrole-alt-terchalcogenophene)s were soluble in non-chlorinated solvent o-xylene.42,43 As a result, OTFTs with the μh of around 10 cm2 V−1 s−1 were fabricated via bar-coating with o-xylene as the processing solvent.43 In addition, the poly(diketopyrrolopyrrole-alt-terchalcogenophene)s based on furan-flanked DPP (FDPP) are even soluble in anisole, which is a green solvent according to the CHEM21 and Sanofi's solvent selection guides44,45 and is used as the fragrance for cosmetics and as the additive for foods. OTFTs with a μh of up to 3.5 cm2 V−1 s−1 were prepared with this solvent.43 Note that the O⋯H non-covalent intramolecular interactions also occur in FDPP,46 which is beneficial for the synthesis of high mobility CPs.
Besides high mobility CPs processed using eco-friendly solvents, other aspects, such as material synthesis/purification and all steps of device fabrication, are expected to be green in the future applications of OTFTs. In this regard, environmentally benign and atom-economical protocols for the synthesis of high mobility CPs are also highly desired. At present, high mobility CPs are mainly prepared by Pd-catalysed Stille or Suzuki polycondensation, which need high-purity organometallic reagents prepared using tedious steps. Moreover, the organotin wastes produced in Stille polycondensation are highly toxic.47–49 By contrast, direct arylation polycondensation (DArP) invokes C–H bond activation in the selected monomer through concerted metalation–deprotonation (CMD) instead of transmetalation, which reduces the synthetic steps of monomers and the production of toxic byproducts.50–55 Therefore, DArP is recognized as an emerging protocol for the environmentally benign and atom-economical synthesis of CPs.50–57
Based on the above discussion, it is desirable to synthesize green solvent processible high mobility CPs via DArP. In the current paper, we selected a FDPP derivative as the C–Br monomer to conduct DArP with two highly reactive C–H monomers, i.e., (E)-1,2-bis(3,4-difluorothien-2-yl)ethene (4FTVT)58–60 and (E)-1,2-bis(3,4-dichlorothien-2-yl)ethene (4ClTVT).61 Two CPs, named Fu-F and Fu-Cl, that are soluble in o-xylene and anisole were successfully synthesized (Scheme 1). Top-gate/bottom-contact (TGBC) OTFTs with a reliable electron mobility (μe) of up to 2.79 cm2 V−1 s−1 have been fabricated with anisole as the solvent. To the best of our knowledge, this has been the highest electron mobility reported so far for the real green solvent processed n-type OTFTs. Our study, for the first time, offers high mobility CPs that are synthesized via an environmentally benign protocol DArP and meanwhile can be processed using a green solvent.
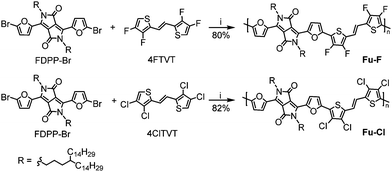 |
| Scheme 1 Synthetic routes for the polymers. Reaction conditions: (i) Herrmann's cat., P(o-MeOPh)3, PivOH, Cs2CO3, toluene, 120 °C. | |
2. Results and discussion
2.1. Synthesis and characterization
The synthetic route to the polymers Fu-F and Fu-Cl is depicted in Scheme 1 and the details of synthesis are summarized in the (ESI†). 4FTVT, 4ClTVT and 3,6-bis(5-bromofuran-2-yl)-2,5-bis(4-tetradecyloctadecyl)-pyrrolo[3,4-c]pyrrole-1,4(2H,5H)-dione (FDPP-Br) were prepared according to previous studies.43,58,61 DArP was conducted following our previously reported procedure by using toluene as the solvent.58,61 The resulting Fu-F and Fu-Cl were purified by precipitation in methanol and Soxhlet extraction with ethanol, acetone and hexane in succession. The molecular weights of the polymers were determined by high-temperature gel permeation chromatography (HT-GPC) at 150 °C using 1,2,4-trichlorobenzene (TCB) as the eluent and polystyrene (PS) as the standard. The number-average molecular weights (Mns) of Fu-F and Fu-Cl are 37.2 and 63.0 kDa, respectively, with the corresponding polydispersity indices (Đ) of 2.1 and 2.3. The thermal properties of the two polymers were characterized by thermogravimetric analysis (TGA) and differential scanning calorimetry (DSC) under a nitrogen atmosphere. Both Fu-F and Fu-Cl exhibited excellent thermal stability with decomposition temperatures (Td, 5% weight loss) of 415 and 407 °C, respectively (Fig. S1a, ESI†). As indicated by DSC scans (Fig. S1b, ESI†), there is no obvious thermal transition in the range of 25 to 300 °C for both polymers.
The solubilities of Fu-F and Fu-Cl in o-DCB, o-xylene, and anisole that are ranked as hazardous, problematic, and recommended,44,45,62,63 respectively, were investigated. As shown in Table S1 (ESI†), both polymers could be easily dissolved in o-xylene and o-DCB. They were also soluble in warm anisole with solubilities reaching 5 mg mL−1 at 70 °C, allowing the fabrication of OTFTs with anisole as the solvent. In contrast, analogous polymers based on thiophene-flanked DPP (TDPP), i.e., Th-F and Th-Cl,61,64 are almost insoluble in anisole (Table S1, ESI†). This observation is consistent with our previous study on poly(diketopyrrolopyrrole-alt-terchalcogenophene)s: introducing furan units at 3,6-positions of DPP can dramatically improve the solubilities of the DPP-based CPs.43
2.2. Optical and electrochemical properties
The solution absorption spectra of Fu-F and Fu-Cl in anisole, o-xylene and o-DCB and the absorption spectra of their thin films prepared using these three solvents were recorded (Fig. 1 and Fig. S2, ESI†), and the related data are summarized in Table 1 and Table S2 (ESI†). Both polymers show similar absorption features with two absorption bands, which is common to DPP-based CPs.42,43,58,61,64 Solvent effect on the absorption spectra in both solution and film states is rather small. As shown in Table 1, the low energy absorption maxima (λmaxs) of Fu-F in three solvents are slightly different, which are at 797, 790 and 792 nm in anisole, o-xylene and o-DCB, respectively. A slightly red-shifted λmax in anisole may be attributed to the stronger aggregation tendency of the polymer in this solvent.65,66 The absorption spectra of the Fu-F pristine films prepared from these three solvents are almost overlapped (Fig. S2, ESI†), with 2–7 nm red-shifted λmaxs compared with those measured in solutions. The absorption spectra of the films before and after thermal annealing (200 °C for 10 min) are similar. Fu-Cl shows similar behaviour, but its λmaxs were red-shifted ca. 20 nm in solution and ca. 10 nm in film when compared with Fu-F. This phenomenon can be attributed to the stronger intramolecular charge transfer (ICT) of Fu-Cl originating from the larger dipole moment of the C–Cl bond.67,68 The absorption spectra of Fu-F and Fu-Cl display ca. 30 nm blue-shifts compared with those of TDPP-based analogous polymers Th-F and Th-Cl,61,64 ascribed to the effect of the size and electronegativity of the heteroatoms.
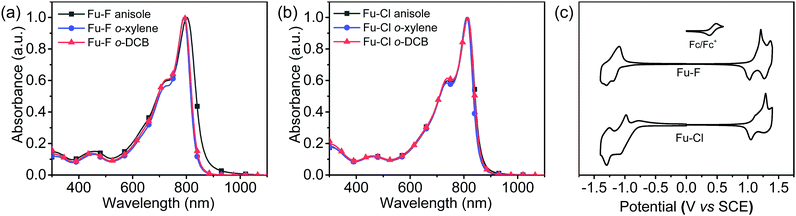 |
| Fig. 1 Solution UV-vis-NIR absorption spectra of Fu-F (a) and Fu-Cl (b) and film cyclic voltammograms (CVs) (c) of the polymers. Solution spectra were measured in anisole, o-xylene and o-DCB with a concentration of 10−5 mol L−1 of the repeating units. Films for CV measurements were prepared by spin-coating the o-DCB solutions on working electrodes. | |
Table 1 Optical properties and energy levels of the polymers
Polymer |
Anisole |
o-Xylene |
o-DCB |
E
reonset/ELUMOb (V/eV) |
E
oxonset/EHOMOb (V/eV) |
λ
solmax (nm) |
λ
filmmax a (nm) |
λ
solmax (nm) |
λ
filmmax a (nm) |
λ
solmax (nm) |
λ
filmmax a (nm) |
The absorption maxima of pristine films without thermal annealing.
E
LUMO and EHOMO calculated according to ELUMO = −(4.40 + Ereonset) eV and EHOMO = −(4.40 + Eoxonset) eV, in which Ereonset and Eoxonset represent reduction and oxidation onset potentials of the polymers versus SCE, respectively.
|
Fu-F
|
724, 797 |
719, 799 |
721, 790 |
713, 797 |
725, 792 |
713, 799 |
−0.84/−3.56 |
1.04/−5.44 |
Fu-Cl
|
741, 813 |
735, 810 |
736, 811 |
737, 811 |
744, 813 |
733, 814 |
−0.82/−3.58 |
1.05/−5.45 |
Film cyclic voltammograms (CVs) of the polymers (Fig. 1c) were recorded to estimate their highest occupied molecular orbital (HOMO) and lowest unoccupied molecular orbital (LUMO) energy levels. The redox onset potentials (Ereonset and Eoxonset) and calculated HOMO and LUMO energy levels (EHOMO and ELUMO) are summarized in Table 1. Both Fu-F and Fu-Cl exhibited quasi-reversible reduction and oxidation processes. The EHOMO/ELUMO values are −5.44/−3.56 eV for Fu-F and −5.45/−3.58 eV for Fu-Cl. Fu-F and Fu-Cl display deeper HOMO levels than Th-F and Th-Cl, which had the EHOMO values of −5.34 and −5.41 eV, respectively, owing to the high ionization potential of furan.69
2.3. Charge transport properties
TGBC OTFTs were fabricated to evaluate the charge transport properties of the polymers. The semiconducting layer was deposited either by spin-coating or bar-coating followed by thermal annealing. The device performance was measured under ambient conditions, and the mobility values were extracted from the transfer characteristics in the saturation regime. The performance data of spin-coated and bar-coated OTFTs are summarized in Table S3 (ESI†) and Table 2, respectively.
Table 2 The performance of bar-coated OTFT devices of the polymersa
Polymer |
Solvent |
T
A
(°C) |
Without modification |
With PEIE modification |
n-Channel |
p-Channel |
n-Channel |
μ
e,max
(μe,avgd) (cm2 V−1 s−1) |
V
T
(V) |
I
on/Iofff |
μ
h,max
(μh,avgh) (cm2 V−1 s−1) |
V
T
(V) |
I
on/Iofff |
μ
e,max
(μe,avgd) (cm2 V−1 s−1)ri (%) |
V
T
(V) |
I
on/Iofff |
The devices were measured under ambient conditions.
Thermal annealing temperature.
Maximum electron mobility calculated from the saturation regime.
Average electron mobility calculated from at least 15 devices.
Threshold voltage.
Current on/off ratio.
Maximum hole mobility calculated from the saturation regime.
Average hole mobility calculated from at least 15 devices.
Reliability factor.
|
Fu-F
|
Anisole |
150 |
8.6 × 10−3 (7.5 × 10−3) |
49–54 |
103–104 |
2.9 × 10−3 (2.1 × 10−3) |
−30–−35 |
102–103 |
|
|
|
|
|
200 |
2.76 (2.42) |
42–46 |
102–103 |
0.53 (0.41) |
−48–−53 |
102–103 |
2.79 (2.48)78% |
7–10 |
106–107 |
|
|
250 |
1.03 (0.88) |
50–55 |
103–104 |
0.34 (0.26) |
−58–−61 |
103–104 |
|
|
|
Fu-F
|
o-Xylene |
200 |
1.07 (0.90) |
51–55 |
103–104 |
0.43 (0.35) |
−59–−63 |
104–105 |
0.70 (0.58)75% |
7–11 |
106–107 |
Fu-F
|
o-DCB |
200 |
2.23 (1.97) |
48–54 |
103–104 |
0.73 (0.62) |
−56–−60 |
103–104 |
2.21 (2.01)82% |
8–13 |
103–104 |
Fu-Cl
|
Anisole |
150 |
6.3 × 10−3 (5.1 × 10−3) |
40–45 |
103–104 |
1.5 × 10−3 (1.0 × 10−3) |
−30–−35 |
103–104 |
|
|
|
|
|
200 |
1.3 × 10−2 (9.9 × 10−3) |
44–48 |
102–103 |
3.5 × 10−3 (2.5 × 10−3) |
−45–−50 |
102–103 |
1.5 × 10−2 (1.1 × 10−2)77% |
10–20 |
103–104 |
|
|
250 |
3.6 × 10−3 (2.9 × 10−3) |
45–50 |
103–104 |
2.9 × 10−3 (1.8 × 10−3) |
−45–−49 |
103–104 |
|
|
|
First, OTFTs of Fu-F and Fu-Cl were prepared by spin-coating with anisole as the solvent and bare gold (Au) as source and drain electrodes. All devices exhibited electron-dominant ambipolar charge transport properties (Fig. S3 and S4, ESI†), consistent with the HOMO and LUMO energy levels of the polymers. The devices based on the semiconducting layers thermally annealed at 200 °C demonstrated the highest mobilities, with the maximum electron and hole mobilities (μe,max and μh,max) of 1.30 and 0.36 cm2 V−1 s−1 for Fu-F and 1.1 × 10−2 and 2.7 × 10−3 cm2 V−1 s−1 for Fu-Cl (Table S3, ESI†).
Bar-coating is a printing technique suitable for the preparation of large area semiconducting layer in laboratory. Then, we fabricated OTFTs via bar-coating with anisole as the solvent. Fig. 2 shows the representative transfer and output curves of the bar-coated devices based on Fu-F and Fu-Cl, which are typical for ambipolar OTFTs. As shown in Table 2, bar-coated OTFTs of Fu-F with thermal annealing under optimized conditions (200 °C for 10 min) exhibited remarkably improved performance compared with spin-coated OTFTs, with μe,max and μh,max of 2.76 and 0.53 cm2 V−1 s−1, respectively. However, the μe,max and μh,max of OTFTs based on Fu-Cl were only slightly enhanced to 1.3 × 10−2 and 3.5 × 10−3 cm2 V−1 s−1, respectively. Bar-coated OTFTs of Fu-F were also fabricated with o-DCB and o-xylene as the solvents. The μe,max/μh,max of the Fu-F-based devices processed with o-DCB and o-xylene were 2.23/0.73 and 1.07/0.43 cm2 V−1 s−1, respectively. This indicates that green solvent can also deliver OTFTs with device performance superior to conventional chlorinated solvents. The above solvent dependence of device performance will be discussed thereinafter. Note that although a high μe was obtained, the threshold voltage (VT) was rather high (Fig. 2 and Fig. S2–S6, ESI†) probably due to the parasitic resistance caused by the mismatch between the LUMO energy levels of the polymers and the work function of Au electrodes, resulting in non-ideal transistor behaviour.
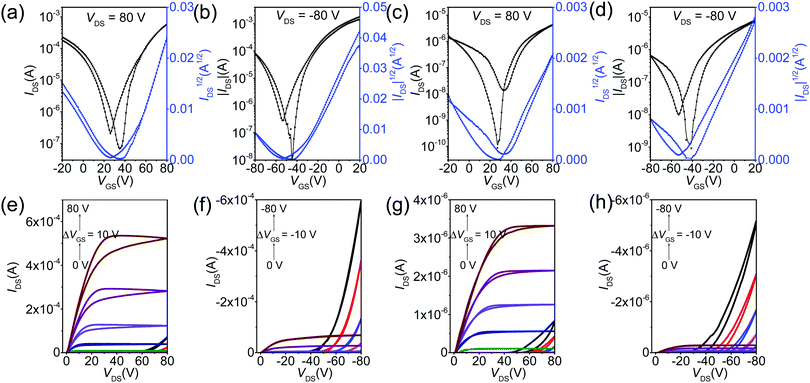 |
| Fig. 2 Typical transfer (a–d) and output (e–h) curves of the OTFTs of Fu-F (a, b, e and f) and Fu-Cl (c, d, g and h) prepared by bar-coating. Thin films were prepared from anisole solutions on bare Si/SiO2 substrates and annealed at 200 °C for 10 min in an inert atmosphere. | |
To address the above issue, an ultrathin polyethylenimine ethoxylated (PEIE) layer was inserted between the Au electrodes and the polymer layer. The corresponding energy level diagrams are depicted in Fig. S7 (ESI†). It has been demonstrated that PEIE modification can effectively lower the work function of Au, and then facilitate efficient electron injection and block the hole injection from electrodes to the semiconducting layer.70,71 Accordingly, all OTFTs with PEIE interlayer exhibited pure electron transport characteristics with much lower VT (Fig. 3 and Fig. S8, S9, ESI†). As shown in Fig. 3c and d, obvious linear and saturation regions present in the output curves, and almost no hysteresis can be observed at different gate voltages (VGS). Moreover, (IDS)1/2versus VGS curves are linear with the absence of kinks. All these indicate that the devices operated in a near-ideal field-effect transistor mode. As shown in Table 2, bar-coated OTFTs of Fu-F with PEIE modification exhibited the μe,max of 2.79, 0.70 and 2.21 cm2 V−1 s−1, respectively, when anisole, o-xylene and o-DCB were used as processing solvents. Bar-coated OTFTs of Fu-Cl with PEIE modification still showed inferior performance with a μe,max of 1.5 × 10−2 cm2 V−1 s−1, similar to the results observed in the OTFTs without the PEIE interlayer (Table 2). As shown in Fig. 3e and f, the electron mobility (μe) of the devices was almost independent of VGS, indicating that the μe values are highly reliable.
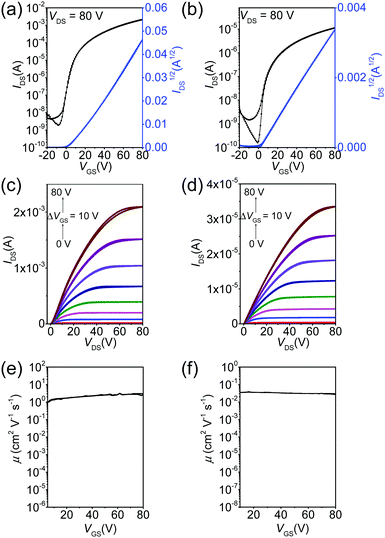 |
| Fig. 3 Typical transfer (a and b), output (c and d) curves and saturation mobility versus VGS (e and f) of OTFT devices based on Fu-F (a, c and e) and Fu-Cl (b, d and f) prepared via bar-coating. Thin films were prepared from anisole solution on 0.08 wt% PEIE-modified substrates and annealed at 200 °C for 10 min in an inert atmosphere. | |
2.4. Film morphology and microstructures
Fig. 4 shows the atomic force microscopy (AFM) height images of the spin- and bar-coated films of the polymers on bare Si/SiO2 substrates. The bar-coated films of Fu-F from all three solvents exhibited a fibre-like morphology (Fig. 4b–d), while the short grains were observed in the film spin-cast from anisole (Fig. 4a). The fibre-like nanostructures were oriented along the bar-coating direction for the films processed with anisole and o-DCB, while the nanostructures distributed almost isotropically in the film prepared with o-xylene. The films of Fu-Cl, prepared by either spin-coating or bar-coating, featured a fine granular morphology. A similar film morphology was observed for both polymers on PEIE-modified substrates (Fig. S10, ESI†). All these observations are consistent with the OTFT performance of the polymers discussed above. Fu-F is characterized by a favourable film morphology and can form aligned films via bar-coating with both anisole and o-DCB as the solvents, giving rise to high mobility. In contrast, the fine granular morphology of Fu-Cl films is not beneficial to charge transport, and the OTFTs based on Fu-Cl thus exhibited low charge carrier mobility. The bar-coated polymer films were also characterized by X-ray diffraction (XRD) with the incident light parallel to the coating direction. As shown in Fig. S11 (ESI†), only a weak (100) peak was observed in the out-of-plane diffraction patterns for the films of Fu-F and Fu-Cl. This phenomenon indicates the almost amorphous nature of these two polymers and is consistent with their better solubility compared to TDPP counterparts Th-F and Th-Cl.
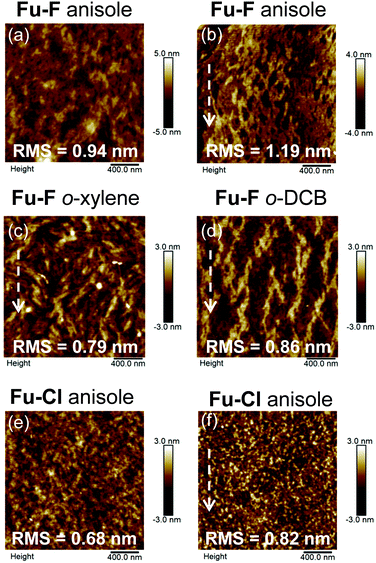 |
| Fig. 4 AFM height images (2 μm × 2 μm) of the spin-coated (a and e) and bar-coated (b, c, d and f) polymer films from anisole, o-xylene and o-DCB solutions. Thin films were prepared on bare Si/SiO2 substrates and annealed at 200 °C for 10 min under an inert atmosphere. The arrows in the images denote the bar-coating direction. | |
To further confirm the formation of aligned films of Fu-F with bar-coating, film absorption spectra under linearly polarized light were measured and the corresponding dichroic ratio (R) values were calculated (Fig. S12, ESI†). As expected, the R values of the Fu-F films bar-coated with anisole and o-DCB were 2.89 and 2.38, respectively. This implies that the polymer chains of Fu-F were aligned along the bar-coating direction. In contrast, all the Fu-Cl films and the film of Fu-F bar-coated with o-xylene showed the R values close to 1, indicative of the isotropic nature of the films.
3. Conclusions
In summary, two FDPP-based CPs have been synthesized by DArP. The resulting CPs Fu-F and Fu-Cl can also be dissolved in a green solvent anisole, besides being soluble in o-xylene and o-DCB. Fu-F displayed a much higher mobility compared to Fu-Cl, mainly ascribed to the favourable film morphology of Fu-F. OTFTs of Fu-F processed with anisole exhibited a performance comparable to and better than that of the devices fabricated with o-DCB and o-xylene, respectively. With source/drain electrodes modified via a PEIE interlayer, n-type OTFTs of Fu-F with reliable μe values of up to 2.79 cm2 V−1 s−1, have been fabricated. This study demonstrates a high mobility CP that can be synthesized via an environmentally benign protocol (i.e., DArP) and processed using the green solvent anisole, which is of significance for future commercialization of OTFTs. To further improve the device performance of green solvent processed CPs, the comprehensive design of side chains and conjugated backbones based on the deep understanding on the solution aggregation behaviour of CPs in different organic solvents is highly desired.
Conflicts of interest
There are no conflicts to declare.
Acknowledgements
This work is supported by the National Natural Science Foundation of China (no. 51933008) and the Tianjin Natural Science Foundation (no. 19JCYBJC18100).
Notes and references
- X. Guo, A. Facchetti and T. J. Marks, Chem. Rev., 2014, 114, 8943–9021 CrossRef CAS
.
- S. Holliday, J. E. Donaghey and I. McCulloch, Chem. Mater., 2013, 26, 647–663 CrossRef
.
- Z. Liu, G. Zhang and D. Zhang, Acc. Chem. Res., 2018, 51, 1422–1432 CrossRef CAS
.
- H. Sirringhaus, Adv. Mater., 2014, 26, 1319–1335 CrossRef CAS
.
- S. Yuvaraja, A. Nawaz, Q. Liu, D. Dubal, S. G. Surya, K. N. Salama and P. Sonar, Chem. Soc. Rev., 2020, 49, 3423–3460 RSC
.
- F. Huang, Z. Bo, Y. Geng, X. Wang, L. Wang, Y. Ma, J. Hou, W. Hu, J. Pei, H. Dong, S. Wang, Z. Li, Z. Shuai, Y. Li and Y. Cao, Acta Polym. Sin., 2019, 50, 988–1046 CAS
.
- Z. F. Yao, Q. Y. Li, H. T. Wu, Y. F. Ding, Z. Y. Wang, Y. Lu, J. Y. Wang and J. Pei, SmartMat, 2021, 2, 378–387 CrossRef
.
- J. Ouyang, SmartMat, 2021, 2, 263–285 CrossRef
.
- J. Y. Back, H. Yu, I. Song, I. Kang, H. Ahn, T. J. Shin, S.-K. Kwon, J. H. Oh and Y.-H. Kim, Chem. Mater., 2015, 27, 1732–1739 CrossRef CAS
.
- G. Kim, S. J. Kang, G. K. Dutta, Y. K. Han, T. J. Shin, Y. Y. Noh and C. Yang, J. Am. Chem. Soc., 2014, 136, 9477–9483 CrossRef CAS
.
- J. Lee, S. H. Kang, S. M. Lee, K. C. Lee, H. Yang, Y. Cho, D. Han, Y. Li, B. H. Lee and C. Yang, Angew. Chem., Int. Ed., 2018, 57, 13629–13634 CrossRef CAS PubMed
.
- J. Li, Y. Zhao, H. S. Tan, Y. Guo, C. A. Di, G. Yu, Y. Liu, M. Lin, S. H. Lim, Y. Zhou, H. Su and B. S. Ong, Sci. Rep., 2012, 2, 754 CrossRef
.
- C. Luo, A. K. Kyaw, L. A. Perez, S. Patel, M. Wang, B. Grimm, G. C. Bazan, E. J. Kramer and A. J. Heeger, Nano Lett., 2014, 14, 2764–2771 CrossRef CAS
.
- Y. Yang, Z. Liu, J. Chen, Z. Cai, Z. Wang, W. Chen, G. Zhang, X. Zhang, L. Chi and D. Zhang, Adv. Sci., 2018, 5, 1801497 CrossRef
.
- Y. Sui, Y. Deng, T. Du, Y. Shi and Y. Geng, Mater. Chem. Front., 2019, 3, 1932–1951 RSC
.
- J. Liu, L. Jiang, W. Hu, Y. Liu and D. Zhu, Sci. China: Chem., 2019, 62, 313–330 CrossRef CAS
.
- F. Campana, C. Kim, A. Marrocchi and L. Vaccaro, J. Mater. Chem. C, 2020, 8, 15027–15047 RSC
.
- J. Cho, S. H. Yu and D. S. Chung, J. Mater. Chem. C, 2017, 5, 2745–2757 RSC
.
- Agency for Toxic Substances & Disease Registry. Toxicological Profile for Chloroform, 2021, https://www.atsdr.cdc.gov/ToxProfiles/tp.asp.
- Agency for Toxic Substances & Disease Registry. Toxicological Profile for Chlorobenzene, 2021, https://www.atsdr.cdc.gov/ToxProfiles/tp.asp.
- Agency for Toxic Substances & Disease Registry. Public Health Statement for Dichlorobenzenes, 2021, https://www.atsdr.cdc.gov/phs/phs.asp.
- D. Ho, J. Lee, S. Park, Y. Park, K. Cho, F. Campana, D. Lanari, A. Facchetti, S. Seo, C. Kim, A. Marrocchi and L. Vaccaro, J. Mater. Chem. C, 2020, 8, 5786–5794 RSC
.
- J. Lee, S. A. Park, S. U. Ryu, D. Chung, T. Park and S. Y. Son, J. Mater. Chem. A, 2020, 8, 21455–21473 RSC
.
- Y. Yang, Z. Liu, G. Zhang, X. Zhang and D. Zhang, Adv. Mater., 2019, 31, e1903104 CrossRef
.
- C. B. Nielsen, M. Turbiez and I. McCulloch, Adv. Mater., 2013, 25, 1859–1880 CrossRef CAS PubMed
.
- M. Kim, S. U. Ryu, S. A. Park, K. Choi, T. Kim, D. Chung and T. Park, Adv. Funct. Mater., 2019, 30, 1904545 CrossRef
.
- H. H. Choi, J. Y. Baek, E. Song, B. Kang, K. Cho, S. K. Kwon and Y. H. Kim, Adv. Mater., 2015, 27, 3626–3631 CrossRef CAS PubMed
.
- Y. Ji, C. Xiao, Q. Wang, J. Zhang, C. Li, Y. Wu, Z. Wei, X. Zhan, W. Hu, Z. Wang, R. A. Janssen and W. Li, Adv. Mater., 2016, 28, 943–950 CrossRef CAS PubMed
.
- H. J. Yun, G. B. Lee, D. S. Chung, Y. H. Kim and S. K. Kwon, Adv. Mater., 2014, 26, 6612–6616 CrossRef CAS
.
- M. Lee, M. J. Kim, S. Ro, S. Choi, S. M. Jin, H. D. Nguyen, J. Yang, K. K. Lee, D. U. Lim, E. Lee, M. S. Kang, J. H. Choi, J. H. Cho and B. Kim, ACS Appl. Mater. Interfaces, 2017, 9, 28817–28827 CrossRef CAS PubMed
.
- W.-Y. Lee, G. Giri, Y. Diao, C. J. Tassone, J. R. Matthews, M. L. Sorensen, S. C. B. Mannsfeld, W.-C. Chen, H. H. Fong, J. B. H. Tok, M. F. Toney, M. He and Z. Bao, Adv. Funct. Mater., 2014, 24, 3524–3534 CrossRef CAS
.
- J. R. Matthews, W. Niu, A. Tandia, A. L. Wallace, J. Hu, W.-Y. Lee, G. Giri, S. C. B. Mannsfeld, Y. Xie, S. Cai, H. H. Fong, Z. Bao and M. He, Chem. Mater., 2013, 25, 782–789 CrossRef CAS
.
- B. Fu, C.-Y. Wang, B. D. Rose, Y. Jiang, M. Chang, P.-H. Chu, Z. Yuan, C. Fuentes-Hernandez, B. Kippelen, J.-L. Brédas, D. M. Collard and E. Reichmanis, Chem. Mater., 2015, 27, 2928–2937 CrossRef CAS
.
- M. M. Dereje, D. Ji, S.-H. Kang, C. Yang and Y.-Y. Noh, Dyes Pigm., 2017, 145, 270–276 CrossRef CAS
.
- I. Song, H.-W. Kim, J. Ahn, M. Han, S.-K. Kwon, Y.-H. Kim and J. H. Oh, J. Mater. Chem. C, 2019, 7, 14977–14985 RSC
.
- H.-J. Yun, J. Cho, D. S. Chung, Y.-H. Kim and S.-K. Kwon, Macromolecules, 2014, 47, 7030–7035 CrossRef CAS
.
- G.-J. N. Wang, F. Molina-Lopez, H. Zhang, J. Xu, H.-C. Wu, J. Lopez, L. Shaw, J. Mun, Q. Zhang, S. Wang, A. Ehrlich and Z. Bao, Macromolecules, 2018, 51, 4976–4985 CrossRef CAS
.
- G. Feng, Y. Xu, C. Xiao, J. Zhang, X. Zhang, C. Li, Z. Wei, W. Hu, Z. Wang and W. Li, Polym. Chem., 2016, 7, 164–170 RSC
.
- S. Ding, Z. Ni, M. Hu, G. Qiu, J. Li, J. Ye, X. Zhang, F. Liu, H. Dong and W. Hu, Macromol. Rapid Commun., 2018, 39, e1800225 CrossRef PubMed
.
- P. Sonar, J. Chang, J. H. Kim, K. H. Ong, E. Gann, S. Manzhos, J. Wu and C. R. McNeill, ACS Appl. Mater. Interfaces, 2016, 8, 24325–24330 CrossRef CAS
.
- S. M. Lee, H. R. Lee, A. R. Han, J. Lee, J. H. Oh and C. Yang, ACS Appl. Mater. Interfaces, 2017, 9, 15652–15661 CrossRef CAS PubMed
.
- Z. Wang, X. Song, Y. Jiang, J. Zhang, X. Yu, Y. Deng, Y. Han, W. Hu and Y. Geng, Adv. Sci., 2019, 6, 1902412 CrossRef CAS
.
- Z. Wang, Y. Shi, Y. Deng, Y. Han and Y. Geng, Adv. Funct. Mater., 2021, 2104881 CrossRef CAS
.
- D. Prat, A. Wells, J. Hayler, H. Sneddon, C. R. McElroy, S. Abou-Shehada and P. J. Dunn, Green Chem., 2016, 18, 288–296 RSC
.
- D. Prat, O. Pardigon, H.-W. Flemming, S. Letestu, V. Ducandas, P. Isnard, E. Guntrum, T. Senac, S. Ruisseau, P. Cruciani and P. Hosek, Org. Process Res. Dev., 2013, 17, 1517–1525 CrossRef CAS
.
- C. Fu, F. Bélanger-Gariépy and D. F. Perepichka, CrystEngComm, 2016, 18, 4285–4289 RSC
.
- J. R. Pouliot, F. Grenier, J. T. Blaskovits, S. Beaupre and M. Leclerc, Chem. Rev., 2016, 116, 14225–14274 CrossRef CAS
.
- Y. Ran, Y. Guo and Y. Liu, Mater. Horiz., 2020, 7, 1955–1970 RSC
.
- Y. Geng and Y. Sui, Acta Polym. Sin., 2019, 20, 109–116 Search PubMed
.
- T. Bura, S. Beaupre, M. A. Legare, J. Quinn, E. Rochette, J. T. Blaskovits, F. G. Fontaine, A. Pron, Y. Li and M. Leclerc, Chem. Sci., 2017, 8, 3913–3925 RSC
.
- N. S. Gobalasingham and B. C. Thompson, Prog. Polym. Sci., 2018, 83, 135–201 CrossRef CAS
.
- A. Facchetti, L. Vaccaro and A. Marrocchi, Angew. Chem., Int. Ed., 2012, 51, 3520–3523 CrossRef CAS PubMed
.
- M. Wakioka and F. Ozawa, Asian J. Org. Chem., 2018, 7, 1206–1216 CrossRef CAS
.
- S. Phan and C. K. Luscombe, Trends Chem., 2019, 1, 670–681 CrossRef CAS
.
- A. S. Dudnik, T. J. Aldrich, N. D. Eastham, R. P. Chang, A. Facchetti and T. J. Marks, J. Am. Chem. Soc., 2016, 138, 15699–15709 CrossRef CAS PubMed
.
- Z. Ni, H. Wang, H. Dong, Y. Dang, Q. Zhao, X. Zhang and W. Hu, Nat. Chem., 2019, 11, 271–277 CrossRef CAS PubMed
.
- X. Guo, Y. Zhang, Y. Hu, J. Yang, Y. Li, Z. Ni, H. Dong and W. Hu, Angew. Chem., Int. Ed., 2021, 60, 14902–14908 CrossRef CAS
.
- Y. Gao, X. Zhang, H. Tian, J. Zhang, D. Yan, Y. Geng and F. Wang, Adv. Mater., 2015, 27, 6753–6759 CrossRef CAS
.
- K. Guo, J. Bai, Y. Jiang, Z. Wang, Y. Sui, Y. Deng, Y. Han, H. Tian and Y. Geng, Adv. Funct. Mater., 2018, 28, 1801097 CrossRef
.
- K. Guo, Y. Jiang, Y. Sui, Y.-F. Deng and Y.-H. Geng, Chin. J. Polym. Sci., 2019, 37, 1099–1104 CrossRef CAS
.
- Y. Sui, Y. Shi, Y. Deng, R. Li, J. Bai, Z. Wang, Y. Dang, Y. Han, N. Kirby, L. Ye and Y. Geng, Macromolecules, 2020, 53, 10147–10154 CrossRef CAS
.
- R. K. Henderson, C. Jiménez-González, D. J. C. Constable, S. R. Alston, G. G. A. Inglis, G. Fisher, J. Sherwood, S. P. Binks and A. D. Curzons, Green Chem., 2011, 13, 854–862 RSC
.
- C. M. Alder, J. D. Hayler, R. K. Henderson, A. M. Redman, L. Shukla, L. E. Shuster and H. F. Sneddon, Green Chem., 2016, 18, 3879–3890 RSC
.
- Y. Gao, J. Bai, Y. Sui, Y. Han, Y. Deng, H. Tian, Y. Geng and F. Wang, Macromolecules, 2018, 51, 8752–8760 CrossRef CAS
.
- B. Fan, L. Ying, Z. Wang, B. He, X.-F. Jiang, F. Huang and Y. Cao, Energy Environ. Sci., 2017, 10, 1243–1251 RSC
.
- Z. Wang, Z. Liu, L. Ning, M. Xiao, Y. Yi, Z. Cai, A. Sadhanala, G. Zhang, W. Chen, H. Sirringhaus and D. Zhang, Chem. Mater., 2018, 30, 3090–3100 CrossRef CAS
.
- H. Zhang, H. Yao, J. Hou, J. Zhu, J. Zhang, W. Li, R. Yu, B. Gao, S. Zhang and J. Hou, Adv. Mater., 2018, 30, e1800613 CrossRef PubMed
.
- Q. Zhao, J. Qu and F. He, Adv. Sci., 2020, 7, 2000509 CrossRef CAS PubMed
.
- D. Chandran, T. Marszalek, W. Zajaczkowski, P. K. Madathil, R. K. Vijayaraghavan, Y.-H. Koh, S.-Y. Park, J. R. Ochsmann, W. Pisula and K.-S. Lee, Polymer, 2015, 73, 205–213 CrossRef CAS
.
- J. Bai, Y. Jiang, Z. Wang, Y. Sui, Y. Deng, Y. Han and Y. Geng, Adv. Electron. Mater., 2019, 6, 1901002 CrossRef
.
- Y. Zhou, C. Fuentes-Hernandez, J. Shim, J. Meyer, A. J. Giordano, H. Li, P. Winget, T. Papadopoulos, H. Cheun, J. Kim, M. Fenoll, A. Dindar, W. Haske, E. Najafabadi, T. M. Khan, H. Sojoudi, S. Barlow, S. Graham, J.-L. Brédas, S. R. Marder, A. Kahn and B. Kippelen, Science, 2012, 336, 317–332 CrossRef
.
Footnote |
† Electronic supplementary information (ESI) available. See DOI: 10.1039/d1tc04207f |
|
This journal is © The Royal Society of Chemistry 2022 |
Click here to see how this site uses Cookies. View our privacy policy here.