DOI:
10.1039/D4NA00474D
(Review Article)
Nanoscale Adv., 2024,
6, 4751-4780
Biopolymeric and lipid-based nanotechnological strategies for the design and development of novel mosquito repellent systems: recent advances
Received
8th June 2024
, Accepted 15th August 2024
First published on 22nd August 2024
Abstract
Mosquitoes are the most medically important arthropod vectors of several human diseases. These diseases are known to severely incapacitate and debilitate millions of people, resulting in countless loss of lives. Over the years, several measures have been put in place to control the transmission of mosquito-borne diseases, one of which is using repellents. Repellents are one of the most effective personal protective measures against mosquito-borne diseases. However, conventional delivery systems of repellents (e.g., creams, gels, and sprays) are plagued with toxicity and short-term efficacy issues. The application of biopolymeric and lipid-based systems has been explored over the years to develop better delivery systems for active pharmaceutical ingredients including mosquito repellents. These delivery systems (e.g., solid lipid micro/nanoparticles, micro/nanoemulsions, or liposomes) possess desirable properties such as high biocompatibility, versatility, and controlled/sustained drug delivery, and thus are very important in tackling the clinical challenges of conventional repellent systems. Their capability for controlled/sustained drug release has improved patient compliance as it removes the need for consistent reapplication of repellents. They can also be engineered to reduce repellents' skin permeation, consequently improving their safety. However, despite the benefits that these systems offer very few of them have been successfully translated to the global market for commercial use, a vital challenge that previous reports have not thoroughly examined. The issue of limited clinical translation of novel repellent systems is a vital aspect to consider, as the ultimate goal is to move these systems from bench to bedside. As such, this study seeks to highlight the recent advances in the use of biopolymeric and lipid-based systems for the development of novel mosquito-repellent systems and also analyze the challenges that have limited the clinical translation of these systems while proposing possible strategies to overcome these challenges.
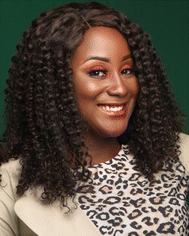 Chinekwu Nwagwu | Chinekwu Sherridan Nwagwu is a lecturer and researcher at the Department of Pharmaceutics, University of Nigeria, Nsukka. She holds a Master's degree in Pharmaceutics with a specialization in drug delivery and nanomedicine. Her research interests include the development of effective and targeted nanotherapeutics for infectious diseases and cancer. Chinekwu has received several prestigious awards, including the Otto Bayer Research Fellowship and the AGNES-PAWS Intra-Africa Mobility Grant. She continues to contribute to her field through research and international collaborations. |
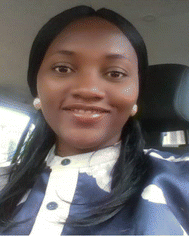 Adaeze Onugwu | Onugwu Adaeze is a senior lecturer at the Department of Pharmaceutics, University of Nigeria Nsukka. She is also a member of the Drug Delivery and Nanomedicines Research Laboratory at the same university. Adaeze is a Commonwealth scholar and was a visiting researcher at the University of Reading, United Kingdom. She obtained a master's degree and PhD in Pharmaceutics from the University of Nigeria in 2015 and 2023, respectively. Her research interests include nanotechnology, ocular drug delivery, vaccine delivery, cancer therapy, novel drug delivery systems and phytochemical/herbal medicine. Currently, she is seeking a post-doctoral position in the area of novel drug delivery systems. |
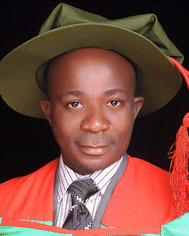 Frankline Kenechukwu | Franklin Chimaobi Kenechukwu obtained a PhD in Pharmaceutics from the University of Nigeria Nsukka, UNN (2017) where he graduated as the Overall Best Graduating PhD student. He is a Senior Lecturer and Researcher at UNN. His research profile (https://orcid.org/0000-0002-1619-4474) attests to his research independence as a young/upcoming scholar/researcher. With >150 research publications in top journals (https://scholar.google.com/citations?user=s87G-ucAAAAJ%26hl=en), his research undertakings have earned him many academic awards across the globe. He was recently rated as one of the top 500 most influencial researchers by Scholarly Output in Nigeria (https://www.scival.com/overview/authors?uri=Country/566). Kenechukwu belongs to many prestigious professional bodies. He has won several research and travel grants/awards/scholarships. |
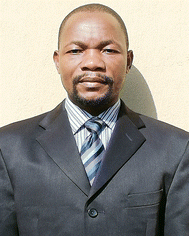 Clemence Tarirai | Prof Clemence Tarirai is an Associate Professor of Pharmaceutical Sciences in the Faculty of Science at the Tshwane University of Technology (TUT). He holds a Bachelor's of Pharmacy degree (Medunsa/TUT), a Master's of Technology in Pharmaceutical Sciences degree (TUT), a Master's of Public Health degree (UWC), and a Doctor of Technology in Pharmaceutical Sciences degree (TUT). Additionally, he received postdoctorate training in nanomedicine manufacturing at the University of Michigan, USA. Prof. Tarirai has expertise in pharmaceutical sciences, i.e. biopharmaceutics and pharmaceutics, nanotechnology, immunology, pharmacological sciences, biotechnology and food science technology, public health, research ethics, and pre-clinical studies. |
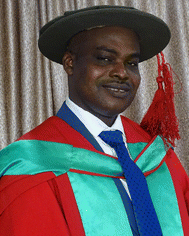 Kenneth Ofokansi | Professor Ofokansi holds Bachelor of Pharmacy, Master of Pharmacy, and PhD degrees from the University of Nigeria, Nsukka. He was a postdoctoral Research Fellow at Ludwig-Maximilians University, Munich, Germany and at Ruprechts-Karl University, Heidelberg, Germany between March 2007 and April 2009. An erudite scholar, Prof. Ofokansi has over 100 scholarly articles in reputable local and international peer-reviewed journals with more than 1500 citations, an i-10 index of 37 and an h-index of 22. He has served in various departmental, faculty and university committees and has supervised more than twenty-five postgraduate students at Master's and doctoral levels. He is currently the Director of Lion Science Park, University of Nigeria, Nsukka and has expertise in the formulation of polymer-based nano- and micro-particulate drug delivery systems, novel controlled delivery of various active pharmaceutical ingredients, targeted drug delivery and formulation and delivery of proteins and peptide-based drugs, transdermal drug delivery systems, solid dispersions and lipid-based colloidal delivery systems. |
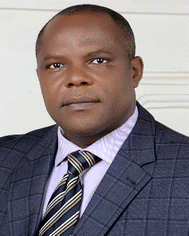 Anthony Attama | Anthony A. Attama is a Professor of Pharmaceutics at the University of Nigeria, Nsukka, where he is the Director, Institute for Drug-Herbal Medicine-Excipient Research and Development and the Founder and Research Lead, Drug Delivery and Nanomedicines Research Laboratory. He was an Alexander von Humboldt postdoctoral researcher at Technische Universitat Braunschweig, Germany and a Visiting Professor at Hebrew University of Jerusalem, Israel. He has researched extensively on nano drug delivery systems for oral, parenteral, dermal/transdermal and ocular applications for the treatment of infectious and non-infectious diseases. Prof. Attama has won many research grants and awards. He is a fellow of the Nigerian Academy of Science (FAS) and West African Postgraduate College of Pharmacists (FPCPharm.). |
1 Introduction
Mosquitoes represent the most important vectors of human as well as veterinary diseases. The prevalence of mosquito-borne diseases affects millions of people annually, causing approximately 1
000
000 deaths worldwide.1 Mosquitoes have also been implicated in transmitting viruses causing several infections such as dengue, yellow fever, lymphatic filariasis, chikungunya, and Japanese encephalitis among a host of others2,3 (Fig. 1). In addition to their unparalleled ability to transmit various deadly diseases, mosquito bites have been reported to cause allergic reactions with symptoms ranging from moderate to severe pruritus, edema and rashes.3 The absence of commercially available vaccines for many mosquito-transmitted diseases such as malaria has made the tool of vector control very important in the prevention of disease transmission, as effective vector control programs have the potential to improve overall human health.1
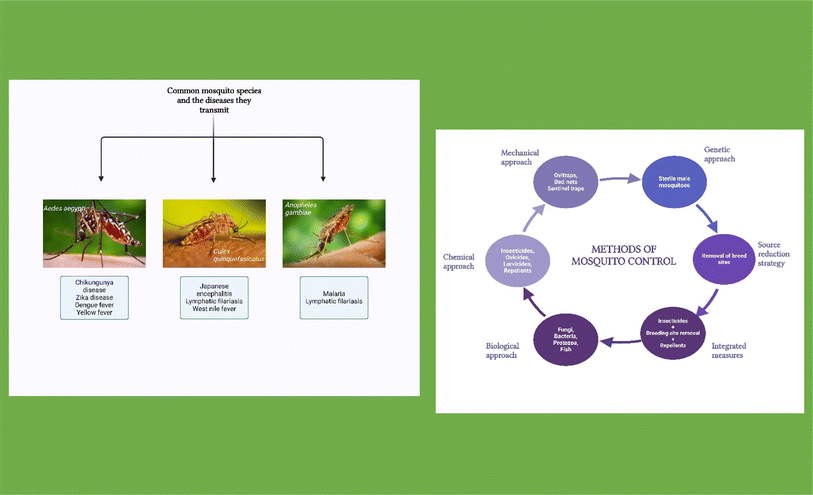 |
| Fig. 1 Common mosquito species, diseases they transmit and general methods of mosquito control (created with BioRender). | |
Although several tools and methods have been developed over the years for the control and prevention of mosquito-transmitted diseases (Fig. 1), certain limitations have made these methods ineffective. For instance, despite the diverse public health strategies, which predominantly target population reduction of mosquitoes, certain unique abilities of mosquitoes such as ubiquity, stemming from their great population and reproductive capacity, genomic flexibility, and the ability to undergo metamorphosis, have indeed made them very difficult to eradicate.4,5 The source reduction approach (Fig. 1), which involves eliminating mosquito breeding sites, is one of the most common strategies employed over decades but it is ineffective, labor-intensive, costly, and detrimental to maintaining the balance of natural ecosystems.3,5 Also, many breeding sites go unnoticed and it is almost impossible to eliminate mosquitoes from locations close to forests and heavily vegetated areas as materials such as leaf litter could very well suffice as breeding grounds for mosquitoes. In addition, the issue of climate change which is continuously expanding the hot and humid areas of the planet has further increased the population of these vectors as this has provided more conducive environments for mosquito multiplication as well as the emergence of various mosquito-borne diseases.1,5 Several mechanical approaches such as trapping have also been extensively used to control the rapid multiplication of mosquitoes (Fig. 1). This involves the use of a wide range of techniques such as ovitraps which aim to destroy eggs laid by mosquitoes, bed net traps, sentinel traps, and light and chemical-based traps.5 Despite the success obtained with this approach, it is largely inadequate, especially for exophilic mosquitoes like the Aedes albopictus.5 The application of synthetic chemical compounds such as organophosphates and organochlorides has been extensively employed in the past as a major tool in mosquito control as adulticides, ovicides, and larvicides. However, their use has been associated with the destruction of non-target organisms thereby disrupting the environment.5 More so, the development of resistance to these substances by the increasing mosquito population has continued to threaten the efficacy of the approach.3,6 The need to develop mosquito control techniques that are more eco-friendly and safer for humans brought about extensive interest in developing effective biological methods for mosquito control as certain organisms such as bacteria, fungi, viruses, protozoa, and even fish have been explored as bio-insecticides for mosquitoes.3,6 The fact that there is a limited supply of these organisms has placed a huge limitation on the use of these organisms on a commercial scale for mosquito control. Several novel biotechnological approaches have been developed in a bid to limit the population of mosquitoes. These include the use of sterile genetically modified male mosquito species, the use of attractive sugar, baits, and auto-dissemination of hormone mimetics.5,6 Despite the benefits that result from these approaches, certain factors such as high cost, toxicity issues especially for other non-target organisms, public skepticism, and other logistical deployment hurdles continue to pose a challenge to the commercial deployment of these tools of mosquito control.6 Most recently, integrated measures of mosquito control which involve a combination of the aforementioned approaches simultaneously have been advocated. This usually involves the simultaneous eradication of breeding sites, use of mosquito traps, indoor residual spraying (IRS), long-lasting insecticide-treated nets (LLITNs), and sterile insect techniques.4,5,7 However, the awareness of the toxic effects of synthetic chemical compounds involved in these processes on both humans and the environment at large is a huge drawback to this method of control.3
These numerous limitations of the various public strategies of mosquito control have promoted overall increased interest in the development of personal protective measures such as indoor residual-spraying (IRS), long-lasting insecticide-treated nets (LLITNs), and the use of repellents against mosquito bites. The use of IRS and LLITNs has been very useful in preventing mosquito bites especially indoors, but is largely inefficient against outdoor bites, hence the need for developing effective as well as safe repellents.8,9 The use of mosquito repellents, which are substances that induce mosquitoes to move in the opposite direction away from their target, is one of the various strategies that have been employed over the years in the control of mosquitoes.4 These substances work by providing a vapor barrier, deterring the mosquito from coming into contact with the skin surface.3 Despite the advantages that these systems provide, toxicity problems associated with synthetic repellents, which range from mild effects such as urticaria and skin eruptions to severe effects such as encephalopathy as well as their ability to damage plastics and synthetic fabrics, have greatly discouraged their use.7,10 To ameliorate these problems, natural alternatives (e.g. essential oils) are being explored as they are considered safer and more environmentally friendly. However, the problem of short protection time against mosquito bites as a result of their high volatility poses a huge challenge to their use.10,11 Consequently, strategies such as the use of novel biopolymeric and lipid-based drug delivery systems have been explored to reduce the toxicity of synthetic repellents and promote the repellent activity of volatile oils and other natural repellents.10,12,13 These systems have played a vital role in the design and development of wearable repellents.14,15
Several reviews have been published on the recent discoveries and development of mosquito repellents.2,3,9,11 One such study critically examined newly discovered mosquito-repellent compounds.3 A more recent report considered the use of nanomaterials for the design of controlled-release formulations for essential oils as a tool to prevent mosquito-borne diseases.11 Our review however focuses on the use of biopolymeric and lipid-based systems, highlighting their potential to be used for the development of novel delivery systems for both synthetic as well natural repellents. We also consider the contribution of these systems in the development of safer and more eco-friendly repellent systems. In addition, our study also critically describes more recent delivery systems as well as several strategies for the design and characterization of these systems. More importantly, despite the vast amount of research conducted in this area and many potentially useful formulations and repellent systems that have been developed especially in the last three decades, very few of these formulations have been successfully translated to commercial use. A recent study by Campos et al.2 evaluated the various nanotechnological platforms applied in the detection and control of mosquitoes. However, the study like many other reviews in this area did not address the challenges responsible for the limited clinical translation of these systems.2 Therefore, in addition to examining recent advances in the use of biopolymeric and lipid-based systems for the delivery of mosquito repellents as other similar reviews have done in the past, this study also seeks to discuss the intricate process of development and evaluation of these novel repellent systems. This study also critically examines the challenges that have limited the clinical translation of these systems from bench to bedside and proposes possible ways to tackle these challenges.
2 Overview of mosquito repellents
Studies have established that mosquitoes are anthropophilic and that only female mosquitoes bite, while male mosquitoes feed majorly on the nectar of flowers. This is because female mosquitoes obtain energy from blood meals for the production of eggs.16,17 Female mosquitoes, although greatly influenced by species variations, have an uncanny ability to differentiate host odors from multiple odors. This is largely because they possess diverse transmembrane odorant receptor proteins, located in the olfactory membrane neurons.16 The process of host identification involves several intricate behavioral steps, the first of which is the activation of the odorant receptor. The mosquito then proceeds to navigate the odor plume using olfactory cues and this is then followed by a close-range movement towards the skin and finally landing on the skin for a bite.3,16 As shown in Fig. 2, factors such as exhaled carbon dioxide (CO2) from the nostrils and skin emanations, sweat gland outputs, and the activities of certain microorganisms such as the Corynebacterium in the skin, have been reported to attract mosquitoes.18 Reports also show that mosquitoes are more attracted to men than women and more attracted to adults than children.16,18 Other non-olfactory factors such as humidity, visual stimuli, movements, and temperature have also been observed to affect the host-seeking behavior of mosquitoes. Repellents elicit their actions by providing a vapor barrier that either masks human scents or generates an odor that repels mosquitoes.18 An ideal repellent (Fig. 2) should be cost-effective, chemically stable, non-toxic, non-irritant to the skin, and have no adverse effects on non-target organisms. It should effectively repel diverse insect species for reasonably long periods.
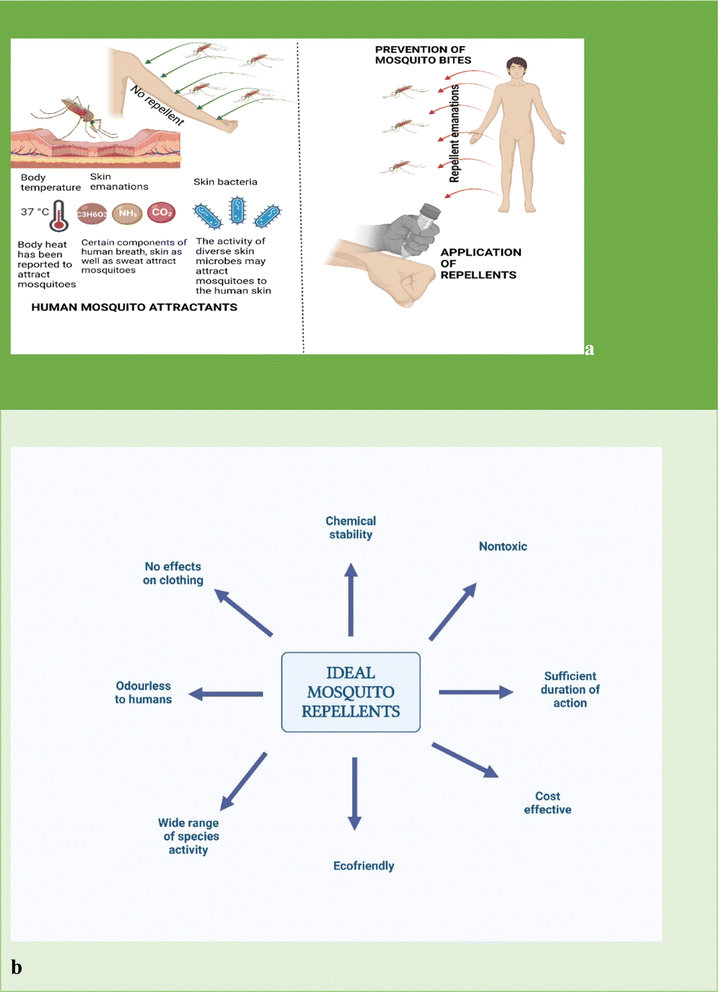 |
| Fig. 2 (a) Role of repellents in the prevention of disease transmission; (b) properties of an ideal repellent (created with BioRender). | |
2.1 Classes of repellents
Based on their chemical nature, repellents are classified into two classes, as follows.
2.1.1 Synthetic repellents.
Synthetic repellents represent a major tool for the control of mosquito-transmitted diseases. N,N-diethyl-3-methyl benzamide (DEET), a synthetic chemical compound, is considered the gold standard of repellents due to its broad-spectrum efficacy. It is an oily, volatile, slightly yellowish liquid at room temperature.19–21 Several studies propose that DEET repels insects by binding to the olfactory receptors and neurons of insects, thus providing a vapor barrier that consequently deters the insects from coming into contact with the human skin.19–21 This compound, similar to some other synthetic repellents, has been reported to have lower efficacy against several anopheline species, and as such is rarely used in tropical rural communities for the prevention of malaria.22 In addition, the safety concerns associated with the use of DEET have also limited its use. Toxicity concerns ranging from mild irritations to severe effects such as dermatitis, allergic reactions, and encephalopathy, especially in children, have been reported particularly in situations involving long-term and repeated application of DEET.23 Its low molecular weight and oily nature promote a high level of cutaneous absorption and hence the compound alone should not be applied directly to the skin.19–21 These safety issues necessitate the design and development of novel delivery strategies to ensure safer and more effective repellent systems.
Ethyl butylacetylaminopropionate (IR3535), developed by Merck, is an effective repellent with a wide range of effects against mosquitoes, flies, wasps, bees and ticks.24 Although its repellent action has been reported to be lower than that of DEET, IR3535 is employed in developing repellent formulations for children and pregnant women. This is because it is more biocompatible and has a lower toxicity profile compared to DEET.24
Icaridin, chemically known as 1-piperidine carboxylic acid 2-(2-hydroxyethyl)-1-methyl propyl ester, developed by Bayer, is also a synthetic repellent, which possesses a wide range of activities against mosquitoes, flies, fleas and bees.21,24 Because it evaporates slowly from the skin surface, it is reported to have a longer duration of action than DEET.24 Studies have also reported that it is more effective for Aedes species compared to DEET. In addition, unlike DEET, icaridin is non-greasy, odorless to humans, and also does not destroy plastics.24 Another major synthetic repellent is diethyl phenyl acetamide (DEPA), a long-acting repellent with over eight hours of action and it offers protection not just against mosquito bites but also black flies, land leeches, and phlebotomine sand flies.24 Permethrin although classified as a pyrethroid pesticide has been employed extensively in mosquito control programs. It is usually used to treat clothes and other materials worn outdoors. On exposure to this compound, insects are either repelled or killed, depending on the concentration of permethrin on the fabric.21,24 This compound, on the other hand, is vastly implicated in the precipitation of several environmental challenges since it contaminates groundwater, soil, and plants.24 Another challenge associated with the use of synthetic repellents like permethrin is the problem of emerging resistance against these compounds.18 As a result of this, there has been a rapid increase in research to develop novel delivery strategies for these synthetic repellents to improve their safety while some others are focused on exploring and developing natural repellents as safer and more effective alternatives.
2.1.2 Natural repellents.
Substances of natural origin especially plant-based materials have long been used to repel or kill medically important arthropods even before the advent of synthetic chemicals.24 These materials have been used indigenously either by hanging the plant materials inside houses or burning the materials to generate smoke which drives away blood-sucking insects. The presence of secondary metabolites in natural materials especially those of plant origin which include diverse compounds such as phenols, terpenes, quinones lactones, and alkaloids, makes them important sources of natural repellents.18 The repellent effect of essential oils from some aromatic plants such as Acantholippia seriphioides, Achyrocline satureioides, Aloysia citriodora, Anemia tomentosa, Baccharis spartioides, neem (Azadirachta indica, A. Juss), citronella grass (Cymbopogon nardus Rendle), basil (Ocimum gratissimum L., Ocimum americanum L.), clove (Syzygium aromaticum L), prickly straggler (Solanum trilobatum L.), musk basil (Moschosma polystachyum L.) and thyme (Thymus vulgaris L.) has been investigated against various mosquitoes species over the years.25,26 Several reports propose that essential oil-based repellents provide both effective and eco-friendly protection against mosquito bites and other blood-sucking insects.18,27,28 In addition, because plant-based repellents have been reported to consist of diverse chemical compounds compared to synthetic repellents, they are more complex thereby making difficult the development of resistance to these compounds.18,29 However, the high volatility of most of these substances causes them to have a short duration of action thus necessitating continuous and repeated application of the oils to ensure adequate protection from mosquito bites. As a result, several nanotechnological strategies have been utilized to improve the efficacy of these substances.
3 Common bio-polymers used in the development of mosquito repellents
3.1 Alginates
Alginate is a natural polysaccharide found in brown seaweed. It is a polyanionic linear biopolymer composed of α-L-guluronic acid and β-D-mannuronic acid blocks. This biopolymer has found extensive use in the development of various novel drug delivery systems such as nanoparticles, nanostructured lipid carriers, and micro/nanocapsules, to mention a few. This is primarily because of its ability to bond with divalent cations thereby forming complexes.30 It is also renowned for its biocompatibility, non-toxicity, and biodegradability. All these features as well as its ability to provide prolonged release of drugs have led to its use in the delivery of repellents. Oliveira et al.30 developed an alginate/cashew gum nanoparticle for essential oil encapsulation via spray drying.30 The resulting nanoparticles developed were found to have average sizes in the range 223–399 nm, zeta potential values ranging from −30 to −36 mV, and relatively good encapsulation efficiency (55%). The data from the in vitro drug release shows up to 95% release of the encapsulated oil over 50 h. This strongly suggests that the resulting nanoparticles were able to prolong the duration of release of the essential oil, thus providing a possible tool for designing more effective repellent systems. Rakkiyappan et al.31 designed DEPA-loaded calcium alginate microspheres via the emulsion-gelation technique. The microspheres gave an encapsulation efficiency of 70% and were also able to cause a prolonged release of DEPA for up to 5 h.31 Xiao also modified calcium alginate beads containing DEET via graft polymerization with a vinyl monomer. This study revealed that the release of DEET from the modified bead was slower and more prolonged compared to that from the unmodified beads.32 In addition to the development of repellent formulations, alginates have also been extensively used in the design of effective mosquito-repellent fabrics. Sumithra,33via gelation, developed sodium alginate microcapsules encapsulating plant essential oils which were fixed on fabrics by the pad-dry-cure method. It was observed that the sodium alginate microcapsules were able to extend the repellent activity of the essential oil by maintaining 52% repellence after 25 industrial washes.33 A chitosan–alginate assembly was also explored by Soroh and colleagues for the microencapsulation of essential oils for functional coatings for textiles. The developed textiles demonstrated 71.43% repellence compared to 52.94% repellence of conventional essential oil-impregnated cotton. The fabrics in addition to stellar repellent properties also showed anti-microbial effects on S. aureus, E. coli, S. epidermidis, and Ps. aeruginosa.34 The biocompatibility, cost-effectiveness, and versatility of this biopolymer make it very useful in the design of repellents as it can be used for designing both repellent formulations as well as wearable repellent systems.
3.2 Cellulose
This biopolymer is considered the most abundant natural polymer. It is a vital structural component of the primary cell wall of green plants, some algae and oomycetes. Its availability and biodegradability have brought about its extensive use in various research areas. Kadam et al. (2019) developed sustained-release repellent microcapsules with modified cellulose nanofibers. The study showed these systems provided very high encapsulation efficiency for DEET (98%) as well as an extended-release rate for the drug.35 Rogerio et al.36 designed cellulose hydrogels containing geraniol and icaridin encapsulated in zein nanoparticles as a tool for mosquito control. These nanoparticles showed 85% encapsulation efficiency for icaridin and 98% for geraniol. The drug release kinetics for the various formulations showed prolonged release of the active ingredients as well as a remarkable safety profile as the study recorded no DNA or mitochondrial damage.36 Ethyl cellulose was used to encapsulate limonene and permethrin via coacervation in a study by Türkoğlu et al.37 It was then applied on a piece of cotton fabric and the repellent activity against Culex pipiens was evaluated. The micro/nanocapsules were spherical with smooth surfaces. The laser diffraction analysis also showed a homogeneous size distribution of 1 and 1.3 μm for permethrin and limonene formulations respectively. The fabrics were reported to still maintain a remarkable level of repellence even after 20 washing cycles.37 As such, cellulose aids in the development of delivery systems that not only prolong the duration of action of natural repellents but that of synthetic repellents as well and can also be utilized in designing systems for simultaneous delivery of these repellents. In addition, these studies suggest that they can also be used to create safer and more biocompatible repellent systems.
3.3 Chitosan
After cellulose, chitosan is the second most abundant biopolymer. It is a linear polysaccharide, consisting of randomly distributed β-linked-D-glucosamine and N-acetyl-D-glucosamine.38 It is made by the deacetylation of chitin obtained from shells of shrimps as well as other crustaceans (Fig. 3). Its bioadhesive properties, biocompatibility, and biodegradability have encouraged its widespread application in the design of drug delivery systems over the years.38 A study by Maji and Hussain (2008), aimed at developing a genipin crosslinked chitosan–gelatin complex for encapsulating Zanthoxylum limonella oil, revealed that the chitosan–gelatin complex was able to prolong the release of Zanthoxylum limonella oil for up to 70 h.39 As such, this system could revolutionize the development of repellents as the need for frequent reapplication of formulations will be minimized, thus improving patient compliance and disease prevention. Singh and Sheikh (2021) were also able to design multifunctional linen finished with chitosan–gelatin microcapsules loaded with cinnamon oil. The microcapsules offered significant encapsulation efficiency (78.67%) and the scanning electron microscopy images revealed that the microcapsules were spherical with irregular surfaces. Also, the fabric showed excellent mosquito-repellent properties (100% repellence) even after 20 washes. In addition, the fabric also showed antibacterial properties against S. aureus and E. coli.40 Hence, it could be utilized as a protective textile against mosquito-borne diseases as well as a wide range of other microbial infections. The authors also developed a linen fabric for diverse applications using chitosan phosphate microcapsules loaded with Thymus vulgaris oil. The finished fabric showed excellent antibacterial properties (>98%), antioxidant activity (96%), flame retardancy (LOI > 28), and excellent mosquito repellence (100%) after 20 washes.41 Bano (2014) employed chitosan as a repellent binder for cotton textiles using the pad-dry-bake technique, which made the textile able to maintain 100% repellence even after washing.42 Kala et al. (2019) formulated chitosan nanocapsules containing lemon grass (Cymbopogon citratus) oil which was then transformed into gels and impregnated on fabrics to achieve long-lasting and wash-durable mosquito repellence. The chitosan-acrylate nanogel-treated fabric presented significantly high mosquito repellence (75%) after 15 washes compared to the untreated fabric (51%). There were also no signs of dermal toxicity as a result of the use of the fabric and as such it represents a potential tool in the prevention of mosquito-transmitted diseases.43 These highlight the pivotal role of chitosan in the development of novel repellent formulations as well as devices.
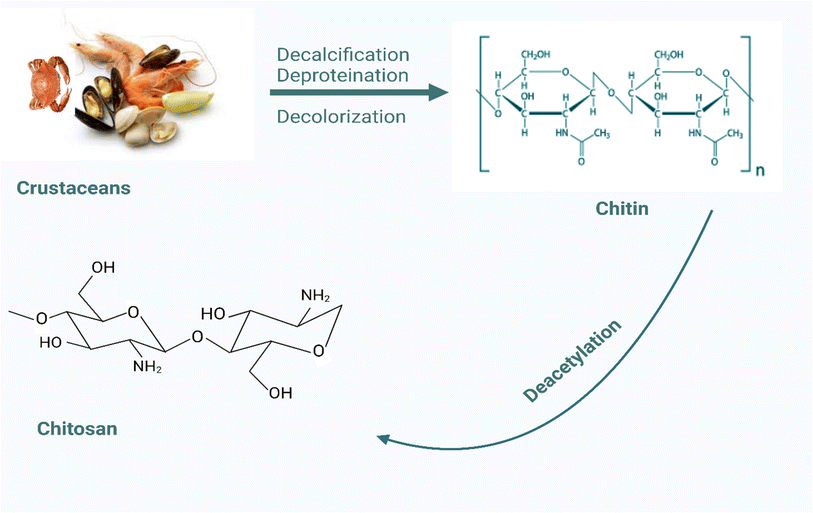 |
| Fig. 3 Process of chitosan extraction. | |
3.4 Gelatin
Gelatin is obtained by partial hydrolysis of collagen, which is a major protein component in skin, bones, hides, cartilage, and other connective tissues in animals. Gelatin is broadly classified into type A and type B, with type A being produced by acid processing of collagenous materials and type B being produced by alkaline processing. Gelatin is one of the most commonly used biopolymers in the design of drug delivery systems because it is cost-effective, biodegradable, and also biocompatible. It has also been widely applied in the design of controlled-release delivery systems for diverse drugs. Solomon et al.,44 using gelatin and formaldehyde as the crosslinking agent, were able to develop microcapsules of citronella oil. The study showed that the encapsulation efficiency of the microcapsules was about 66% and the microcapsules were able to prolong the release of citronella oil for 8 h.44 Teli and Chavan45 evaluated the repellent activity of gelatin-based microcapsules applied to cellulosic biopolymers. The microcapsules were dried in three different ways with those dried by freeze drying having the highest encapsulating efficiency (95.37%). The fabric treated with the microcapsules showed a repellence of 89% compared to 61.38%, 57.65%, and 49.25% for freeze-dried, spray, and oven-dried microcapsules respectively.45 These findings thus suggest that gelatin possesses suitable features for the development of novel repellent systems.
3.5 Natural gums
Natural gums such as gum arabic, tragacanth, xanthan gum, pectin, and gum karaya, to mention a few, have been used as pharmaceutical excipients as well as for the design of novel dosage forms for many decades.46 These are mainly composed of polysaccharides obtained as plant exudates. These biopolymers possess the ability to hydrate and form gels when placed in water. A combination of gelatin and gum arabic was employed in the development of microcapsules for encapsulating the repellent geraniol via the complex coacervation process by Ogilvie-Battersby et al.47 The geraniol contents of the microcapsules were found to be 90%, 68%, and 73% for mixing rates of 700 rpm, 1000 rpm, and 1300 rpm. The study, therefore, showed longer-lasting retention of geraniol compared to conventional systems.47 The release data from the study showed that these capsules brought about a steady release of the repellent (geraniol) without any burst effect and prolonged its release for about 26 days. This remarkably prolonged duration of release makes these systems potentially useful not only for designing topical repellent formulations but also in the design of repellent clothing as well as other repellent devices. Boominathan et al.46 also developed microcapsules using acacia gum as shell materials and Coleus aromaticus leaf extract as the core. This system was used as a finishing on the cotton fabric as an antibacterial and repellent tool and showed excellent repellent activities (up to 99.4%).46 Although the study did not present data to describe the release of the essential oil from the microcapsules, it did however show that natural gums can be very useful in designing novel delivery systems for repellent substances.
4 Repellent systems
4.1 Classical/conventional repellent systems
Conventional repellent systems are traditional dosage forms employed in the delivery of repellents. These include sprays, lotions, creams, and gels among others (Table 1). Of all these pharmaceutical forms, the spray is the most common and is available commercially as a spray pump or spray with propellant gas.48,49 Permethrin is commonly formulated as a spray for application on clothing and other inanimate objects at a very low concentration of <2%. Sprays are suitable for application to large areas and spray solutions contain a high amount of ethanol and propylene glycol used as co-solvents to solubilize repellents. This may sometimes cause a burning sensation and dryness on application to the skin.48 Furthermore, ethanol is a renowned permeation enhancer,48 employed in diverse formulations to improve the delivery of drugs transdermally. This poses a huge challenge to the use of sprays for the delivery of repellents especially synthetic repellents as improved permeation of these substances can bring about toxic effects in the body. Lotions in the form of a solution, suspension, or emulsion for topical application are also common delivery systems for both natural and synthetic repellents. When applied to the skin, lotions give a cooling sensation on evaporation. They have fast-spreading properties and consequently a low occlusive effect.48 However, repellents have been known to rapidly evaporate from these systems, resulting in the need for constant reapplication to keep mosquitoes away. Creams and gels offer the advantages of easy application and good skin adhesion. They are more viscous than the other conventional forms. This means that they are less prone to cause skin dryness and are suitable for application on specific areas of the body. They offer high protection at the application sites. The synthetic repellent, picaridin/icaridin, is presented in these forms at 10–30% concentration.
Table 1 Some classical mosquito repellent formulations with the pharmaceutical form, the concentration of repellent, action time, and manufacturer
Product form |
Repellent |
Conc. of repellent (%) |
Duration of action |
Manufacturer |
Lotion |
DEET |
10 |
NA |
Sun Pharmaceutical Corp |
IR 3535 |
10 |
8 h |
EMD Millipore Corporation |
12.5 |
4 h |
S.C. Johnson & Son, Inc. |
Picaridin/icaridin |
20 |
14 h |
Sawyer Products |
Citronella oil |
4.2 |
1 h |
Tender Corp. |
Catnip oil |
7 & 15 |
7 h |
DuPont |
Lemon eucalyptus oil |
10 |
2 h |
S. C. Johnson & Son, Inc. |
30 |
6 h |
Citrefine International Ltd |
Citronella/Rosemary |
4.2 |
1 h |
W.F. Young Inc. |
Pump spray |
DEET |
98.11 |
10 h |
Spectrum |
7 |
2 h |
S. C. Johnson & Son, Inc. |
IR 3535 |
30 |
7 h |
ISDIN |
Picaridin/icaridin |
10 |
5 h |
Reckitt Benckiser Group PLC |
25 |
8 h |
Reckitt Benckiser Group PLC |
Permethin |
0.5 |
2 weeks |
3M |
Citronella oil |
5 |
2.5 h |
Quantum Inc. |
Citronella/Rosemary |
10 |
2 h |
Nano Vectors Technology S.A. |
Geraniol |
5 |
8 h |
TyraTech Inc. |
Oil of lemon eucalyptus |
40 |
6 h |
United Industries Corp. |
Spray with propellant gas |
IR 3535 |
12.5 |
4 h |
Sun Lau |
DEPA |
20 |
8 h |
Shinathji Chemicals |
Picaridin/icaridin |
20 |
10 h |
Rejet |
Permethin |
0.5 |
5 weeks |
Sawyer |
Cream |
DEPA |
20 |
8 h |
Shinathji Chemicals |
Picaridin/icaridin |
20 |
14 h |
S. C. Johnson & Son, Inc. |
Citronella/rosemary |
10 |
2 h |
Nano Vectors Technology S.A. |
Gel |
Picaridin/icaridin |
10.6 |
5 h |
Reckitt Benckiser Group PLC |
20 |
10 h |
Osler |
Also, natural repellents, such as citronella essential oil, lemon eucalyptus, geraniol, and lemongrass oil, have been delivered using various conventional dosage forms. These dosage forms usually contain one or a combination of these essential oils for synergistic activity.48,50 However, the volatility of essential oils as mosquito repellents is largely responsible for their short duration of action especially when delivered in traditional pharmaceutical dosage forms. Moreover, free-repellent molecules in some classical formulations can easily permeate the skin and be absorbed into the systemic circulation leading to adverse effects.24,50 To overcome these problems of classical formulations, novel biopolymeric and lipid-based formulations are being investigated as alternative delivery systems for mosquito repellents to create safer and more effective systems. Some classical mosquito repellent formulations that are commercially available are listed in Table 1.
4.2 Novel biopolymeric and lipid-based strategies for the development of repellent systems
Biopolymeric and lipid-based drug delivery systems are composed of a diverse group of formulations. These systems possess different properties, which are usually a result of the differences in the nature of the lipids or polymers (Table 2) as well as other excipients that they contain. They provide industrially viable tools for the development of challenging active ingredients such as repellents and have been engineered for use in a wide range of pharmaceutical products. Some of the systems under study are polymeric microcapsules, solid lipid micro and nanoparticles, micro and nanoemulsions, nanostructured lipid carriers, liposomes, polymeric micelles, niosomes, porous polymeric nanomaterials, cyclodextrins, and nanogels. These systems can decrease the rate of evaporation of repellents from the skin surface thus prolonging their duration of repellence activity (Fig. 4). As illustrated in Fig. 4, they can also limit skin penetration of repellents thus reducing the occurrence of adverse effects.44,48,63 They also possess versatile and remarkable characteristics that can be employed in the development of wearable repellents.
Table 2 Common biopolymers and lipids used in the development of novel repellent systems
Classes |
Example(s) |
Repellent substance(s) |
Delivery system(s) |
References |
Natural biopolymers |
Gelatin |
Citronella oil |
Microcapsules |
51
|
Gelatin + Cellulose |
Citronella oil |
Microcapsules |
45
|
Natural gum (acacia gum) |
Coleus aromaticus extract |
Microcapsules |
46
|
Alginate |
Geraniol |
Microcapsules |
13
|
Cellulose |
DEET |
Nanofibers |
35
|
Cellulose |
Icaridin |
Microcapsules |
36
|
Geraniol |
Chitosan + gelatin |
Zanthoxylum limonella oil |
Microcapsules |
39
|
Chitosan + gelatin |
Cinnamon oil |
Microcapsules |
40
|
Chitosan |
Thymus vulgaris oil |
Microcapsules |
41
|
β-Cyclodextrin |
Citronella oil |
Inclusion complex |
52
|
Semi-synthetic biopolymers |
Carboxymethyl cellulose |
Alpinia galanga oil, Citrus grandis oil, Citrus aurantifolia oil, DEET |
Microcapsules |
48
|
Carboxymethyl cellulose |
Carvacrol, cinnamaldehyde, thymol |
Nanogels |
53
|
Calcium-alginate |
DEPA |
Microspheres |
31
|
Calcium-alginate |
DEET |
Microbeads |
32
|
Chitosan-acrylate |
Lemon grass oil |
Nanogels |
43
|
Ethyl cellulose |
Limonene |
Microcapsules |
54
|
Permethin |
Synthetic biopolymers |
Polyvinyl alcohol |
p-Menthane-3-8-diol |
Nanofibers |
55
|
Permethin, chilli and catnip oil |
Polylactic acid |
Lemon grass oil |
Microcapsules |
56
|
Bergamot oil, turmeric oil, galangal oil |
Basil oil |
Poly(ε-caprolactone) |
Citronella oil |
Microcapsules |
57
|
Poly(ε-caprolactone) |
IR3535 |
Polymeric nanoparticles |
58
|
Geraniol |
Poly(ε-caprolactone) |
Betel essential oil |
Nanocapsules |
59
|
Waxes |
Beeswax |
Nutmeg extract |
Microcapsules |
60
|
Langsat |
Beeswax |
Holy basil oil |
Microcapsules |
61
|
Carnuba wax |
Clove oil |
Nanostructured lipid carriers |
62
|
Eugenol |
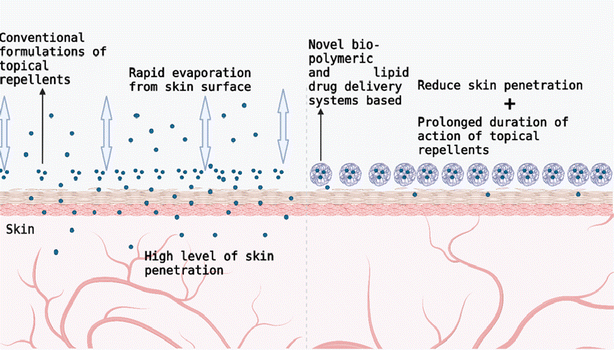 |
| Fig. 4 Benefits of novel bio-polymeric and lipid-based drug delivery systems in the development of topical repellent systems (created with BioRender). | |
4.2.1 Polymeric micro/nanocapsules.
About 45% of novel polymeric systems for repellent formulations described in the literature are polymeric micro/nanocapsules.13,24 Polymeric micro/nanocapsules are systems that consist of the active compound in tiny particles or droplets as the core material surrounded by a uniform layer of polymeric material (Fig. 5). Natural and biodegradable polymers such as gelatin, chitosan, alginate, and gum arabic are commonly used as shell materials in the formulation of microcapsules. The polymeric material limits the diffusion of the repellent encapsulated in the core thereby providing controlled release and long-lasting activity of the repellent substance.64 On application to the skin, there is disruption of the microcapsule leading to diffusion and volatilization of the repellent from the matrix.65 Some benefits of microencapsulation include an increase in the duration of repellence, a decrease in skin permeation, and protection of the active ingredient from environmental degradation.5,40,66 The polymers used in the formulation process are readily available, biocompatible, and biodegradable and as such provide a possible strategy for industrial scale-up. Several methods have been used to prepare biopolymeric microcapsules for the delivery of repellents. However, coacervation13,44,67 and spray drying methods are the most widely used in the microencapsulation of mosquito repellents.41,66,68 Misni et al.48 investigated the repellence effect of natural and synthetic repellents encapsulated in carboxymethylcellulose microcapsules. The natural repellent compounds used were essential oils from Alpinia galanga, Citrus grandis, and Citrus aurantifolia, while the synthetic repellent used was DEET. The microencapsulated formulations demonstrated a repellence effect of over 98% lasting 4 h against Culex quinquefasciatus. Formulations containing microencapsulated essential oil had a 100% repellent effect which lasted longer than the formulations of free and commercial citronella essential oil.48 The study suggests that the microencapsulated formulations could serve as a more effective alternative to commercially available repellents. They also provide a delivery system that could simultaneously deliver both synthetic and natural repellents (DEET and essential oils) and as such could be very useful, especially in the prevention of bites from mosquitoes that have developed resistance to DEET.
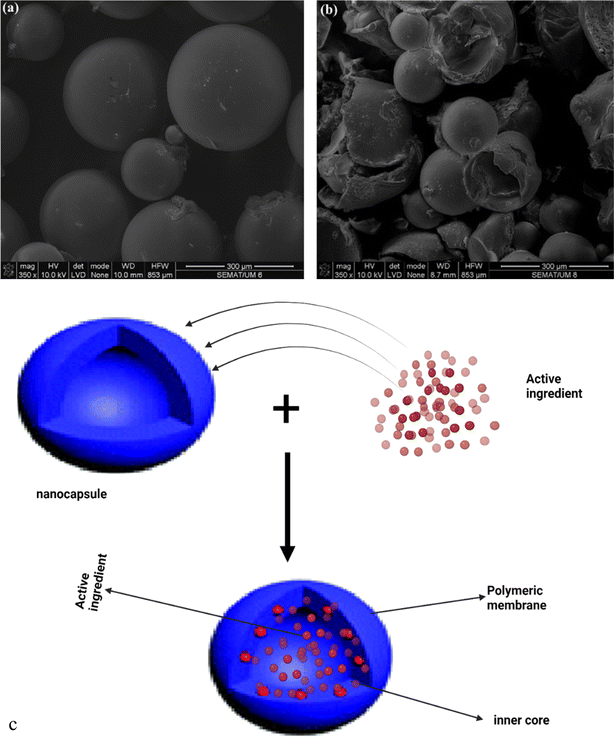 |
| Fig. 5 Scanning electron microscopy micrographs of citronella-loaded polyurethane microcapsules (a) before and (b) after mechanical stress. Reproduced from ref. 50 with permission from Elsevier, copyright 2016. (c) Schematic representation of the structure and drug loading processes for micro/nanocapsules (created with BioRender). | |
Several studies have reported that the evaporation rate of repellents plays a huge role in determining their efficacy.25 This is the major reason why volatile repellents with rapid evaporation rates offer limited protection against mosquitoes. Thus, to reduce the rate of evaporation of citronella oil, Bezerra et al.69 formulated citronella oil microcapsules by complex coacervation using gelatin and gum arabic as the polymeric coat. The formulations demonstrated a controlled release of the oil that occurred in two phases. The first stage of the active ingredient release showed Fickian diffusion, while the second stage followed a non-Fickian diffusion mechanism.69 In addition to prolonging the release of citronella oil, the authors also demonstrated the thermal stability of the repellent-loaded microcapsules. The data from the thermal analysis conducted revealed that the microcapsules possessed remarkably higher thermal stability compared to the unformulated citronella oil as the microcapsule structure limited the rapid evaporation of the citronella oil. This also suggests that these microcapsules not only prolong the duration of repellent release but can also protect the repellents loaded in them. As such, they represent an important tool in the delivery of repellents such as essential oils as these chemicals are highly prone to several forms of degradation. Singh et al.68 investigated the mosquito-repellent effect of Thymus vulgaris oil microcapsules. The microcapsules were formulated by the spray drying technique and in situ synthesized chitosan phosphate was used as the shell material. The results obtained showed 100% mosquito repellence which lasted on the fabric even after 20 washes.68 Ribeiro et al.50 developed polymeric microcapsules functionalized with titanium oxide as a solar-activated control release tool for citronella oil. This was aimed at developing a mosquito repellent tool that allows for the release of active ingredients without human intervention by using sunlight as a release activator. The authors employed the modified sol–gel and hydrothermal methods in the synthesis of titanium oxide nanoparticles, while the polymeric microcapsules were prepared using a modified interfacial polymerization technique. The synthesized microcapsules possessed spherical shapes, with the size ranging from 50 to 250 μm. Some of the microcapsules had unreacted or subproducts adsorbed on their external surfaces. Fig. 5a and b show the morphology of microcapsules before and after being subjected to mechanical stress. This suggests that the capsules have a hollow interior with an average thickness of 10 μm. The in vitro bioassay with live mosquito also suggested the microcapsules were able to bring about a controlled release of citronella oil and that microcapsules functionalized with titanium oxide nanoparticles resulted in a prolonged release of citronella oil into the core, thus increasing the repellent activity of the system.50 These studies typify the very versatile nature of nano/microcapsules as an effective tool in regulating the evaporation of repellents in addition to many other benefits they offer in the design of more effective repellent systems, ultimately resulting in prolonged repellent release. They have also been designed to offer protection from degradation to the loaded repellent substances and also bring about a reduction in skin permeation of repellents thus improving the safety of the systems. As such, these systems provide a very important platform that could be utilized in diverse ways to design both topical repellent systems as well as wearable devices.
4.2.2 Micro/nanoemulsion.
Microemulsions are homogeneous and isotropic micro-dispersions with low viscosity, optical transparency, and thermodynamic or kinetic stability. Unlike microemulsions, nanoemulsions are thermodynamically unstable. However, both are simply prepared by mixing oil and water with the addition of surfactant to stabilize the system. The construction of a pseudo-ternary phase diagram usually precedes the development of micro/nanoemulsions as the diagram helps to highlight the relationship between the phase behavior of the different mixtures and their composition.70 This is especially important as the formation of stable micro/nanoemulsions usually occurs in a specific range of surfactants, oil, and water and the pseudo-ternary phase helps to determine the suitable range of these components.70 Co-surfactants are usually added to complement the action of the surfactant in reducing the interfacial tension between the oil and water phases.19,71 Micro/nanoemulsions are divided into oil-in-water (o/w), water-in-oil (w/o), and complex emulsions. These systems are more stable than conventional emulsions and their intrinsic properties including small size and low viscosity make them attractive alternatives to conventional emulsions.72 As such, they are less prone to physical instabilities such as creaming, sedimentation and Ostwald ripening. They easily spread on the skin, with excellent aesthetic appearance.54,72 More so, the production techniques for micro/nanoemulsions are simple, cheap, and scalable.73,74 In addition to prolonged repellent release usually via encapsulation and solubilization, micro/nanoemulsions possess improved water solubility, and tunable loading capacity and can improve the chemical stability of the active ingredients. The major mechanism of repellent release from these systems is via the process of diffusion. The process is usually activated by the application of the formulation on the skin.70 Sugumar et al.75 formulated a eucalyptus oil nanoemulsion by an ultrasonication method and investigated its repellent activity against Culex quinquefasciatus. Tween 80® was used as the surfactant. A stable nanoemulsion was formed at an oil/surfactant ratio of 1
:
2. The results showed a size-dependent activity of essential oil nanoemulsions on the mosquito.75 Similarly, Navayan et al.63 developed a microemulsion of eucalyptus essential oil by a water titration method using Tween 80® and Span 20® as the surfactants and propylene glycol as a co-surfactant. Depending on the concentration of the oil, the time of protection ranged from 82 to 170 min. This was significantly more than that obtained from the non-formulated oil. A 15% eucalyptus oil microemulsion demonstrated the same mosquito repellence as the standard DEET repellent.63 The study thus suggests that the process of microemulsion of eucalyptus oil increased its protection time against mosquitoes by delaying the evaporation of the oil resulting in a comparable repellent effect to a conventional DEET formulation. As such, this system could be employed as a safer and more eco-friendly alternative to DEET formulations as eucalyptus as well other constituents of the microemulsions are not known to possess any harmful effect on humans as well as nontarget organisms, unlike DEET. Soroh et al.34 developed a microemulsion of a 1
:
2 mixture of Litsea and lemon oils by the microemulsification method. The results of the mosquito repellence study demonstrated a 71% repellence activity against Aedes aegypti, which suggested that the Litsea and lemon oil microemulsion showed very strong mosquito-repellent properties.34 The improved level of repellency seen with the use of micro/nanoemulsions in designing repellent systems stems from the ability of these systems to modulate the rate of evaporation of the active repellents, thus ensuring the repellents are available for prolonged periods.25 Despite the many advantages the micro/nanoemulsions offer in the delivery of repellents, there are some concerns about the suitability of these systems for the design of repellents, especially topical/dermal repellents. This is because the presence of surfactants has been implicated in the improvement of drug penetration into the skin.70 It is therefore imperative to evaluate the skin permeability for topical micro/nanoemulsion as this provides information on the safety of these systems.
4.2.3 Solid lipid micro and nanoparticles.
Solid lipid microparticles (SLM) and solid lipid nanoparticles (SLN) are lipid-based delivery systems consisting of solid lipids dispersed in the aqueous surfactant phase. The active ingredient is dispersed or dissolved in the lipid core (as illustrated in Fig. 6). They were developed as alternatives to nanoemulsions and formulated by replacing the oil in nanoemulsions with solid lipids. Therefore, the essential components of solid lipid nanoparticles are the solid lipid, surfactant, and active ingredient. Lipids used in the preparation of SLN/SLM include triglycerides, glycerides, steroids, fatty acids, and waxes.76,77 The type and concentration of lipids and surfactants can influence the encapsulation efficiency, release profile, and stability of the formulations. Repellents are released from SLN/SLM via erosion, diffusion, and/or degradation of the lipid matrix. Loaded repellents can diffuse across the lipid matrix via pores on the matrix or diffuse through the lipid chains. As a result, the release of repellents from these systems can be altered by modification of the lipid matrix, surfactant concentration and other formulation parameters.76–78
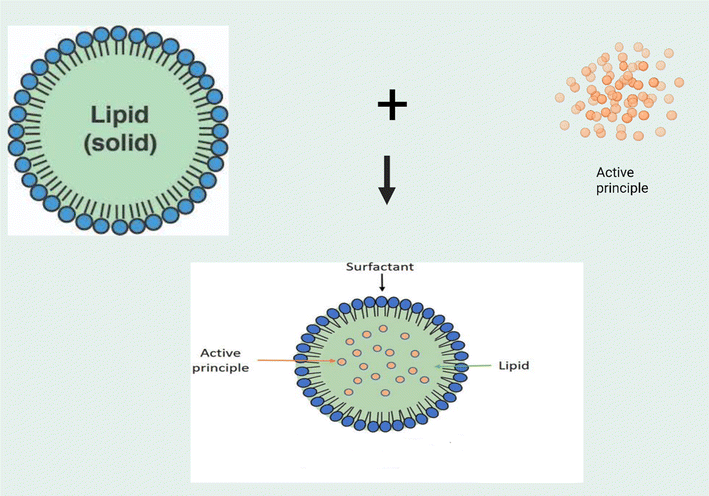 |
| Fig. 6 Schematic representation of the structure and drug loading processes for solid lipid micro/nanoparticles (created with BioRender). | |
SLN/SLM as carriers for mosquito repellents offer many benefits as a result of their small particle size and high surface area, high drug loading capacity, and good stability. Physiologically biocompatible and non-toxic lipids are usually employed in designing these systems, thus enhancing their safety.78,79 They also offer controlled and sustained release of the repellent by regulating the rate of evaporation of repellents from the delivery systems.79–81 The essential oil of Zataria multiflora was loaded in SLN produced by the high-pressure homogenization method. The particle size of 134 nm and encapsulation efficiency of 65% as well as a protection time of 93 min was obtained. Although the protection time was not very long, it was three times longer than that of the non-formulated essential oil. Thus, the nanoparticles brought about a 300% improvement in the efficacy of the essential oil. Although nanoparticles of size above 250 nm are usually preferred in the design of topical repellents to limit the skin penetration of these systems, the authors were able to demonstrate the safety of the formulation as there was no observed cytotoxic effect on the human skin normal cell line (HFFF2) used in the experiment.79 Thus, the SLN improved the duration of repellency of Zataria multiflora essential oil. Nwagwu et al.23 investigated the repellence activity of solid lipid microparticles loaded with neem oil. Encapsulation efficiencies of 52–71% were obtained for various batches of prepared microparticles. There was no skin irritation on the application of the formulation. The results also showed a higher and more prolonged repellent activity against Aedes aegypti than the unformulated oil.23 These studies further support the fact that SLN/SLM can be engineered to modulate the rate of evaporation of volatile repellents such as plant essential oils, thus prolonging their duration of action and ultimately their efficacy against mosquito bites. In summary, SLN/SLM are important drug delivery platforms that could contribute immensely to the design of safer and more effective repellent systems. Seeing that many of the repellents are highly hydrophobic (especially essential oils), the lipid core provides a very efficient environment to improve their solubilization and encapsulation within the particles, hence the relatively high encapsulation efficiency of repellents observed with these systems. These systems also possess very tunable features that can be engineered for the design of several repellent systems. However, the problem of drug expulsion from the lipid matrix, especially on long-term storage has greatly limited the use of these systems in the design of repellent systems.
4.2.4 Nanostructured lipid carriers (NLCs).
Nanostructured lipid carriers (NLCs) are the second generation of lipid nanoparticles and were developed to solve some problems associated with the use of SLN. They are colloidal carriers, whose solid lipid core is composed of a combination of solid and liquid lipids.82 This structure gives the system a unique nanostructure that can improve drug loading and prevent drug expulsion on storage. Recently, NLCs have received a lot of attention as novel systems of drug delivery, especially for topical and transdermal applications. This is because these systems solubilize active ingredients and in the same vein improve stability and efficacy.36 This is invaluable for the delivery of repellents as these systems ensure a longer duration of action and better stability of the active ingredient, especially in storage. In addition, they are biocompatible with a high potential to be adapted to industrial-scale production. Fonseca-Santos et al.83 developed NLCs loaded with tea tree oil (M. alternifolia) using hydrogenated castor oil and PEG-40 (S). The repellence was tested against the Aedes aegypti mosquito. The study showed that the formulations had remarkable bio-adhesiveness and significantly improved the repellent action of tea tree oil (about 90%).83 Abrantes et al.82 using the emulsion/solvent evaporation technique prepared NLCs containing a combination of icaridin (synthetic repellent) and geraniol (natural repellent). The formulation showed a very high encapsulation efficiency (>99%) of the two repellents and also ensured a prolonged and systematic release of both the synthetic and natural repellents at different rates.82 This shows huge promise as a possible tool for exploring the synergistic effect of more than one class of repellents and could prove to be invaluable, especially in the prevention of the development of resistance to repellents by mosquitoes.
4.2.5 Liposomes.
Liposomes are spherical vesicular lipid-based delivery systems with aqueous cores encapsulated by phospholipid bilayers.84 They are usually classified based on their size, number, or charge on the phospholipid bilayer. They are classified into small unilamellar vesicles (SUV), large unilamellar vesicles (LUV), giant unilamellar vesicles (GUV), and multilamellar vesicles (MLV).85 Liposomes are biocompatible and well-suited for topical drug administration. Their ability to promote drug encapsulation, reduce evaporation of active ingredients, especially volatile drugs, and consequently prolong the duration of action of drugs, makes them suitable for the delivery of mosquito repellents. In addition, they can be engineered to promote a significant reduction of skin permeation of drugs, making them a vital tool in preventing toxicities associated with synthetic repellents.24 Liposomal systems also possess the ability to protect topical drugs from degradation.85 This is also important for repellents especially essential oils which have been reported to be sensitive to oxidation and photodegradation.85 Osanloo et al.86 developed nanoliposomes containing 0.5 and 2.5% w/v essential oil from Cinnamomum zeylanicum. These systems had particle sizes of 119 ± 6 and 195 ± 9 nm. The complete protection time of the nanoliposomal gel containing 2.5% C. zeylanicum essential oil was significantly longer than that of 2.5% DEET against Anopheles stephensi (303 ± 10 and 242 ± 12 min respectively at p < 0.001).86 This study demonstrates that the liposomal formulation of Cinnamomum zeylanicum essential oil could be used as a safer and more effective alternative to DEET. Ultra 30®, a repellent formulation from Sawyer and available on the market for commercial use, is a liposomal formulation, composed of 30% DEET. This system can provide a protection time of up to 11 h, making a frequency of twice daily application sufficient to provide adequate protection from mosquito bites. The system also offers a non-greasy, non-staining and sweat-proof formulation.87 Despite being one of the very few formulations, the successful development and translation of Ultra 30® for commercial use further strengthens the possibility of industrial upscaling of biopolymeric and lipid-based systems.
4.2.6 Polymeric micelles.
Polymeric micelles are composed of a core and shell architecture. The inner region is a hydrophobic region of a block copolymer which is very suitable for entrapping poorly water-soluble active ingredients.88 On the other hand, the shell which is the outer layer as the hydrophilic part of the block copolymer protects the encapsulated drugs from an aqueous environment. Common polymers and copolymers used in the development of micelles include polyethylene glycol, poly(aspartic acid), and poly(isopropyl acrylamide).88,89 The process of drug-loading repellent agents into polymeric micelles is usually done either via physical entrapment or chemical conjugation. The technique of repellent loading has also been reported to play an important role in determining the mechanism of their release. Studies have shown that those loaded via physical encapsulation are usually released via diffusion. As such, the stability of the micelles and the rate of copolymer degradation affect the rate of diffusion of repellents and consequently release.88 On the other hand, chemically conjugated repellents are released via surface erosion and degradation of the polymer shells.88 Barradas et al.89 formulated polymeric micelles loaded with DEET using a poly(ethylene oxide)–poly(propylene oxide) block copolymer. The study showed that the formulation reduced DEET skin permeation by 35% compared to the conventional DEET formulation and also prolonged DEET release for 7 h.89 Seeing as one of the primary causes of toxic reactions to repellents is the permeation of these agents via the skin, the use of polymeric micelles not only prolongs the duration of action of these substances but also makes for the development of safer repellent systems as the micelle system can be engineered to limit the penetration of repellents.90
4.2.7 Niosomes.
Niosomes are lipid-based vesicular systems composed of non-ionic bilayer-forming surfactants. They possess similar structures to liposomes; however, synthetic surfactants are used in place of the phospholipids as seen in liposomes. They are typically unilamellar or multilamellar vesicles resulting from reacting cholesterol and non-ionic surfactants.91,92 The use of synthetic surfactants has been known to confer on these systems higher and better chemical stability compared to phospholipids in liposomes. The structure also results in the ability of the niosomes to encapsulate a wide range of active ingredients with improved encapsulation efficiency, bringing about prolonged/controlled release of said drugs, a feature that is highly valuable in the delivery of repellents (especially natural repellents).91 They can also be engineered to reduce the permeability of formulations through the skin. Although niosomes have been more extensively employed in the design of novel larvicidal systems against mosquitoes and other disease vectors,92,93 Amanatfard et al.91 developed a niosomal system for DEET using the dehydration–rehydration method, direct mixing method, and homogenization as a novel mosquito repellent tool. The study showed that the niosomal formulation could provide a prolonged release of DEET for 7 h and reduce skin permeation of the repellent, consequently improving its safety.91 It represents a very diverse tool in the design of safer and more effective strategies for mosquito control.
4.2.8 Cyclodextrins.
Cyclodextrins (CD) are oligosaccharide-based drug delivery systems produced by enzymatic conversion, degradation, and cyclization of starch as well as other α-(1-4)-glucans by cyclodextrin glucotransferase.94 The ability of cyclodextrins to form inclusion complexes with volatile drugs has made them very suitable for the delivery of repellents, especially essential oils.94 The formation of these complexes results in the formation of systems that protect the essential oils from oxidation, thus improving their chemical stability (as seen in Fig. 7). In addition, these systems have brought about the prolonged duration of action of volatile repellents. Proniuk et al.94 developed a cyclodextrin–DEET system as an alternative delivery system to ethanol-based DEET formulations. This was due to the many reports of increased toxicity of the ethanol-based DEET systems. Ethanol, which is a good permeation enhancer, increases the permeation of DEET through the skin and as such increases its systemic absorption, thereby increasing the toxicity issues associated with these formulations.94 The study showed that the cyclodextrin-based formulations developed extended the release of DEET while limiting the absorption of DEET via the skin, thus representing a promising tool for safer and more efficient delivery of repellents. Songkro and Hayook52 developed an inclusion complex of cyclodextrin and citronella oil, using β-cyclodextrins via the kneading method. The study showed a more extended release of citronella oil compared to that of conventional lotions.52 Cecone et al. studied the use of electrospun pyromellitic dianhydride (PMDA) and β-cyclodextrin-based microfibers for the controlled release of DEET. The resulting microfibers had an average diameter of about 2.8 ± 0.8 μm. The study revealed that the microfibers were able to prolong the release of DEET for about two weeks.95 The biocompatibility, versatility, and ability for controlled/sustained drug release by cyclodextrins make them highly beneficial in the design of novel repellent systems.
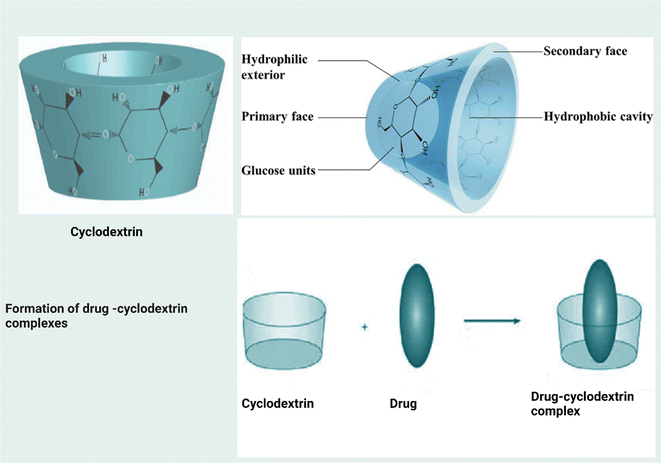 |
| Fig. 7 Schematic representation of the structure and drug loading processes for cyclodextrins (created with BioRender). | |
4.2.9 Porous polymeric nanomaterials.
The use of porous polymeric nanomaterials has over the years received a lot of attention in the design of insect control strategies. These systems are renowned for efficient drug loading as well as sustained/controlled drug release.96 This is primarily because they possess high surface area, large pore volume, and tunable pore sizes. In addition, they are very stable in diverse environments with easily manipulated surface chemistry. Repellents can be loaded in these systems via simple techniques such as adsorption, entrapment, or encapsulation.96 Various porous nanomaterials for mosquito repellence have been extensively designed with biopolymers such as alginate, chitosan, lignin, starch, and cellulose, as these polymers possess the ability to prolong drug release and are also nontoxic to humans and the environment.96 Mapossa et al.15 created microporous polymeric-based strands as a controlled-release device for mosquito repellents. The strands were prepared using a twin-screw extrusion technique to create a reservoir for the repellents (DEET and icaridin). The study showed that the strands that were woven into anklets (Fig. 8a) were able to be loaded with up to 30 wt% of either DEET or icaridin. The system also provided remarkable protection against mosquito bites even after 12 weeks of aging at 50 °C. The SEM results (Fig. 9) also showed that the repellent type as well as concentration affected the morphology of the systems. The micrographs (Fig. 9) also showed the microporous nature of the strands which may be a result of the phase separation of the repellent oil and the polymer via spinodal decomposition.15 This process results in an open cellular microporous scaffold that traps the liquid repellents inside the polymer matrix (schematic Fig. 8b). In addition to the general morphology, the type and concentration of repellents also affected the final microcellular structure of the system.15 The strands developed in this study showed remarkable prolonged drug release (up to 12 weeks) even at a very high temperature (50 °C). This suggests that these systems would be very stable in tropical as well as subtropical regions, where the temperatures are usually high all year round. This is vital as these regions are more plagued by various mosquito-transmitted diseases. The system also provided an efficient platform for the co-delivery of two different repellents, thus limiting the possibility of the development of resistance by mosquitoes to individual repellents. It was also very interesting to note that the study employed the use of inexpensive polymers (poly(ethylene-co-vinyl acetate) and linear low-density polyethylene) in the design of the microporous systems. This was particularly important as it proposes an effective as well as an affordable mosquito control device. More so, since temperature affects the rate of evaporation of repellents from delivery systems,25 these polymeric systems which were stable at very high temperatures (50 °C) present promising tools for achieving controlled repellent release even in regions with high temperatures. Sibanda et al.97 developed bicomponent fibers for the controlled release of mosquito repellents. The fibers were prepared via the process of melt spinning using high-density polyethylene (HDPE) as the sheath and DEET in poly(ethylene-co-vinyl acetate) as the core. The fibers were employed to knit repellent textiles, in this case socks and gloves as seen in Fig. 10. These repellent textiles possessed high residual repellent activity after 20 cold washes or after 8 months of aging under laboratory conditions.97 This study was quite insightful as it sought to develop a strategy to prevent mosquito bites that can be used both indoors and outdoors. Another remarkable application of polymers in the development of novel porous nanomaterials for the delivery of mosquito repellent was reported by Sitoe et al.98 in the development of nanocomposite strands loaded with either DEET or icaridin in a poly(ethylene-co-vinyl acetate) matrix. The study developed microporous polymer-based systems for prolonged delivery of DEET and icaridin. The nanocomposite strands were developed using pyrogenic silica or organoclay as the nanofiller. The study reported that the nature as well as the concentration of both the repellent and nanofillers affected the morphology of the microporous materials. The study also extensively evaluated the mechanism of repellent release from the nanocomposite strands using a wide range of mathematical models and for most of the batches, the Korsmeyer–Peppas model was the best fit for the data obtained.98 This study provided very vital information on the mechanism of repellent release from the polymer matrices and differentiated between diffusion and relaxation mechanisms. Although not tested against live mosquitoes, the study suggested that the strands were able to prolong the release of the repellents used (DEET, icaridin) over 40 days. Loss from water wash-off, sweating, and abrasion (that occurs primarily through friction with clothing and other body surfaces) as well as other physical activities are key causes of loss of repellency.15,25 Thus, the development of wearable repellent systems using porous polymeric materials as reported above could help tackle these challenges while providing long-lasting repellent devices.
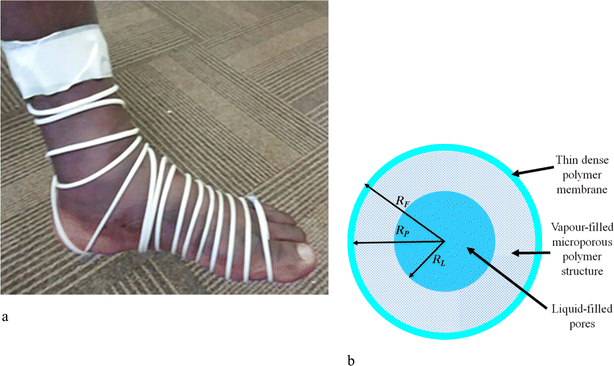 |
| Fig. 8 (a) Photograph of a footlet prepared from the microporous polymers. (b) Model of the microporous strand showing the liquid core location, the vapour-filled microporous region, and the outer skin layer that functions like a membrane that limits the rate at which the repellent is released. Reproduced from ref. 15 with permission from Elsevier, copyright 2019. | |
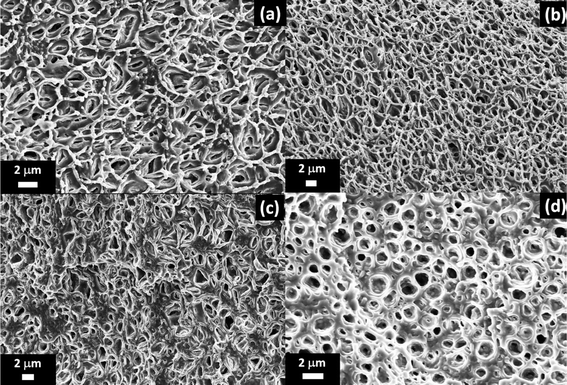 |
| Fig. 9 SEM micrographs showing the effect of insect repellent type and concentration on the structure of the internal microporous region of extruded LLDPE strands. (a) 20 wt% DEET, (b) 30 wt% DEET, (c) 20 wt% icaridin, and (d) 30 wt% icaridin. Reproduced from ref. 15 with permission from Elsevier, copyright 2019. | |
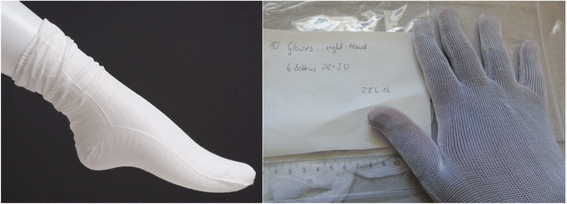 |
| Fig. 10 Images of a sample repellent sock and glove made from porous polymeric materials. Reproduced from ref. 97 with permission from Elsevier, copyright 2018. | |
4.2.10 Nanogels.
Nanogels are nanosized hydrogel systems, primarily consisting of cross-linked polymer networks. These systems are renowned for the unique advantages they offer in drug delivery, such as easy drug loading, high drug encapsulation efficiency, physical stability as well as stability of the encapsulated active moiety, flexibility in design, high biocompatibility, and controlled/extended release of active ingredients. These features have made them highly desirable in the development of novel repellent systems. Sanei-Dehkordi et al.26 developed nanogels from carboxymethylcellulose. These nanogels were loaded individually with carvacrol, cinnamaldehyde, and thymol as well as a combination of these natural repellents. These systems had average droplet sizes of 36.7 ± 5, 114 ± 6, 20 ± 3, and 229 ± 10 nm and PDIs of 0.4, 0.17, 0.38, and 0.23, respectively. The study also revealed that the nanogels produced comparable repellent activities with a commercial DEET sample.26 This suggests that these novel repellent nanogels could be used as an alternative to DEET as they are more biocompatible and possess comparable repellent action to DEET formulations. Moemenbellah-Fard et al.99 also formulated nanoemulsion-based nanogels of Elettaria cardamomum and Zataria multifora essential oils. These systems were reported to have average sizes of 8 ± 1 nm and 86 ± 5 nm for nanogels loaded with Zataria multifora and Elettaria cardamomum essential oil respectively. The repellent effects of the nanogels were evaluated against Anopheles stephensi. The nanogel loaded with Z. multifora essential oil was reported to exhibit a minimum complete protection time of 600 min which was significantly higher than that of DEET (242 ± 12 min) and the nanogel loaded with E. cardamomum essential oil (63 ± 15 min) (P < 0.001).99 The study reported an easy and reproducible method for the development of nanogels and highlighted the advantages as well as the possibility of developing the Z. multifora nanogels as a novel and safer repellent option. Also, to limit the transmission of mosquito-borne diseases, Abrantes et al.58 explored the use of polymeric nanoparticles and a thermosensitive hydrogel-based system for a dual encapsulation of IR3535 and geraniol. The study employed polycaprolactone (PCL) polymer in the design of the nanoparticle system and poloxamer 407 for the hydrogel systems. The resulting nanoparticles were spherical and stable for 4 months, with an encapsulation efficiency of 60% and 99% for IR3535 and geraniol respectively. Also, the cytotoxicity study conducted revealed that the nanoparticle-loaded hydrogel system exhibited a less toxic effect compared to conventional emulsions of active pharmaceutical agents. The release study showed that after 24 h only 7 ± 0.1% and 83 ± 2% of the geraniol and IR3535 were released from the PCL-based nanoparticles. However, when the nanoparticles are incorporated into the hydrogel system 11 ± 0.9 and 29 ± 3% of geraniol and IR3535 were released.58 The study showed a relatively low release of the active ingredients, which may be indicative of the fact that these systems possess the ability to prolong drug release or that the active ingredients irreversibly interact with components of the nanogels limiting their release. The authors concluded that these systems could be used as a potent alternative to conventional repellents however the study did not conduct any repellent assay. Without a repellent assay, it may be difficult to determine if the relatively small amount of IR3535 and geraniol released is sufficient to prevent mosquito bites. Sreedharan Nair et al.100 developed hydrogel systems loaded with DEET microparticles using chitosan and sodium tripolyphosphate. The resulting hydrogels contain particles with sizes ranging from 0.45 to 8.3 μm and relatively good encapsulation efficiencies (>50%). The study also demonstrated a slow and controlled release of DEET from the system via Higuchi kinetics over 24 h and reported that the hydrogel system was able to bring about >50-fold reduction in the skin permeation of DEET as it reduced the cumulative amount of DEET permeated (over 24 h) from 211.6 ± 19.5 μg cm−3 (from a conventional DEET preparation) to 4.07 ± 0.08 μg cm−3 (from hydrogels).100 As such, this suggests a less toxic formulation as the systemic absorption of DEET was remarkably reduced. In addition, the thixotropic evaluation of the microparticle-loaded hydrogels demonstrated a hysteresis loop that closely fit the Herschel–Bulkley model.100 This suggests that the hydrogel can be applied without excessive effort and will most likely possess prolonged skin retention. These studies have demonstrated the applicability of micro/nanogel systems in the design of novel delivery systems for both natural and synthetic repellents. In addition, the reports also provide evidence to support the ability of these systems to prolong the repellent activity of both natural and synthetic repellents while improving their safety.
5 Evaluation of biopolymeric and lipid-based delivery systems in the development of novel repellent formulations
The characterization of novel drug delivery systems to ascertain their physicochemical and biological properties is important in the design of effective and safe repellent formulations. In the development of innovative mosquito-repellent formulations, it is pertinent to evaluate the particle size, particle distribution, encapsulation efficiency, drug loading, morphology, drug release/permeability analysis, rheological properties and the safety profile of these systems. Most of these systems are applied topically as such parameters evaluated for topical formulations provide important information about these systems.
5.1 Particle size
Particle size is a vital parameter, especially for micro/nanosized particulate delivery systems. This is because particle size plays a key role in the physical stability of these systems.101 Photon correlation spectroscopy (PCS) or dynamic light scattering (DLS) is a very common and easy method of evaluating the particle size of the systems. This method provides information on the average particle size and size distribution of the entire system estimated by the polydispersity index (PDI).101,102 This parameter describes whether a system is monodisperse (PDI close to zero) or polydisperse (PDI close to one) which gives an idea of the stability of the system and if the system is prone to destabilizing processes such as creaming, coalescence, Ostwald ripening flocculation and sedimentation.101,102 The particle size of these systems also plays an important role in the release of the active ingredients loaded in them. Reports have shown that systems with smaller particle sizes and consequently larger surface area tend to possess a faster rate of drug release compared to those with larger particle sizes.102 Also, the storage of biopolymeric and lipid-based systems with very small particle sizes usually poses a challenge as very small particles are prone to aggregation on storage.102 As a result, it is important to strike a balance between attaining the maximum stability of the delivery system and particle size. The particles in the drug dispersion, which are usually in Brownian motion, cause a Doppler shift when exposed to a laser beam, and this is explored in the evaluation of the particle size of various drug delivery systems. As the laser beam hits the particles, there is a resulting change in the wavelength of the light and the extent of this change in wavelength is determined by the size of the particles in the dispersion.101
5.2 Morphological properties
The surface structure of polymeric and lipid-based delivery systems has been reported to affect the pharmacological properties and consequently overall safety of these systems.101 Novel lipid and polymeric drug carriers have been reported to possess various shapes, such as spheres, cubes, and rods, and diverse microscopical strategies have been employed to properly study the morphology of these systems.101 One of these includes scanning electron microscopy (SEM). SEM is a versatile instrument that utilizes focused beams of electrons to produce high-resolution, three-dimensional images of various materials and systems, thus providing information about the topography, morphology, and sometimes size of the particles in a system (Fig. 9). Usually, for the SEM analysis, the biopolymeric and lipid-based systems are converted to dry forms by the removal of the continuous phase. The dried samples are then placed on a sample holder and coated with a conductive metal, usually gold, before image analysis. Transmission electron microscopy (TEM) also provides key information regarding the morphology and surface structure of novel drug delivery systems. Although the process of sample preparation is more complex than SEM, the availability of sub-angstrom electron probes provides high-resolution imaging for evaluation of the shape, and internal and surface crystal structures, as well as measurement of particle size.103 Atomic force microscopy (AFM) is also a common technique for studying the surface properties of novel systems. This method employs the use of a high-resolution scanning probe to obtain surface images of particles in the system. AFM is a very useful tool as it provides the possibility of imaging non-conducting samples without specialized treatment, making it very beneficial in imaging biological and delicate biopolymeric and lipid-based drug delivery systems.101,103
5.3 Zeta potential
The zeta potential analysis provides information about the nature and magnitude of the charge on the surface of the particles of a formulation in aqueous dispersions, thus providing insight into the stability of these systems over time.104 This parameter describes the nature of the surface of particles in the biopolymeric and lipid-based systems which can be determined by the nature of the solvent as well as the concentration of the sample used in the study. Also, other factors such as pH, ionic strength, nature, and concentration of electrolytes in the sample solution can affect the zeta potential.103
5.4 Encapsulation efficiency and drug loading capacity
An ideal drug delivery system should possess reasonably high drug encapsulation efficiency and drug loading capacity. This is to bring about a reduction in the amount of matrix materials required for drug administration.5,103 These parameters thus provide information about the cost-effectiveness of the delivery system for a particular active ingredient. The encapsulation efficiency and drug loading are highly affected by the solubility of the drug in the lipid matrix or polymer solution/dispersion. Also, the polymer molecular weight and chemistry that influence drug–polymer interactions are vital factors that affect drug loading in biopolymeric systems.101 The drug payload of a system is commonly determined by using the process of ultracentrifugation (usually at 15
000–30
000 rpm) for 10–30 min to separate the unloaded active ingredient from the entrapped drug. The drug content of the supernatant is then ascertained using high-performance liquid chromatography (HPLC) or spectrophotometry.41
5.5 Crystallinity
Thermal analysis procedures which involve measuring the physical properties of the system as the system is subjected to a controlled temperature change have been employed in evaluating the crystallinity of polymeric and lipid matrices.102 Thermal processes such as thermogravimetric analysis (TGA) and differential scanning calorimetry (DSC) have been employed over the years to determine the degree of crystallinity of not just solid systems but also semi-solid systems such as liposomes and solid lipid nanoparticles. These evaluations are also able to provide information on possible interactions between the active pharmaceutical ingredient(s) (API) and polymer/lipid matrix or other excipients and also API encapsulation or holding capacity. The crystal structure of various biopolymeric and lipid-based delivery systems can also be evaluated by powder/wide-angle X-ray diffractometry.102
5.6 Rheological analysis
The evaluation of the rheological properties of formulations especially those designed for topical application is very vital. The rheology of biopolymeric and lipid-based systems has been reported to affect the rate of drug release as well as spreadability and skin permeability. As such, it is an important parameter to evaluate for topically administered repellent formulations. Reports have shown that various polymeric and lipid-based delivery systems possess different rheological properties and that the increased viscosity of these delivery systems is reported with the presence of highly organized liquid crystal microstructures.105 Sreedharan Nair et al.100 showed that modification of the rheological properties of DEET-loaded microparticle hydrogels was essential in ensuring both ease of application and prolonged retention of the repellent on the skin. The hydrogel's rheological properties, particularly its yield stress, were optimized to enhance retention on the skin. A higher yield stress implies that the hydrogel resists flow under low shear conditions, meaning it will not easily run off or be absorbed quickly, thus ensuring that the DEET remains on the skin's surface to exert its repellent action. The authors also reported that the rheological properties also contributed to a controlled release of DEET as the formulation's structure, influenced by its rheology, allowed DEET to be released slowly over time rather than all at once. This slow release was essential for providing long-lasting protection against mosquitoes. Also, many polymeric and lipid-based delivery systems exhibit plastic and pseudoplastic flow characteristics. Usually, a rheometer is employed in studying the rheological behavior of these systems.105
5.7 Drug release/permeability studies
Evaluation of the in vitro drug release/permeability is very important in the development of novel biopolymeric and lipid-based systems for the delivery of repellents. It is vital to evaluate the degree of drug release from the carrier systems to ensure that the delivery system can maintain the drug concentration at the therapeutic level thus limiting the occurrence of treatment failure and the development of adverse effects. In the development of topical repellents, it is important to limit the skin permeation of the repellent substances as increased permeability is associated with the occurrence of adverse effects. The in vitro drug release and ex vivo permeability studies of novel polymeric and lipid-based systems are usually carried out using a Franz diffusion cell.101 A dialysis membrane is employed as a barrier in in vitro drug release while excised human skin or a weanling pig is used to evaluate the ex vivo permeation. Phosphate buffered saline (pH 5.5) is used as the release medium and the test is conducted at 32 °C to mimic the normal human skin temperature. The cumulative amount of drug released/permeated through the membrane/skin is determined using a UV spectrophotometer or an HPLC and plotted as a function of time and parameters such as flux and apparent permeability coefficient determined.106 Several mathematical models (e.g. Higuchi, Korsmeyer–Peppas, and Weibull models) have been employed over the years for evaluating the release kinetics of repellents. These models are vital tools in the design of novel repellent systems as they provide information that helps evaluate the mechanism of in vitro drug release.25 The Higuchi model is one of the earliest and most widely used models for describing the release of drugs from solid and semi-solid matrices. It describes a square root of time-dependent release, indicating that the release rate decreases over time as the concentration gradient driving the diffusion diminishes.101,104 This model applies to biopolymeric and lipid-based systems where diffusion is the primary mechanism of drug release and assumes that the drug release is governed by Fickian diffusion through a porous matrix.25,98,104 This model is evaluated using the equation:where Q is the cumulative amount of drug released at time t, KH is the Higuchi release constant, and t is the time.
Studies have shown this model to be particularly useful for predicting the release of active ingredients from systems such as polymeric microcapsules and lipid nanoparticles. For example, in the study by Abrantes et al.,58 the release of a repellent like IR3535 from polycaprolactone (PCL) nanoparticles was best described by the Higuchi model. This indicates that the release mechanism was predominantly diffusion-controlled. The researchers observed that the diffusion of IR3535 from the nanoparticles to the surrounding medium followed the linear relationship typical of the Higuchi model.58 The Higuchi model's simplicity and ease of use make it a popular choice for early-stage formulation development. However, it is limited to systems where the drug release is diffusion-controlled and does not account for other mechanisms such as matrix erosion or swelling.58,97,98
The Korsmeyer–Peppas model is a semi-empirical model used to describe drug release from polymeric systems, especially when the release mechanism is not purely Fickian diffusion. It is often applied to systems where the release follows a combination of diffusion and polymer relaxation or erosion.25,101
The mathematical equation for the Korsmeyer–Peppas model is:
|  | (2) |
where
Qt is the amount of drug released at time
t,
Q∞ is the total amount of drug released at infinite time,
K is a kinetic constant, and
n is the release exponent, which indicates the mechanism of drug release.
The value of the release exponent n provides insights into the release mechanism:
• n = 0.5 indicates Fickian diffusion,
• 0.5 < n < 1 suggests anomalous (non-Fickian) transport,
• n = 1 corresponds to zero-order release.
The Korsmeyer–Peppas model is widely used to describe the release of repellents from biopolymeric and lipid-based systems, particularly when the release mechanism involves both diffusion and matrix relaxation. For instance, the release of essential oils from a polymeric microcapsule may be better described by this model due to the complex interplay between diffusion and polymer relaxation. Abrantes et al.82 reported the release mechanism of geraniol and icaridin from the nanostructured lipid carriers they developed was a combination of diffusion and relaxation of the polymeric chains of the gel matrix. The model's ability to accommodate different release mechanisms makes it versatile and broadly applicable.82,97
The Weibull model is a flexible, empirical model that can describe various release profiles, including exponential, sigmoid, and S-shaped curves. It is often used when the release data do not fit well into other models.
The mathematical equation for the Weibull model is:
where
F(
t) is the fraction of drug released at time
t,
λ is the scale parameter (related to the time scale of release), and
β indicates the release mechanism.
The shape parameter β determines the type of release:
• β = 1 indicates exponential (first-order) release,
• β < 1 suggests a decelerating release rate,
• β > 1 suggests an accelerating release rate.
The Weibull model is particularly useful in describing the release of repellents from complex systems where the release profile does not fit simple kinetic models. For example, the release of a repellent from a multilayered lipid nanoparticle might exhibit a release profile best captured by the Weibull model. The Weibull model's flexibility makes it suitable for a wide range of release profiles.97,98
The Mapossa model is a recently developed model tailored specifically for the release of mosquito repellents from advanced delivery systems. This model considers the complexities involved in repellent release, including factors like environmental conditions, repellent volatility, and the interaction between the repellent and the delivery matrix. The mathematical formulation of the Mapossa model is more complex and incorporates elements from existing models while addressing the specific challenges associated with mosquito-repellent delivery. It is particularly suited for predicting the release of repellents from systems such as solid lipid nanoparticles and polymeric microcapsules, where traditional models may fall short. It offers a more accurate representation of the release dynamics under real-world conditions, making it a valuable tool for designing effective mosquito-repellent formulations. The Mapossa model's ability to account for a wide range of factors makes it highly versatile.25,97,98 However, its complexity may limit its use to more advanced research and development stages. It is described by the equation:
| Q(t) = Q0[1 − exp(−t/τ)α] + ktn | (4) |
where
Q(
t) is the cumulative amount of repellent released at time
t,
Q0 is the total amount of repellent initially loaded in the system,
τ is the characteristic time constant that reflects the time scale of the release process,
α is a parameter that describes the shape of the release curve (similar to the shape parameter in the Weibull model),
k is a constant related to the system's diffusion properties, and
n is the release exponent, which can vary depending on the release mechanism.
5.8 Toxicity assays
Testing the safety profile of new formulations represents one of the fundamental tests in evaluating their toxicological profile.107 It is therefore pertinent to determine the safety of novel repellent systems. Toxicity assays for novel topical formulations are broadly classified into two-animal/in vivo models and non-animal/in vitro models. While the in vivo models require the evaluation of the toxic effects of new formulations on animals such as guinea pigs, Wistar rats and rabbits, the in vitro model utilizes various human cells and cell lines to determine the safety of these systems. In vitro assays include in vitro skin irritation tests, in vitro skin corrosion tests, in vitro sensitivity tests and skin genotoxicity while the in vivo model includes in vivo dermal irritation test, phototoxicity, in vivo skin sensitivity and corrosion tests.107
5.9 Stability studies
Similar to conventional drug delivery systems, evaluating the stability of lipid and biopolymeric systems is also very vital in the development of these delivery systems. Studies have reported stability issues such as creaming, sedimentation, coalescence and Ostwald ripening as major stability challenges in the design of novel lipid and polymeric delivery systems. Reports have shown that changes such as a change in the crystallinity of the polymers or lipid modification in these systems can precipitate physical instability.101 It is therefore very important that the formulation techniques for developing these systems be properly designed to optimize their physicochemical characteristics. The stability of lipid and polymeric systems can be evaluated over time by analyzing changes in particle size, zeta potential, encapsulation efficacy and drug content over time.101 Certain studies have also evaluated the stability of active-repellent substances in these delivery systems. A study by Bezerra et al.108 evaluated the effect of microencapsulation on the thermal stability of the repellent citronella oil. The study showed that the resulting citronella oil-loaded microcapsules possessed better thermal stability compared to the unloaded citronella oil. This brought about a slower rate of evaporation of citronella oil and thus prolonged release over time.108 Also, Lucia et al. reported the long-term stability of essential oil-loaded nanoemulsions by aging them for 28 months. The data from the study showed that the nanoemulsions containing eugenol oil maintained their homogeneity for 28 months. This was further confirmed by comparing the PDI and droplet size of the nanoemulsion before and after aging.109
5.10 Repellence testing
Repellence screening of novel systems can be conducted either in a laboratory or in the field and is usually aimed at determining whether the system is repellent, what quantity is required for optimal repellence, and how long the repellent action lasts.110,111 Repellent assays are broadly grouped into in vitro and in vivo repellent assays. The in vitro assays utilize inanimate systems such as cloth fibers, membranes, filter paper, and olfactometers in the evaluation of repellents whereas the in vivo models utilize animals and human subjects for these evaluations. Although the in vitro models present a fast, inexpensive, and safe way to test numerous repellents irrespective of the toxicity of the materials, they provide the least comparable results to animals, especially humans, who are the major end users of these formulations, hence the current rise in the use of in vivo models in testing repellents. Repellent bioassays usually involve a dosage of repellent formulations applied either on human skin, animal skin, or non-living materials such as fabric, membranes, or filter paper exposed to live, blood-sucking arthropods such as mosquitoes either in cages (as is the case in laboratory tests) or in the wild (field experiment) and the corresponding required response recorded.110,111
6 Benefits of biopolymeric and lipid-based systems in the delivery of mosquito repellents
Lipid-based and biopolymeric delivery systems have received much global attention because of their diverse application in designing novel dosage forms for administration via different routes.112 Over the years, many other delivery systems such as metallic nanoparticles, carbon-based nanomaterials, and polymeric systems made from synthetic polymers have been explored in the design of novel mosquito-repellent systems.15,24–26 However, the inherent biocompatibility and biodegradability of biopolymeric and lipid-based systems provide them with significant advantages over other types of nanosystems. These properties ensure that they are not only safer for human use but also safer for nontarget organisms in the environment, thus making them superior candidates for developing sustainable and eco-friendly repellent systems.25 In addition, biopolymeric and lipid-based systems have also been reported to have a remarkable ability to protect active ingredients from degradation especially active ingredients sensitive to light, oxidation, or even hydrolysis, thereby improving the stability of the substance, a phenomenon that makes them invaluable in the development of repellents. In addition, they also modify the release profiles of repellents and thus have been employed as slow-release retarding agents to ensure long-lasting spatial emanation of mosquito repellents at various concentrations.3 This is especially important to improve patient compliance by eliminating the need for multiple reapplications, thus improving the overall efficacy of these systems in preventing mosquito-transmitted diseases. Lipid-based and biopolymeric systems are known to be biocompatible and possess good physical stability.112 Suitable delivery systems for topical repellents should ultimately ensure that the repellent is retained on the skin surface to maximize the repellent action. As such, excessive and rapid evaporation from the skin and skin permeation which will consequently result in the entry of the repellent into the body's circulatory system should be avoided (Fig. 4). Several studies have shown the ability of these systems to reduce the subcutaneous absorption of repellent agents, especially chemical repellents. This plays a vital role in reducing the toxicity issues arising from their use.112 This ability of lipid-based and polymeric systems to prolong the release of drugs while maintaining a low level of skin penetration is highly desirable for repellent formulations. Studies have also shown that by decreasing the droplet size and therefore increasing the surface-to-volume ratio, these systems can improve the solubility of essential oils and ultimately lead to increased drug loading and improved efficacy. These systems are also able to provide a thin film covering the skin that is not easily removed by the normal process of sweating.26 Aside from their use in the development of topical repellents, biopolymeric and lipid-based systems have also been extensively employed in the development of wearable mosquito repellent devices such as bracelets, anklets, gloves, and socks as well as finishing for repellent fabrics.14,15,113,114 The use of these systems in the design of wearable repellents has recently received a lot of attention due to their ability to create safer and more effective control tools for mosquito control, as they ensure prolonged repellent release while offering excellent biocompatibility.
7 Approval status and challenges associated with the clinical translation of biopolymeric and lipid-based mosquito repellents
Despite the benefits of lipid-based and biopolymeric systems for the delivery of repellents, very few studies have focused on the possibility of producing these formulations on a commercial scale. In putting together this review, we came across hundreds of studies that developed impeccable novel lipid and biopolymeric-based repellent systems, some of which were highlighted in the study. However, we found that very few of these systems (e.g. Ultra 30®) have been translated for clinical use and fewer evaluated in any clinical trials. This is as a result of several challenges that have limited the further development of these systems for clinical translation.
Over the years, the stability of biopolymeric and lipid-based systems has posed a huge challenge in designing repellents as some of these systems contain moisture adsorbed by either the biopolymer or lipid matrix.41 This can initiate the process of degradation in the lipid/polymer structure which may ultimately result in the alteration of the physicochemical properties of these systems and consequently their pharmacological performance. Also, changes in the pH of these systems have been reported to cause various forms of instability. It is therefore important to ascertain and maintain the optimal conditions for these systems during storage. Lipid-based and biopolymeric systems are usually composed of generally regarded as safe (GRAS) materials and thus considered to be generally safe for the environment.3 However, there is a dearth of research on the effect of these delivery systems on non-target organisms. Therefore, further research should be geared towards understanding the impact of these delivery systems on the environment which could validate their safety and efficacy as potential smart mosquito delivery systems.
Even though the process of experimental design and development of lipid and biopolymeric-based repellent systems is relatively fast, challenges such as complex manufacturing processes and characterization have posed a huge challenge in commercializing these systems.115 The structural and physicochemical complexities of these repellent strategies compared to conventional drug delivery systems pose a huge limitation to clinical translation as manufacturers are faced with challenges such as poor-quality control protocols and batch-to-batch variations.116 Also, low product yield either as a result of an unsuitable production process or as a result of chemical instability or degradation of active repellent agents during the manufacturing process represents a major hurdle for the commercialization of these systems.117 The high cost of materials and infrastructure for the production of these systems are also major limiting factors to their clinical translation as pharmaceutical industries are less inclined to undertake the heavy cost of redesigning already functional production plants or setting up new ones, which in most cases involves more complex machines and the need to employ experts to make these systems.115,117 It is therefore very vital to provide a detailed cost–benefit analysis that clearly shows that these lipids and biopolymeric repellent systems, although complex to make, possess the ability to bring about an overall reduction in health care costs by increasing the efficacy of the active repellents, thus better preventing the transmission of mosquito-borne diseases and as such reducing the cost of hospitalization and treatment of these diseases. Lipid and biopolymeric-based repellent systems like many other novel drug delivery systems generally undergo more rigorous approval processes. This is because their unique characteristics (e.g. particle size, surface charge, morphology, etc.) vary quite distinctly from conventional materials.118 In addition, the fact that these systems are composed of different types of delivery systems with different properties further makes it more difficult to develop standardized regulatory protocols for the systems.118,119 As such, better understanding of these systems and their physicochemical properties is vital in creating suitable regulatory protocols for their standardization. Furthermore, an inadequate understanding of the in vivo pharmacokinetics and pharmacodynamics of these systems as well as the limited data on the acute and chronic toxicity also poses a huge limitation to the translation of these systems. Thus, research should be geared towards generating actionable data that supports the safety of these systems both for short- and long-term use while also providing information about the in vivo pharmacokinetic effect of these systems. The limited understanding of mosquitoes and the factors that affect their host-seeking behaviors as well as a clear understanding of the mechanism of action of repellents have also greatly limited the clinical translation of lipid and biopolymeric repellent systems. Most researchers focus on engineering drug delivery systems that primarily focus on mechanically improving the efficacy of the process of delivery of the repellents (e.g. creating systems that prolong the duration of action of active repellents). While this is very relevant, a proper understanding of the various factors that affect the host-seeking behaviors in mosquitoes can aid the design and development of more effective repellent systems as the several processes involved in host identification and biting can be targeted by these systems.
Another huge challenge to the translation of these novel repellent systems is limited in vivo repellent data. Many researchers focus on evaluating the in vitro efficacy of these systems using parameters such as in vitro drug release.98,108,109,113 Although these studies can show controlled or prolonged drug release from the novel repellent systems, in vivo repellent assays are also very necessary to demonstrate that the amount of repellent released can prevent mosquito bites and thus prevent disease transmission. Several in vitro models have been designed to mimic the scenario between mosquitoes and the target host; however, in vivo repellent assays provide more clinically relevant data as well as better evaluations of efficacy of these novel systems.120
Overall, there are several missing pieces to the puzzle of very few lipids and biopolymeric repellent systems making it to the market, and thus a collaboration between experts in academia (especially those in pharmaceutical sciences, engineering, materials science, biology, infectious disease management, and toxicology), industry and also regulatory agencies is very necessary in providing the missing information that would enhance the movement of these systems from bench to bedside. This collaboration should be focused on designing strategies for detailed characterization, generation of preclinical data for regulatory agencies and also designing studies for clinical studies and scale-up.
8 Conclusion and future perspectives
In summary, the elucidation of the pivotal role of arthropods such as mosquitoes in the transmission of several diseases has not only helped scientists and researchers to properly understand several diseases but has also armed them with a vital tool in the prevention and control of these diseases. The use of repellents over the years has proven not just to be effective and practical in preventing the transmission of mosquito-borne diseases, but also an economical approach. However, the safety concerns of synthetic repellents as well as the short duration of action of essential oils as a result of their high volatility have greatly limited the use of this all-important preventive tool.
Biopolymeric and lipid-based delivery systems thus present a promising solution by enhancing the safety, efficacy, and longevity of mosquito repellents. These systems offer controlled and/or sustained release of active ingredients, reducing the need for frequent application and thus improving the efficacy of the active repellents. Additionally, they enhance the stability of volatile natural repellents, providing longer-lasting protection. In conclusion, biopolymeric and lipid-based delivery systems represent a significant advancement in developing safer and more effective mosquito repellents.
Future research should focus on overcoming challenges such as complex manufacturing processes and regulatory safety concerns, thus improving scalability and their integration with other vector control strategies. Moving forward, it is important to develop effective and robust laboratory and field-testing protocols for these novel systems, especially against mosquito species that are disease vectors. Application of these systems in the development of life-long repellent products such as bracelets, socks, clothes, and dermal patches could be explored on a commercial scale as these could be invaluable tools for mosquito control, especially in endemic regions. The application of these biopolymeric and lipid-based systems could also encourage the development of resistance-proof mosquito repellents. For instance, the development of dual-release liposomal systems having a highly encapsulated immediate-release hydrophilic core and a moderately loaded, sustained-release shell will ensure that the repellent action is effectively initiated and sustained over time. These dual-release liposomal systems could also be further explored to co-deliver different repellent types or a combination of repellents and insecticides as a more effective tool for the prevention of various mosquito-borne diseases. Also, the alternating layers in biopolymeric systems could be designed to provide a platform for the co-delivery of different classes of repellents, a strategy that could greatly reduce or possibly prevent the occurrence of resistance to repellents by mosquitoes.
Data availability
The authors declare that no primary research results, software, or code has been included and no new data were generated or analyzed as part of this review.
Author contributions
Nwagwu CS: conceptualization, design, investigation, writing – original draft preparation, writing – manuscript review and editing; Onugwu AL: writing – original draft preparation, manuscript review; Echezona AC: writing – original draft preparation; Uzondu WS: writing – original draft preparation; Agbo CP: writing – original draft preparation; Kenechukwu FC: writing – original draft preparation and manuscript revision; Ogbonna JDN: writing – manuscript revision; Ugorji OL: writing – original draft preparation; Nwobi LG: writing – original draft preparation and manuscript revision; Nwobi OC: manuscript revision; Mmuotoo JO: writing – original draft preparation; Ezeibe EN: writing – original draft preparation; Agumah N: manuscript revision; Loretz B: writing – manuscript revision; Tarirai C: conceptualization, design, writing – manuscript review and editing, supervision; Mbara K: writing – manuscript revision; Nnamani PO: writing – manuscript revision; Ofokansi KC: writing – manuscript revision; Lehr CM: writing – manuscript revision and supervision; Attama AA: conceptualization, design, writing – manuscript review and editing, supervision.
Conflicts of interest
The authors declare no conflict of interest.
Acknowledgements
Nwagwu CS wishes to acknowledge the financial support provided by the Bayer Foundation via the Otto-Bayer-Fellowship for Drug Discovery 2022 (OB-2022-034) and the African-German Network of Excellence in Science (AGNES) for granting a Mobility Grant in 2020; the grant was generously sponsored by the German Federal Ministry of Education and Research and supported by the Alexander von Humboldt Foundation.
References
-
World Health Organization, Seventeenth meeting of the WHO Vector Control Advisory Group, 3–6 October 2022, available from https://www.who.int/southeastasia/publications/i/item/9789240066021, accessed on April 30th, 2023.
- E. V. R. Campos, J. L. De Oliveira, D. C. Abrantes, C. B. Rogério and C. Bueno,
et al., Recent Developments in Nanotechnology for Detection and Control of Aedes aegypti -Borne Diseases, Front. Bioeng. Biotechnol., 2020, 8, 1–17, DOI:10.3389/fbioe.2020.00102.
- J. Islam, K. Zaman, S. Duarah and P. Srinivas, Acta Tropica Mosquito repellents: An insight into the chronological perspectives and novel discoveries, Acta Trop., 2017, 167, 216–230, DOI:10.1016/j.actatropica.2016.12.031.
- E. Lupi, C. Hatz and P. Schlagenhauf, ScienceDirect The efficacy of repellents against Aedes , Anopheles , Culex and Ixodes spp e A literature review, Travel Med. Infect. Dis., 2013, 11, 374–411, DOI:10.1016/j.tmaid.2013.10.005.
-
N. Singh, A. Madhu and P. D. Venkatraman, Advances in encapsulation of organic compounds for biological protective textiles, Advances in Healthcare and Protective Textiles, Elsevier, 2023, pp. 509–534, DOI:10.1016/B978-0-323-91188-7.00009-1.
- V. A. Paz-Soldan, V. Plasai, A. C. Morrison, E. J. Rios-Lopez, S. Guedez-Gonzales and J. P. Grieco,
et al., Initial assessment of the acceptability of a push-pull Aedes aegypti control strategy in Iquitos, Peru and Kanchanaburi, Thailand, Am. J. Trop. Med. Hyg., 2011, 84, 208–217, DOI:10.4269/ajtmh.2011.09-0615.
- F. Licciardello, G. Muratore, P. Suma, A. Russo, C. Nerín and M. M. L. Sciences,
et al., Mini review: Mode of action of mosquito repellents, Pestic. Biochem. Physiol., 2013, 7, 149–155, DOI:10.1016/j.pestbp.2013.02.006.
- G. J. Devine, H. J. Overgaard and R. E. Paul, Global vector control guidelines-the need for co-creation, Trends Parasitol., 2019, 35, 267–270, DOI:10.1016/j.pt.2018.12.003.
- N. L. Achee, J. P. Grieco, H. Vatandoost, J. Pinto, L. Ching-ng and A. J. Martins,
et al., Alternative strategies for mosquito-borne arbovirus control, PLoS Neglected Trop. Dis., 2019, 13, 1–22, DOI:10.1371/journal.pntd.0006822.
- S. Arif, H. Rizvi, S. Ling and X. Zeng, Seriphidium brevifolium essential oil: a novel alternative to synthetic insecticides against the dengue vector Aedes albopictus, Environ. Sci. Pollut. Res., 2020, 27, 31863–31871, DOI:10.1007/s11356-020-09108-1/Published.
- F. Esmaili, A. Sanei-dehkordi, F. Amoozegar and M. Osanloo, A Review on the Use of Essential Oil-Based Nanoformulations in Control of Mosquitoes, Biointerface Res. Appl. Chem., 2021, 11, 12516–12529, DOI:10.33263/BRIAC115.1251612529.
- S. Songkro, M. Jenboonlap, M. Boonprasertpon, D. Maneenuan, K. Bouking and N. Kaewnopparat,
et al., Effects of Glucam P-20 , Vanillin , and Fixolide on Mosquito Repellency of Citronella Oil Lotions, J. Med. Entomol., 2012, 49(3), 672–677, DOI:10.1603/me11141.
- J. D. Ogilvie-Battersby, R. Nagarajan, R. Mosurkal and N. Orbey, Microencapsulation and controlled release of insect repellent geraniol in gelatin/gum arabic microcapsules, Colloids Surf., A, 2022, 640, 128494, DOI:10.1016/J.COLSURFA.2022.128494.
- A. Sitoe, A. B. Mapossa, W. W. Focke, H. Muiambo, R. Androsch and J. Wesley-Smith, Development, characterization and modeling of mosquito repellent release from microporous devices, SPE Polym., 2020, 1, 90–100, DOI:10.1002/pls2.10021.
- A. B. Mapossa, M. M. Sibanda, A. Sitoe, W. W. Focke, L. Braack, C. Ndonyane, J. Mouatcho, J. Smart, H. Muaimbo, R. Androsch and M. T. Loots, Microporous polyolefin strands as controlled-release devices for mosquito repellents, Chem. Eng. J., 2019, 360, 435–444, DOI:10.1016/j.cej.2018.11.237.
- C. Henrique, M. Galu, K. Santana, A. R. Santana, S. Carvalho and L. Diniz,
et al., Exploring the Potentiality of Natural Products from Essential Oils as Inhibitors of Odorant-Binding Proteins: A Structure- and Ligand- Based Virtual Screening Approach To Find Novel Mosquito Repellents, ACS Omega, 2019, 27, 22475–22486, DOI:10.1021/acsomega.9b03157.
- A. Lucia, P. G. Argudo, E. Guzmán, R. G. Rubio and F. Ortega, Formation of surfactant free microemulsions in the ternary system water/eugenol/ethanol, Colloids Surf., A, 2017, 521, 133–140, DOI:10.1016/j.colsurfa.2016.04.062.
- P. Chattopadhyay, S. Dhiman, S. Borah and B. Rabha, Acta Tropica Essential oil based polymeric patch development and evaluating its repellent activity against mosquitoes, Acta Trop., 2015, 147, 45–53, DOI:10.1016/j.actatropica.2015.03.027.
- P. Xu, Y.-M. Choo, A. De La Rosa and W. S. Leal, Mosquito odorant receptor for DEET and methyl jasmonate, Proc. Natl. Acad. Sci. U. S. A., 2014, 111, 16592–16597, DOI:10.1073/pnas.1417244111.
- W. S. Leal, The enigmatic reception of DEET – The gold standard of insect repellents, Curr. Opin. Insect Sci., 2014, 6, 93–98, DOI:10.1016/j.cois.2014.10.007.
- J. Bonnet, C. Pennetier, S. Duchon, B. Lapied and V. Corbel, Multi-function oxidases are responsible for the synergistic interactions occurring between repellents and insecticides in mosquitoes, Parasit. Vectors, 2023, 17, 1–7, DOI:10.1186/1756-3305-2-17.
- C. Costantini, A. Badolo and E. Ilboudo-sanogo, Field evaluation of the efficacy and persistence of insect repellents DEET , IR3535 , and KBR 3023 against Anopheles gambiae complex and other Afrotropical vector mosquitoes, Trans. R. Soc. Trop. Med. Hyg., 2004, 98, 644–652, DOI:10.1016/j.trstmh.2003.12.015.
- C. S. Nwagwu, J. D. Ogbonna, L. G. Nwobi, A. C. Echezona, C. Ugwu and E. N. Ezeibe,
et al., Design, Development and Evaluation of the Repellent Activity of Azadirachta indica Oil-Based Solid Lipid Microparticles against Aedes aegypti (Linn), Trop. J. Nat. Prod. Res., 2020, 4, 471–478, DOI:10.26538/tjnpr/v4i8.24.
- M. Tavares, M. R. M. da Silva, L. B. de Oliveira de Siqueira, R. A. S. Rodrigues, L. Bodjolle-d’Almeira and E. P. dos Santos,
et al., Trends in insect repellent formulations: A review, Int. J. Pharm., 2018, 539, 190–209, DOI:10.1016/j.ijpharm.2018.01.046.
- A. B. Mapossa, W. W. Focke, R. K. Tewo, R. Androsch and T. Kruger, Mosquito-repellent controlled-release formulations for fighting infectious diseases, Malar. J., 2021, 20, 165, DOI:10.1186/s12936-021-03681-7.
- A. Sanei-Dehkordi, S. Hatami, E. Zarenezhad, Z. Montaseri and M. Osanloo, Efficacy of nanogels containing carvacrol, cinnamaldehyde, thymol, and a mix compared to a standard repellent against Anopheles stephensi, Ind. Crops Prod., 2022, 189, 115883, DOI:10.1016/j.indcrop.2022.115883.
- S. Adusei and S. Azupio, Neem: A Novel Biocide for Pest and Disease Control of Plants, J. Chem., 2022, 2022, 1–12, DOI:10.1155/2022/6778554.
- Z. Ladan, B. Okoli and F. Mtunzi, Efficacy and Safety of Essential Oils in The Control of Mosquito: A Review of Research Findings, Int. J. Chem., 2022, 14, 45–59, DOI:10.5539/ijc.v14n2p45.
- M. D. Chatzidaki, S. Demisli, E. Zingkou, P. G. V. Liggri, D. P. Papachristos and G. Balatsos,
et al., Essential oil-in-water microemulsions for topical application: structural study, cytotoxic effect and insect repelling activity, Colloids Surf., A, 2022, 654, 130159, DOI:10.1016/j.colsurfa.2022.130159.
- E. F. De Oliveira, H. C. B. Paula and R. C. M. De Paula, Colloids and Surfaces B : Biointerfaces Alginate/cashew gum nanoparticles for essential oil encapsulation, Colloids Surf., B, 2014, 113, 146–151, DOI:10.1016/j.colsurfb.2013.08.038.
- C. Rakkiyappan, R. P. Singh and A. Bhattacharya, Study on Encapsulation of Diethyl Phenyl Acetamide in Calcium-Alginate Microsphere for Enhanced Repellent Efficacy Study on Encapsulation of Diethyl Phenyl Acetamide in Calcium-Alginate Microsphere for Enhanced Repellent Efficacy, Int. J. Polym. Mater., 2012, 61, 1154–1163, DOI:10.1080/00914037.2011.641635.
- C. Xiao, M. Zhou, X. Lin and R. Li, Chemical Modification of Calcium Alginate Gel Beads for Controlling the Release of Insect Repellent N , N -Diethyl-3-methylbenzamide, J. Appl. Polym. Sci., 2006, 102, 4850–4855, DOI:10.1002/app.24826.
- M. Sumithra, Effect of insect repellent property using microencapsulation technique, World J. Pharm. Res., 2016, 5, 715–719, DOI:10.20959/wjpr20164-5761.
- A. Soroh, L. Owen, N. Rahim, J. Masania, A. Abioye and O. Qutachi,
et al., Microemulsification of essential oils for the development of antimicrobial and mosquito repellent functional coatings for textiles, J. Appl. Microbiol., 2021, 131, 2808–2820, DOI:10.1111/jam.15157.
- S. L. Kadam, P. Yadav, S. Bhutkar, V. D. Patil, P. G. Shukla and K. Shanmuganathan, Sustained release insect repellent microcapsules using modified cellulose nanofibers (mCNF) as pickering emulsifier, Colloids Surf., A, 2019, 582, 123883, DOI:10.1016/j.colsurfa.2019.123883.
- C. B. Rogerio, D. C. Abrantes, J. L. De Oliveira, D. Ribeiro, D. Arau and T. Germano,
et al., Cellulose Hydrogels Containing Geraniol and Icaridin Encapsulated in Zein Nanoparticles for Arbovirus Control, ACS Appl. Bio Mater., 2022, 5, 1273–1283, DOI:10.1021/acsabm.1c01286.
- G. C. Türkoğlu, A. M. Sarıışık, G. Erkan, M. S. Yıkılmaz and O. Kontart, Micro- and nano-encapsulation of limonene and permethrin for mosquito repellent finishing of cotton textiles, Iran. Polym. J., 2020, 29, 321–329, DOI:10.1007/s13726-020-00799-4.
- A. Sanei-Dehkordi, M. Djaefar, M. Fard and H. Sereshti, Chitosan nanoparticles containing Elettaria cardamomum and Cinnamomum zeylanicum essential oils ; repellent and larvicidal effects against a malaria mosquito vector , and cytotoxic effects on a human skin normal cell line, Chem. Pap., 2021, 75, 6545–6556, DOI:10.1007/s11696-021-01829-y.
- T. K. Maji and R. Hussain, Microencapsulation of Zanthoxylum limonella Oil (ZLO) in Genipin Crosslinked Chitosan – Gelatin Complex for Mosquito Repellent Application, Bioresour. Technol., 2009, 111, 779–785, DOI:10.1016/j.biortech.2006.03.005.
- N. Singh and J. Sheikh, Multifunctional Linen Fabric Obtained through Finishing with Chitosan-gelatin Microcapsules Loaded with Cinnamon Oil Multifunctional Linen Fabric Obtained through Finishing with Chitosan-gelatin Microcapsules Loaded with Cinnamon Oil, J. Nat. Fibers, 2021, 1–11, DOI:10.1080/15440478.2020.1870625.
- N. Singh and J. Sheikh, Novel Chitosan-Gelatin microcapsules containing rosemary essential oil for the preparation of bioactive and protective linen, Ind. Crops Prod., 2022, 178, 114549, DOI:10.1016/J.INDCROP.2022.114549.
- R. Bano, Use of Chitosan in Mosquito Repellent Finishing for Cotton Textiles, J. Text. Sci. Eng., 2014, 4, 4–6, DOI:10.4172/2165-8064.1000162.
- S. Kala, A. Agarwal, N. Sogan and S. N. Naik, Chitosan-acrylate nanogel for durable anti mosquito finishing of cotton fabric and its dermal toxicity profiling on Swiss albino mice, Colloids Surf., B, 2019, 181, 789–797, DOI:10.1016/j.colsurfb.2019.06.022.
- B. Solomon, F. F. Sahle, T. Gebre-mariam, K. Asres and R. H. H. Neubert, Microencapsulation of citronella oil for mosquito-repellent application : Formulation and in vitro permeation studies, Eur. J. Pharm. Biopharm., 2012, 80, 61–66, DOI:10.1016/j.ejpb.2011.08.003.
- M. D. Teli and P. P. Chavan, Application of Gelatine Based Microcapsules Containing Mosquito Repellent Oils on Cellulosic Biopolymer, J. Bionanosci., 2016, 10, 1–6, DOI:10.1166/jbns.2016.1396.
- S. Boominathan, G. Magesh and S. Balakrishnan, Antimicrobial and Mosquito Repellent Finish on Cotton Fabric Using Coleus Aromaticus Leaf Extract, J. Nat. Fibers, 2021, 1–11, DOI:10.1080/15440478.2020.1870620.
- J. D. Ogilvie-Battersby, R. Nagarajan, R. Mosurkal and N. Orbey, Microencapsulation and controlled release of insect repellent geraniol in gelatin/gum arabic microcapsules, Colloids Surf., A, 2022, 640, 128494, DOI:10.1016/j.colsurfa.2022.128494.
- N. Misni, Z. M. Nor and R. Ahmad, Repellent effect
of microencapsulated essential oil in lotion formulation against mosquito bites, J. Vector Borne Dis., 2017, 54(1), 44–53 CrossRef CAS PubMed.
- A. Mihranyan, N. Ferraz and M. Strømme, Current status and future prospects of nanotechnology in cosmetics, Prog. Mater. Sci., 2012, 57, 875–910, DOI:10.1016/j.pmatsci.2011.10.001.
- A. D. Ribeiro, J. Marques, M. Forte, F. C. Correia, P. Parpot and C. Oliveira,
et al., Microencapsulation of citronella oil for solar-activated controlled release as an insect repellent, Appl. Mater. Today, 2016, 5, 90–97, DOI:10.1016/j.apmt.2016.09.003.
- B. Solomon, F. F. Sahle, T. Gebre-mariam, K. Asres and R. H. H. Neubert, European Journal of Pharmaceutics and Biopharmaceutics Microencapsulation of citronella oil for mosquito-repellent application : Formulation and in vitro permeation studies, Eur. J. Pharm. Biopharm., 2012, 80, 61–66, DOI:10.1016/j.ejpb.2011.08.003.
- S. Songkro, N. Hayook, J. Jaisawang, D. Maneenuan, T. Chuchome and N. Kaewnopparat, Investigation of inclusion complexes of citronella oil, citronellal and citronellol with β-cyclodextrin for mosquito repellent, J. Inclusion Phenom. Macrocyclic Chem., 2012, 72, 339–355, DOI:10.1007/s10847-011-9985-7.
- A. Sanei-Dehkordi, A. Abdollahi, M. Safari, F. Karami, G. Ghaznavi and M. Osanloo, Nanogels Containing Foeniculum vulgare Mill. and Mentha piperita L. Essential Oils: Mosquitoes’ Repellent Activity and Antibacterial Effect, Interdiscip. Perspect. Infect. Dis., 2022, 2022, 4510182, DOI:10.1155/2022/4510182.
- S. A. Chime and I. V. Onyishi, Lipid-based drug delivery systems (LDDS): Recent advances and applications of lipids in drug delivery, Afr. J. Pharm. Pharmacol., 2013, 7, 3034–3059, DOI:10.5897/AJPPX2013.0004.
- L. Ciera, L. Beladjal, L. Van Landuyt, D. Menger, M. Holdinga and J. Mertens,
et al., Electrospinning repellents in polyvinyl alcohol-nanofibres for obtaining mosquito-repelling fabrics, R. Soc. Open Sci., 2019, 6, 182139, DOI:10.1098/rsos.182139.
- T. Manangan and S. Shawaphun, Poly(lactic acid) Microencapsulation Prolongs the Mosquito Repellent Activity of Essential Oils from Thai Herbs, J. Appl. Sci. Emerg. Technol., 2023, 22, 1–11, DOI:10.14416/JASET.KMUTNB.2023.01.008.
- F. Pardini, Á. Iregui, P. Faccia, J. Amalvy, A. González and L. Irusta, Development and characterization of electrosprayed microcaspules of poly ε-caprolactone with citronella oil for mosquito-repellent application, Int. J. Polym. Anal. Charact., 2021, 26, 497–516, DOI:10.1080/1023666X.2021.1916726.
- D. C. Abrantes, C. B. Rogerio, E. V. R. Campos, T. Germano-Costa, A. A. Vigato and I. P. Machado,
et al., Repellent active ingredients encapsulated in polymeric nanoparticles: potential alternative formulations to control arboviruses, J. Nanobiotechnol., 2022, 20(10), 520, DOI:10.1186/s12951-022-01729-7.
- A. Kamari, S. N. M. Yusoff, S. T. S. Wong, E. Phillip, J. S. J. Hargreaves and H. Othman, Betel essential oil-loaded lipid-core nanocapsules as mosquito repellent spray formulations for fabric finishes, J. Text. Inst., 2023, 114, 10–21, DOI:10.1080/00405000.2021.2018787.
- T. M. K. Rettob, E. Zebua, I. S. Butar-butar, F. G. Tular and Y. S. Mokosuli, The Utilization Of Beehive Wax a Combination of Nutmeg Extract (Myristica fragrans Houtt.) and Langsat (Lansium domesticum L.) as Aromatherapy and Mosquito Repellent, Jurnal Biologi Tropis, 2021, 21, 845–853, DOI:10.29303/jbt.v21i3.2938.
- N. Ngamekaue and P. Chitprasert, Effects of beeswax-carboxymethyl cellulose composite coating on shelf-life stability and intestinal delivery of holy basil essential oil-loaded gelatin microcapsules, Int. J. Biol. Macromol., 2019, 135, 1088–1097, DOI:10.1016/j.ijbiomac.2019.06.002.
-
T. N. da Silva, S. A. Cardoso and T. N. Barradas, Nanostructured pharmaceutical formulations for topical application of clove oil and eugenol, Clove (Syzygium Aromaticum), 2022, pp. 363–403, DOI:10.1016/B978-0-323-85177-0.00019-7.
- A. Navayan, E. Moghimipour, M. J. Khodayar, B. Vazirianzadeh, A. Siahpoosh and M. Valizadeh,
et al., Evaluation of the Mosquito Repellent Activity of Nano-sized Microemulsion of Eucalyptus globulus Essential Oil Against Culicinae, Jundishapur J. Nat. Pharm. Prod., 2017, 12(4) DOI:10.5812/jjnpp.55626.
- H. Jing, D. Weijun, L. Liqin and X. Zuobing, Synthesis and application of polyacrylate nanocapsules loaded with lilial, J. Appl. Polym. Sci., 2014, 131, 40182, DOI:10.1002/app.40182.
- Y. Li, Y.-Q. Huang, H.-F. Fan and Q. Xia, Heat-resistant sustained-release fragrance microcapsules, J. Appl. Polym. Sci., 2014, 131, 40053, DOI:10.1002/app.40053.
- G. C. Türkoğlu, A. M. Sarıışık, G. Erkan, M. S. Yıkılmaz and O. Kontart, Micro- and nano-encapsulation of limonene and permethrin for mosquito repellent finishing of cotton textiles, Iran. Polym. J., 2020, 29, 321–329, DOI:10.1007/s13726-020-00799-4.
- F. M. Bezerra, Ó. G. Carmona, C. G. Carmona, A. Mathias, S. Plath and M. Lis, Biofunctional wool using β-cyclodextrins as vehiculizer of citronella oil, Process Biochem., 2019, 77, 151–158, DOI:10.1016/j.procbio.2018.11.018.
- N. Singh and J. Sheikh, Sustainable development of mosquito-repellent, flame-retardant, antibacterial, fragrant and antioxidant linen using microcapsules containing Thymus vulgaris oil in in situ generated chitosan-phosphate, Cellulose, 2021, 28, 2599–2614, DOI:10.1007/s10570-020-03629-1.
- F. M. Bezerra, M. Lis, Ó. G. Carmona, C. G. Carmona, M. P. Moisés and G. M. Zanin,
et al., Assessment of the delivery of citronella oil from microcapsules supported on wool fabrics, Powder Technol., 2019, 343, 775–782, DOI:10.1016/j.powtec.2018.11.001.
- E. B. Souto, A. Cano, C. Martins-Gomes, T. E. Coutinho, A. Zielińska and A. M. Silva, Microemulsions and Nanoemulsions in Skin Drug Delivery, Bioengineering, 2022, 9(4), 158, DOI:10.3390/bioengineering9040158.
-
S. A. Chime, F. C. Kenechukwu and A. A. Attama, Nanoemulsions — Advances in Formulation, Characterization and Applications in Drug Delivery, Application of Nanotechnology in Drug Delivery, InTech, 2014, DOI:10.5772/58673.
- V. K. Rai, N. Mishra, K. S. Yadav and N. P. Yadav, Nanoemulsion as pharmaceutical carrier for dermal and transdermal drug delivery : Formulation development , stability issues , basic considerations and applications, J. Controlled Release, 2018, 270, 203–225, DOI:10.1016/j.jconrel.2017.11.049.
- A. Mihranyan, N. Ferraz and M. Strømme, Current status and future prospects of nanotechnology in cosmetics, Prog. Mater. Sci., 2012, 57, 875–910, DOI:10.1016/j.pmatsci.2011.10.001.
- R. Pavela, F. Maggi, R. Iannarelli and G. Benelli, Plant extracts for developing mosquito larvicides: From laboratory to the field, with insights on the modes of action, Acta Trop., 2019, 193, 236–271, DOI:10.1016/j.actatropica.2019.01.019.
- S. Sugumar, S. K. Clarke, M. J. Nirmala, B. K. Tyagi, A. Mukherjee and N. Chandrasekaran, Nanoemulsion of eucalyptus oil and its larvicidal activity against Culex quinquefasciatus, Bull. Entomol. Res., 2014, 104, 393–402, DOI:10.1017/S0007485313000710.
-
A. Zielińska and I. Nowak, Solid lipid nanoparticles and nanostructured lipid carriers as novel carriers for cosmetic ingredients, Nanobiomaterials in Galenic Formulations and Cosmetics, Elsevier, 2016, pp. 231–255, DOI:10.1016/B978-0-323-42868-2.00010-3.
- R. Liu, L. Dai, C. Xu, K. Wang and C. Zheng, C. Si Lignin-based micro-and nanomaterials and their composites in biomedical applications, ChemSusChem, 2020, 13, 4266 CrossRef CAS PubMed.
- C. D. Ferreira and I. L. Nunes, Oil nanoencapsulation: development, application, and incorporation into the food market, Nanoscale Res. Lett., 2019, 14, 9, DOI:10.1186/s11671-018-2829-2.
- H. R. Kelidari and M. D. Moemenbellah-fard, Solid-lipid nanoparticles (SLN) s containing Zataria multiflora essential oil with no-cytotoxicity and potent repellent activity against Anopheles stephensi, J. Parasit. Dis., 2021, 45(1), 101–108, DOI:10.1007/s12639-020-01281-x.
- J. I. Karr, T. J. Speaker and G. B. Kasting, A novel encapsulation of N,N-diethyl-3-methylbenzamide (DEET) favorably modifies skin absorption while maintaining effective evaporation rates, J. Controlled Release, 2012, 160, 502–508, DOI:10.1016/j.jconrel.2012.04.023.
- G. Yang, X. Li, Y. He, J. Ma, G. Ni and S. Zhou, From nano to micro to macro: Electrospun hierarchically structured polymeric fibers for biomedical applications, Prog. Polym. Sci., 2018, 81, 80–113, DOI:10.1016/j.progpolymsci.2017.12.003.
- D. C. Abrantes, C. B. Rogerio, J. L. De Oliveira, E. V. R. Campos, D. R. De Araújo and L. C. Pampana, Development of a Mosquito Repellent Formulation Based on Nanostructured Lipid Carriers, Front. Pharmacol, 2021, 12, 1–13, DOI:10.3389/fphar.2021.760682.
- B. Fonseca-Santos, C. Del, N. Pacheco, M. C. Pinto and M. Chorilli, Industrial Crops & Products An effective mosquito-repellent topical product from liquid crystal-based tea tree oil, Ind. Crops Prod., 2019, 128, 488–495, DOI:10.1016/j.indcrop.2018.11.020.
- A. M. S. Simão, M. Bolean, T. A. C. Cury, R. G. Stabeli, R. Itri and P. Ciancaglini, Liposomal systems as carriers for bioactive compounds, Biophys. Rev., 2015, 7, 391–397, DOI:10.1007/s12551-015-0180-8.
- G. Zorzi, E. Carvalho, P. G. Von and H. F. Teixeira, On the use of nanotechnology-based strategies for association of complexes matrices from plant extracts, Rev. Bras. Farmacogn., 2015, 1–11, DOI:10.1016/j.bjp.2015.07.015.
- M. Osanloo, S. Firoozian, E. Zarenezhad, Z. Montaseri and S. Satvati, A Nanoliposomal Gel Containing Cinnamomum zeylanicum Essential Oil with Effective Repellent against the Main Malaria Vector Anopheles stephensi, Interdiscip. Perspect. Infect. Dis., 2022, 2022, 1–6, DOI:10.1155/2022/1645485.
-
Sawyer 2015, available at https://www.sawyer.com/products/controlled-release-insect-repellent, accessed on April 25th, 2023.
- U. Kedar, P. Phutane, S. Shidhaye and V. Kadam, Advances in polymeric micelles for drug delivery and tumor targeting, Nanomedicine, 2010, 6, 714–729, DOI:10.1016/j.nano.2010.05.005.
- T. N. Barradas, J. P. Senna, E. R. Júnior, C. Regina and E. Mansur, Polymer-based Drug Delivery Systems Applied to Insects Repellents Devices : A Review, Curr. Drug Delivery, 2015, 13(2), 221–235, DOI:10.2174/1567201813666151207110515.
- J. J. G. Aswin, S. Maria Packiam and E. Nakkeeran, Contact in-vivo larvicidal toxicity and histological studies of Indian herb essential oils loaded niosomes against Aedes aegypti and Culex quinquefasciatus (Diptera: Culicidae), Int. J. Trop. Insect Sci., 2021, 40, 1373–1387, DOI:10.1007/s42690-021-00656-8.
- A. Amanatfard, P. Khazaeli, A. Pardakhty, M. Najafi and A. Mohammadi, Preparation and characterization of physicochemical properties of N, N-diethyl-meta-toluamide niosomes, Int. J. Pharm. Invest., 2015, 5, 259, DOI:10.4103/2230-973x.167691.
- J. Pardeike, A. Hommoss and R. H. Müller, Lipid nanoparticles (SLN, NLC) in cosmetic and pharmaceutical dermal products, Int. J. Pharm., 2009, 366, 170–184, DOI:10.1016/j.ijpharm.2008.10.003.
- A. Kalaiselvi, J. G. Aswin Jeno, S. M. Roopan, G. Madhumitha and E. Nakkeeran, Chemical composition of clove bud oil and development of clove bud oil loaded niosomes against three larvae species, Int. Biodeterior. Biodegrad., 2019, 137, 102–108, DOI:10.1016/j.ibiod.2018.12.004.
- S. Proniuk, B. M. Liederer, S. E. Dixon, J. A. Rein, M. A. Kallen and J. Blanchard, Topical Formulation Studies with DEET (N,N-Diethyl-3-methylbenzamide ) and Cyclodextrins, J. Pharm. Sci., 2002, 91, 101–110, DOI:10.1002/jps.1172.
- C. Cecone, F. Caldera, F. Trotta, P. Bracco and M. Zanetti, Controlled release of DEET loaded on fibrous mats from electrospun PMDA/cyclodextrin polymer, Molecules, 2018, 23(7), 1694, DOI:10.3390/molecules23071694.
- G. Singh, K. Ramadass, P. Sooriyakumar, O. Hettithanthri, M. Vithange and N. Bolan,
et al., Nanoporous materials for pesticide formulation and delivery in the agricultural sector, J. Controlled Release, 2022, 343, 187–206, DOI:10.1016/j.jconrel.2022.01.036.
- M. Sibanda, W. Focke, L. Braack, A. Leuteritz, H. Brünig and N. H. A. Tran,
et al., Bicomponent fibres for controlled release of volatile mosquito repellents, Mater. Sci. Eng., C, 2018, 91, 754–761, DOI:10.1016/j.msec.2018.06.016.
- A. Sitoe, A. B. Mapossa, W. W. Focke, H. Muiambo and R. Androsch, Development , characterization and modeling of mosquito repellent release from microporous devices, SPE Polym., 2020, 1, 90–100, DOI:10.1002/pls2.10021.
- M. D. Moemenbellah-Fard, S. Firoozian, M. Shahriari-Namadi, E. Zarenezhad, G. Roozitalab and M. Osanloo, A natural nanogel with higher efficacy than a standard repellent against the primary malaria mosquito vector, Anopheles stephensi Liston, Chem. Pap., 2022, 76, 1767–1776, DOI:10.1007/s11696-021-02006-x.
- R. Sreedharan Nair, H. Rahman, M. Kong, X. Yi Tan, K. Chen and S. Shanmugham, Development and Rheological Evaluation of DEET (N,N-DiethyL-3-Methylbenzamide) Microparticles Loaded Hydrogel For Topical Application, Turk. J. Pharm. Sci., 2021, 18, 352–359, DOI:10.4274/tjps.galenos.2020.88725.
- A. L. Onugwu, C. S. Nwagwu, O. S. Onugwu, A. C. Echezona, C. P. Agbo and S. A. Ihim,
et al., Nanotechnology based drug delivery systems for the treatment of anterior segment eye diseases, J. Controlled Release, 2023, 354, 465–488, DOI:10.1016/j.jconrel.2023.01.018.
- A. Dubey, P. Prabhu and V. J. Kamath, Nano Structured lipid carrier: A Novel Topical drug delivery system, Int. J. Pharmtech Res., 2012, 4(2), 705–714 CAS.
-
S. Bhatia, Nanoparticles Types, Classification, Characterization, Fabrication Methods and Drug Delivery Applications, Natural Polymer Drug Delivery Systems, Springer International Publishing, Cham, 2016, pp. 33–93, DOI:10.1007/978-3-319-41129-3_2.
- A. Gordillo-Galeano and C. E. Mora-Huertas, Solid lipid nanoparticles and nanostructured lipid carriers: A review emphasizing on particle structure and drug release, Eur. J. Pharm. Biopharm., 2018, 133, 285–308, DOI:10.1016/j.ejpb.2018.10.017.
- C. C. Müller-Goymann, Physicochemical characterization of colloidal drug delivery systems such as reverse micelles, vesicles, liquid crystals and nanoparticles for topical administration, Eur. J. Pharm. Biopharm., 2004, 58, 343–356, DOI:10.1016/j.ejpb.2004.03.028.
- V. Domínguez-Villegas, B. Clares-Naveros, M. L. García-López, A. C. Calpena-Campmany, P. Bustos-Zagal and M. L. Garduño-Ramírez, Development and characterization of two nano-structured systems for topical application of flavanones isolated from Eysenhardtia platycarpa, Colloids Surf., B, 2014, 116, 183–192, DOI:10.1016/j.colsurfb.2013.12.009.
- S. Parasuraman, S. Balamurugan and R. Vanishya, Overview of Safety Assessment and Toxicological Screening of Dermal Formulations, SBV J. Basic Clin. Appl. Health Sci., 2020, 3, 96–103, DOI:10.5005/jp-journals-10082-02258.
- F. M. Bezerra, O. G. Carmona, C. G. Carmona, M. J. Lis and F. F. de Moraes, Controlled release of microencapsulated citronella essential oil on cotton and polyester matrices, Cellulose, 2016, 23, 1459–1470, DOI:10.1007/s10570-016-0882-5.
- A. Lucia, A. C. Toloza, M. Fanucce, L. Fernández-Peña, F. Ortega and R. G. Rubio,
et al., Nanoemulsions based on thymol-eugenol mixtures: characterization, stability and larvicidal activity against Aedes aegypti, Bull. Insectology, 2020, 73, 153–160 Search PubMed.
- D. R. Barnard, Biological assay methods for mosquito repellents, J. Am. Mosq. Control Assoc., 2005, 21, 12–16, DOI:10.2987/8756.
- A. Matope and G. M. Foster, A bioassay method validation framework for laboratory and semi-eld tests used to evaluate vector control tools, Malar. J., 2023, 22, 289, DOI:10.21203/rs.3.rs-2706711/v1.
- C. Puglia and F. Bonina, Lipid nanoparticles as novel delivery systems for cosmetics and dermal pharmaceuticals, Expert Opin. Drug Delivery, 2012, 9(4), 429–441, DOI:10.1517/17425247.2012.666967.
- H. E. Yener, R. Erdmann, K. Jariyavidyanont, A. B. Mapossa, W. W. Focke and G. Hillrichs,
et al., Slow-DEET-Release Mosquito-Repellent System Based on Poly(butylene succinate), ACS Omega, 2022, 7, 8377–8384, DOI:10.1021/acsomega.1c05897.
- A. B. Mapossa, A. Sitoe, W. W. Focke, H. Izadi, E. L. Toit and R. Androsch,
et al., Mosquito repellent thermal stability, permeability and air volatility, Pest Manage. Sci., 2020, 76, 1112–1120, DOI:10.1002/ps.5623.
- J. I. Hare, T. Lammers, M. B. Ashford, S. Puri, G. Storm and S. T. Barry, Challenges and strategies in anti-cancer nanomedicine development: An industry perspective, Adv. Drug Delivery Rev., 2017, 108, 25–38, DOI:10.1016/j.addr.2016.04.025.
- T. C. Ezike, U. S. Okpala, U. L. Onoja, C. P. Nwike, E. C. Ezeako and O. J. Okpara,
et al., Advances in drug delivery systems, challenges and future directions, Heliyon, 2023, 9(6), e17488, DOI:10.1016/j.heliyon.2023.e17488.
- S. Hua, M. B. C. de Matos, J. M. Metselaar and G. Storm, Current trends and challenges in the clinical translation of nanoparticulate nanomedicines: Pathways for translational development and commercialization, Front. Pharmacol, 2018, 9 DOI:10.3389/fphar.2018.00790.
- A. Hafner, J. Lovrić, G. P. Lako and I. Pepić, Nanotherapeutics in the EU: An overview on current state and future directions, Int. J. Nanomed., 2014, 9, 1005–1023, DOI:10.2147/IJN.S55359.
- S. Tinkle, S. E. Mcneil, S. Mühlebach, R. Bawa, G. Borchard and Y. C. Barenholz,
et al., Nanomedicines: Addressing the scientific and regulatory gap, Ann. N. Y. Acad. Sci., 2014, 1313, 35–56, DOI:10.1111/nyas.12403.
- H. A. Luker, A critical review of current laboratory methods used to evaluate mosquito repellents, Front. Insect Sci., 2024, 4 DOI:10.3389/finsc.2024.1320138.
|
This journal is © The Royal Society of Chemistry 2024 |