DOI:
10.1039/D3VA00113J
(Critical Review)
Environ. Sci.: Adv., 2024,
3, 543-560
Environmental contamination and climate change in Antarctic ecosystems: an updated overview
Received
2nd May 2023
, Accepted 9th February 2024
First published on 13th February 2024
Abstract
Abiotic and biotic components of Antarctic ecosystems are valuable archives of past and current trends in global processes and play an important role in assessing emissions and long-range transport of persistent contaminants. After the ban on the production and use of alkyl-lead fuel additives, lead concentrations in Antarctic environmental matrices (snow, ice, sediments and biota) have decreased, just as the hole in the Antarctic stratospheric ozone layer is slowly shrinking following the ban on ozone-depleting gases. With the entry into force of the Stockholm Convention, the occurrence of persistent organic pollutants (POPs) in the Antarctic ecosystems could also decrease. However, the increasing anthropogenic sources of POPs in the Southern Hemisphere and the remobilization of those previously deposited in Antarctic ice could counteract the possible decreasing trend. Legacy pollutant concentrations in Antarctica are among the lowest reported in the global environment, with an exception of the bioaccumulation in various marine organisms of mercury (Hg) and cadmium (Cd) naturally occurring in Southern Ocean waters, or that of POPs in some long-lived seabirds with particular migration routes and life histories. However, despite the protection guidelines, long-range transport processes and especially the increase in human activities in Antarctica are sources of many persistent contaminants not yet subject to regulatory criteria and often lacking standardized sampling and analytical procedures. Chronic exposure to anthropogenic contaminants (legacy and of emerging interest) and pathogenic microorganisms near coastal scientific stations could cause synergistic or additive effects on marine biota. Most Antarctic marine organisms are endemic, with unique ecophysiological adaptations, and are also exposed to climate-related stressors. Warming and acidification of Southern Ocean waters along with increased melting of ice will likely affect the transport, pathways and environmental fate of persistent contaminants and could interfere with the metabolic processes of Antarctic organisms involved in the uptake and detoxification of environmental contaminants. Therefore, to implement environmental protection protocols around the coastal stations, the Council of Managers of National Antarctic Programs should evaluate the possible cumulative impact on biotic communities in the context of changing climatic and environmental conditions.
Environmental significance
Reports of new persistent synthetic chemicals, not yet subject to regulatory criteria, and plastic debris reaching Antarctica via long-range transport are continuously increasing. Scientific, tourism and fishing activities in Antarctica are also on the rise, inevitably contributing to localized environmental contamination and the introduction of alien species. The acidification of Southern Ocean waters and other climate-related stressors such as changes in sea ice cover and ocean freshening by glacial meltwater could reduce the resilience of Antarctic ecosystems, increasing the risk of biological effects of contaminants, especially in areas affected by human activities. This review discusses such possible cumulative impact. The most critical conditions and vulnerable species are also highlighted to guide future research and to implement protocols for human activity management and environmental monitoring particularly around science stations.
|
Introduction
Pesticides and other synthetic organic compounds, neither produced nor applied in Antarctica, have been detected in Antarctic animal tissue since the 1960s.1,2 In 1974, Molina and Rowland3 suggested that chlorofluoromethanes, used primarily in the Northern Hemisphere as refrigerants and aerosol propellants, could promote the destruction of stratospheric ozone through reactions catalyzed by chlorine atoms. Ten years later, Farman et al.4 discovered a marked decrease in stratospheric ozone concentrations during the austral spring over Halley station (75°36′45′′S 26°11′52′′W) and showed that this “ozone hole” had started forming several years earlier. These discoveries increased global concern about the possible effects of UV-B and UV-C radiation and formed the basis for the implementation of the Montreal Protocol, one of the most successful environmental policies of the twentieth century.5 Above all, it has emerged that, despite their remoteness and geographical isolation (increased by currents flowing clockwise around the continent, which is also surrounded by a cyclonic vortex of cold air), Antarctica and the Southern Ocean are linked to global environmental processes. However, although the Antarctic Treaty's Protocol on Environmental Protection (ATPEP; signed in Madrid in 1991 and entered into force in 1998) designated the region south of latitude 60°S as a “nature reserve, devoted to peace and science”, it has no purview over global issues. Therefore, the growing global population, the continuing exploitation of natural resources, generation of wastes and release of environmental contaminants, including persistent organic pollutants (POPs), pose increasing threats to the mostly undisturbed nature of the Antarctic environment.6–10 Despite some international agreements, such as the Minamata Convention to reduce the global Hg pollution and the Stockholm Convention to ban the production and use of some POPs, these compounds can persist in the environment for decades and, through repeated cycles of volatilization and condensation,11 will continue to move along gradients in air temperature until they reach their final sinks in the polar regions. Climate change may also promote the remobilization and bioavailability of POPs previously deposited in Antarctica, through melting ice and thawing of permafrost soils.10,12 Global chemical production continues to grow, along with concerns for the ever-increasing number of reports on the occurrence of many new persistent synthetic chemicals, not yet subjected to regulatory criteria (contaminants of emerging interest, CEI), and plastic debris in the Antarctic environment (e.g., ref. 13–18) (Fig. 1).
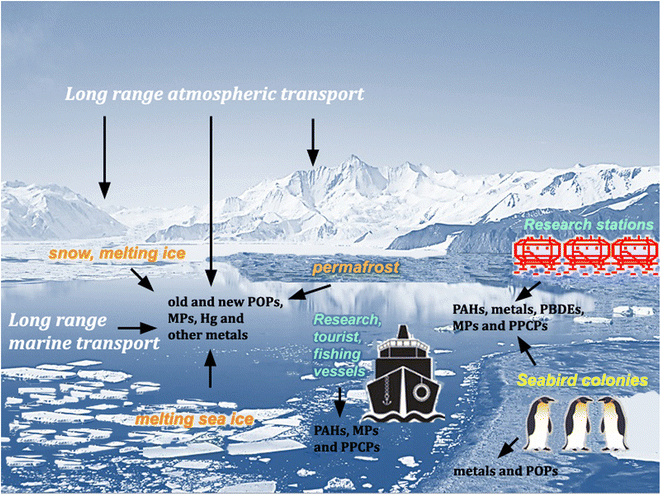 |
| Fig. 1 Main sources of persistent contaminants in Antarctic ecosystems. POPs, persistent organic pollutants; MPs, microplastics; PAHs, polycyclic aromatic hydrocarbons; PBDEs, polybrominated dyphenyl ethers; PPCPs, pharmaceuticals and personal care products. | |
South of 60°S, all human activities are regulated by ATPEP. However, the increase in scientific, tourism and fishing activities in Antarctica19 inevitably contributes to the introduction of alien species and localized environmental contamination through combustion of fuels, accidental oil spills, wastewater and waste production (e.g., 20,21). Until the 1990s, at several Antarctic stations, wastes were simply disposed of in landfills, burned in the open air or dumped into the sea. Therefore, although limited in space, the impact of past human activities in some coastal areas has influenced the diversity of biotic communities and the functioning of ecosystems.8,21,22 National organizations operating in Antarctica are mandated by ATPEP to prevent and monitor the impact of ongoing activities. However, many scientific stations and vessels lack wastewater treatment facilities. Where such facilities are present, the harsh Antarctic conditions often cause operational problems and malfunctions.23 Several Antarctic coastal ecosystems are affected by pathogenic microorganisms and contaminants, such as metals, hydrocarbons, detergents, flame retardants, personal care products, pharmaceuticals and microplastics. An improvement in environmental monitoring and control protocols around scientific stations is therefore urgently needed (e.g., ref. 14, 15, 24 and 25).
In sharp contrast to the very poor terrestrial biotic communities of the Antarctic cold desert, coastal marine ecosystems host dense and diverse populations of benthic organisms. Through long evolutionary processes in isolation, they have acquired unique ecophysiological adaptations. By storing lipids as an energy source, they are prone to the accumulation of lipophilic and potentially toxic compounds such as POPs.26–29 The involvement of benthic invertebrate communities in the transfer of energy and persistent pollutants to omnivorous fish and their predators lengthens the Antarctic food chain, thereby increasing the biomagnification of POPs and monomethyl mercury (MeHg)28,30,31 and Cd bioaccumulation.32–35 Thus, in some seabirds such as the south polar skua (Stercorarius maccormicki), concentrations of pollutants can reach levels close to those causing adverse health effects.36 Furthermore, the Southern Ocean is among the seas most vulnerable to increasing CO2 concentrations and water acidification.37 Marine organisms may also experience additional global warming stresses, such as changes in sea-ice cover and the freshening of seawater due to melting glaciers. Therefore, climate-related stressors could reduce the resilience of Antarctic organisms and ecosystems, increasing the risk of possible biological effects of anthropogenic contaminants, especially in coastal areas affected by human activities. With these topics in mind, after 15 (ref. 6) and 23 years38,39 from previous reviews, this review will update the knowledge on the occurrence of legacy and new anthropogenic contaminants in Antarctic ecosystems, and discuss the scientific evidence of their possible cumulative impact in the context of climate and environmental changes. Our aim is to summarize the most recent scientific acquisitions on the deposition of contaminants not in the Antarctic Plateau, nor throughout the continent, but above all in the ecosystems of the ice-free coastal areas and in the marine areas located near Antarctic stations, more impacted by scientific, logistical and tourism activities. The ecosystems of the Antarctic Peninsula and the surrounding archipelagos are the most exposed to such impacts. In the text, we refer to the “Scotia Arc” to indicate the island arc system (54.5 to 62°S, 65 to 25°W) located north of the Antarctic Peninsula and including, among others, South Georgia, the South Sandwich, South Orkney and South Shetland islands.
The most critical conditions and vulnerable species will also be highlighted to guide future research, and to implement protocols for the management of human activities and environmental monitoring around the scientific stations.
Trace metals
Wet and dry atmospheric deposition contribute to the biogeochemical cycle of major and trace elements in Antarctic terrestrial ecosystems. The snow elemental composition shows that these elements originate mainly from local rock and soil dust, sea-salt spray, active volcanoes such as Mount Erebus or Deception Island, and natural or anthropogenic sources in South America, South Africa and Australia.40–43 As a rule, past and ongoing human activities in Antarctica are associated with highly localized depositions of metals, such as lead (Pb), copper (Cu), arsenic (As), nickel (Ni) and zinc (Zn) around scientific stations (e.g., ref. 44). The temporal distribution of Pb concentrations in Antarctic snow is paradigmatic of the long-range atmospheric transport and deposition of metals from anthropogenic sources in the Southern Hemisphere. The increase in Pb concentrations began at the beginning of the 20th century with mining and smelting activities, and especially with the global use of gasolines with alkyl-Pb additives. Values peaked in the 1970s and declined in the 1990s, following the use of ethanol fuel blended with gasoline in Brazil and unleaded gasoline in Australia and other countries.45 In Antarctic snow samples collected in 2017, mean concentrations of Pb (4.4 pg L−1) were about half those recorded in 1970–1980 (9.0 pg L−1), and the contribution of metals from remote and local anthropogenic sources is probably currently lower than that from natural sources.46
Most Antarctic scientific stations are located in coastal ice-free areas, and historical and/or ongoing human activities have resulted in localized environmental contamination by metals21,34,47–49 (Table 1). Despite the low soil formation rate, terrestrial ecosystems in the vicinity of stations can harbor lichens, mosses, arthropods, nematodes, rotifers, tardigrades and very diverse communities of microorganisms.6 Moreover, coastal ice-free areas are essential breeding grounds for seabirds and seals. Penguins form large colonies, and transfer significant amounts of nutrients, heavy metals and POPs from the marine environment to terrestrial ecosystems through their excrements.50–52 For example, according to Chu et al.,53 the impact of metals from penguin guano on Ardley Island may exceed that of human activities near scientific stations. Through satellite digitization of visible disturbances around active and abandoned infrastructures, Brooks et al.54 estimated that more than 5000 km2 of Antarctic ice-free areas have been affected by human activities.
Table 1 Metal concentrations (μg g−1 dry wt; mean ± SD) in Antarctic surface soils sampled in control areas and near active or abandoned infrastructures. NA, not analyzed
Locations |
Cd |
Cu |
Hg |
Ni |
Pb |
Zn |
References |
Victoria Land
|
(Volcanic) |
0.13 ± 0.05 |
21 ± 8 |
0.04 ± 0.02 |
12 ± 9 |
8.8 ± 3.5 |
12 ± 9 |
38
|
(Granitic) |
0.22 ± 0.08 |
16 ± 9 |
0.05 ± 0.03 |
10 ± 6 |
11 ± 6 |
10 ± 6 |
Larsemann Hills
|
0.04 ± 0.08 |
17 ± 15 |
0.03 ± 0.08 |
14 ± 6 |
1.6 ± 1.1 |
32 ± 19 |
55
|
![[thin space (1/6-em)]](https://www.rsc.org/images/entities/char_2009.gif) |
Fildes Peninsula
|
Manned stations |
0.17 ± 0.08 |
122 ± 32 |
0.02 ± 0.01 |
14 ± 5 |
16 ± 13 |
59 ± 9 |
56
|
Baseline values |
0.09 |
89 |
0.01 |
10 |
5.4 |
51 |
Marambio Station
|
<0.8 |
15 ± 7 |
NA |
12 ± 4 |
44 ± 54 |
97 ± 103 |
47
|
Control sites |
<0.8 |
8.2 ± 3.0 |
NA |
11 ± 8 |
10 ± 0.1 |
41 ± 8 |
O'Higgins Base (near penguin col.) |
4.3 ± 1.5 |
422 ± 151 |
NA |
28 ± 10 |
28 ± 87 |
485 ± 182 |
57
|
![[thin space (1/6-em)]](https://www.rsc.org/images/entities/char_2009.gif) |
Robert Island
|
(Refuge) |
<0.2 |
107 ± 1 |
NA |
38 ± 0.5 |
102 ± 2 |
148 ± 5 |
48
|
Control soil |
<0.2 |
48 ± 0.4 |
NA |
40 ± 0.5 |
7.3 ± 0.5 |
44 ± 0.2 |
Several species of lichens and mosses have a wide geographic distribution in Antarctic ice-free areas and large-scale surveys, or those performed around scientific stations, demonstrate their reliability as biomonitors of atmospheric deposition of metals and other persistent contaminants (e.g., ref. 30 and 58–61). As shown in Table 2, baseline concentrations of metals in widespread Antarctic cryptogam species are in the same range as those measured in other lichen and moss species collected in reference areas of the Southern and Northern Hemispheres, prepared and analyzed with the same procedures.
Table 2 Metal concentrations (μg g−1 dry wt; mean ± SD) in macrolichen (L) and moss (M) species from East Antarctica and other relatively uncontaminated areas in the Southern and Northern Hemispheres. NA, not analyzed
Locations/species |
Cd |
Cu |
Hg |
Ni |
Pb |
Zn |
References |
Victoria Land
|
L Umbilicaria decussata |
0.20 ± 0.10 |
4.8 ± 2.6 |
0.39 ± 0.27 |
NA |
0.50 ± 0.33 |
19 ± 4 |
39
|
M Bryum pseudotriquetrum |
0.21 ± 0.12 |
9.9 ± 3.3 |
0.14 ± 0.06 |
4.4 ± 2.1 |
2.2 ± 1.2 |
63 ± 20 |
![[thin space (1/6-em)]](https://www.rsc.org/images/entities/char_2009.gif) |
Chilean Patagonia
|
L Nephroma antarcticum |
0.15 ± 0.08 |
5.6 ± 1 |
0.07 ± 0.02 |
3.3 ± 1.9 |
0.74 ± 0.38 |
40 ± 13 |
62
|
L Usnea sp. |
0.08 ± 0.03 |
1.5 ± 0.3 |
0.14 ± 0.04 |
0.86 ± 0.36 |
0.49 ± 0.29 |
27 ± 9 |
![[thin space (1/6-em)]](https://www.rsc.org/images/entities/char_2009.gif) |
Italian Alps/Apennines
|
L Pseudevernia furfuracea |
0.18 ± 0.09 |
5.4 ± 2.1 |
0.20 ± 0.06 |
1.7 ± 0.9 |
4.5 ± 2.9 |
41 ± 17 |
63
|
![[thin space (1/6-em)]](https://www.rsc.org/images/entities/char_2009.gif) |
North-Eastern Italy
|
L Pseudevernia furfuracea |
0.21 ± 0.03 |
6.0 ± 0.5 |
0.17 ± 0.02 |
2.9 ± 1.7 |
5.4 ± 0.9 |
53 ± 5 |
64
|
M Hypnum cupressiforme |
0.23 ± 0.02 |
5.7 ± 0.3 |
0.13 ± 0.01 |
2.7 ± 1.2 |
3.4 ± 0.9 |
41 ± 11 |
![[thin space (1/6-em)]](https://www.rsc.org/images/entities/char_2009.gif) |
Tuscany (Italy)
|
L Flavoparmelia caperata |
0.26 ± 0.11 |
5.8 ± 1.3 |
0.17 ± 0.08 |
2.7 ± 1.9 |
3.9 ± 2.5 |
35 ± 7 |
65
|
M Hypnum cupressiforme |
0.19 ± 0.06 |
6.1 ± 1.6 |
0.12 ± 0.04 |
4.8 ± 3.1 |
2.7 ± 1.7 |
26 ± 9 |
Unlike other metals emitted from natural and anthropogenic sources, which are associated with atmospheric aerosol, mercury (Hg) is mainly present in the atmosphere as gaseous Hg0, with a lifetime of about one year.66 Once deposited in terrestrial and aquatic ecosystems, inorganic Hg can be transformed by microorganisms into MeHg, a potent neurotoxin with a remarkably long biological half-life (some months instead of a few days or weeks for inorganic Hg). Thus, through a process of biomagnification, MeHg accumulates in primary producers and its concentrations increase in the muscle and other tissues of organisms along the food webs, reaching peak concentrations in long-lived species at higher trophic levels. Aquatic food webs are much more complex and longer than those of terrestrial ecosystems, and seabirds and marine mammals accumulate much more MeHg than their terrestrial counterparts. Although there appears to be no greater bioavailability of MeHg in the Southern Ocean than in other seas, its concentrations in seabirds such as the long-lived wandering albatross, Diomedea exulans, are probably among the highest ever reported for marine vertebrates (e.g., ref. 67 and 68). Analysis of contemporary and historical albatross feather specimens shows only a slight increase in Hg concentrations,69,70 suggesting that metal accumulation is primarily a natural process due to species-specific life histories, metabolic pathways, and the unique trophic web of the Southern Ocean. The wandering albatross feeds atop trophic webs and also frequents temperate waters (probably with higher MeHg bioavailability). Furthermore, molting and egg laying are important mechanisms for Hg excretion in seabirds. D. exulans replaces feathers over a period of years rather than annually, and has a low reproductive rate (one egg every two years). Seabirds and mammals can demethylate MeHg in the liver and accumulate selenium, an antagonist to Hg toxicity.71 Hence, they are probably adapted to metabolize and tolerate MeHg intake without deleterious biological effects.6,72
Although there are very few local sources of Hg in Antarctica, a biomonitoring survey performed in the summers 1989/90 and 1990/91 with the lichen Umbilicaria decussata across Victoria Land showed total concentrations of the metal in samples from ice-free areas in the Nansen Ice Sheet to be in the same range or higher than those measured in lichens of the same genus from contaminated sites in Europe.58 In the Nansen Ice Sheet, there were no human, volcanic, or geothermal activities, and mean atmospheric Hg0 in the Southern Hemisphere is lower than in the Northern Hemisphere. Therefore, there was no satisfactory explanation for the unexpected and localized Hg bioaccumulation. In the following years, it was demonstrated that in the coastal Arctic ecosystems facing ice-free marine areas in winter, the spring sunrise promotes photochemical reactions with the activation of bromine compounds which oxidize atmospheric Hg0, causing its deposition (so-called “Hg depletion events”;73). In winter, the sea in front of the Nansen Ice Sheet is free of ice. This is due to the recurring formation of the Terra Nova Bay coastal polynya. In spring, the depletion of Hg determines its accumulation in mosses, lichens, soils, lacustrine sediments and algal mats.74 Interestingly, a recent survey of surface soils collected in Antarctic ice-free areas near scientific stations, penguin rookeries, coastal polynyas, and in control ice-free areas shows that Hg accumulation in soils is mainly dependent on their organic carbon content. In all the samples with an accentuated metal deposition, the value of the ratio [Hg ng g−1]/[OC%] is usually >11.75
Emissions of Hg0 are increasing in some Southern Hemisphere countries76 and global warming will likely reduce the sea-ice cover in some Antarctic coastal areas; thus, concern is growing about Antarctica's potential role as the ultimate sink of Hg. Furthermore, studies on the metal cycling between air and snow in the Antarctic Plateau77,78 and the Hg accumulation pattern in cryptogams collected along the Reeves Glacier79 indicate that coastal ecosystems also receive Hg0via katabatic winds (i.e., the cold and dense air masses sliding down-slope from the Antarctic Plateau towards the sea).
Since the earliest investigations on the elemental composition of marine organisms in the Southern Ocean, it was unexpectedly found that they can accumulate much higher Cd concentrations than those of related species from seas more impacted by anthropogenic activities.32,80,81 In the highly productive waters of the Southern Ocean, the low input of trace elements such as Zn from the icy continent can limit the development of phytoplankton. Therefore, Cd is actively taken up by diatoms as a substitute for Zn to synthesize carbonic anhydrase (a metalloenzyme that supplies carbon for photosynthesis) (e.g., ref. 82). From primary producers, the metal is transferred to consumers and accumulates in their kidneys and liver (or in the digestive gland of invertebrates). In general, the Cd concentrations in these organs do not increase with age and, depending on diet, may be higher in juveniles than in adults of the same species. Like Hg bioaccumulation, Cd bioaccumulation appears to be a natural process. During their evolution in the Southern Ocean, Antarctic marine organisms likely acquired metabolic pathways to regulate and tolerate Cd accumulation.7
Like mosses and lichens, penguins have a circumpolar distribution. Due to their longevity (about 20 years) and tendency to return to their annual breeding sites, they are valuable bioindicators of temporal changes in the bioavailability of contaminants in the different foraging areas.83–85 Comparison of the mean metal concentrations in penguin feathers from different Antarctic regions (Table 3) mainly shows higher bioavailability of Pb for colonies living in the Antarctic Peninsula and the Scotia Arc archipelagos, and higher bioaccumulation of Hg in feathers of emperor penguin from the Ross Sea. However, the latter penguin feeds at higher trophic levels than the other species and feather samples were collected in an area that may be affected by Hg emissions from the Mount Erebus volcano.85 When comparing the elemental composition of penguin feathers, it should be noted that even for samples collected in the same breeding areas, the results are influenced not only by interspecies differences (e.g., foraging habits, diet, migrations, molting pattern and detoxification mechanisms), but also by sex and stage of maturity.86 Furthermore, Sun et al.87 showed that feather sampling is itself a critical step since samples taken from different parts of the penguin body contained different concentrations of metals. By applying a standardized sampling protocol, they found higher As concentrations in the feathers of the Adélie penguin (range: from 0.62 to 2.95 μg g−1 dry wt) than in those of seabirds from other seas (max 0.07 μg g−1 dry wt), and higher concentrations of Cd, Cu, Hg, Ni, and Zn in samples from the Ross Sea than in those from the Antarctic Peninsula.
Table 3 Metal concentrations (μg g−1 dry wt; mean ± SD) in feathers of penguins from different Antarctic areas. NA, not analyzed
Locations/species |
Cd |
Cu |
Hg |
Ni |
Pb |
Zn |
References |
King George Island
|
Pygoscelis antarcticus
|
0.21 ± 0.14 |
26 ± 5 |
NA |
0.28 ± 0.15 |
NA |
89 ± 20 |
86
|
Pygoscelis adeliae
|
0.14 ± 0.06 |
17 ± 2 |
NA |
0.24 ± 0.08 |
NA |
71 ± 11 |
Pygoscelis papua
|
0.14 ± 0.07 |
18 ± 6 |
NA |
0.27 ± 0.12 |
NA |
80 ± 28 |
P. papua (chicks) |
0.06 ± 0.07 |
7 ± 2 |
NA |
0.01 ± 0.01 |
0.87 ± 0.86 |
81 ± 11 |
83
|
P. adeliae (chicks) |
0.13 ± 0.08 |
13 ± 8 |
NA |
0.05 ± 0.03 |
0.24 ± 0.83 |
61 ± 20 |
![[thin space (1/6-em)]](https://www.rsc.org/images/entities/char_2009.gif) |
Livingston Island
|
P. antarcticus
|
0.14 ± 0.11 |
29 ± 6 |
NA |
0.16 ± 0.07 |
NA |
117 ± 32 |
86
|
P. papua
|
0.14 ± 0.07 |
18 ± 2 |
NA |
0.20 ± 0.05 |
NA |
103 ± 11 |
P. antarcticus
|
NA |
NA |
0.67 ± 0.46 |
NA |
NA |
NA |
35
|
P. papua
|
NA |
NA |
0.22 ± 0.09 |
NA |
NA |
NA |
![[thin space (1/6-em)]](https://www.rsc.org/images/entities/char_2009.gif) |
South Georgia
|
P. papua
|
NA |
NA |
0.85 ± 0.88 |
NA |
NA |
NA |
84
|
![[thin space (1/6-em)]](https://www.rsc.org/images/entities/char_2009.gif) |
Antarctic Peninsula
|
P. antarcticus
|
NA |
NA |
0.62 ± 0.30 |
NA |
NA |
NA |
57
|
P. adeliae
|
NA |
NA |
0.35 ± 0.09 |
NA |
NA |
NA |
P. papua
|
NA |
NA |
0.31 ± 0.10 |
NA |
NA |
NA |
Neko Harbor (P. papua) |
0.05 ± 0.07 |
14 ± 2 |
NA |
1.6 ± 1.6 |
0.06 ± 0.06 |
37 ± 6 |
Doumer Island (P. papua) |
0.09 ± 0.07 |
15 ± 4 |
NA |
1.0 ± 1.2 |
0.10 ± 0.17 |
33 ± 4 |
Stranger Point (P. papua) |
0.14 ± 0.09 |
20 ± 2 |
NA |
3.8 ± 2.4 |
0.60 ± 0.34 |
64 ± 11 |
O'Higgins Base (P. papua) |
0.21 ± 0.28 |
21 ± 4 |
NA |
5.9 ± 8.2 |
0.63 ± 0.27 |
64 ± 11 |
![[thin space (1/6-em)]](https://www.rsc.org/images/entities/char_2009.gif) |
East Antarctica
|
Cape Bird (P. adeliae) |
0.11 ± 0.01 |
19 ± 0.3 |
0.59 ± 0.02 |
NA |
0.09 ± 0.02 |
75 ± 1 |
85
|
Cape Crozier (Aptenodytes forsteri) |
0.04 ± 0.02 |
14 ± 1 |
1.35 ± 0.06 |
NA |
0.05 ± 0.06 |
68 ± 3 |
Legacy and new persistent organic pollutants
The first synthetic pesticide (dichloro-diphenyl-thrichloroethane, DDT) was produced in the 1940s. Due to its effectiveness in combating insect vectors of human diseases, it was later also extensively used against species harmful to crops and livestock production. In 1962, with the book “Silent Spring”, Rachel Carson88 warned against the biological effects of pesticides. A few years later, Sladen et al.1 found DDT in Antarctic penguins and seals. The discovery raised the question of how chemicals never used in Antarctica were able to reach such a remote region. As later confirmed by Wania and Mackay,11 since the first reports on the occurrence of pesticides in Antarctica, it was hypothesized that semi-volatile organic compounds could enter the atmosphere in warmer regions, and be transported by air masses towards the poles to then condense and settle. Shortly thereafter, other organochlorine pesticides, polychlorinated biphenyls (PCBs), polybrominated diphenyl ethers (PBDEs) and other POPs, mostly produced and used in the Northern Hemisphere, were detected in the Antarctic environment (e.g., ref. 2 and 89–94).
Historically, the most frequent causes of environmental contamination in Antarctica have been oil spills during vehicle/aircraft refueling and spills caused by shipwrecks, collisions or accidents when transporting bunker fuel. Indeed, high concentrations of polycyclic aromatic hydrocarbons (PAHs) have been detected near some scientific stations.95–97 At Arthur Harbor, the sinking of the Argentine supply ship Bahia Paraiso on 28 January 1989 released approximately 600 m3 of diesel fuel. Because it was the middle to end of the seabird breeding season, thousands of seabirds were affected and some hundreds died.98 Small amounts of oil on penguin plumage can cause waterlogging, reduced buoyancy and reduced thermal insulation. Furthermore, PAHs ingestion can cause histopathological changes in the liver, kidney and intestine, impaired osmoregulation and reduced fertility.28,99,100
In Antarctic terrestrial ecosystems, contamination by aliphatic and aromatic compounds reduces soil microbial diversity and promotes the development of hydrocarbon-degrading bacteria.101 However, due to the extreme climatic and environmental conditions, the biodegradation of hydrocarbons proceeds very slowly. In marine sediments, a field experiment was performed that aimed at investigating the biological effects of various hydrocarbon products on the benthic macrofauna. The study showed that diesel fuel had the greatest initial impact, especially on annelids. However, after 5 years, the communities treated with diesel fuel seemed to recover faster than those treated with lubricating oils.29
Being largely due to local anthropogenic activities, rather than long-range atmospheric transport or local natural sources, contamination by PAHs in Antarctic marine sediments and soils is significantly higher near scientific stations than in reference areas (Table 4).
Table 4 Concentrations of ΣPAHs (mean ± SD or range, ng g−1 dry wt) in Antarctic marine sediments and soils differently affected by anthropogenic activities
Locations |
Matrix |
n of PAH species |
ΣPAHs |
References |
McMurdo Station (outfall) |
Coastal sediments |
16 |
779 ± 590 |
102
|
Winter Quarter Bay |
1095 ± 1082 |
Cape Hermitage (control) |
36 ± 37 |
Turtle Rock (control) |
5.7 ± 3.6 |
Prydz Bay |
Sediments (up to >1000 m depths) |
15 |
18 ± 6 (13–31) |
103
|
Anvers Is., Palmer Station (outfall) |
Sediments (18–24 m depth) |
16 |
32–302 |
104
|
Reference area |
6–30 |
Potter Cove, Carlini Station |
Surface sediment (20–30 m) |
21 |
18–146 |
105
|
South Fildes Pen. (some stations) |
19–143 |
Reference areas |
4.6–16.6 |
King George Island, Arctowski St. |
Coastal sediments |
16 |
56–445 |
106
|
King George Island |
Soil |
15 |
1.8–32.9 |
107
|
Fildes Peninsula |
Soil |
15 |
61 (mean 2013–2019) |
108
|
Bioaccumulation of PAHs has been reported in many species of marine organisms from contaminated coastal sites, but concentrations do not appear to increase along food webs.109 Palmer et al.,102,104 for example, measured higher levels in benthic invertebrate species, such as the soft coral Alcyonium antarcticum or the limpet Nacella concinna, than in their predators.
Other POPs listed under the Stockholm Convention, such as organochlorine pesticides, PCBs and PBDEs, have been reported in the Antarctic environment. Analyzing air and snow samples from the western Antarctic Peninsula, Khairy et al.110 found the highest concentrations of these compounds in snow (suggesting the melting of ice and snow as a possible secondary source), while the vapor phases of hexachlorobenzene (HCB), PCBs, heptachlor and PBDEs prevailed in air. It is also probable that these latter compounds had originated from local sources. Although ATPEP bans the import and release of POPs in Antarctica, organohalogen compounds have been used as flame retardants in a range of household items, plastics, electronics, and materials used in the construction and/or renovation of scientific stations. Bengtson Nash et al.111 found atmospheric PBDEs contamination around two stations. During the building of the Troll Station, they showed a marked increase in the concentrations of their penta-formulation congeners. As early as 2008, Hale et al.112 had detected PBDEs in the indoor dust and sewage sludge of McMurdo Station and Scott Base. Their concentrations in sediments, fish and invertebrates from McMurdo Sound were in the same range as those measured in samples from urbanized North American areas. By analyzing legacy POPs in key species of the Ross Sea food web, such as Euphausia superba and Pleuragramma antarctica and their predators, Corsolini et al.113 found the highest concentrations of PBDEs in Adélie penguins and skuas nesting near a long-term field camp. Markham et al.114 detected PBDEs in all samples of phytoplankton, krill, fish and fur seal milk collected over a 14 year period. Moreover, between 2000 and 2014, the values increased in samples of fur seal milk, phytoplankton and zooplankton.
Most POPs reach Antarctica and the Southern Ocean through long-range transport processes, and Antarctic organisms are deemed to be reliable biomonitors of global POP emissions. As a rule, the concentrations of these contaminants in their tissues are lower than in related species from lower latitudes, and are usually much lower than those that would cause adverse health effects.115 However, upon returning to Antarctica, opportunistic predators/scavengers (such as the south polar skua that migrate to the more polluted Northern Hemisphere during the austral winter) contribute to the contamination of terrestrial environments through their guano, abandoned eggs, and dead individuals.116
Following the entry into force of global conventions such as the Long-range Transboundary Air Pollution117 and the Stockholm Convention,118 legacy POPs have gradually been banned worldwide. However, there are few long-term surveys on POPs concentrations in the Antarctic environment. Furthermore, the assessment of bioaccumulation temporal trends in the different study areas is made difficult by latitudinal variations in climatic and environmental conditions, and by differences in feeding and migratory behavior of organisms. Corsolini,119 for example, highlighted the different temporal trends in PCB accumulation in a variety of marine species from West and East Antarctica. van den Brink et al.120 found decreasing trends in PCBs, HCB and dieldrin levels in Adélie penguins, southern fulmars and pelagic fish from 1993–1994 to 2003–2004, even though their concentrations were steady or increasing in benthic organisms; thus, they questioned whether the POPs contamination in Antarctica is actually declining. Isla et al.121 detected cytotoxic activity in extracts of marine sediments collected along thousands of kilometers of the Antarctic continental shelf and up to 1200 m depth. However, some more recent works would seem to indicate a decreasing trend of long-range transport and bioavailability of POPs in the Antarctic environment. Hao et al.122 monitored the atmospheric concentrations of POPs in the Fildes Peninsula (King George Island) from 2010 to 2018. With the exception of HCB, they found low and declining levels of PCBs, HCHs, DDTs, and endosulfans. Summarizing the literature data on POP concentrations in penguin fat and eggs from 1964 to 2011, Ellis et al.123 showed a decrease in the concentrations of DDTs, HCB and HCHs (hexachlorocyclohexane). In embryos from failed eggs of Wilson's storm-petrel collected on King George Island from 1998 to 2003 and 2014 to 2016, Kuepper et al.124 found that concentrations of legacy pollutants were higher in 1998, 2001 and 2003, than in 2014–2016. A decrease in the bioaccumulation of HCB, HCHs, PCDDs (polychlorinated dibenzodioxins) and PCDF (polychlorinated dibenzofurans) was also reported by Cincinelli et al.31 in the Antarctic fish Trematomus bernacchii over a 30 year period (early 1980s to 2010).
Among the new POPs listed in the Stockholm Convention are a few poly- and perfluoroalkyl substances (PFAS): perfluorooctane sulfonate (PFOS), perfluorooctanoic acid (PFOA), perfluorohexane sulfonate (PFHxS) and related compounds. Some of these proteinophilic compounds have been detected in Antarctic snow and waters,125,126 seabirds and marine mammals.127,128 In general, PFAS concentrations in Antarctica are lower than those reported for the Arctic or other remote regions. To assess possible trophic transfer along food webs, Gao et al.129 analyzed sediments, algae, molluscs, fish, petrel and penguin feathers from King George and Ardley Islands. They found a unique PFAS profile that suggests long-range transport as the prevailing source. Furthermore, perfluorobutyric acid (PFBA) was predominant in all Antarctic organisms. No biomagnification was detected for the short-chain PFAS, such as C4–C7 perfluorocarboxylic (PFCA) and perfluorobutanesulfonic acids (PFBS), but trophic magnification factors of 2.09 and 2.92 were calculated for C-8 PFHxS and PFOS, respectively.
Pharmaceuticals and personal care products
In Antarctica, there are more than 80 summer and year-round research stations, most of which are located in ice-free coastal areas and discharge wastewater into the marine environment. Several stations do not have wastewater treatment plants, and where they are installed, the wastewater treatment plants often experience operational problems mainly due to the extreme climatic and environmental conditions and the great variability in station attendance.23 Therefore, scientific and logistics personnel together with tourists are sources of alien microorganisms and many synthetic chemicals: pharmaceuticals and personal care products (PPCPs), micro- and nanoplastics. Also, due to the dry atmosphere and high levels of UV-B radiation, people operating in Antarctica make heavy use of sunscreens and moisturizers, and there is widespread consumption of personal hygiene products in order to prevent the spread of diseases. While many of these chemicals are not yet subject to regulatory criteria, they are increasingly being recognized as ubiquitous contaminants. Many PPCPs released into the environment usually undergo photochemical and biological degradation.130 However, in Antarctic coastal ecosystems, their persistence is increased by seasonal formation of sea-ice, polar night and reduced metabolic rate of microorganisms in the cold waters of the Southern Ocean.
In the blubber of Antarctic fur seals collected in January–February 2004 on Livingston Island (Antarctic Peninsula), Schiavone et al.131 found polycyclic musks in addition to pesticides, PCBs, PBDEs and polychlorinated naphthalenes (PCNs). These synthetic fragrances are used extensively in skin cream, soaps, detergents and deodorants, and can have anti-estrogenic effects. A few years ago, these compounds were also detected in East Antarctica in coastal waters collected near the Italian Station “Mario Zucchelli”.132 In the same region, near McMurdo and Scott Base stations, Emnet et al.133 found several personal care products and steroid hormones in samples of seawater, sea-ice and marine organisms. PPCP concentrations in seawater and sea-ice were in similar ranges, and many compounds were detected up to 25 km away from wastewater discharges.
The north of the Antarctic Peninsula is the Antarctic region most affected by research, tourism and fishing activities, and the human influence is also emphasized by the presence of a number of PPCPs. Gonzáles-Alonso et al.134 analyzed 25 selected pharmaceuticals and 21 recreational (licit or illicit) drugs in water samples from streams, ponds, glacier drains, and a wastewater discharge near Marambio Station. The highest concentrations were found in wastewaters, and analgesic and anti-inflammatory residues (acetaminophen, diclofenac and ibuprofen) were the most abundant pharmaceuticals, while caffeine and ephedrine were the most widespread recreational drugs. In water samples from Seymour/Marambio Island, King George Island and Deception Island, Olalla et al.15 analyzed 54 substances belonging to drugs/medicines of abuse, endocrine disruptors, pyrethroids, perfluorinated compounds and sunscreens. By calculating the ratio of measured environmental concentrations of PPCPs to their predicted no-effect concentration, they found higher environmental risks for residues of acetaminophen, diclofenac, ibuprofen, the antibiotic clarithromycin, nonylphenol diethoxylate and long-chain PFASs. In untreated wastewater from Arctowski Station (Admiralty Bay, King George Island), among more than 170 substances analyzed, Szopińska et al.18 identified 34 PPCPs and other emerging contaminants. The highest environmental risk was calculated for residues of ketoconazole (azole antifungal), diclofenac, ibuprofen and caffeine. In wastewater and sea water, they also detected antibiotic and sulphonamide resistance genes. By analyzing a sample of phytoplankton from Port Foster Bay (Deception Island) by high-resolution Fourier-transform ion cyclotron-resonance mass spectrometry (FT-ICR-MS), Duarte et al.24 found residues of 5 personal care products and 40 pharmaceuticals, including anticonvulsants, antihypertensives and beta-blockers, antibiotics, analgesics and anti-inflammatories.
Numerous multi-antibiotic resistant bacterial strains have been found in Antarctic ecosystems (e.g., ref. 135). Antibiotic resistance genes can emerge naturally in microorganisms, especially in the extreme conditions of the Antarctic environment where bacteria can compete for nutrients.136 However, Jara et al.137 analyzed the antibiotic-resistance patterns and antibiotic-resistance genes in strains of bacteria from freshwater samples collected in human-affected and control areas in the Fildes Peninsula. They found that isolates from the impacted sites had a greater richness and diversity of antibiotic-resistance genes, and were resistant to synthetic and semi-synthetic drugs, while those from relatively undisturbed sites were highly susceptible to antibiotics. Therefore, there is growing concern about the impact of wastewater from scientific stations, which can modify the native resistome of microbial communities, and can also introduce antibiotic resistance gene carriers such as transposons, integrons and conjugative plasmids into the environment.18
Microplastics
Plastic debris has become one the most common and persistent synthetic wastes in the global environment. Microplastics (MPs, size 1–5000 μm), produced deliberately or by the fragmentation of larger debris, are among the most enduring evidence of past and recent human activities even in Antarctica.17 In regions with the strongest human footprint, such as the Northern Antarctic Peninsula and the Scotia Arc, entanglement of seals in plastic waste or plastic ingestion by seabirds have been reported for forty years.138,139 However, only in recent years, after the environmental impact of MPs had assumed global significance, have numerous investigations been conducted on the presence of MPs in the Antarctic environment. Most of the studies focused on coastal marine ecosystems with higher human pressure, and only plastic fragments >300 μm were often considered. Thus, the distribution pattern of MPs in the Southern Ocean, their background concentrations and potential impact on pelagic communities are currently unknown. Furthermore, it is very difficult to compare the available data and evaluate their reliability because of the differences in sampling and analytical methods among the different studies, and the lack of standardized protocols for assessing the contamination of samples in the field and in the laboratory. Further difficulties in assessing the potential impact of MPs arise from the spatio-temporal variations of the Southern Ocean environmental characteristics, such as seasonal changes in sea-ice cover and exposure to UV-radiation. Depending on the polymer composition, these factors can contribute to the fragmentation and degradation of plastic wastes and influence their environmental fate.
Antarctic marine organisms include many endemic species with unique ecophysiological adaptations to the Southern Ocean. Without better knowledge of the spread of the tiniest plastic particles (nanoplastics, size < 1 μm) that are potentially more dangerous, it seems impossible to tell what impact these organisms will suffer. Although the Southern Ocean also probably receives plastic particles from lower latitudes through long-range marine and atmospheric transport,140 their concentrations in open ocean waters appear to be very low. By sampling floating MPs (>100 μm) in subsurface waters from the Arctic to the Scotia Sea, Pakhomova et al.141 found significantly lower concentrations in the Southern Hemisphere than in the Northern. In surface and subsurface waters of the Weddell Sea gyre, Leistenschneider et al.142 measured MP concentrations of 0.01 ± 0.01 m−3 and 0.04 ± 0.1 m−3, respectively. In addition, they found that about half of the particles sampled (>300 μm) originated from paints of the research vessel. In a circum-Antarctic survey, Kuklinski et al.143 collected fibers that appeared to be plastic, but analysis via Fourier-transform infrared (FT-IR) spectroscopy showed that they were composed of silica (i.e., likely of biogenic origin). By contrast, several surveys have reported relatively high concentrations of MPs in seawaters, sediments and organisms in coastal ecosystems of the northern Antarctic Peninsula, South Georgia Island, and the Ross Sea, especially those directly affected by wastewater from scientific stations and/or rather intensive tourism and fishing activities (e.g., ref. 16, 144 and 145). In general, fibers from washing clothes are among the most common MPs. In some coastal waters and sediments, their concentrations are in the same range as those reported in coastal environments outside Antarctica.
The Ross Sea is one of the largest marine protected areas in the world. Near the Italian scientific station “Mario Zucchelli”, synthetic fibers have been found in zoobenthic species146 and in the fish Trematomus bernacchii.147 Other scientific stations along the Ross Sea coasts such as McMurdo and Scott Base have been in operation for several decades. Zhang et al.148 found more MPs in fish from the Ross Sea than in those from the nearby Amundsen Sea, where there are no scientific stations. The presence of MPs has also been reported in penguin scats, particularly in samples collected in the Antarctic Peninsula and the Scotia Sea.149–151 Although these studies seem to suggest trophic transfer and possible biomagnification of MPs along food webs, Sfriso et al.146 found 3–5 times higher concentrations in filter-feeder bivalves and benthic grazers than in omnivorous or predatory species of Antarctic invertebrates. Furthermore, Leistenschneider et al.152 found that none of the 85 particles ingested by chicks of the emperor penguin (Aptenodytes forsteri, the only penguin species breeding around Antarctica during the austral winter) collected in Queen Maud Land were MPs, and the synthetic fibers found were due to contamination during the analysis and processing of the samples. Also, Garcia-Garin et al.153 found that the fibers and fragments found in scats of male Antarctic fur seals (Arctocephalus gazella) collected at Deception Island (a hotspot of tourism and scientific activities in Antarctica) were silicate minerals and chitin fibers, and not MPs. Recently, the absence of MP particles (>80 μm) has also been reported in the stomachs of 10 ringed seals from the western Canadian Arctic.154
Possible cumulative impacts of climate changes and environmental contaminants
With the exception of some local impacts in the vicinity of the scientific stations, marine and terrestrial ecosystems in continental Antarctica are scarcely affected by anthropogenic contaminants or by macroscopic climatic and environmental changes so far. By contrast, in the Antarctic Peninsula and the islands of the Scotia Arc, the larger and growing scientific, tourist and fishing activities constitute important local sources of metals, PAHs, PBDE, PCBs and PCPPs.155 Rapid warming has led to the thinning and disintegration of ice shelves, the retreat and melting of ice sheets and the thawing of permafrost soils. The expansion of ice-free areas, together with the increase in liquid water availability, has promoted soil-formation processes and changes in terrestrial biotic communities.156 Marine ecosystems harbour many endemic species, which through long evolutionary processes in isolation, have adapted to the cold waters of the Southern Ocean (−1.8 °C). Compared to related species in other seas, these organisms tolerate much smaller temperature increases, which could impair their metabolism and ability to detoxify and eliminate environmental contaminants.
The role of climate change in shaping the responses of Antarctic marine organisms to environmental pollutants has recently been highlighted in coastal benthic communities at McMurdo Station.94,157 Until the mid-1980s, the waste produced at the station was disposed of in snow pits, by open incineration or dumped directly into sea. In 1988, the US National Science Foundation initiated a dumpsite cleanup and long-term environmental monitoring.49 Although the concentrations of most pollutants in sediments and marine organisms have progressively decreased to values typical of nearly pristine environments, Palmer et al.102,157 found no signs of recovery in macrobenthic communities. Moreover, they found that temporal changes in species composition correlated with the Interdecadal Pacific Oscillation climatic index, and were also influenced by the Antarctic Oscillation climatic index and maximum sea-ice extent rather than by decreases in environmental pollution.
About 15 million km2 of sea-ice grows around Antarctica each year, and its summer melt promotes blooms of sympagic and pelagic communities at the ice edges. Sea ice intercepts and accumulates persistent contaminants from atmospheric deposition and seawater, and likely contributes to their uptake by sea-ice algae and other organisms in cryopelagic communities. These communities play a crucial role in Southern Ocean productivity, and ice-algae are critical for the wintering of juvenile Antarctic krill (Euphausia superba, age class 0), the keystone species of Antarctic food webs.158 However, after a few years of record highs, the sea-ice cover of the Southern Ocean has begun to exhibit a rapid decline since 2016 (the most pronounced since the beginning of 40 years of satellite observations;159). This decrease is likely due to progressive ocean warming and southward advection of atmospheric heat that adversely affects ocean productivity, and may also contribute to the transport of POPs and MPs from lower latitudes to the Antarctic environment.
Seawater acidification could be another climate-related stressor affecting the resilience of Antarctic marine organisms and increasing their sensitivity to anthropogenic contaminants.37 In seawater, aragonite saturation is essential for calcifying organisms. Negrete-García et al.160 estimated that the Southern Ocean could experience aragonite undersaturation by 2050. Preliminary laboratory experiments by Ericson et al.161 indicate that adult E. superba specimens are resilient to one-year exposure to near-future levels of ocean acidification (1000–2000 μatm ρCO2). However, other experiments showed inhibition of embryonic development of this key species at simulated concentrations of 2000 μatm ρCO2.162 Furthermore, Morley et al.163 have emphasized the sensitivity of E. superba to increasing water temperatures and sea-ice loss.
Any climatic and environmental stress can affect the biological thresholds of organisms for other stressors, such as environmental contaminants. Although no field data from Antarctic coastal ecosystems exist yet, some ecotoxicological experiments show impaired larval development or survival of Antarctic marine species exposed to the combined effects of water acidification and nanoplastics.164,165 Additional cumulative stresses and threats to Antarctic organisms may arise from alien species and pathogenic microorganisms accidently introduced by the growing number of scientists and tourists. Shimada et al.166 found strains of Legionella spp., Pseudomonas spp., and Mycobacterium spp. in water facilities of the Syowa station and in waters collected in the surrounding glacial lakes. Again, climate change could play an important role in enabling the colonization and spread of pre-adapted and invasive alien species.
A wide range of microorganisms, microalgae and some invertebrate species have been found on the surface of plastic debris collected in the Antarctic Peninsula, and strains of bacteria with multiple antibiotic resistance have been isolated from a polystyrene fragment beached on King George Island.167 Wastewater from scientific and tourist stations and vessels are also sources of PPCPs. Furthermore, the laundering of technical clothing releases plastic fibers, water repellents and flame-retardant chemicals. In the marine environment, plastic debris can adsorb and accumulate metals and hydrophobic organic contaminants in much higher concentrations than in the surrounding seawater.168 Lu et al.169 found that MPs affect the bioaccumulation and chronic toxicity of Cd in zebrafish. Since Antarctic marine organisms are already exposed to a naturally high bioaccumulation of Cd and Hg, ingestion of MPs could contribute to their exceeding the tolerance thresholds for these metals (Fig. 2).
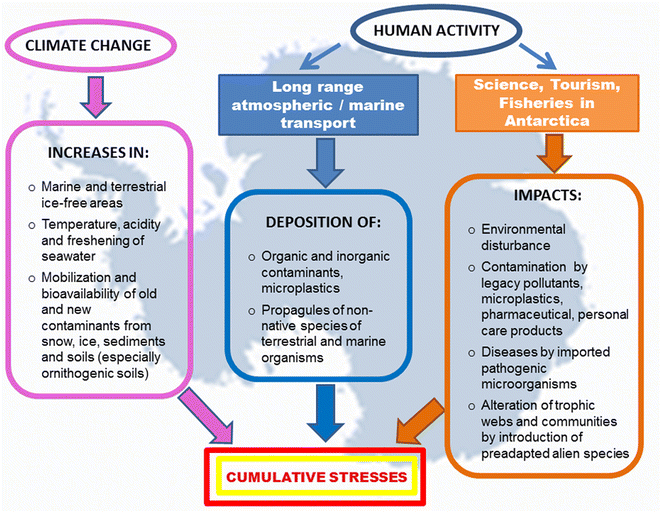 |
| Fig. 2 Simultaneous and cumulative impacts of climate changes and human activities on Antarctic organisms and ecosystems. | |
Conclusions
Most of continental Antarctica and the Southern Ocean are minimally affected by local human activities, and receive persistent contaminants primarily through long-range transport processes. Therefore, levels of contamination in snow, water, soil, and sediment are among the lowest reported in the global environment. Several species of Antarctic lichens, mosses, seabirds and marine organisms with a circumpolar distribution are reliable biomonitors of global emissions of metals and POPs, and usually accumulate these contaminants to levels below or in the same range as those measured in related species from other remote regions. The main exception appears to be the “natural” accumulation of Cd and Hg, which is likely due to the unique features of the Southern Ocean environment and its food webs. The species-specific ecophysiological characteristics, migration routes and life histories of some long-lived seabirds (such as the south polar skua) may also contribute to significant bioaccumulation of some organic contaminants. However, as some recent surveys have suggested, after the declining Pb contamination, the POPs concentrations in Antarctic organisms are likely to be declining with the entry into force of global conventions banning POPs. Nonetheless, climate change can (directly or indirectly) affect the transport, pathways and environmental fate of persistent contaminants released in the Southern Hemisphere, and can also remobilize and make bioavailable those deposited in the past. Therefore, continental-scale monitoring with standardized procedures is needed to evaluate the long-term distribution pattern of legacy contaminants and their possible biological effects.
ATPEP bans the import and release of POPs in Antarctica, and provides stringent guidelines for the protection of its near-pristine environment and its value for studying global processes. However, the research results summarized in this review show that the global chemical production and the growing number of visitors and activities undertaken in certain Antarctic regions are sources of numerous (and quite overlooked) persistent contaminants. Several scientific and tourism stations and vessels lack wastewater treatment facilities, resulting in the release of metals, hydrocarbons, detergents, flame retardants, personal care products, pharmaceuticals, MPs and pathogenic microorganisms into marine ecosystems. Many of the chemicals are not yet subject to regulatory criteria. For some of them, there is also a lack of standardized methodologies for sampling and analytical determinations. Efforts directed at a reliable assessment of their occurrence and distribution appear to be particularly urgent in the marine ecosystems of the Antarctic Peninsula and the islands of Scotia Arc, where most human activities are concentrated. Moreover, these regions are more exposed to the transport of contaminants from South America, and have experienced conspicuous temperature increases in the recent past. In coastal marine ecosystems near scientific stations, chronic co-exposure to some contaminants of emerging interest and to pathogenic microorganisms can give rise to synergistic or additive biological effects. Most Antarctic marine organisms are endemic species with unique ecophysiological adaptations to living in seawater with near freezing temperatures and tolerate only minimal variations. Small increases in water temperature can affect the properties of cell membranes and the metabolic processes involved in the absorption and detoxification of environmental contaminants. Thus, when evaluating biological responses to simultaneous exposure to metals, POPs, PCPPs, MPs, and pathogenic organisms, it is necessary to consider possible exacerbations due the concomitant impact of climate-related stressors and other anthropogenic disturbances.
The Southern Ocean is very sensitive to water acidification, and has shown a marked decrease in sea-ice formation in recent years. These processes can modify the biogeochemical cycling of nutrients, the productivity of marine ecosystems, and the composition of biotic communities. Warming and changes in sea-ice cover, along with rising Hg emissions in the Southern Hemisphere, could also enhance Antarctica's role as a metal “cold trap” through increased inputs of reactive halogens into the continental atmosphere and increased Hg depletion events.
Conflicts of interest
There are no conflicts to declare.
References
- W. J. L. Sladen, C. M. Menzie and W. L. Reichel, DDT residues in Adélie penguins and a crabeater seal from Antarctica, Nature, 1966, 210, 670–673, DOI:10.1038/210670a0.
- R. W. Risebrough, P. Rieche, D. B. Peakall, S. G. Herman and M. N. Kirven, Polychlorinated biphenyls in the global ecosystem, Nature, 1968, 220, 1098–1102, DOI:10.1038/2201098a0.
- M. J. Molina and F. S. Rowland, Stratospheric sink for chlorofluoromethanes: chlorine atom-catalysed destruction of ozone, Nature, 1974, 249, 810–812, DOI:10.1038/249810a0.
- J. Farman, B. Gardiner and J. Shanklin, Large losses of total ozone in Antarctica reveal seasonal ClOx/NOx interaction, Nature, 1985, 315, 207–210, DOI:10.1038/315207a0.
-
F. Albrecht and C. F. Parker, Healing the ozone layer: the Montreal Protocol and the lessons and limits of a global governance success story, in Great Policy Successes, ed. M. Compton and P. Hart, Oxford University Press, Oxford, 2019, pp. 304–322, DOI:10.1093/oso/9780198843719.003.0016.
-
R. Bargagli, Antarctic Ecosystems: Environmental Contamination, Climate Change, and Human Impact, Springer-Verlag, Berlin, 2005 Search PubMed.
- R. Bargagli, Environmental contamination in Antarctic ecosystems, Sci. Total Environ., 2008, 400, 212–226, DOI:10.1016/j.scitotenv.2008.06.062.
- R. B. Aronson, S. Thatje, J. B. McClintock and K. A. Hughes, Anthropogenic impacts on marine ecosystems in Antarctica, Ann. N. Y. Acad. Sci., 2011, 1223, 82–107, DOI:10.1111/j.1749-6632.2010.05926.x.
-
Antarctic Futures: Human Engagement with the Antarctic Environment, ed. T. Tin, D. Liggett and P. T. Maher, Springer, Berlin, 2014 Search PubMed.
- Z. Xie, P. Zhang, Z. Wu, S. Zhang, L. Wei and L. Mi,
et al., Legacy and emerging contaminants in the polar regions, Sci. Total Environ., 2022, 835, 155376, DOI:10.1016/j.scitotenv.2022.155376.
- F. Wania and D. Mackay, A global distribution model for persistent organic chemicals, Sci. Total Environ., 1995, 160–161, 211–232, DOI:10.1016/0048-9697(95)04358-8.
- J. Potapowicz, D. Szumińska, M. Szopińska and Z. Polkowska, The influence of global climate change on the environmental fate of anthropogenic pollution released from the permafrost: Part I. Case study of Antarctica, Sci. Total Environ., 2019, 651, 1534–1548, DOI:10.1016/j.scitotenv.2018.09.168.
- S. Esteban, L. Moreno-Merino, R. Matellanes, M. Català, M. Gorga, M. Petrovic and M. López de Alda,
et al., Presence of endocrine disruptors in freshwater in the northern Antarctic Peninsula region, Environ. Res., 2016, 147, 179–192, DOI:10.1016/j.envres.2016.01.034.
- A. Krasnobaev, G. ten Dam, R. Boerrigter-Eenling, F. Peng, S. P. J. van Leeuwen and S. A. Morley,
et al., Legacy and emerging organic pollutants in Antarctic benthic invertebrates near Rothera Point, western Antarctic Peninsula, Environ. Sci. Technol., 2020, 54, 2763–2771, DOI:10.1021/acs.est.9b06622.
- A. Olalla, L. Moreno and Y. Valcárcel, Prioritisation of emerging contaminants in the northern Antarctic Peninsula based on their environmental risk, Sci. Total Environ., 2020, 742, 140417, DOI:10.1016/j.scitotenv.2020.140417.
- G. Caruso, E. Bergami, N. Singh and I. Corsi, Plastic occurrence, sources, and impacts in Antarctic environment and biota, Water Biol. Secur., 2022, 2, 100034, DOI:10.1016/j.watbs.2022.100034.
- E. Rota, E. Bergami, I. Corsi and R. Bargagli, Macro-and microplastics in the Antarctic environment: ongoing assessment and perspectives, Environments, 2022, 9, 93, DOI:10.3390/environments9070093.
- M. Szopińska, J. Potapowicz, K. Jankowska, A. Luczkiewicz, O. Svahn and E. Björklund,
et al., Pharmaceuticals and other contaminants of emerging concern in Admiralty Bay as a result of untreated wastewater discharge: status and possible environmental consequences, Sci. Total Environ., 2022, 835, 155400, DOI:10.1016/j.scitotenv.2022.155400.
-
COMNAP (Council of Managers of National Antarctic Programs), Antarctic facilities information, 2020, https://www.comnap.aq/antarctic-facilities-information, accessed 26 October 2022 Search PubMed.
- T. Tin, Z. L. Fleming, K. A. Hughes, D. G. Ainley, P. Convey and C. A. Moreno,
et al., Impacts of local human activities on the Antarctic environment, Antarct. Sci., 2009, 21, 3–33, DOI:10.1017/S0954102009001722.15.
- K. A. Fryirs, E. G. Hafsteindottir, S. C. Stark and S. C. Gore, Metal and petroleum hydrocarbon contamination at Wilkes Station, East Antarctica, Antarct. Sci., 2014, 27, 1–16, DOI:10.1017/S0954102014000443.
- M. C. Kennicutt II, A. Klein, P. Montagna, S. Sweet, T. Wade and T. Palmer,
et al., Temporal and spatial patterns of anthropogenic disturbance at McMurdo Station, Antarctica, Environ. Res. Lett., 2010, 5, 034010, DOI:10.1088/1748-9326/5/3/034010.
- F. Gröndahl, J. Sidenmark and A. Thomsen, Survey of waste water disposal practices at Antarctic research stations, Polar Res., 2008, 28, 298–306, DOI:10.3402/polar.v28i2.6109.
- B. Duarte, C. Gameiro, A. R. Matos, A. Figueiredo, M. Sousa Silva and C. Cordeiro,
et al., First screening of biocides, persistent organic pollutants, pharmaceutical and personal care products in Antarctic phytoplankton from Deception Island by FT-ICR-MS, Chemosphere, 2021, 274, 129860, DOI:10.1016/j.chemosphere.2021.129860.
- E. Bergami, E. Ferrari, M. G. J. Löder, G. Birarda, C. Laforsch, L. Vaccari and I. Corsi, Textile microfibres in wild Antarctic whelk Neobuccinum eatoni (Smith, 1875) from Terra Nova Bay (Ross Sea, Antarctica), Environ. Res., 2022, 216, 114487, DOI:10.1016/j.envres.2022.114487.
- H. S. Lenihan, Benthic marine pollution around McMurdo Station, Antarctica. A summary of findings, Mar. Pollut. Bull., 1992, 25, 318–323, DOI:10.1016/0025-326X(92)90689-4.
- L. Cunningham, J. S. Stark, I. Snape, A. McMinn and M. J. Riddle, Effects of metals and petroleum hydrocarbon contamination on benthic diatom communities near Casey Station, Antarctica: an experimental approach, J. Phycol., 2003, 39, 490–503, DOI:10.1046/j.1529-8817.2003.01251.x.
- R. C. Montone, S. Taniguchi, F. I. Colabuono, C. C. Martins, C. V. Z. Cipro and H. S. Barroso,
et al., Persistent organic pollutants and polycyclic aromatic hydrocarbons in penguins of the genus Pygoscelis in Admiralty Bay—an Antarctic specially managed area, Mar. Pollut. Bull., 2016, 106, 377–382, DOI:10.1016/j.marpolbul.2016.02.047.
- J. S. Stark, Effects of lubricant oil and diesel on macrofaunal communities in marine sediments: a five-year field experiment in Antarctica, Environ. Pollut., 2022, 311, 119885, DOI:10.1016/j.envpol.2022.119885.
- R. Bargagli, J. C. Sanchez-Hernandez, L. Martella and F. Monaci, Mercury, cadmium and lead accumulation in Antarctic mosses growing along a nutrient and a moisture gradient, Polar Biol., 1998, 19, 316–322 CrossRef.
- A. Cincinelli, T. Martellini, K. Pozo, P. Kukučka, O. Audy and S. Corsolini,
Trematomus bernacchii as an indicator of POP temporal trend in the Antarctic seawater, Environ. Pollut., 2015, 217, 19–25, DOI:10.1016/j.envpol.2015.12.057.
- R. Bargagli, L. Nelli, S. Ancora and S. Focardi, Elevated cadmium accumulation in marine organisms from Terra Nova Bay (Antarctica), Polar Biol., 1996, 16, 513–520, DOI:10.1007/BF02329071.
- W. Espejo, J. d. A. Padilha, K. A. Kidd, P. R. Dorneles, R. Barra and O. Malm,
et al., Trophic transfer of cadmium in marine food webs from Western Chilean Patagonia and Antarctica, Mar. Pollut. Bull., 2018, 137, 246–251, DOI:10.1016/j.marpolbul.2018.10.022.
- A. L. Webb, K. A. Hughes, M. M. Grand, M. C. Lohan and L. S. Peck, Sources of elevated heavy metal concentrations in sediments and benthic marine invertebrates of the western Antarctic Peninsula, Sci. Total Environ., 2020, 698, 134268, DOI:10.1016/j.scitotenv.2019.134268.
- R. S. Matias, H. R. Guímaro, J. C. Xavier, P. Bustamante, J. Seco, N. Chipev and J. Fragão,
et al., Mercury biomagnification in an Antarctic food web of the Antarctic Peninsula, Environ. Pollut., 2022, 304, 119199, DOI:10.1016/j.envpol.2022.119199.
- H. K. Midthaug, D. J. Hitchcock, J. O. Bustnes, A. Polder, S. Descamps and A. Tarroux,
et al., Within and between breeding-season changes in contaminant occurrence and body conditions in the Antarctic breeding south polar skua, Environ. Pollut., 2021, 284, 117434, DOI:10.1016/j.envpol.2021.117434.
- A. M. Hancok, C. K. King, J. S. Stark, A. McMinn and A. T. Davidson, Effects of ocean acidification on Antarctic marine organisms: a meta-analysis, Ecol. Evol., 2020, 10, 4495–4514, DOI:10.1002/ece3.6205.
- R. Bargagli, Trace metals in Antarctica related to climate change and increasing human impact, Rev. Environ. Contam. Toxicol., 2000, 166, 129–173 CAS , PMID: 10868078..
- R. Bargagli, Trace metals in Antarctic organisms and the development of circumpolar biomonitoring network, Rev. Environ. Contam. Toxicol., 2001, 171, 53–110, DOI:10.1007/978-1-4613-0161-5_2.
- F. A. M. Planchon, C. F. Boutron, C. Barbante, G. Cozzi, V. Gaspari and E. W. Wolff,
et al., Changes in heavy metals in Antarctic snow from Coats Land since the mid-19th to the late-20th century, Sci. Total Environ., 2002, 200, 207–222, DOI:10.1016/S0012-821X(02)00612-X.
- K. Liu, S. Hou, S. Wu, W. Zhang, X. Zou and J. Yu,
et al., Assessment of heavy metal contamination in the atmospheric deposition during 1950–2016 A.D. from a snow pit at Dome A, East Antarctica, Environ. Pollut., 2021, 268B, 115848, DOI:10.1016/j.envpol.2020.115848.
- C. Han, S. Kim, A.-H. Lee, Y. Han, S. Lee and C. Chang,
et al., Variations in lead isotopes in Antarctic snow from northern Victoria Land during 2012–2015, Environ. Res. Commun., 2022, 4, 055006, DOI:10.1088/2515-7620/ac6cd1.
- C. Marina-Montes, L. V. Pérez-Arribas, J. Anzano, S. Fdez-Ortiz de Vallejuelo, J. Aramendia and L. Gómez-Nubla,
et al., Characterization of atmospheric aerosols in the Antarctic region using Raman Spectroscopy and Scanning Electron Microscopy, Spectrochim. Acta, Part A, 2022, 266, 120452, DOI:10.1016/j.saa.2021.120452..
- E. D. Suttie and E. W. Wolff, The local deposition of heavy metal emissions from point sources in Antarctica, Atmos. Environ., Part A, 1993, 27, 1833–1841, DOI:10.1016/0960-1686(93)90288-A.
- C. Barbante, C. Turetta, G. Capodaglio and G. Scarponi, Recent decrease in the lead concentrations in Antarctic snow, Int. J. Environ. Anal. Chem., 1997, 68, 457–477, DOI:10.1080/03067319708030847.
- S. Bertinetti, F. Ardini, M. A. Vecchio, L. Caiazzo and M. Grotti, Isotopic analysis of snow from Dome C indicates changes in the source of atmospheric lead over the last fifty years in East Antarctica, Chemosphere, 2020, 255, 126858, DOI:10.1016/j.chemosphere.2020.126858.
- M. Chaparro, H. Nuñez, J. Lirio, C. Gogorza and A. Sinito, Magnetic screening and heavy metal pollution studies in soils from Marambio Station, Antarctica, Antarct. Sci., 2007, 19, 379–393, DOI:10.1017/S0954102007000454.
- E. de Lima Neto, M. B. B. Guerra, A. Thomazini, M. Daher, A. Medeiros de Andrade and C. E. G. R. Schaefer, Soil contamination by toxic metals near an Antarctic refuge in Robert Island, Maritime Antarctica: A monitoring strategy, Water, Air, Soil Pollut., 2017, 228, 66, DOI:10.1007/s11270-017-3245-4.
-
A. G. Klein, S. T. Sweet, M. C. Kennicutt II, T. L. Wade, T. A. Palmer and P. Montagna, Long-term monitoring of human impacts to the terrestrial
environment at McMurdo Station, in Antarctic Futures, ed. T. Tin, D. Liggett, P. Maher and M. Lamers, Springer, Dordrecht, 2017, pp. 213–227, DOI:10.1007/978-94-007-6582-5_9.
- S. Ancora, V. Volpi, S. Olmastroni, S. Focardi and C. Leonzio, Assumption and elimination of trace elements in Adélie penguins from Antarctica: a preliminary study, Mar. Environ. Res., 2002, 54, 341–344, DOI:10.1016/s0141-1136(02)00198-8.
- E. Sparaventi, A. Rodríguez-Romero, A. Barbosa, L. Ramajo and A. Tovar-Sánchez, Trace elements in Antarctic penguins and the potential role of guano as source of recycled metals in the Southern Ocean, Chemosphere, 2021, 285, 131423, DOI:10.1016/j.chemosphere.2021.131423.
- M. F. Castro, M. Meier, J. C. L. Neves, M. R. Francelino, C. Ernesto and S. S. Oliveira, Influence of different seabird species on trace metal content in Antarctic soils, An. Acad. Bras. Cienc., 2022, 94, e20210623, DOI:10.1590/0001-3765202220210623.
- Z. Chu, Z. Yang, Y. Wang, L. Sun, L. Yang and Y. Gao, Assessment of heavy metal contamination from penguins and anthropogenic activities on Fildes Peninsula and Ardley Island, Antarctic, Sci. Total Environ., 2019, 646, 951–957, DOI:10.1016/j.scitotenv.2018.07.152.
- S. T. Brooks, J. Jabour, J. van den Hoff and D. M. Bergstrom, Our footprint on Antarctica competes with nature for rare ice-free land, Nat. Sustain., 2019, 2, 185–190, DOI:10.1038/s41893-019-0237-y.
- S. Bhakta, T. K. Rout, D. Karmakar, C. Pawar and P. K. Padhy, Trace elements and their potential risk assessment on polar ecosystem of Larsemann Hills, East Antarctica, Polar Sci., 2022, 31, 100788, DOI:10.1016/j.polar.2022.100788.
- Z. Lu, M. Cai, J. Wang, H. Yang and J. He, Baseline values for metals in soils of Fildes Peninsula, King George Island, Antarctica: the extent of anthropogenic pollution, Environ. Monit. Assess., 2012, 184, 7013–7021, DOI:10.1007/s10661-011-2476-x.
- J. E. Celis, R. Barra, W. Espejo, D. González-Acuña and S. Jara, Trace element concentrations in biotic matrices of gentoo penguins (Pygoscelis papua) and coastal soils from different locations of the Antarctic Peninsula, Water, Air, Soil Pollut., 2015, 226, 2266, DOI:10.1007/s11270-014-2266-5.
- R. Bargagli, E. Battisti, S. Focardi and P. Formichi, Preliminary data on environmental distribution of mercury in northern Victoria Land, Antarctica, Antarct. Sci., 1993, 5, 3–8, DOI:10.1017/S0954102093000021.
- A. Poblet, S. Andrade, M. Scagliola, C. Vodopivez, A. Curtosi, A. Pucci and J. Marcovecchio, The use of epilithic Antarctic lichens (Usnea aurantiacoatra and U. antarctica) to determine deposition patterns of heavy metals in the Shetland Islands, Antarctica, Sci. Total Environ., 1997, 207, 187–194, DOI:10.1016/S0048-9697(97)00265-9.
- A. Cabrerizo, J. Dachs, D. Barcelò and K. C. Jones, Influence of organic matter content and human activities on the occurrence of organic pollutants in Antarctic soils, lichens, grass, and mosses, Environ. Sci. Technol., 2012, 46, 1396–1405, DOI:10.1021/es203425b.
- C. Carvallo, N. Godoy, B. Aguila, C. Egas, R. Fuentealba and M. Préndz, Long-term monitoring of atmospheric pollution in the Maritime Antarctic with the lichen Usnea aurantiaco-atra (Jacq.) Bory: a magnetic and elemental study, Antarct. Sci., 2021, 33, 660–673, DOI:10.1017/S0954102021000419.
- F. Monaci, F. Fantozzi, R. Figueroa, O. Parra and R. Bargagli, Baseline element composition of foliose and fruticose lichens along the steep climatic gradients of SW Patagonia (Aisén Region, Chile), J. Environ. Monit., 2012, 14, 2309–2316, 10.1039/C2EM30246B.
- E. Cecconi, G. Incerti, F. Capozzi, P. Adamo, R. Bargagli and R. Benesperi,
et al., Background element content of the lichen Pseudevernia furfuracea: a supra-national state of art implemented by novel field data from
Italy, Sci. Total Environ., 2018, 622–623, 282–292, DOI:10.1016/j.scitotenv.2017.11.276.
- P. Adamo, R. Bargagli, S. Giordano, P. Modenesi, F. Monaci and E. Pittao,
et al., Natural and pre-treatment variability in the chemical composition and morphology of lichens and mosses selected for active monitoring of airborne elements, Environ. Pollut., 2007, 152, 11–19, DOI:10.1016/j.envpol.2007.06.008.
- R. Bargagli, F. Monaci, F. Borghini, F. Bravi and C. Agnorelli, Mosses and lichens as biomonitors of trace metals. A comparison study of Hypnum cupressiforme and Parmelia caperata in a former mining district in Italy, Environ. Pollut., 2002, 116, 279–287, DOI:10.1016/s0269-7491(01)00125-7.
- F. Slemr, E.-G. Brunke, R. Ebinghaus and J. Kuss, Worldwide trend of atmospheric mercury since 1995, Atmos. Chem. Phys., 2011, 11, 4779–4787, DOI:10.5194/acp-11-4779-2011.
- M. A. Hindell, N. Brothers and R. Gales, Mercury and cadmium concentrations in the tissues of three species of southern albatrosses, Polar Biol., 1999, 22, 102–108, DOI:10.1007/s003000050396.
- S. Tavares, J. C. Xavier, R. A. Phillips, M. E. Pereira and M. A. Pardal, Influence of age, sex, and breeding status on mercury accumulation patterns in the wandering albatross Diomedea exulans, Environ. Pollut., 2013, 181, 315–320, DOI:10.1016/j.envpol.2013.06.032.
- D. R. Thompson, R. W. Furness and S. A. Lewis, Temporal and spatial variation in mercury concentrations in some albatrosses and petrels from the sub-Antarctic, Polar Biol., 1993, 13, 239–244, DOI:10.1007/BF00238759.
- A.-T. E. Vo, M. S. Bank, J. P. Shine and S. V. Edwards, Temporal increase in organic mercury in an endangered pelagic seabird assessed by century-old museum specimens, Proc. Natl. Acad. Sci. U. S. A., 2011, 108, 7466–7471, DOI:10.1073/pnas.1013865108.
-
A. V. Skalny, M. G. Skalnaya, A. A. Nikonorov and A. A. Tinkov, Selenium antagonism with mercury and arsenic: From chemistry to population health and demography, in Selenium, ed. D. Hatfield, U. Schweizer, P. Tsuji and V. Gladyshev, Springer, Cham, 2016, pp. 401–412, DOI:10.1007/978-3-319-41283-2_34.
- P. Bustamante, A. Carravieri, A. Goutte, C. Barbraud, K. Delord and O. Chastel,
et al., High feather mercury concentrations in the wandering albatross are related to sex, breeding status and trophic ecology with no demographic consequences, Environ. Res., 2016, 144A, 1–10, DOI:10.1016/j.envres.2015.10.024.
- W. H. Schroeder, K. G. Alauf, L. A. Barrie, J. Y. Lu, A. Steffen, D. R. Schneeberger and T. Berg, Arctic springtime depletion of mercury, Nature, 1998, 394, 331–332, DOI:10.1038/28530.
- R. Bargagli, C. Agnorelli, F. Borghini and F. Monaci, Enhanced deposition and bioaccumulation of mercury in Antarctic terrestrial ecosystems facing a coastal polynya, Environ. Sci. Technol., 2005, 39, 8150–8155, DOI:10.1021/es0507315.
- Y. Wang, G. Shi, D. Wang, Q. Zhao, S. Jiang and Y. Li,
et al., Relationships between concentrations of mercury and organic carbon in soils allow the identification of Antarctic ice-free areas with enhanced deposition of the metal, Catena, 2022, 220, 106718, DOI:10.1016/j.catena.2022.106718.
- J. A. Fisher, L. Schneider, A.-H. Fostier, S. Guerrero, J. R. D. Guimarães and C. Labuschagne,
et al., A synthesis of mercury research in the Southern Hemisphere, part 2: anthropogenic perturbations, Ambio, 2023, 52, 918–937, DOI:10.1007/s13280-023-01840-5.
- H. Angot, O. Magand, D. Helmig, P. Ricaud, B. Quennehen and H. Gallée,
et al., New insights into the atmospheric mercury cycling in central Antarctica and implications on a continental scale, Atmos. Chem. Phys., 2016, 16, 8249–8264, DOI:10.5194/acp-16-8249-2016.
- C. Li, J. Chen, H. Angot, W. Zheng, G. Shi and M. Ding,
et al., Seasonal variation of mercury and its isotopes in atmospheric particles at the coastal Zhongshan Station, eastern Antarctica, Environ. Sci. Technol., 2020, 54, 11344–11355, DOI:10.1021/acs.est.0c04462.
- R. Bargagli, Atmospheric chemistry of mercury in Antarctica and the role of cryptogams to assess deposition patterns in coastal ice-free areas, Chemosphere, 2016, 163, 202–208, DOI:10.1016/j.chemosphere.2016.08.007.
- J. W. Lock, D. R. Thompson, R. W. Furness and J. A. Bartle, Metal concentrations in seabirds of the New Zealand region, Environ. Pollut., 1992, 75, 289–300, DOI:10.1016/0269-7491(92)90129-x.
- P. Szefer, J. Pempkowiak, B. Skwarzec, R. Bojanowski and E. Holm, Concentration of selected metals in penguins and other representative fauna of the Antarctica, Sci. Total Environ., 1993, 138, 281–288, DOI:10.1016/0048-9697(93)90421-2.
- F. M. M. Morel and N. M. Price, The biogeochemical cycle of trace metals in the oceans, Science, 2003, 300, 944–947, DOI:10.1126/science.1083545.
- S. Jerez, M. Motas, J. Benzal, J. Diaz and A. Barbosa, Monitoring trace elements in Antarctic penguin chicks from South Shetland Islands, Antarctica, Mar. Pollut. Bull., 2013, 69, 67–75, DOI:10.1016/j.marpolbul.2013.01.004.
- R. L. Brasso, A. Chiaradia, M. J. Polito, A. Raya Rey and S. D. Emslie, A comprehensive assessment of mercury exposure in penguin populations throughout the Southern Hemisphere: Using trophic calculations to identify sources of population-level variation, Mar. Pollut. Bull., 2015, 97, 408–418, DOI:10.1016/j.marpolbul.2015.05.059.
- N. Pilcher, S. Gaw, R. Eisert, T. W. Horton, A. M. Gormely, T. L. Cole and P. O. ’B. Lyver, Latitudinal, sex and inter-specific differences in mercury and other trace metal concentrations in Adélie and Emperor penguins in the Ross Sea, Antarctica, Mar. Pollut. Bull., 2020, 154, 111047, DOI:10.1016/j.marpolbul.2020.111047.
- J. A. Padilha, G. O. Carvalho, W. Espejo, J. S. Souza, A. C. Pizzochero and L. S. T. Cunha,
et al., Factors that influence trace element levels in blood and feathers of Pygoscelis penguins from South Shetland Islands, Antarctica, Environ. Pollut., 2021, 284, 117209, DOI:10.1016/j.envpol.2021.117209.
- Y. Sun, Z. Lu, K. Xiao, L. Zeng, J. Wang and G. W. Gabrielsen, Antarctic Adélie penguin feathers as bio-indicators of geographic and temporal variations in heavy metal concentrations in their habitats, Ecotoxicol. Environ. Saf., 2020, 206, 111135, DOI:10.1016/j.ecoenv.2020.111135.
-
R. Carson, Silent Spring, Houghton Mifflin Harcourt, Boston, MA, USA, 1962 Search PubMed.
- S. Tanabe, H. Hidaka and R. Tatsukawa, PCBs and chlorinated hydrocarbon pesticides in Antarctic atmosphere and hydrosphere, Chemosphere, 1983, 12, 277–288, DOI:10.1016/0045-6535(83)90171-6.
- M. Kawano, T. Inoue, H. Hidaka and R. Tatsukawa, Chlordane compounds residues in Weddell seals (Leptonychotes weddelli) from the Antarctic, Chemosphere, 1984, 13, 95–100, DOI:10.1016/0045-6535(84)90011-0.
- E. Bacci, D. Calamari, C. Gaggi, R. Fanelli, S. Focardi and M. Morosini, Chlorinated hydrocarbons in lichen and moss samples from the Antarctic Peninsula, Chemosphere, 1986, 15, 747–754, DOI:10.1016/0045-6535(86)90041-X.
- P. Larsson, C. Järnmark and A. Södergren, PCBs and chlorinated pesticides in the atmosphere and aquatic organisms of Ross Island, Antarctica, Mar. Pollut. Bull., 1992, 25, 281–287, DOI:10.1016/0025-326X(92)90683-W.
- T. F. Bidleman, M. D. Walla, R. Roura, E. Carr and S. Schmidt, Organochlorine pesticides in the atmosphere of Southern Ocean and Antarctica, January-March, 1990, Mar. Pollut. Bull., 1993, 26, 258–262, DOI:10.1016/0025-326X(93)90064-Q.
- S. Focardi, S. Corsolini and R. Bargagli, Isomer-specific analysis and toxic potential evaluation of polychlorinated biphenyls in Antarctic fish, seabirds and Weddell seals from Terra Nova Bay (Ross Sea), Antarct. Sci., 1995, 7, 31–35, DOI:10.1017/S095410209500006X.
- M. C. Kennicutt II, S. T. Sweet, W. R. Fraser, W. L. Stockton and M. Culver, Grounding of the Bahia Paraiso at Arthur Harbor, Antarctica. 1. Distribution and fate of oil spill related hydrocarbons, Environ. Sci. Technol., 1991, 25, 509–518, DOI:10.1021/es00015a020.
- S. J. McDonald, M. C. Kennicutt II and J. M. Brooks, Evidence of polycyclic aromatic hydrocarbon (PAH) exposure in fish from the Antarctic Peninsula, Mar. Pollut. Bull., 1992, 25, 313–317, DOI:10.1016/0025-326X(92)90688-3.
- J. S. Stark, I. Snape and M. J. Riddle, The effects of petroleum hydrocarbon and heavy metal contamination of marine sediments on recruitment of Antarctic soft-sediment assemblages: a field experimental investigation, J. Exp. Mar. Biol. Ecol., 2003, 283, 21–50, DOI:10.1016/S0022-0981(02)00449-5.
- M. C. Kennicut II, Oil spillage in Antarctica: initial report of the National Science Foundation-sponsored Quick Response Team on the grounding of the Bahia Paraiso, Environ. Sci. Technol., 1990, 24, 620–624, DOI:10.1021/es00075a601.
- M. D. King, J. E. Elliott and T. D. Williams, Effects of petroleum exposure on birds: A review, Sci. Total Environ., 2021, 755, 142834, DOI:10.1016/j.scitotenv.2020.142834.
- N. A. Puasa, A. Zulkharnain, G. Verasoundarapandian, C.-Y. Wong, K. N. M. Zahri and F. Merican,
et al., Effects of diesel, heavy metals and plastics pollution on penguins in Antarctica: A review, Animals, 2021, 11, 2505, DOI:10.3390/ani11092505.
- A. Gran-Scheuch, J. Ramos-Zuñiga, E. Fuentes, D. Bravo and J. M. Pérez-Donoso, Effect of co-contamination by PAHs and heavy metals on bacterial communities of diesel contaminated soils of South Shetland Islands, Antarctica, Microorganisms, 2020, 8, 1749, DOI:10.3390/microorganisms8111749.
- T. A. Palmer, A. G. Klein, S. T. Sweet, A. J. Frazier, P. A. Montagna, T. L. Wade and J. B. Pollack, Using epibenthic fauna as biomonitors of local marine contamination adjacent to McMurdo Station, Antarctica, Mar. Pollut. Bull., 2022, 178, 113621, DOI:10.1016/j.marpolbul.2022.113621.
- R. Xue, L. Chen, Z. Lu, J. Wang, H. Yang, J. Zhang and M. Cai, Spatial distribution and source apportionment of PAHs in marine surface sediments of Prydz Bay, East Antarctica, Environ. Pollut., 2016, 219, 528–536, DOI:10.1016/j.envpol.2016.05.084.
- T. A. Palmer, A. G. Klein, S. T. Sweet, P. A. Montagna, L. J. Hyde, T. L. Wade and J. Beseres Pollack, Anthropogenic effects on the marine environment adjacent to Palmer Station, Antarctica, Antarct. Sci., 2022, 34, 79–96, DOI:10.1017/S0954102021000535.
- C. Vodopivez, A. Curtosi, E. Pelletier, R. Saint-Louis, L. U. Spairani and E. A. Hernández,
et al., Low levels of PAHs and organotin compounds in surface sediment samples from a broad marine area of 25 de Mayo (King George) Island, South Shetland Islands, Sci. Total Environ., 2021, 785, 147206, DOI:10.1016/j.scitotenv.2021.147206.
- J. Potapowicz, M. Szopińska, D. Szumińska, R. J. Bialik and Z. Polkowska, Sources and composition of chemical pollution in Maritime Antarctica (King George Island), part 1: Sediment and water analysis for PAH sources evaluation in the vicinity of Arctowski station, Chemosphere, 2022, 288, 132637, DOI:10.1016/j.chemosphere.2021.132637.
- W. Deelaman, S. Pongpiachan, D. Tipmanee, C. Choochuay, O. Suttinun, T. Charoenkalunyuta and K. Promdee, Ecotoxicological risk and health risk characterization of polycyclic aromatic hydrocarbons (PAHs) in terrestrial soils of King George Island, Antarctica, Polar Sci., 2021, 29, 100715, DOI:10.1016/j.polar.2021.100715.
- G. Na, Y. Gao, R. Li, H. Gao, C. Hou, J. Ye, S. Jin and Z. Zhang, Occurrence and sources of polycyclic aromatic hydrocarbons in atmosphere and soil from 2013 to 2019 in the Fildes Peninsula,
Antarctica, Mar. Pollut. Bull., 2020, 156, 11173, DOI:10.1016/j.marpolbul.2020.111173.
- S. Jin, S. Cao, R. Li, H. Gao and G. Na, Trophic transfer of polycyclic aromatic hydrocarbons through the food web of the Fildes Peninsula, Antarctica, Environ. Sci. Pollut. Res., 2023, 30, 55057–55066, DOI:10.1007/s11356-023-26049-7.
- M. A. Khairy, J. L. Luek, R. Dickhut and R. Lohmann, Levels, sources and chemical fate of persistent organic pollutants in the atmosphere and snow along the western Antarctic Peninsula, Environ. Pollut., 2016, 216, 304–313, DOI:10.1016/j.envpol.2016.05.092.
- S. M. Bengtson Nash, S. Wild, S. Broomhall and P. Bohlin-Nizzetto, Brominated flame retardant in Antarctic air in the vicinity of two all-year research stations, Atmosphere, 2021, 12, 668, DOI:10.3390/atmos12060668.
- R. C. Hale, S. L. Kim, E. Harvey, M. J. La Guardia, T. M. Mainor, E. O. Bush and E. M. Jacobs, Antarctic Research Bases: local sources of polybrominated diphenyl ether (PBDE) flame retardants, Environ. Sci. Technol., 2008, 42, 1452–1457, DOI:10.1021/es702547a.
- S. Corsolini, N. Ademollo, T. Martellini, D. Randazzo, M. Vacchi and A. Cincinelli, Legacy persistent pollutants including PBDEs in the trophic web of the Ross Sea (Antarctica), Chemosphere, 2017, 185, 699–708, DOI:10.1016/j.chemosphere.2017.07.054.
- E. Markham, E. K. Brault, M. Khairy, A. R. Robuck, M. E. Goebel, M. G. Cantwell, R. M. Dickhut and R. Lohmann, Time trends of polybrominated diphenyl ethers (PBDEs) in Antarctic biota, ACS Omega, 2018, 3, 6595–6604, DOI:10.1021/acsomega.8b0044098.
- A. Schiavone, S. Corsolini, N. Borghesi and S. Focardi, Contamination profiles of selected PCB congeners, chlorinated pesticides, PCDD/Fs in Antarctic fur seal pups and penguin eggs, Chemosphere, 2009, 76, 264–269, DOI:10.1016/j.chemosphere.2009.03.007.
- S. Wild, I. Eulaers, A. Covaci, R. Bossi, D. Hawker and R. Cropp,
et al., South polar skua (Chataracta maccormicki) as biovectors for long-range transport of persistent organic pollutants to Antarctica, Environ. Pollut., 2022, 292, 118358, DOI:10.1016/envpol.2021.118358.
-
UNECE (United Nations Economic Commission for Europe), Convention on Long-Range-Transboundary Air Pollution, 2021, https://unece.org/sites/default/files/2021-05/1979%20CLRTAP.e.pdf Search PubMed.
- The Stockholm Convention on Persistent Organic Pollutants, opened for signature May 23, 2001, United Nation Doc. UNEP/POPS/CONF/4, App. II (2001), reprinted in 40 ILM 532 (2001), available at https://chm.pops.int.
- S. Corsolini, Industrial contaminants in Antarctic biota, J. Chromatogr. A, 2009, 1216, 598–612, DOI:10.1016/j.chroma.2008.08.012.
- N. W. van den Brink, M. J. Riddle, M. van den Heuvel-Greve and J. A. van Franeker, Contrasting time trends of organic contaminants in Antarctic pelagic and benthic food webs, Mar. Pollut. Bull., 2011, 62, 128–132, DOI:10.1016/j.marpolbul.2010.09.002.
- E. Isla, E. Pérez-Albaladejo and C. Porte, Toxic anthropogenic signature in Antarctic continental shelf and deep sea sediments, Sci. Rep., 2018, 8, 9154, DOI:10.1038/s41598-018-27375-4.
- Y. Hao, Y. Li, X. Han, T. Wang, R. Yang and P. Wang,
et al., Air monitoring of polychlorinated biphenyls, polybrominated diphenyl ethers and organochlorine pesticides in West Antarctica during 2011-2017: concentrations, temporal trends and potential sources, Environ. Pollut., 2019, 249, 381–389, DOI:10.1016/j.envpol.2019.03.039.
- D. Ellis, C. Cipro, C. A. Ogletree, K. E. Smith and R. B. Aronson, A 50-year retrospective of persistent organic pollutants in the fat and eggs of penguins of the Southern Ocean, Environ. Pollut., 2018, 241, 155–163, DOI:10.1016/j.envpol.2018.05.003.
- N. D. Kuepper, L. Böhm, C. Braun, P. Bustamante, R.-A. Düring, M. Libertelli and P. Quillfeldt, Persistent organic pollutants and mercury in a colony of Antarctic seabirds: higher concentrations in 1998, 2001, and 2003 compared to 2014 to 2016, Polar Biol., 2022, 45, 1229–1245, DOI:10.1007/s00300-022-03065-w.
- M. Cai, H. Yang, X. Zhiyong, Z. Zhao, F. Wang, Z. Lu, R. Sturm and R. Ebinghaus, Per- and polyfluroalkyl substances in snow, lake, surface runoff water and coastal seawater in Fildes Peninsula, King George Island, J. Hazard. Mater., 2012, 209–210, 335–342, DOI:10.1016/j.jhazmat.2012.01.030.
- G. Shan, Q. Xiang, X. Feng, W. Wu, L. Yang and L. Zhu, Occurrence and sources of per- and polyfluoroalkyl substances in the ice-melting lakes of Larsemann Hills, East Antarctica, Sci. Total Environ., 2021, 781, 146747, DOI:10.1016/j.scitotenv.2021.146747.
- A. Schiavone, S. Corsolini, K. Kannan, L. Tao, W. Trivelpiece, D. Torres Jr and S. Focardi, Perfluorinated contaminants in fur seal pups and penguin eggs from South Shetland, Antarctica, Sci. Total Environ., 2009, 407, 3899–3904, DOI:10.1016/j.scitotenv.2008.12.058.
- J. L. Roscales, A. Vicente, P. G. Ryan, J. Gonzáles-Solis and B. Jiménez, Spatial and interspecies heterogeneity in concentrations of perfluoroalkyl substances (PFASs) in seabirds of the Southern Ocean, Environ. Sci. Technol., 2019, 53, 9855–9865, DOI:10.1021/acs.est.9b02677.
- K. Gao, X. Miao, J. Fu, Y. Chen, H. Li and W. Pan,
et al., Occurrence and trophic transfer of per- and polyfluoroalkyl substances in an Antarctic ecosystem, Environ. Pollut., 2020, 257, 113383, DOI:10.1016/j.envpol.2019.113383.
- R. M. Baena-Nogueras, E. Gonzáles-Mazo and P. A. Lara-Martin, Degradation kinetics of pharmaceuticals and personal care products in surface waters: photolysis vs biodegradation, Sci. Total Environ., 2017, 590–591, 643–654, DOI:10.1016/j.scitotenv.2017.03.015.
- A. Schiavone, K. Kannan, Y. Horii, S. Focardi and S. Corsolini, Occurrence of brominated flame retardant, polycyclic musks, and chlorinated naphthalenes in seal bubbler from Antarctica: comparisons to organochlorines, Mar. Pollut. Bull., 2009, 58, 1415–1419, DOI:10.1016/j.marpolbul.2009.05.019.
- M. Vecchiato, E. Gregoris, E. Barbaro, C. Barbante, R. Piazza and A. Gambaro, Fragrances in the seawater of Terra Nova Bay, Antarctica, Sci. Total Environ., 2017, 593–594, 375–379, DOI:10.1016/j.scitotenv.2017.03.197.
- P. Emnet, S. Gaw, G. Nothcott, B. Storey and L. Graham, Personal care products and steroid hormones in the Antarctic coastal environment associated with two Antarctic research stations, McMurdo Station and Scott Base, Environ. Res., 2015, 136, 331–342, DOI:10.1016/j.envres.2014.10.019.
- S. Gonzáles-Alonso, L. Moreno-Merino, S. Esteban, M. López de Alda, D. Barceló and J. J. Durán,
et al., Occurrence of pharmaceutical, recreational and psychotropic drug residues in surface water on the northern Antarctic Peninsula region, Environ. Pollut., 2017, 229, 241–254, DOI:10.1016/j.envpol.2017.05.060.
- H. K. Tam, C. M. V. L. Wong, S. T. Yong, J. Blamey and M. Gonzáles, Multiple-antibiotic-resistant bacteria from the maritime Antarctic, Polar Biol., 2015, 38, 1129–1141, DOI:10.1007/s00300-015-1671-6.
- T. H. Bell, K. L. Callender, L. G. Whyte and C. W. Greer, Microbial competition in polar Soils: A review of an understudied but potentially important control on productivity, Biology, 2013, 2, 533–554, DOI:10.3390/biology2020533.
- D. Jara, H. Bello-Toledo, M. Domínguez, C. Cigarroa, P. Fernández and L. Vergara,
et al., Antibiotic resistance in bacterial isolates from freshwater samples in Fildes Peninsula, King George Island, Antarctica, Sci. Rep., 2020, 10, 3145, DOI:10.1038/s41598-020-60035-0.
- W. N. Bonner and T. S. McCann, Neck collars on fur seals, Arctocephalus gazella, at South Georgia, Br. Antarct. Surv.
Bull., 1982, 57, 73–77 Search PubMed.
- J. A. van Franeker and P. J. Bell, Plastic ingestion by petrels breeding in Antarctica, Environ. Pollut., 1988, 19, 672–674, DOI:10.1016/0025-326X(88)90388-8.
- G. Suaria, V. Perold, J. R. Lee, F. Lebouard, S. Aliani and P. G. Ryan, Floating macro- and microplastics around the Southern Ocean: Results from the Antarctic Circumnavigation Expedition, Environ. Int., 2020, 136, 105494, DOI:10.1016/j.envint.2020.105494.
- S. Pakhomova, A. Berezina, A. L. Lusher, I. Zhdanov, K. Silvestrova and P. Zavialov,
et al., Microplastic variability in subsurface water from the Arctic to Antarctica, Environ. Pollut., 2022, 298, 118808, DOI:10.1016/j.envpol.2022.118808.
- C. Leistenschneider, P. Burkhardt-Holm, T. Mani, S. Primpke, H. Taubner and G. Gerdts, Microplastics in the Weddell Sea (Antarctica): A forensic approach for discrimination between environmental and vessel-induced microplastics, Environ. Sci. Technol., 2021, 55, 15900–15911, DOI:10.1021/acs.est.1c05207.
- P. Kuklinski, L. Wicikowski, M. Koper, T. Grala, H. Leniec-Koper and M. Barasiński,
et al., Offshore surface waters of Antarctica are free of microplastics, as revealed by a circum-Antarctic study, Mar. Pollut. Bull., 2019, 149, 110573, DOI:10.1016/j.marpolbul.2019.110573.
- C. L. Waller, H. J. Griffiths, C. M. Waluda, S. E. Thorpe, I. Loaiza and B. Moreno,
et al., Microplastics in the Antarctic marine systems: An emerging area of research, Sci. Total Environ., 2017, 598, 220–227, DOI:10.1016/j.scitotenv.2017.03.283.
- A. K. Mishra, J. Singh and P. P. Mishra, Microplastics in polar regions: an early warning to the world's pristine ecosystem, Sci. Total Environ., 2021, 784, 147149, DOI:10.1016/j.scitotenv.2021.147149.
- A. A. Sfriso, Y. Tomio, B. Rosso, A. Gambaro, A. Sfriso and F. Corami,
et al., Microplastic accumulation in benthic invertebrates in Terra Nova Bay (Ross Sea, Antarctica), Environ. Int., 2020, 137, 105587, DOI:10.1016/j.envint.2020.105587.
- T. Bottari, V. Conti Nibali, C. Branca, M. Grotti, S. Savoca and T. Romeo,
et al., Anthropogenic microparticles in the emerald rockcod Trematomus bernacchii (Nototheniidae) from the Antarctic, Sci. Rep., 2022, 12, 17214, DOI:10.1038/s41598-022-21670-x.
- M. Zhang, S. Liu, J. Bo, R. Zheng, F. Hong and F. Gao,
et al., First evidence of microplastic contamination in Antarctic fish (Actinopterygii, Perciformes), Water, 2022, 14, 3070, DOI:10.3390/w14193070.
- F. Bessa, N. Ratcliffe, V. Otero, P. Sobral, J. C. Marques and C. M. Waluda,
et al., Microplastics in gentoo penguins from the Antarctic region, Sci. Rep., 2019, 9, 14191, DOI:10.1038/s41598-019-50621-2.
- C. Le Guen, G. Suaria, R. B. Sherley, P. G. Ryan, S. Aliani, L. Boehme and A. S. Brierley, Microplastic study reveals the presence of natural and synthetic fibres in the diet of king penguins (Aptenodytes patagonicus) foraging from South Georgia, Environ. Int., 2020, 134, 105303, DOI:10.1016/j.envint.2019.105303.
- J. Fragao, F. Bessa, V. Otero, A. Barbosa, P. Sobral and C. M. Waluda,
et al., Microplastics and other anthropogenic particles in Antarctica: Using penguins as biological samplers, Sci. Total Environ., 2021, 788, 147698, DOI:10.1016/j.scitotenv.2021.147698.
- C. Leistenschneider, C. Le Bohec, O. Eisen, A. Houstin, S. Nelf and S. Primpke,
et al., No evidence of microplastic ingestion in emperor penguin chicks (Aptenodytes forsteri) from the Atka Bay colony (Dronning Maud Land, Antarctica), Sci. Total Environ., 2022, 851, 158314, DOI:10.1016/j.scitotenv.2022.158314.
- O. Garcia-Garin, I. García-Cuevas, M. Drago, D. Rita, M. Parga, M. Gazo and L. Cardona, No evidence of microplastics in Antarctic fur seal scats from a hotspot of human activity in Western Antarctica, Sci. Total Environ., 2020, 737, 140210, DOI:10.1016/j.scitotenv.2020.140210.
- A. M. Jardine, J. F. Provencher, S. J. Insley, L. Tauzer, W. D. Halliday and M. P. T. Bourdages,
et al., No accumulation of microplastics detected in western Canadian ringed seals (Pusa hispida), Mar. Pollut. Bull., 2023, 188, 114692, DOI:10.1016/j.marpolbul.2023.114692.
- J. Turner, H. Lu, I. White, J. C. King, T. Phillips and J. S. Hosking,
et al., Absence of 21st century warming on Antarctic Peninsula consistent with natural variability, Nature, 2016, 535, 411–415, DOI:10.1038/nature18645.
- R. Bargagli, Terrestrial ecosystems of the Antarctic Peninsula and their responses to climate changes and anthropogenic impacts, Ukr. Antarct. J., 2020, 2, 84–97, DOI:10.33275/1727-7485.2.2020.656.
- T. A. Palmer, A. G. Klein, S. T. Sweet, P. A. Montagna, L. J. Hyde and J. Sericano,
et al., Long-term changes in contamination and macrobenthic communities adjacent to McMurdo Station, Antarctica, Sci. Total Environ., 2021, 764, 142798, DOI:10.1016/j.scitotenv.2020.142798.
- D. Veytia, S. Bestley, S. Kawagushi, K. M. Meiners, E. J. Murphy and A. D. Fraser,
et al., Overwinter sea-ice characteristics important for Antarctic krill recruitment in the southwest Atlantic, Ecol. Indic., 2021, 129, 107934, DOI:10.1016/j.ecolind.2021.107934.
- C. Eayrs, X. Li, M. N. Raphael and D. M. Holland, Rapid decline in Antarctic sea ice in recent years hints at future changes, Nat. Geosci., 2021, 14, 460–464, DOI:10.1038/S41561-021-00768-3.
- G. Negrete-García, N. S. Lovenduski, C. Hauri, K. M. Krumhardt and S. K. Lauvset, Sudden emergence of a shallow aragonite saturation horizon in the Southern Ocean, Nat. Clim. Change, 2019, 9, 313–317, DOI:10.1038/s41558-019-0418-8.
- J. A. Ericson, N. Hellessey, S. Kawaguchi, S. Nicol, P. D. Nichols, N. Hoem and P. Virtue, Adult Antarctic krill proves resilient in a simulated high CO2 ocean, Commun. Biol., 2018, 1, 190, DOI:10.1038/s42003-018-0195-3.
- S. Kawaguchi, H. Kurihara, R. King, L. Hale, T. Berli and J. P. Robinson,
et al., Will krill fare well under Southern Ocean acidification?, Biol. Lett., 2011, 7, 288–291, DOI:10.1098/rsbl.2010.0777.
- S. A. Morley, D. Abele, D. K. A. Barnes, C. A. Cárdenas, C. Cotté and J. Gutt,
et al., Global drivers on Southern Ocean ecosystems: Changing physical environments and anthropogenic pressure in an Earth system, Front. Mar. Sci., 2020, 7, 547188, DOI:10.3389/fmars.2020.547188.
- E. Rowland, T. Galloway, M. Cole, C. Lewis, V. Peck, S. Thorpe and C. Manno, The effects of combined ocean acidification and nanoplastic exposures on the embryonic development of Antarctic krill, Front. Mar. Sci., 2021, 8, 709763, DOI:10.3389/fmars.2021.709763.
- C. Manno, V. L. Peck, I. Corsi and E. Bergami, Under pressure: nanoplastics as a further stressor for sub-Antarctic pteropods already tackling ocean acidification, Mar. Pollut. Bull., 2022, 174, 113176, DOI:10.1016/j.marpolbul.2021.113176.
- S. Shimada, R. Nakai, K. Aoki, N. Shimoeda, G. Ohno and S. Kudoh,
et al., Chasing waterborne pathogens in Antarctic human-made and natural environments, with special reference to Legionella spp, Appl. Environ. Microbiol., 2021, 87, e02247, DOI:10.1128/aem.02247-20.
- P. Laganà, G. Caruso, I. Corsi, E. Bergami, V. Venuti and D. Majolino,
et al., Do plastics serve as a possible vector for the spread of antibiotic resistance? First insights from bacteria associated to a polystyrene piece from King George Island (Antarctica), Int. J. Hyg. Environ. Health, 2019, 222, 89–100, DOI:10.1016/j.ijheh.2018.08.009.
- T. Atugoda, M. Vithanage, H. Wijesekara, N. Bolan, A. K. Sarmah and M. S. Bank,
et al., Interactions between microplastics, pharmaceuticals and personal care products: Implications for vector transport, Environ. Int., 2021, 149, 106367, DOI:10.1016/j.envint.2020.106367.
- K. Lu, R. Qiao, H. An and Y. Zhang, Influence of microplastics on the accumulation and chronic toxic effects of cadmium in zebrafish (Danio rerio), Chemosphere, 2018, 202, 514–520, DOI:10.1016/j.chemosphere.2018.03.145.
|
This journal is © The Royal Society of Chemistry 2024 |