DOI:
10.1039/D2NH00575A
(Communication)
Nanoscale Horiz., 2023,
8, 1106-1112
Multifunctional DNA nanoprobe for tumor-targeted synergistic therapy by integrating chemodynamic therapy with gene silencing†
Received
12th December 2022
, Accepted 24th May 2023
First published on 29th May 2023
Abstract
Due to the high complexity, diversity and heterogeneity of tumor occurrence and development, multi-mode synergistic therapy is more effective than single treatment modes to improve the antitumor efficacy. Also, multifunctional probes are crucial to realize synergistic therapy. Herein, a multifunctional DNA tetrahedron nanoprobe was ingeniously designed to simultaneously achieve chemodynamic therapy (CDT) and gene silencing for synergistic antitumor. The multifunctional DNA tetrahedron nanoprobe, DNA tetrahedron-silver nanocluster-antagomir-21 (D-sgc8-DTNS-AgNCs-Anta-21), integrated a CDT reagent (DNA-AgNCs) and miRNA-21 inhibitor (Anta-21) with a specific recognition probe (aptamer). After targeted entry in cancer cells, D-sgc8-DTNS-AgNCs-Anta-21 silenced endogenous miRNA-21 by Anta-21 and produced highly toxic ˙OH by reacting with H2O2, which induced apoptosis in the tumor cells. The targeted recognition of aptamers led to the concentration-dependent death of HeLa cells. On the contrary, the cell survival rate of normal cells was basically unaffected with an increase in the concentration of D-sgc8-DTNS-AgNCs-Anta-21. Therefore, the diverse functions, biocompatibility and programmability of DNA provide a useful and easy way to assemble multifunctional probes for synergistic therapy.
New concepts
Multifunctional nanoprobes have received great attention and have been widely explored for the diagnosis and treatment of diseases. Most of these nanoprobes are fabricated by stacking diverse building blocks with different functions, which requires sophisticated design, complicated synthesis and modification processes. At present, it is still a challenge to construct synchronized multifunctional therapeutic nanoprobes with simplicity and safety. Herein, a multifunctional DNA nanoprobe was ingeniously designed to simultaneously achieve chemodynamic therapy (CDT) and gene silencing for a synergistic antitumor effect. Different from conventional nanomaterials, the multifunctional DNA nanoprobe is easy to be precisely assembled and functionalized due to the programmability and diversity of nucleic acids. Importantly, the good biocompatibility and targeted recognition of DNA endowed the DNA nanoprobe with excellent antitumor efficacy, high specificity and good biosafety. Besides, DNA was first served as a template to synthesize metal clusters for antitumor therapy. Therefore, the diverse functions, biocompatibility and programmability of DNA provide a useful and easy way to assembly multifunctional probes for synergistic therapy.
|
Introduction
In modern society, malignant tumors pose a serious threat to human health, and thus researchers are focused on cancer treatments. Consequently, to date, several technologies have been developed, such as chemotherapy,1 CDT,2–4 photothermal therapy (PTT),5,6 photodynamic therapy (PDT),7,8 radiotherapy (RT),9,10 and gene therapy.11,12 These efforts and developments may improve the ability to combat malignant tumors. However, it is generally difficult to achieve an ideal therapeutic effect using a single treatment mode due to the high complexity, diversity and heterogeneity in the process of tumor occurrence and development. Thus, researchers have attempted the use of different combinations to explore multi-mode and multi-functional synergistic therapy.
To date, different strategies have been developed for multi-mode and multi-functional synergistic therapy. Recently, Wang et al. proposed a mesoporous magnetic copper ferrite nanoagent (CuFe2O4 NPs) for synergistically enhanced cancer therapy via multi-magnetothermal and autophagy inhibition in situ self-cycling redox nanocatalysis.13 Dong et al. developed a mesoporous ZnFe2O4 upconversion-mediated multifunctional nanoplatform (PEG/Y-UCSZ&DOX) for photo-enhanced CDT, PDT and chemotherapy.14 Liu et al. synthesized Cu-DNAzyme nanohybrids by integrating Cu2+ with DNAzyme for chemodynamic therapy and gene therapy.15 Besides, a novel pH-responsive nanoplatform based on copper sulfide-covalent organic frameworks (CuS@COFs-BSA-FA/DOX) was established for chemo/photothermal/chemodynamic synergistic therapy.16 These achievements confirm the feasibility and potential of synergistic therapy. Among them, multifunctional reagents play a crucial role in the efficacy of synergistic therapy. Generally, these multifunctional reagents are nanomaterials that need to be synthesized and functionalized. Therefore, it is important to design and assemble multifunctional nanoprobes under mild and simple synthesis conditions with good biosafety and precise targeting for synergistic antitumor therapy.
Herein, we designed a multifunctional DNA nanoprobe to achieve CDT and gene therapy simultaneously, which possessed the advantages of the diverse functions, biocompatibility, programmability and easy assembly of DNA.17–22 DNA tetrahedral nanostructures (DTNS) were employed as carriers23–25 to integrate a CDT reagent, gene inhibitor and targeted recognition probe due to their excellent biocompatibility, high nuclease stability and remarkable transmembrane capability.26–30 Antagomir as a gene inhibitor is a specially labeled and chemically modified single-stranded small RNA.31,32 However, despite the good antitumor effect and stability of antagomir, it still cannot realize controlled release due to the tissue barrier. After assembly on DTNS, antagomir can be easily delivered in cancer cells. Silver nanoclusters (AgNCs) usually have peroxidase-like activity for catalyzing H2O2 to produce ˙OH.33–36
Importantly, DNA-AgNC can be easily and rapidly synthesized within 30 min using cytosine-rich DNA as a template. Briefly, four DNA probes were carefully designed and assembled into a DNA tetrahedron, which had two sgc8 aptamers, two antagomir-21 and template for the synthesis of silver nanoclusters of DNA (DNA-AgNCs) at the four vertexes, respectively (Scheme 1). After reacting with AgNO3 and NaBH4 solution, DNA tetrahedron-silver nanocluster-antagomir-21 (D-sgc8-DTNS-AgNC-Anta-21) was formed. Both aptamers could exert multivalent effects to achieve the efficient and precise targeted delivery of DNA-AgNCs and Anta-21. The Fenton-like reaction of DNA-AgNCs with intracellular H2O2 could kill tumor cells by CDT. Meanwhile, Anta-21 silenced endogenous miRNA-21 in tumor cells to induce apoptosis. Thus, CDT and gene silencing could synergistically accelerate apoptosis in tumor cells. Therefore, the diverse functions, biocompatibility and programmability of DNA provide a useful and easy way to assemble multifunctional probes for synergistic therapy.
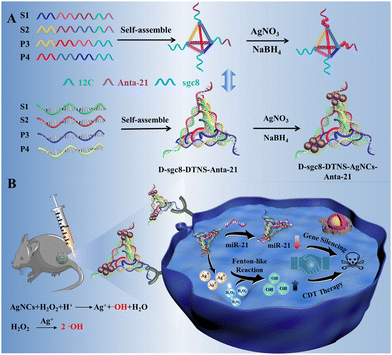 |
| Scheme 1 Schematic illustration of multifunctional DNA nanoprobe-mediated chemodynamic/gene therapy. | |
Results and discussion
To achieve efficient and targeted anti-tumor therapy, we used a DNA tetrahedron to combine CDT with gene therapy. As shown in Scheme 1, DTNS was prepared via a simple thermal annealing method with four customized single-stranded nucleic acid chains (S1, S2, P3, and P4) (Scheme 1A). The sequence of DNAs is shown in Table S1. The four DNA probes were carefully designed to contain different domains to assembly into rigid tetrahedra. The end of S1 and S2 contained 12c (green) as the template of DNA-AgNC and Anta-21 (purple) for gene silencing, respectively. The end of P3 and P4 contained sgc8 aptamers (blue) for the targeted recognition of PTK7 on the surface of tumor cells. Thus, the assembly of DTNS caused the two sgc8 aptamers, two Anta-21 and the template for the synthesis of DNA-AgNC (12C) to be located at the four vertexes, respectively, for the construction of the multifunctional DNA tetrahedron nanoprobe (D-sgc8-DTNS-AgNCs-antagomir-21) (Scheme 1A). Due to the specificity of the tumor microenvironment (TME), the DNA-AgNCs of D-sgc8-DTNS-AgNCs-Anta-21 could react with intracellular H2O2 to produce highly toxic ˙OH, which could induce apoptosis in tumor cells. Meanwhile, Anta-21 silenced endogenous miRNA-21 in the tumor cells to kill them (Scheme 1B). Therefore, CDT and gene silencing effectively killed the tumor cells. The multifunctional probe can improve the efficacy of antitumor by synergistic therapy.
Characterization of D-sgc8-DTNS and D-sgc8-DTNS-AgNCs-Anta-21
Due to the important role of D-sgc8-DTNS-AgNCs-Anta-21 in tumor treatment, the assembly of D-sgc8-DTNS and the formation of D-sgc8-DTNS-AgNCs-Anta-21 were characterized by PAGE, fluorescence, SEM and TEM. Firstly, the assembly of D-sgc8-DTNS was verified by PAGE (Fig. 1A). Lanes 1, 2, 3 and 4 correspond to the bands of P1, P2, P3 and P4, respectively. Lanes 5 represents the bands of a P1/P2/P3/P4 mixture. The bands of P1, P2, P3 and P4 disappeared and new bands appeared when P1, P2, P3 and P4 were co-incubated, indicating that P1, P2, P3 and P4 successfully assembled to form D-sgc8-DTNS (lane 5). Secondly, the size and morphology of D-sgc8-DTNS-AgNCs-Anta-21 were characterized by TEM. The TEM results showed (Fig. 1B and C) that D-sgc8-DTNS-AgNCs-Anta-21 was spherical with an average diameter of about 15 nm. The successful formation of D-sgc8-DTNS-AgNCs-Anta-21 was further verified by AFM images. As shown in Fig. S1 (ESI†), the prepared DTNSs were a vertebral-like nanostructure with a diameter of 25 nm (Fig. S1A and B, ESI†). Also, the size of the prepared DTNS-AgNCs is consistent with the TEM results (Fig. S1C and D, ESI†).
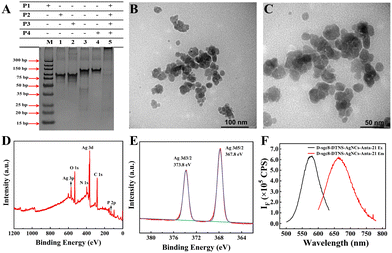 |
| Fig. 1 (A) Gel electrophoresis to verify the formation of D-sgc8-DTNS. (B and C) TEM image of D-sgc8-DTNS-AgNCs-Anta-21. XPS spectrum and (E) high-resolution XPS spectra of Ag 3d of D-sgc8-DTNS-AgNCs. (F) Fluorescence excitation/emission spectra of D-sgc8-DTNS-AgNCs-Anta-21. | |
Thirdly, X-ray photoelectron spectroscopy (XPS) was performed to further characterize the element distribution of D-sgc8-DTNS-AgNCs, which mainly contained Ag, C, N, O, and P (Fig. 1D). According to the Ag3d spectrum (Fig. 1E), the peaks at the binding energies of 373.8 eV and 367.8 eV correspond to Ag 3d3/2 and Ag 3d5/2, respectively. Thus, this indicates that mainly Ag0 is present in DNA-AgNCs. In addition, the fluorescence spectrum of DNA-AgNCs was studied. The maximum excitation of D-sgc8-DTNS-AgNCs-Anta-21 was observed at 575 nm and its maximum emission at 665 nm (Fig. 1F). The UV-vis absorbance spectra of D-sgc8-DTNS-AgNCs-antagomir-21 display an absorption peak at around 450 nm (Fig. S2, ESI†). The absorption and fluorescence spectra are in accordance with the previous reports,37–40 suggesting the formation of DNA-AgNCs.
Targeted recognition of HeLa cells by D-sgc8-DTNS
The multivalent effect and specific recognition function of D-sgc8-DTNS-AgNCs-Anta-21 play a crucial role in the targeted and efficient anti-tumor efficacy. Therefore, SG I was used as a fluorescent tracer to investigate the multivalent effect and targeted recognition of aptamers. Firstly, the concentration of D-sgc8-DTNS was optimized. Fig. S3 (ESI†) demonstrates that the optimal concentration of D-sgc8-DTNS was 100 nM when the concentration of SG I was 0.5×. Upon binding to dsDNA, SG emitted strong green fluorescence. Secondly, a tetrahedron DNA without aptamer (DTNS) and a tetrahedron DNA with a single aptamer (S-sgc8-DTNS) were designed to verify the targeted recognition and multivalent effect of the aptamer. No green fluorescence was observed in both the HeLa cells and normal cells when DTNS was incubated with both types of cells. However, green fluorescence was observed in the HeLa cells when S-sgc8-DTNS and D-sgc8-DTNS were incubated with HeLa cells and normal cells for 3 h, respectively. Specifically, the aptamer targeted the HeLa cells because PTK7 is overexpressed on the surface of cancer cells. Compared with the S-sgc8-DTNS group, intense green fluorescence was observed in the HeLa cells after incubation with SG I-embedded D-sgc8-DTNS for 3 h. Conversely, neither DTNS, S-sgc8-DTNS nor D-sgc8-DTNS could efficiently enter HL-7702 cells. Thus, Fig. 2 solidly confirmed the targeted recognition ability of the aptamer to HeLa cells and the multivalent effect of the dual aptamer improved the efficiency of targeted recognition. To more clearly demonstrate the difference in fluorescence intensity, the mean fluorescent signal under different conditions was calculated using ImageJ (Fig. S4, ESI†). Therefore, D-sg8-DTNS specifically targeted cancer cells due to the multivalent effect and specific recognition of dual aptamers.
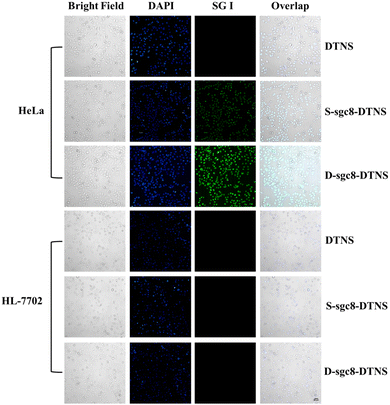 |
| Fig. 2 Fluorescence imaging of HeLa cells and HL-7702 cells under different conditions to verify the targeted recognition by the aptamer. Scale bar, 50 μm. | |
In vitro antitumor therapy of D-sgc8-DTNS-AgNCs-Anta-21
Herein, we design a multifunctional DNA probe to achieve targeted delivery and efficient anti-tumor effect. Fig. 3A verifies the antitumor efficacy of D-sgc8-DTNS-AgNCs-Anta-21. Specifically, the PI Staining Kit assay was applied to identify dead cells. The apoptotic HeLa cells were stained by PI to demonstrate red fluorescence. Firstly, the effect of D-sgc8-DTNS on the growth of tumor cells was studied. Fig. 3A shows the absence of red fluorescence in the PBS- and D-sgc8-DTNS-treated groups. Specifically, D-sgc8-DTNS itself could not induce death in the HeLa cells. Compared with the D-sgc8-DTNS-treated group, the D-sgc8-DTNS-AgNCs and D-sgc8-DTNS-Anta-21 groups alone exhibited red fluorescence, indicating that they both induced apoptosis in the HeLa cells. Significantly, the D-sgc8-DTNS-AgNCs-Anta-21-treated group showed the strongest red fluorescence, indicating that they could notably kill HeLa cells due to the synergistic effect of CDT and gene silencing. The corresponding fluorescence signal was calculated using ImageJ (Fig. S5, ESI†). Thus, the D-sgc8-DTNS-AgNCs-Anta-21-treated group could notably kill HeLa cells due to the synergistic effect. Secondly, the antitumor efficacy of D-sgc8-DTNS-AgNCs-antagomir-21 was further studied (Fig. S6, ESI†). The number of PI-stained HeLa cells increased with an increase in the concentration of D-sgc8-DTNS-AgNCs-Anta-21. Meanwhile, with the extension of the incubation time, the red fluorescence in the HeLa cells was also greatly enhanced. Thus, D-sgc8-DTNS-AgNCs-Anta-21 could effectively induce apoptosis in HeLa cells. More importantly, D-sgc8-DTNS-AgNCs-Anta-21 had little effect on the cell viability of HL-7702 cells. This is mainly attributed to the specific recognition of the dual sgc8 aptamer for HeLa cells. Thirdly, the cytotoxicity of D-sgc8-DTNS-AgNCs-Anta-21 was evaluated using the MTT assay. HeLa cells and HL-7702 cells were treated with different concentrations of D-sgc8-DTNS-AgNCs, D-sgc8-DTNS-Anta-21 and D-sgc8-DTNS-AgNCs-Anta-21 for 24 h, respectively. As the concentration increased from 5 nM to 500 nM, the cell survival rate of HL-7702 cells remained basically unchanged (Fig. 3B), while the HeLa cell viability was obviously inhibited (Fig. 3C). More importantly, compared with the D-sgc8-DTNS-AgNCs- and D-sgc8-DTNS-Anta-21-treated groups, the D-sgc8-DTNS-AgNCs-Anta-21-treated HeLa cells had the lowest cell viability. The MTT assay confirmed that D-sgc8-DTNS-AgNCs-Anta-21 had no cytotoxicity to HL-7702 cells, while the death of the HeLa cells was highly dependent on the concentration of D-sgc8-DTNS-AgNCs-Anta-21. It should be noted that the antitumor efficacy of D-sgc8-DTNS-AgNCs-Anta-21 was the best due to the synergistic effect of DNA-AgNCs and Anta-21. Finally, the antitumor efficacy of D-sgc8-DTNS-AgNCs-Anta-21 was further confirmed by flow cytometry. Fig. 3D and Fig. S7 (ESI†) verify that the growth of the D-sgc8-DTNS-treated HeLa cells was almost identical to that of the blank group after incubation of 12 h. The results again verified that D-sgc8-DTNS had no effect on the cell viability of HeLa cells. In contrast, the apoptosis rate of the D-sgc8-DTNS-AgNCstreated HeLa cells and D-sgc8-DTNS-Anta-21-treated HeLa cells was 24.17% and 19.57%, respectively. However, 50 nM D-sgc8-DTNS-AgNCs-Anta-21 induced 28.54% apoptosis in the HeLa cells, indicating that the synergistic effect of DNA-AgNCs and Anta-21 improved the apoptosis rate of HeLa cells. In the case of the HL-7702 cells, 50 nM D-sgc8-DTNS-AgNCs, D-sgc8-DTNS-Anta-21 and D-sgc8-DTNS-AgNCs-Anta-21 had little effect on their growth within 12 h. The experimental results again confirmed that D-sgc8-DTNS-AgNCs-Anta-21 could induce apoptosis in HeLa cells with high efficiency and specificity, while it had almost no effect on normal cells.
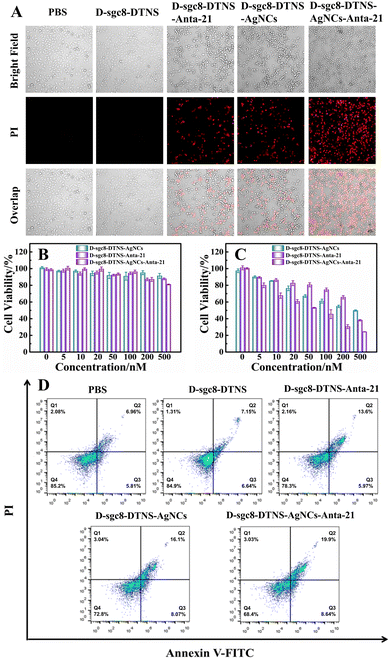 |
| Fig. 3 Fluorescence images (A) of HeLa cells in different treatment groups. The concentration of D-sgc8-DTNS, D-sgc8-DTNS-Anta-21 and D-sgc8-DTNS-AgNCs-Anta-21 is 100 nM, respectively. Red fluorescence indicates the PI-stained dead cells. Scale bar, 50 μm. Cytotoxicity of D-sgc8-DTNS-AgNCs, D-sgc8-DTNS-Anta-21 and D-sgc8-DTNS-AgNCs-Anta-21-treated (B) HL-7702 and (C) HeLa cells. (D) Apoptosis in HeLa cells using Annexin V-FITC/PI assay after different treatments for 12 h. | |
Verification of gene silence of D-sgc8-DTNS-Anta-21
Anta-21 can silence endogenous miRNA-21 in cancer cells, inhibit the migration and invasion of tumor cells, and eventually induce cell apoptosis. Firstly, the influence of DNA-AgNC on Anta-21 was studied given that Anta-21 is adjacent to DNA-AgNCs. The principle is shown in Fig. 4A. The fluorescent-labeled DNA P1 and P2 probes were named P1-FAM and P2-FAM, respectively. Then, the FAM-labeled DNA tetrahedron (D-sgc8-DTNS-FAM) was assembled by mixing P1-FAM, P2-FAM and P3 with P4. After reacting with AgNO3 and NaBH4 solution, D-sgc8-DTNS-AgNCs-FAM was obtained. Finally, D-sgc8-DTNS- AgNCs-FAM reacted with BHQ1-cDNA, which is the quencher-tagged complementary DNA of Anta-21, leading to the fluorescence quenching of FAM. In the presence of miRNA-21, the fluorescence FAM will recover to verify the effective hybridization between miRNA-21 and Anta-21. Fig. 4B demonstrates that D-sgc8-DTNS-AgNCs-FAM emits strong fluorescence at 520 nm after excitation at 488 nm. Meanwhile, D-sgc8-DTNS-AgNCs had no fluorescence peak at 520 nm when it was excited at 488 nm, indicating that it would not cause spectral interference to the fluorescence emission of FAM. Fig. 4C indicates that the fluorescence of FAM was effectively quenched by BHQ1, and then recovered in the presence of miRNA-21. Thus, D-sgc8-DTNS-AgNCs could not affect the interaction between miRNA-21 and Anta-21. Fig. 4D further verifies the specific recognition of miRNA-21. Several miRNAs were designed as negative controls, including miR-20a, miR-222, miR-223, and three-base mismatched miR-21 (TM miR-21). The fluorescence intensity of FAM remained almost unchanged when DTNS was treated with these miRNAs. Upon the addition of miRNA-21, there was an obvious fluorescence signal at 520 nm. Thus, Fig. 4 confirms that the adjacent DNA-AgNCs did not affect the specific gene silence of miRNA-21 by Anta-21. Next, intracellular gene silence of miRNA-21 by Anta-21 was studied by fluorescence imaging. Fig. 4E shows the fluorescence images of the complexes of D-sgc8-DTNS-AgNCs-FAM and BHQ1-cDNA co-incubated with HeLa cells and HL-7702 cells, respectively. The HeLa cells presented green fluorescence, while there was no green fluorescence in HL-7702 cells. This is due to the fact that miRNA-21 is widely overexpressed in various tumor cells and is involved in tumorigenesis and progression. The corresponding fluorescence signal was calculated using ImageJ (Fig. S8, ESI†).
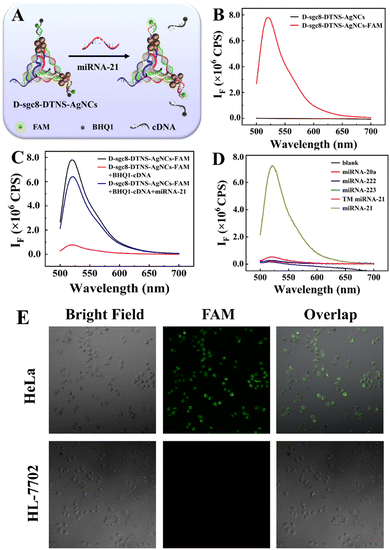 |
| Fig. 4 (A) Verification of the interaction between miRNA-21 and D-sgc8-DTNS-AgNC. (B) Fluorescence spectra of D-sgc8-DTNS-AgNCs and D-sgc8-DTNS-AgNCs-FAM. (C) Fluorescence spectra of D-sgc8-DTNS-AgNCs-FAM under different conditions. (D) Fluorescence spectra of D-sgc8-DTNS-AgNCs-FAM in the presence of different miRNAs. Confocal fluorescence images (E) of D-sgc8-DTNS-AgNCs-FAM and BHQ1-cDNA complex after incubation with HeLa cells and HL-7702 cells. The concentration of D-sgc8-DTNS-AgNCs and D-sgc8-DTNS-AgNCs-FAM is 100 nM, respectively. Scale bar, 50 μm. | |
Verification of chemodynamic therapy of D-sgc8-DTNS-AgNCs
As described in Scheme 1, D-sgc8-DTNS-AgNCs-antagomir-21 contained DNA-AgNC and Anta-21 to simultaneously achieve CDT and gene silence. Fig. 4 confirms the feasibility of antagomir-21-induced gene silencing. Subsequently, the verification of CDT was performed. According to Scheme 1, DNA-AgNC on D-sgc8-DTNS-AgNCs-Anta-21 reacted with intracellular H2O2 to produce highly toxic ˙OH. Firstly, TMB/H2O2 was used to verify ˙OH. In acidic medium, the ˙OH will oxidize the colorless TMB solution to blue (Fig. 5A). Moreover, the ESR spectra also demonstrated the generation of ˙OH when DTNS-AgNCs reacted with H2O2 (characteristic peaks, 1
:
2:2
:
1) (Fig. 5B).
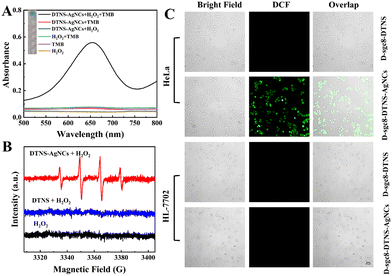 |
| Fig. 5 (A) Verification of ˙OH generation under different conditions. The concentrations of DTNS-AgNCs, H2O2 and TMB are 2 μM, 5 mM and 1.0 mM, respectively. (B) ESR spectra for verifying ˙OH. (C) Verification of ˙OH generation inside HeLa cells and HL-7702 cells by ROS staining. Scale bar, 50 μm. | |
Secondly, the influence of pH on the generation of ˙OH was studied. The pH in lysosomes/endosomes is around 5.0, that of the TME is around 6.5 and pH 7.4 was used to mimic the physiological conditions. Fig. S9 (ESI†) shows that the ability of DTNS-AgNCs to generate ˙OH was dependent on the pH. The acidic medium of tumor tissues may benefit the formation of ROS, leading to more efficient CDT. Thirdly, the stability of DNA-AgNCs in blood serum was studied. Fig. S10 (ESI†) demonstrates that the stability of DNA-AgNCs slowly decreased with the prolonging of the storage time. Its catalytic ability still remained at 71.7% within 10 days in diluted serum. Thus, the multifunctional DNA nanoprobe possesses favorable stability in blood serum. Finally, the ROS staining kit was used to verify the intracellular generation of ROS. If ROS is present, green fluorescence is observed inside the cell. Fig. 5C clearly demonstrates green fluorescence inside the HeLa cells after incubation with 50 nM D-sgc8-DTNS-AgNCs for 10 h. Specifically, the level of ROS in the HeLa cells increased when D-sgc8-DTNS-AgNCs was incubated with HeLa cells. On the contrary, no green fluorescence was observed inside the HL-7702 cells after incubation with 50 nM D-sgc8-DTNS-AgNCs for 10 h. Significantly, HeLa cells and HL-7702 cells did not exhibit green fluorescence when D-sgc8-DTNS-AgNCs was replaced by D-sgc8-DTNS. Therefore, fluorescence imaging consistently verified the generation of ˙OH by reaction between DNA-AgNCs and intracellular H2O2. More importantly, it also confirmed the specific recognition of HeLa cells by sgc8 aptamers because ROS were only generated inside the HeLa cells. The corresponding fluorescence signal was calculated using ImageJ (Fig. S11, ESI†).
Conclusions
In summary, we designed and prepared a multifunctional DNA nanoprobe to improve the antitumor efficacy. The two sgc8 aptamers of D-sgc8-DTNS-AgNCs-Anta-21 targeted tumor cells and improved the recognition efficiency through multivalent effects. Due to the integration of DNA-AgNCs with Anta-21 on the tetrahedron DNA, they were specifically delivered inside cancer cells without additional carriers. D-sgc8-DTNS-AgNCs-Anta-21 specifically and efficiently induced apoptosis in tumor cells through DNA-AgNCs/H2O2 Fenton-like reaction and silencing endogenous miRNA-21. The in vitro study confirmed the targeted and efficient antitumor efficacy of the multifunctional DNA nanoprobe with good biocompatibility to normal cells. Therefore, the diverse functions, biocompatibility and programmability of DNA provide a useful and easy way to assembly multifunctional probes and realize antitumor effects, showing great potential for biomedical studies.
Conflicts of interest
There are no conflicts to declare.
Acknowledgements
We acknowledge financial support through grants from the National Natural Science Foundation of China (No. 21974083), the Fundamental Research Funds for the Central Universities (GK 202002002) and Natural Science Basic Research Program of Shaanxi (No. 2021JZ-23).
References
- S. Liu, A. R. Khan, X. Yang, B. Dong, J. Ji and G. Zhai, J. Controlled Release, 2021, 335, 1–20 CrossRef CAS PubMed.
- C. Zhang, W. Bu, D. Ni, S. Zhang, Q. Li, Z. Yao, J. Zhang, H. Yao, Z. Wang and J. Shi, Angew. Chem., Int. Ed., 2016, 55, 2101–2106 CrossRef CAS PubMed.
- B. Ma, S. Wang, F. Liu, S. Zhang, J. Duan, Z. Li, Y. Kong, Y. Sang, H. Liu, W. Bu and L. Li, J. Am. Chem. Soc., 2019, 141, 849–857 CrossRef CAS PubMed.
- Z. Tang, Y. Liu, M. He and W. Bu, Angew. Chem., Int. Ed., 2019, 58, 946–956 CrossRef CAS PubMed.
- B. N. Im, H. Shin, B. Lim, J. Lee, K. S. Kim, J. M. Park and K. Na, Biomaterials, 2021, 271, 120745 CrossRef CAS PubMed.
- C. Xu and K. Pu, Chem. Soc. Rev., 2021, 50, 1111–1137 RSC.
- D. W. Zheng, B. Li, C. X. Li, J. X. Fan, Q. Lei, C. Li, Z. Xu and X. Z. Zhang, ACS Nano, 2016, 10, 8715–8722 CrossRef CAS PubMed.
- W. Sun, L. Luo, Y. Feng, Y. Qiu, C. Shi, S. Meng, X. Chen and H. Chen, Adv. Mater., 2020, 32, e2000377 CrossRef PubMed.
- C. Hennequin, S. Guillerm, S. Wong and L. Quéro, Cancer Radiother., 2018, 22, 367–371 CrossRef CAS PubMed.
- S. Ohashi, S. Miyamoto, O. Kikuchi, T. Goto, Y. Amanuma and M. Muto, Gastroenterology, 2015, 149, 1700–1715 CrossRef PubMed.
- L. M. Riedmayr, K. S. Hinrichsmeyer, N. Karguth, S. Böhm, V. Splith, S. Michalakis and E. Becirovic, Nat. Protoc., 2022, 17, 781–818 CrossRef CAS PubMed.
- C. Jia, Y. Guo and F.-G. Wu, Small, 2022, 18, 2103868 CrossRef CAS PubMed.
- M. Wang, Q. Chen, D. Xu, Z. Yang, J. Chen, Y. Zhang and H. Chen, Nano Today, 2022, 43, 101374 CrossRef CAS.
- S. Dong, J. Xu, T. Jia, M. Xu, C. Zhong, G. Yang, J. Li, D. Yang, F. He, S. Gai, P. Yang and J. Lin, Chem. Sci., 2019, 10, 4259–4271 RSC.
- C. Liu, Y. Chen, J. Zhao, Y. Wang, Y. Shao, Z. Gu, L. Li and Y. Zhao, Angew. Chem., Int. Ed., 2021, 60, 14324–14328 CrossRef CAS PubMed.
- S. Wang, Y. Pang, S. Hu, J. Lv, Y. Lin and M. Li, Chem. Eng. J., 2023, 451, 138864 CrossRef CAS.
- M. R. Jones, N. C. Seeman and C. A. Mirkin, Science, 2015, 347, 1260901 CrossRef PubMed.
- Y. Krishnan and N. C. Seeman, Chem. Rev., 2019, 119, 6271–6272 CrossRef CAS PubMed.
- S. Liu, Q. Jiang, X. Zhao, R. Zhao, Y. Wang, Y. Wang, J. Liu, Y. Shang, S. Zhao, T. Wu, Y. Zhang, G. Nie and B. Ding, Nat. Mater., 2021, 20, 421–430 CrossRef CAS PubMed.
- Z. Wang, L. Song, Q. Liu, R. Tian, Y. Shang, F. Liu, S. Liu, S. Zhao, Z. Han, J. Sun, Q. Jiang and B. Ding, Angew. Chem., Int. Ed., 2021, 60, 2594–2598 CrossRef CAS PubMed.
- H. Lee, A. K. Lytton-Jean, Y. Chen, K. T. Love, A. I. Park, E. D. Karagiannis, A. Sehgal, W. Querbes, C. S. Zurenko, M. Jayaraman, C. G. Peng, K. Charisse, A. Borodovsky, M. Manoharan, J. S. Donahoe, J. Truelove, M. Nahrendorf, R. Langer and D. G. Anderson, Nat. Nanotechnol., 2012, 7, 389–393 CrossRef CAS PubMed.
- S. M. Douglas, I. Bachelet and G. M. Church, Science, 2012, 335, 831–834 CrossRef CAS PubMed.
- C. Angell, S. Xie, L. Zhang and Y. Chen, Small, 2016, 12, 1117–1132 CrossRef CAS PubMed.
- D. Xiao, Y. Li, T. Tian, T. Zhang, S. Shi, B. Lu, Y. Gao, X. Qin, M. Zhang, W. Wei and Y. Lin, ACS Appl. Mater. Interfaces, 2021, 13, 6109–6118 CrossRef CAS PubMed.
- J. Zhuang, J. Tan, C. Wu, J. Zhang, T. Liu, C. Fan, J. Li and Y. Zhang, Nucleic Acids Res., 2020, 48, 8870–8882 CrossRef CAS PubMed.
- Q. Tang, L. Zhang, X. Tan, L. Jiao, Q. Wei and H. Li, Biosens. Bioelectron., 2019, 133, 94–99 CrossRef CAS PubMed.
- L. He, D. Q. Lu, H. Liang, S. Xie, C. Luo, M. Hu, L. Xu, X. Zhang and W. Tan, ACS Nano, 2017, 11, 4060–4066 CrossRef CAS PubMed.
- Y. Zhang, Y. Deng, C. Wang, L. Li, L. Xu, Y. Yu and X. Su, Chem. Sci., 2019, 10, 5959–5966 RSC.
- X. Xie, X. Shao, W. Ma, D. Zhao, S. Shi, Q. Li and Y. Lin, Nanoscale, 2018, 10, 5457–5465 RSC.
- T. Zhang, T. Tian, R. Zhou, S. Li, W. Ma, Y. Zhang, N. Liu, S. Shi, Q. Li, X. Xie, Y. Ge, M. Liu, Q. Zhang, S. Lin, X. Cai and Y. Lin, Nat. Protoc., 2020, 15, 2728–2757 CrossRef CAS PubMed.
- J. Krützfeldt, N. Rajewsky, R. Braich, K. G. Rajeev, T. Tuschl, M. Manoharan and M. Stoffel, Nature, 2005, 438, 685–689 CrossRef PubMed.
- J. Ha, M. Kim, Y. Lee and M. Lee, Nanoscale, 2021, 13, 14745–14759 RSC.
- Y. Tao, M. Li, J. Ren and X. Qu, Chem. Soc. Rev., 2015, 44, 8636–8663 RSC.
- Y. Cai, J. Wang, L. Niu, Y. Zhang, X. Liu, C. Liu, S. Yang, H. Qi and A. Liu, Analyst, 2021, 146, 4630–4635 RSC.
- A. Khataee, M. Haddad Irani-Nezhad, J. Hassanzadeh and S. Woo, Joo, J. Colloid Interface Sci., 2018, 515, 39–49 CrossRef CAS PubMed.
- A. Elgamouz, A. Kawde, S. S. Alharthi, M. Laghoub, D. Miqlid, C. Nassab, K. Bajou and S. P. Patole, Colloids Surf., A, 2022, 647, 129035 CrossRef CAS.
- Y. Guo, F. Shen, Y. Cheng, Y. Xie, H. Yu, W. Yao, H. W. Li, H. Qian and R. Pei, Nanoscale, 2018, 10, 11517–11523 RSC.
- H. Que, D. Zhang, B. Guo, T. Wang, H. Wu, D. Han and Y. Yan, Biosens. Bioelectron., 2019, 143, 111610 CrossRef CAS PubMed.
- P. Shah, S. W. Choi, R. Nagda, R. Geczy, S. K. Cho, Y. J. Bhang, T. H. Kim, T. Y. Song, P. H. Lee, J. H. Kang, P. W. Thulstrup, M. J. Bjerrum, I. L. Jung and S. W. Yang, Nanoscale, 2018, 10, 20717–20722 RSC.
- D. Huard, A. Demissie, D. Kim, D. Lewis, R. M. Dickson, J. T. Petty and R. L. Lieberman, J. Am. Chem. Soc., 2019, 141, 11465–11470 CrossRef CAS PubMed.
|
This journal is © The Royal Society of Chemistry 2023 |