Microplastics in ecosystems: their implications and mitigation pathways†
Received
16th September 2021
, Accepted 6th February 2022
First published on 8th February 2022
Abstract
Microplastic (MP) pollution is an emerging threat to terrestrial and aquatic ecosystems. It is abundant, environmentally persistent, and complex. Environmental, economic, and societal concerns over the effect of MP pollution in ecosystems have attracted enormous attention for research on alternatives and potential remediation options. Plastic/MP pollution in aquatic ecosystems has been extensively studied and summarized; however, studies on terrestrial ecosystems are limited. Neither recent technological advances in the remediation of MP pollution nor their economic and societal implications have been thoroughly examined. This study compiled information on MP pollution in ecosystems and food chains, emphasizing the terrestrial ecosystem, recent technological advances, economic and societal implications, and the remediation of microplastic pollution. The perspectives of future activities have also been discussed and a potential remediation pathway has been outlined. MPs are pervasive in all channels (soil, water and atmosphere) of human interactions and hazardous to biota in ecosystems, eventually contaminating food systems and affecting human health. Leaked plastics, plastic-containing products (biosolids, wastewater, fertilizers, and pesticides), and plastic mulch used in agriculture, polyamide fabrics, and cosmetics products are the major sources of MP pollution. The development of alternatives to conventional plastics and materials that can abate or minimize the problems associated with MPs and the improvement in waste management systems to stop plastic waste leakage into ecosystems as well as cleanup drives are critical to eradicating MPs. Biodegradable plastic is recognized as an alternative to conventional plastic as it degrades faster than conventional plastics and is more prone to microorganisms. Biodegradable plastics coupled with bioremediation (eradicating MPs by using microorganisms) of MPs show a potential means to eradicate problems associated with MPs polluting ecosystems. Consequently, biodegradable plastics that are produced from non-edible biomass such as algae can be a potential pathway to eradicate MP pollution for sustainable ecosystems. Therefore, comprehensive studies are essential to assess the environmental, economic and social impacts of biodegradable plastics and bioremediation of MPs in ecosystems to avoid any potential risk to ecosystems and health.
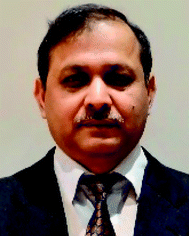 Poritosh Roy | Dr Poritosh Roy is an Agricultural Engineer (P. Eng). His areas of research interest are value-added product development from biomass (thermochemical and biochemical conversion, co-processing), bio-based economy, bioprocessing & bioreactor design, food processing, industrial symbiosis, life cycle assessment and life cycle costing of agri-food industries. He has authored/co-authored 58 peer-reviewed research articles and 5 book chapters. He served as a Guest Editor for special issues of Sustainability (MDPI) and AIMS Energy (AIMS Press). He is an Editorial Board Member of ‘AIMS Energy’ and the ‘Journal of Food Science and Nutrition Therapy’. Currently, Dr Roy is working as a researcher at the Bioproducts Discovery and Development Centre (BDDC) and Special Graduate Faculty at the School of Engineering, University of Guelph, Ontario, Canada. |
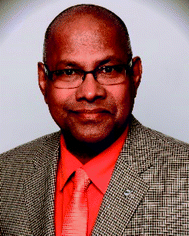 Amar K. Mohanty | Prof. Amar Mohanty is a Distinguished Research Chair in Sustainable Biomaterials at the Ontario Agriculture College and the Director of the Bioproducts Discovery and Development Centre. He is a Professor in the Department of Plant Agriculture and School of Engineering at the University of Guelph, Ontario, Canada. Dr Mohanty is the Editor-in-Chief of Sustainable Composites @ Composites Part C – Open Access. He is one of the most cited researchers worldwide with more than 800 publications to his credit, including 434 peer-reviewed journal papers, 6 edited books, over 400 conference presentations, 25 book chapters, and 67 patents awarded/applied (Google Scholar citations 42,757, h-index 93 as of December 4, 2021). He has received many awards, the most recent one being the prestigious Miroslaw Romanowski Medal in 2021 for his significant scientific contributions to the resolution of environmental problems from the Royal Society of Canada. He has also received the Synergy Award for Innovation from the Natural Sciences and Engineering Research Council of Canada (NSERC), the Andrew Chase Forest Products Division Award from the American Institute of Chemical Engineers (AIChE) and the Lifetime Achievement Award from the BioEnvironmental Polymer Society (BEPS). Prof. Mohanty is a Fellow of the Royal Society of Canada, the American Institute of Chemical Engineers, the Royal Society of Chemistry (UK) and the Society of Plastic Engineers. He is a pioneer in advanced biomaterials research with lifelong dedication to developing sustainable materials to reduce the environmental impacts of plastics. His vision of a circular economy and innovation in bioproducts for mitigating climate change has motivated a number of discoveries. |
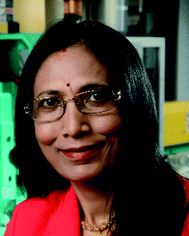 Manjusri Misra | Dr Manjusri Misra is a Professor and Tier 1 Canada Research Chair (CRC) in Sustainable Biocomposites in the School of Engineering and holds a joint appointment in the Department of Plant Agriculture at the University of Guelph. As well, she is the Research Program Director of the Bioeconomy Panel for the Ontario Agri-Food Innovation Alliance, a program between the Ontario Ministry of Agriculture and Rural Affairs (OMAFRA) and the University of Guelph. Dr Misra completed her Bachelors, Master's, MPhil and PhD from Ravenshaw College at Utkal University in India majoring in Chemistry with a specialization in Polymer Chemistry and Natural Fibers during her graduate program. Dr Misra's current research focuses primarily on novel bio-based composites and nanocomposites from agricultural, forestry and recycled resources for a sustainable bioeconomy moving towards a circular economy. She has authored more than 750 publications, including 425 peer-reviewed journal papers, 21 book chapters, and 53 patents. She was the editor or co-editor of 4 books in the area of biocomposites and nano-composites. She is a Fellow of the Royal Society of Chemistry (UK), the American Institute of Chemical Engineers (AIChE), and the Society of Plastic Engineers (SPE). Dr Misra has received many awards including the Synergy Award for Innovation from the Natural Sciences and Engineering Research Council of Canada (NSERC); the Andrew Chase Forest Products Division Award from the American Institute of Chemical Engineers and the Lifetime Achievement Award from the BioEnvironmental Polymer Society (BEPS). In 2020, she was selected as one of Canada's Most Powerful Women: Top 100 Award Winner in the Manulife Science and Technology category from the Women Executive Network. |
Environmental significance
The growing concerns about the environmental, economic, and societal impacts of microplastics have drawn enormous attention towards methods that can help eradicate microplastics from ecosystems. Although plastic/microplastic pollution mitigation strategies have been extensively studied for aquatic ecosystems, terrestrial ecosystems and food systems are both understudied. Technological advances in the plastic sector should have strategies to eradicate microplastics. This study summarizes the role of microplastics centering around their presence in terrestrial ecosystems and food systems, the economic and societal implications, and recent technological advances to combat their pollution. A potential microplastic remediation pathway could include bioremediation coupled with biodegradable plastic from renewable sources. The use of a remediation pathway would be a potential method to eradicate microplastic pollution from ecosystems.
|
Introduction
Plastic products have become an integral part of every human activity because of their light weight and convenience, thus becoming ubiquitous in all facets of the economy.1 The increasing demand for plastics leads to increasing production and waste generation, which has created enormous problems, especially in the form of single-use plastics. In 2018, global plastic production was 359 million tonnes,2,3 and its production is predicted to increase due to growing populations and increasing demands. The packaging sector is the main contributor to total global plastic waste, followed by textiles, consumer products and other sectors.4
Municipal solid waste (MSW) generation is predicted to increase by 70% by 2050.5 Plastics in MSW are also increasing dramatically with the increasing use of plastic products, especially single-use plastics. For example, in the United States of America, plastics in MSW went from 25.6 million tonnes in 2000 to 35.4 million tonnes in 2017.6 Canada produces about 3.3 million tonnes of plastic waste each year and sends 86% (2.8 million tonnes) to landfills.7–9 In Canada, the major sources of waste plastics are packaging, auto and electronics industries, agriculture, etc. Common agricultural plastic waste is plastic mulch, bale/silage wraps, bags, greenhouse film, containers, etc.10 Biodegradable plastic mulch has also been used in agriculture to mitigate the problems associated with the disposal of used plastic mulch.11 The use of plastic mulch in agriculture is also growing. For example, in China, plastic mulching increased from 0.6 million tonnes in 1991 to 2.6 million tonnes in 2015.12 Annually, Canadian agriculture uses 40
000 tonnes of plastics; however, only about 5000 tonnes of this plastic is recycled.13 Plastics used in agriculture are known to be one of the major sources of plastic pollution in soil or agroecosystems.
The benefit of plastics in society is undeniable; however, mismanaged waste plastics become hazardous to ecosystems. Growing plastic pollution has created enormous challenges to ecosystems. Globally, mismanaged plastic waste is predicted to be 69.1 million tonnes in 2025,3 which is expected to end up in landfills or in the oceans, which eventually fragments/degrades into microplastics (MPs) and finally into nanoplastics (NPs). Annually, about 4–23 times more plastic waste is released into terrestrial ecosystems compared to marine ecosystems.12 Plastic waste and MPs in aquatic ecosystems are creating enormous problems for the biota in aquatic ecosystems such as endangered polychaetes, crustaceans, zooplankton, etc.; thus, the marine biodiversity.14 In terrestrial ecosystems, it affects soil biota, seed germination, plant growth and plant productivity.15–17
The primary sources of MPs and NPs are plastic powder used in cosmetics, paint and coating, and detergents; however, waste plastics, abrasion of tires, urban dust, and synthetic cloths are known to be the secondary sources.18,19 Other sources of MPs/NPs are the plastics used in households, industry, fishing, and agriculture.19,20 Both synthetic and biopolymers are responsible for terrestrial and aquatic plastic pollution. Fig. 1 shows an overview of the sources of MPs, their impacts and migration pathways.21 The size of 95% of microbeads used in personal care products was less than 300 μm, and the concentration of microbeads in personal care products was noted to be 1.9–71.9 mg g−1 of products.22 Particles equal to or smaller than 1 mm are the most abundant in aquatic, marine, and terrestrial environments. These tiny particles are recognized as hazardous elements, and their impact on human health is understudied and not well understood.23–25 The presence of degraded plastics in all ecosystems affects the soil, water, and atmospheric environment, thus creating adverse impacts on aquatic and terrestrial biota. At the same time, food and feed chains are gradually becoming contaminated with plastic particles (MPs, NPs, etc.). The growing plastic pollution problem could have a long-lasting impact on ecosystems and the health of living beings.
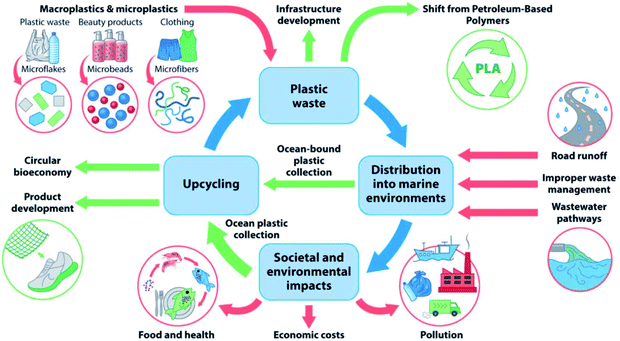 |
| Fig. 1 An overview of the sources of microplastics, their implications and migration pathways [adapted from ref. 21, Copyright the Royal Society of Chemistry]. | |
MPs in terrestrial and aquatic ecosystems and food systems have been extensively studied;17,26–29 however, there are no studies that compiled the information on plastics/MPs/NPs in terrestrial ecosystems and food systems highlighting either recent technological advances or environmental, economic, and societal issues of plastic pollution, especially MPs from biodegradable plastics. Several reviews have been conducted on plastic/MP/NP pollution in aquatic ecosystems,28–33 terrestrial ecosystems34–36 and food systems,37 where the authors have discussed either physiochemical properties, behavior, toxicity or remediation of MPs/NPs. This study compiles information on plastic pollution in terrestrial and aquatic ecosystems, and food systems and discusses some recent initiatives to combat the evolving problems associated with plastic pollution, especially MPs in the atmosphere, water, soil, and food chains from both conventional and biodegradable plastics, and their implications in ecosystems.
Waste plastic management systems
A large amount of plastic leaks into ecosystems due to improper waste management and slowly degrades and affects ecosystems and the environment.38–41 It was estimated that only 9% of the global virgin plastics are recycled, 12% incinerated, and the rest are purposely (landfilled) or unintentionally dumped into the environment.42–44 However, in Europe, waste plastic recycling, incineration, and landfilling shared 30, 39, and 31%, respectively.45 In Canada, 86, 9, 4, and 1% of plastic waste was landfilled, recycled, converted into energy, and abandoned in the environment in 2016, respectively.46 In Europe, the recycling of polyethylene films has enhanced by 30% due to circular economy initiatives and attracted more investment in this sector.18 In addition, pyrolysis/co-pyrolysis (a thermal treatment is given to a feedstock or multiple feedstocks under an oxygen-deprived condition) is also becoming a potential pathway for plastic waste management because of its environmental and economic advantages47–50 and can be an alternative to incineration and landfilling.51
The combined management strategies may help overcome the persisting problems in plastic waste management, such as improved collection and processing, restricted and controlled access to plastic, or environmentally friendly alternative plastics. The alternative material may ease the complexity of waste segregation and collection, and the downstream waste management problems and, thus, may help reduce plastic pollution in ecosystems.
Degradation of waste plastics
Leaked or mismanaged plastics degrade into smaller fragments/particles in ecosystems over time. Plastic particles between 5 mm and 1 μm are defined as microplastics (MPs);12,21 further degradation of MPs generates finer particles which are known as nanoplastics (NPs) (Fig. 2). MPs are found in water, soil, and air,18 which are hazardous to ecosystems as these particles are ingested by soil or marine biota, causing various health problems for them and contaminating food systems. Based on the particle size, MPs are defined as small- (<1 mm), medium- (1–3 mm), and large (3–5 mm);52 however, particle sizes of 1–1000 μm and <1 μm are defined as NPs and picoplastics, respectively.34 The fragmented plastics are also categorized as mesoplastic (5–25 mm) and macroplastic >25 mm.53
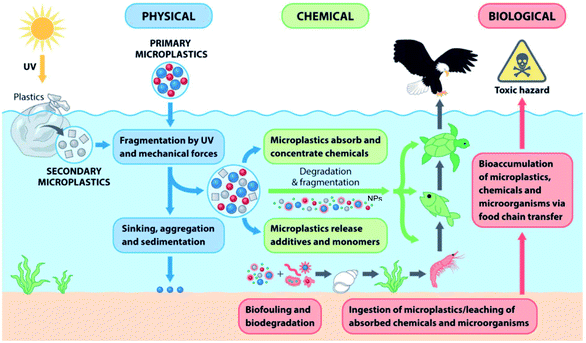 |
| Fig. 2 Schematic diagram of the degradation process of waste plastics [adapted from ref. 21, Copyright the Royal Society of Chemistry]. | |
The degradation processes of leaked plastics are physical-, photo-, chemical-, and biodegradation.54 Microorganisms such as fungi (e.g., Aspergillus sp. and Penicillium sp.), bacteria (e.g., Azotobacter sp. and Pseudomonas sp.), and actinomycetes (e.g., Amycolatopsis sp. and Actinomadura sp.) can degrade both synthetic and natural plastics.55 The degradation of leaked plastic depends on its surrounding conditions (e.g., in the terrestrial or marine ecosystem), types of plastics (synthetic or natural plastics), and their characteristics (e.g., hydrophobicity, molecular weight, crystallinity, hardness, forms of plastics, etc.).55 Hydrophilic degradation is faster compared to hydrophobic degradation.55 For example, the specific surface degradation rate of polylactic acid (PLA) on land is 20 times faster than that of high-density polyethylene (HDPE); however, a similar degradation was observed in the marine environment.56 The characteristics of MPs can keep changing due to fragmentation/degradation during their residence time. The mobility of MPs is influenced by human activities, morphology, and hydrology,57 thus altering their bioavailability and biological fate.58 MPs act as a sink and vectors of toxic inorganic and organic compounds and become more hazardous to biota when those compounds are released into their surroundings.59 The fragmented or degraded plastic particles float on the water surface, settle on marine snow, submerge in different depths of water columns or settle on the seabed depending on particle sizes and absorption of chemicals or contaminants, making them accessible to all the aquatic biota and finally affecting food systems and human health.37,60
The degradation of waste plastics in ecosystems is mainly driven by ultraviolet (UV)-radiation induced photooxidation, which releases monomers and oligomers and forms smaller fragments.58,61 The smaller polymer fragments are more susceptible to biodegradation. First, the plastic polymers degrade into their monomers, and then the monomers are finally mineralized.62,63 Although MPs accumulate from various sources, the oceans become the final sink for all sorts of plastic particles because MPs from upstream (either terrestrial or freshwater plastics) end up in the oceans. In addition, most of the commercial plastics (such as PE: polyethylene, PP: polypropylene, PS: polystyrene, PET: polyethylene terephthalate, PVC: polyvinyl chloride, polycarbonate, etc.)61,64–66 contain additives such as bisphenol A (BPA), phthalates, polybrominated diphenyl ethers (PBDE), etc.,61,67 which are usually not covalently bonded with the polymer and are released during the waste plastic degradation process;61,68 thus, creating a dynamic mixture of polymers and additives binding organic materials and contaminants to develop an ‘ecocorona’, a complex generated between MPs and organic materials present in the environment,69 which changes their toxicity and bioavailability.58 The ecocorona then modulates the absorption of bacteria and can form a thin layer on the surface of plastic particles, which is known as biofilm.58 The settling of MPs and NPs depends on biofouling and the type of polymers.70,71
In addition, MPs exhibit a Trojan horse effect, i.e., absorb contaminants, chemicals, and heavy metals;72,73 thus, increasing their toxicological effects and becoming more harmful to biota.73 The pollutant absorption capacity of aged MPs is higher than that of virgin ones.74,75Fig. 3 represents the adsorption mechanism between MPs and chemicals. The contaminant transportation by MPs in the marine environment depends on salinity, dissolved organic matter and temperature.29 Ciprofloxacin (an organic compound) sorption capacity of MPs decreases with salinity and the cation competition reduces adsorption efficiency by 70%.29,75,76 The sorption behaviour of MPs also depends on the particle size, age, hydrophobicity, hydrogen bonding and specific surface ratio.29 For example, the sorption of polychlorinated biphenyls (PCBs) on PVC decreased with increased chlorinated congeners because of higher cohesive density.77
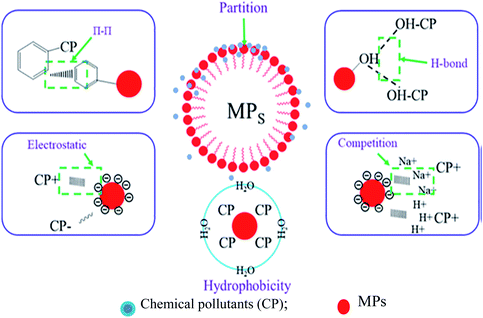 |
| Fig. 3 Adsorption mechanisms between microplastics and chemicals [adapted with permission from ref. 29, Copyright Elsevier, 2021]. | |
Microplastic assessment methods and tools
Several methods are being used for extracting/separating MPs from soil, sludge, sediment, and water (ESI: Table SI-1†). However, universally accepted suitable methods of MP and NP identification and quantification are lacking.18,19,78 Commonly used methods are sieving, filtering, heating (130 °C for 3–5 s), and density suspension for soil samples. Simultaneously, acid-, alkali-, and enzyme digestion and chemical oxidation are used to remove impurities such as organic matter.34 Thermal extraction and desorption gas chromatography (TED-GC) is an integrated approach for environmental samples to characterize multicomponents in complex samples such as particles, chemicals, etc.79 The weighing method (mass of MPs was measured) is used for samples that contain fewer impurities and a high mass of MPs in water or sediment.80
Commonly used methods are visual screening, scanning electron microscopy (SEM), Raman spectroscopy, and FTIR.34 Thermal extraction desorption gas chromatography-mass spectrometry (TED-GC-MS) and pyrolysis-gas chromatography coupled with mass spectrometry (PY-GC-MS) have also been used for identifying and quantifying MPs.81,82 A study on beach and coastal sediments noted that Raman spectroscopy is a better assessment tool for identifying smaller particles, especially with a particle size ≤20 μm compared to FTIR.83 However, the study also recommended FTIR and Raman spectroscopy for identifying MPs of 50–500 μm and 1–50 μm, respectively.84
Microplastics in ecosystems
Aquatic ecosystem
Globally, about 10–20 million tonnes of plastics end up in the oceans every year because of irresponsible public behavior or inadequate waste management systems.85,86 Annually, about 1.2–2.4 million tonnes of plastic enter the oceans via rivers, with Asia contributing to 67% of this plastic waste.87 The presence of microplastics (MPs) in the marine ecosystem was first investigated in 1971.88 Nowadays, the presence of MPs all over the world has been identified, such as in almost all aquatic ecosystems,34,83,89,90 agroecosystems,27 and food and beverage systems.91 MPs are harmful to both marine and human life;25,92 however, their toxicity is not well known.78 The accumulation of MPs was also observed in regions that are far from population centres, such as polar-/Arctic Sea ice90 and in remote mountains,93 which indicates atmospheric deposition of MPs. It has also been reported that global warming may result in melting polar-/Arctic Sea ice and release accumulated MPs in sea ice.90 Consequently, it is essential to understand the leakage of MPs and NPs from the use phase of plastic products.
The wastewater treatment plant is another source of plastic pollution in the aquatic systems.94–96 About 50% of global wastewater streams remain untreated, which adds 3.85 × 1016 MPs into the aquatic systems; however, 90% of this pollution can be abated if wastewater is treated before being released into the aquatic environment.97 Regular laundry processes of synthetic clothes [made of polyester, polyester–elastane and polyamide–elastane (these are known as stretch fabrics, which are different than the rayon and cotton fabrics, usually used in sports clothes)] released 175–560 microfibers per g-garments in 5–10 consecutive washing cycles; where the type of fabric did not influence the release of microfibers.94 However, the release of microfibers can be reduced by a homogeneous coating of biodegradable polymers (PLA: polylactic acid, PBSA: polybutylene succinate-co-butylene adipate) on the surface of polyamide fabrics.98 For example, effluent from a textile wet processing mill contained 361.6 ± 24.5 microfibres per L, and most of them (92%) were shorter than 1000 μm.95 Although wastewater treatment plants (WWTPs) can remove larger MP particles, they are noted to be inefficient for removing smaller particles (<100 μm) which remain in the effluent released into aquatic ecosystems. Consequently, a WWTP annually may release more than 100 billion MP particles.30
In the global marine environment, 15–51 trillion MP particles are floating with varying densities.97,99 For example, MP density in marginal seas and densely populated coastlines is higher than in the deep oceans.100 In addition, some of the plastic particles remain in different depths of water depending on their size and density and some are exported to the seabed.58 MP accumulation and settling on the seafloor are also dependent on the thermohaline-driven current in the sea.101 The concentration of MPs in the freshwater system also varies depending on the geographical location. For example, the density of MPs in river surface water of the Tibet Plateau and Yangtze Estuary was 483–967 particles per m3 and 4137 particles per m3, respectively, because of the difference in population density.102 In New Zealand, the urban water streams are noted to be one of the major sources of MPs in freshwater systems.103 In Canada, yearly MP discharge via wastewater influent and effluent was 28
550 billion and 6939 billion, respectively.97 The effluent from WWTPs contains 0.2–1.8% (0.7 particles per L), which is usually discharged on farmland in Australia.104
The concentration of MPs in marine sediment varied from 42–6595 particles per kg depending on the depth and position of sampling.99 On the other hand, the concentration of MPs in the river-bank sediment was 161–432 MPs per kg, where fibres contributed more than 88%.105 In India, the concentration of MPs in high tide line and low tide line beach sediments along the southeast coast of India was noted to be 1323 ± 1228 mg m−2 and 178 ± 261 mg m−2, respectively.106 MPs in the aquatic environment (1–230 μm; 0.1–10 mg L−1) affected the sea urchin and its offspring.107
In the aquatic environment, waste plastics release chemicals/additives and other components (such as PAHs: polycyclic aromatic hydrocarbons; PCBs: polychlorinated biphenyls; EDCs: endocrine-disrupting chemicals; PBDEs: polybrominated diphenylethers; DOM: dissolved organic matter; DOC: dissolved organic carbon; POM: particle organic matter) during degradation (Fig. 4), which then affect the marine biota and transfer into food systems through ingestion, egestion, reingestion, adsorption, etc.29 Polybutyrate adipate-co-terephthalate (PBAT) also exhibited greater sorption and desorption capacities of phenanthrene (an organic pollutant) compared to polyethylene (PE) and polystyrene (PS) as well as carbonaceous geosorbents.108 The authors also noted that the sorption and desorption rates of MPs are correlated with the rubbery subfraction and the surrounding environment. Although enormous emphasis has been placed on the MP contamination in the marine ecosystem, we still lack adequate information on the oceans. Also, due to the abundance of MPs in the terrestrial ecosystem,109,110 attention also needs to be paid to the terrestrial ecosystems. Studies on fresh water and terrestrial ecosystems will also enhance the scope of identifying the sources of MPs in ecosystems because they are the primary receivers of agricultural and urban waste.111
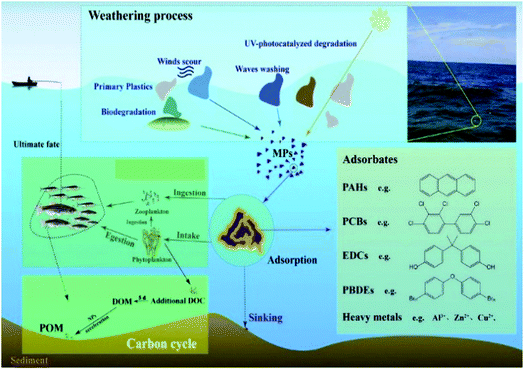 |
| Fig. 4 The role of microplastics in an aquatic environment [adapted with permission from ref. 29, Copyright Elsevier, 2021]. | |
Terrestrial ecosystem
Plastic mulch used on farmland is identified as one of the main sources of microplastic (MP) contamination, along with compost, sewage sludge, biosolids, irrigation water, atmospheric deposition, road dust, etc.52,112–114 Atmospheric deposition of MPs on the ground in urban and remote locations took place during both the- dry and wet periods.115 Biosolid (solid organic matter, recovered from wastewater/sewage and commonly used as fertilizer on farmland) is applied on the surface of farmland or injected depending on the soil saturation. The concentration of MPs in biosolid was 8678–14
407 MPs per kg.116,117 However, a higher range of MPs in biosolid (10
926–64
986 MPs per kg) has also been reported.118 Application of contaminated biosolid (1–15 t ha−1) on farmland resulted in topsoil contamination of 4–150 pieces per kg per year.117 The concentration of MPs in farm soil also depended on the intensity of sewage sludge application.112,114,116 For example, the concentration of MPs increased by 710 pieces per kg for each successive application of sewage sludge (20–22 t ha−1).114 MP contamination was higher in topsoil compared to deep-soil.52 In Xinjiang, plastic contamination was 259–381 kg ha−1 in cotton fields, which are reported to be the most severe source of plastic pollution on farmland in China.119 In Switzerland, more than 90% of floodplain soils (soil deposited on flood-prone land next to a river or stream) are contaminated by MPs. The concentration of MPs in soils depended on the population density of the area, which indicates that plastic waste was the source of MPs.120 The biosolid from waste water treatment plants (WWTPs) contains 8–16% (41.4 particles per g) MPs, which are usually discharged on farmland in Australia.104 Farmlands in the United States of America, the European Union, China, Canada, and Australia annually added about 21
249, 26
042, 13
660, 1,518, and 1241 tonnes of MPs, respectively, through the application of biosolid.118 A widespread application of sewage sludge or biosolid from WWTPs would be a major source of MPs in farm soil and affect food production.
The migration of MPs from soil to the aquatic system (marine, fresh, and groundwater systems) can take place through surface runoff, bioturbation, tillage, water infiltration, wind erosion, animal grazing, etc.15,34,52,113,121 It has also been reported that 99% of MPs in biosolid applied on farmland migrated to the aquatic environment.116 Consequently, terrestrial MPs also contaminate surface and underground water systems as well as marine ecosystems. However, the migration of MPs is dependent on their size, shape and surface characteristics.34
Fragmented plastics and MPs were identified in agricultural soil where plastic mulch was used.20 The concentration of MPs in cropped soil is reported to be 571 pieces per kg and 263 pieces per kg in mulched soil and non-mulched soil, respectively.27 The concentration of MPs in the soil is also dependent on the intensity of mulching. For example, the concentration of MPs was 80.3 ± 49.3, 308 ± 138.1, and 1075.6 ± 346.8 pieces per kg for 5, 15, and 24 years of continuous mulching, which indicates that MPs in soil originated from the plastic mulch.122 The fate of MPs in soil depends on the physicochemical properties of soil and biota in soil123 as well as the type of plastic. For example, the population of bacteria was higher than fungi adjacent to weathered biodegradable mulch compared to the unweathered one. Weathering enhances the degradation process.11 MPs in soil absorb toxic chemicals and heavy metals as well as antibiotics and become more harmful to soil biota.36
Polyethylene (PE), polyvinyl chloride (PVC), and polyethylene terephthalate (PET) are the most commonly found polymers in the terrestrial ecosystem.34 The presence of additives in plastics increases the ecological toxicity of plastics. For example, phthalic acid esters (PAEs) used in agricultural plastics (agricultural films/mulch) are a source of MP contamination in fruits and vegetables grown on the PAE contaminated soil,124 thus accumulate in food systems. The concentration of PAEs in agricultural soil was 1.8–3.5 mg kg−1, where plastic mulch was used.125 However, MPs were also found on farmland where neither agricultural plastics nor MP containing fertilizers were applied126 because of atmospheric deposition.
MPs affect the biophysical properties (damage soil structure, reduce aeration and water permeability and water holding capacity) of soil17,127,128 because of the decreasing absorption capacity of freely available chemicals in soil-water due to the hydrophobicity of MPs.129Fig. 5 shows an overview of the atmospheric deposition of MPs in the terrestrial ecosystem and their effects on soil properties and feedbacks to the atmosphere.130 MPs negatively affect the soils organic carbon, nutrient transfer, nitrogen cycling, microbial activity, and biodiversity;15,130–133 thus, leading to reduced plant growth and productivity.59,127,128 For example, agricultural yields diminish substantially when plastic waste accumulation reaches 72–260 kg ha−1.134,135 The presence of MPs in soil alters soil stability and affects germination, shoot growth, and productivity.
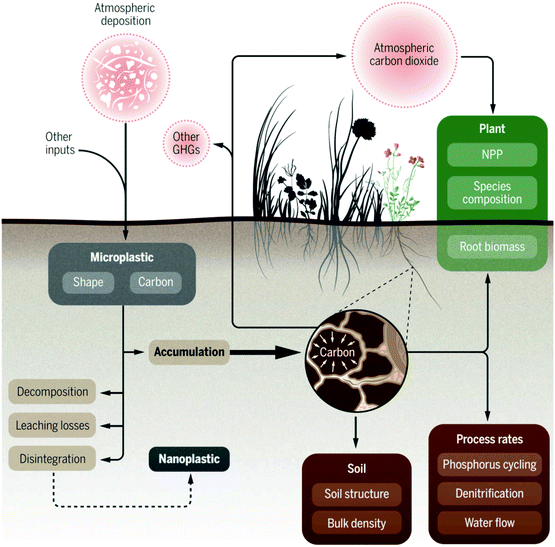 |
| Fig. 5 Atmospheric deposition of MPs in the terrestrial ecosystem, their effect on soil properties and feedbacks to the atmosphere [adapted with permission from ref. 125, Copyright 2020, American Association for the Advancement of Science]. | |
Biodegradable plastics are recognized as an alternative to plastics from fossil sources. Although biodegradable plastic completely degrades in industrial composting facilities,11 it also generates MPs if leaked into the environment.136,137 A model study also confirmed that biodegradable mulch made of polybutyrate adipate-co-terephthalate (PBAT) and non-biodegradable mulch made of low-density polyethylene (LDPE) generate MPs and NPs if weathered.138 For example, biodegradable plastics such as polyhydroxybutyrate (PHB) in a representative abiotic environment generated MPs and NPs, whose impacts on the environment are poorly understood.11,139 Degradation of biodegradable plastics also depends on the types of plastics and the degradation environment.140–142 In soil, the degradation of weathered polylactic acid (PLA)/polyhydroxyalkanoate (PHA)-based biodegradable plastic mulch was greater than that of the unweathered PBAT-based biodegradable plastics because microbes preferred PHA over polylactic acid (PLA), and starch over PBAT.11 The degradation rate of PLA buried in soil at 37 °C is also reported to be much slower than that in the microorganism-rich composting facility.136 After 12 months, the molecular weight loss of PLA in compost was 20%; however, in soil there was no significant weight loss.136
The degradation of polycaprolactone (PCL) was faster compared with PHB, PLA and poly(1,4-butylene) succinate (PBS), and abundant fungal strains were associated with PCL at 50 °C.140 Poly(p-dioxanone) exhibited greater degradation (441 ± 326 and 2103 ± 131 item per g plastic in air and soil, respectively) compared to bioplastic blends and non-biodegradable plastics. However, poly(p-dioxanone) generated numerous MPs after degradation.143 The soil microbiome is also influenced by plastic pollution in terrestrial ecosystems.144
Plant growth depends on the type of MPs and the concentration of MPs in soil because of the alteration of soil stability.17,145–147 For example, the presence of MPs from PLA lowered germination and shoot growth of ryegrass more than MPs from high-density polyethylene (HDPE).17 In another experimental study, different MP particles were mixed with soil and then seeds were sown to study the germination rates and shoot growth of Lepidium sativum, a fast-growing herbaceous plant. The study revealed that MPs produced oxidative burst in plants and among the tested MPs (PP: polypropylene, PE: polyethylene, PVC: polyvinyl chloride, and a mixture of PE & PVC), PVC had more effect on plant biometric parameters (germination, leaf number, plant height and biomass productions) than other MPs.145 A strong correlation was observed between the metal content in MPs and the number of MP particles, indicating that the abundance of MPs influences the heavy metal content. In contrast, interaction between cadmium and MPs from PE has been reported to affect root symbiosis; however, no interaction between MPs from PLA and cadmium was noted, but PLA produced stronger phytotoxicity.148 Consequently, it seems that the coexistence of heavy metals and MPs in soil jointly affects root symbiosis and plant performance; thus, becoming an alarming threat to soil biodiversity and agroecosystems.148,149
A comparative experimental study between MPs from LDPE and biodegradable plastic (starch-based plastic) mulch revealed that MPs from biodegradable plastic mulch have a stronger negative impact on wheat growth than PE mulch, which might be because biodegradable plastic mulch contains 18.3% polybutylene terephthalate and 44.6% PET, which stops starch-induced nitrogen movement.16 Another study on the adsorption and desorption kinetics of PE and PHB MPs also confirmed that triclosan (a common disinfectant used in plastics) equilibrium adsorption and desorption rates of PE is greater than that of PHB (3431.85 and 9442.27 μg g−1, respectively), and PHB easily releases it compared with PE.150
On the other hand, an experimental study revealed that the presence of MPs in soil enhanced the shoot and root mass of an invasive species with drought because MPs in soil helped reduce soil bulk density facilitating better aeration, water holding capacity, and root penetration; thus, they could rapidly reach limited water resources which enhanced the productivity of invasive species.151 The growth of spring onion depended on the type of MPs. For example, total biomass growth of spring onion was better in the presence of primary polyamide (PA) and polyester compared with polystyrene (PS) and PET because of the difference in their composition, i.e., PA contains nitrogen which may have enhanced biomass growth; however, the authors warn that a positive effect of MPs on plants cannot be ascertained.152 Consequently, it is essential to differentiate between the potential impacts of MPs and macroplastics in soil.34 The increasing MP contamination in terrestrial ecosystems may reduce global food production; thus, food security, one of the major world challenges, has to be addressed in the near future.
Biodegradation (degradation by using microorganisms/enzymes) of pretreated (grinding/irradiation) plastic is noted to be a potential route to reduce the problems associated with waste plastics.153,154 It is also noted that earthworms can enhance the degradation of biodegradable plastics in soil.155 Consequently, innovative pre-treatment coupled with technological advances could enhance the degradability of waste plastics. Although the biodegradation process can reduce the problems associated with waste plastic, it may need in-depth studies to confirm whether biodegradation processes eliminate MPs or NPs from the ecosystems.
Microplastics in food systems
Plastics leak into the ecosystems fragment into microplastics (MPs) or nanoplastics (NPs), which are then ingested by terrestrial and aquatic biota and contaminate our food systems.156 For example, plant-root systems, especially vegetables, absorb fine plastic particles (0.2 μm), which then migrate to the shoots; thus, they are very likely to enter into food systems.157,158 MPs were found in crops (such as lettuce) grown in hydroponic systems and sand metrics irrigated with wastewater containing plastic components, thus transferring into food chains.159 Phthalate esters (PAEs) have also been observed in wheat grains grown on contaminated soil.125 Through edible plants (fruits and vegetables), the estimated MP intake was 80 g per person per day.160 In Portugal, MP was detected in 49% of the fish that were analyzed, and 0.054 ± 0.099 MP pieces per g of dorsal muscle were observed.161 On the other hand, only 3 MPs were observed in the gastrointestinal tracts of hamour fish from Kuwait Bay and southern areas.162 The estimated intake by adults was 842 MP particles per year only from fish consumption in Portugal; however, in Europe and America, it varied from 518–3078 MP items per capita per year.161 However, the effect of MPs present in fish is reported to be negligible on human health.163 Another study also confirmed that no health risk was associated with fish consumption when the PAE concentration in fish was 0.15–0.26 μg g−1.164
The increasing application of plastic in the food industry, especially in food and beverage packaging, results in food contamination with MPs/NPs. For example, MPs were identified in bottled water and were argued to be released from packaging and coating as well as from the lubricant used on the caps.91,165,166 On average, 325 MP particles per litre of bottled water were identified where 95% of these particles were 6.5–100 μm and 10.4 particles were found to be greater than 100 μm where the most common morphology was fragments followed by fibres.91 The authors confirmed that the packaging/bottling process is the main source of MPs in bottled water. MP contamination has also been identified in groundwater,167 freshwater, and drinking water (groundwater/supply water).168,169
Melanophores and pigmentation were found in the skin of tadpoles (larval stage of an amphibian) exposed to polyethylene (PE) MPs (60 mg L−1 for 7 days) and external morphological changes were observed.78 The animal model trial confirmed the presence of MPs in aquatic animals and their effect on productivity.78,161 MPs were also detected in earthworms and chickens raised on contaminated garden soil (74.4 ± 20.4 PE bottles per m2); however, in the case of chickens, MPs were found only in gizzards and feces.170 Exposure to microfibers for 28 days injured the gastrointestinal walls of snails and reduced their food intake.171 In addition, a toxicological study revealed that mice could ingest MPs and accumulate them in tissues, which may affect terrestrial food systems and human health.172
MPs were also detected in marine foods, such as shellfish, salt, etc. and affect animals, e.g., birds,162,173–175 and human health.92 For example, MPs (>149 μm) were detected in commercial salts from different countries176,177 and in edible bivalves such as oysters, mussels, and clams.178 The concentration of MPs in blue mussels was reported to be greater than that in wild mussels because of the difference in MP concentration in their surrounding environment.179,180 The presence of MPs in honey and sugar has also been reported.19,181 On average, 9 ± 9 fibres per kg to 166 ± 147 fibres per kg of honey were found in different countries.19,182 Thus, it seems that all segments of human interaction are affected by MP pollution.
Although biodegradable plastic is recognized as an ecological alternative to synthetic plastic, NPs i.e., polyhydroxybutyrate (PHB) released into freshwater ecosystems as a result of the degradation of biodegradable plastics in the environment were harmful and reduced cellular growth and altered physiological parameters of organisms.139 In contrast, no significant oxidative stress of polylactic acid (PLA) MPs (sizes: 0.8–10 μm) was observed in blue mussels during the 8 days of trial while MP concentrations were controlled at 10 and 100 μg L−1.183 However, the World Health Organization (WHO) noted that most plants and soil organisms are unlikely to uptake MPs >150 μm, except mesofauna, and the human body does not absorb these MPs;169 thus, they do not pose a risk to human health. These MPs may pose a health risk if contaminated with toxic chemicals or additives. It seems that crops, livestock and beverages are contaminated by MPs, whether raised on farmland, greenhouse, or home-garden; thus, waste management could be vital in reducing MP contamination in food chains and abating environmental and health risks as well as food insecurity.
Initiatives to mitigate plastic/microplastic pollution
In an attempt to combat increasing plastic pollution, various initiatives (improved waste management, wastewater treatment, innovative design and development, etc.), and regulations57 for single-use plastics and industrial use of microbeads (such as bans or phasedowns or restricted use of single-use plastics; banning the use of microbeads in personal care products, etc.), were enacted to control plastic pollution.57,184 Countries and regions have banned the industrial use of plastic microbeads and agreed to phase them out. For example, the United States of America (USA), Canada, and the United Kingdom (UK) have banned the industrial use of plastic microbeads in 2015, 2017, and 2018, respectively.57,185 In the cases of the USA and the UK, the ban was imposed on the use of microbeads in rinse-off personal care products.57 At the same time, the circular economy initiative is promoting a zero-waste approach. A global campaign has been initiated by the United Nations Environment Programme to eliminate primary sources of plastic litter by 2022.156 The European Union has enacted several regulations such as ‘Water Framework Directive’ (a directive formulated to expand the scope of water protection to all waters, achieving good status by a set deadline based on river basins’ combined approach of emission limits and streamlining the legislation) and the Common Fisheries Policy to abate chemical and nutrient pollution in aquatic systems. On the other hand, Integrated Coastal Zone Management and the Marine Strategy Framework Directive were implemented to control MP pollution.156 Life cycle economy and life cycle assessment were introduced for the design, production, use, and recycling of plastic products.156 Sol–gel induced agglomeration has also been introduced to remove MPs from the aquatic system.186 Air purifiers have been introduced to remove MPs from air (>0.1 mm) in order to improve indoor air quality.187 On the other hand, fungi were used as a potential remedy to degrade MPs in soil.188 In addition, clean-up drives are ongoing to minimize terrestrial and aquatic plastic pollution.
Impacts of waste plastics/microplastics
Environmental impacts
Assessing the environmental impacts of microplastics (MPs) is difficult because of changes in ecosystem functions, hazardous impacts on biota, and varying toxicities induced by their composition.54,57 It also argued that the ecological effect of MPs is yet to be well understood and related data is scarce.189 However, a recent study revealed that under composting environments, unweathered PBAT-enriched mulch released higher amounts of CO2 than weathered biodegradable mulch because environmental weathering enhanced the degradation of biodegradable mulch.11 The authors also confirmed that the microbial degradation of mulches is influenced by their polymeric constituents. For example, microbial degradation of biodegradable mulch is more persistent in bacterial communities than in fungal communities.11
The Canadian landfill sector generated about 13 million tonnes of GHG from landfill waste (CO2 eq) in 2018.190 Annually, the agricultural plastic recycling program can mitigate 20
000 tonnes-CO2 eq in Ontario.191 The environmental impact of plastic used in consumer goods was 6.7 t CO2 eq per tonne of plastic used.1 The environmental impacts of plastic production and disposal also depend on the type of plastic and disposal methods (Tables 1 and 2). For example, a comparative life cycle study on mixed plastic waste was conducted under three perspectives: product, disposal, and combination of both (entire life cycle). In the case of disposal (chemical recycling via pyrolysis and mechanical recycling with energy recovery and compared with virgin plastic), chemical recycling had a 50% lower climate change impact compared with mechanical recycling. Although the climate change impact was similar when the quality of the recyclate was considered, other impact categories (acidification, eutrophication, human toxicity, etc.) were higher in the case of chemical recycling.192 However, chemical recycling released a lesser amount of greenhouse gas (GHG) (2.3 t CO2 eq per tonne less) than virgin plastics which can be environmentally beneficial because the climate change impact of the recyclate from chemical recycling was −0.45 t CO2 eq per tonne while it was 1.89 t CO2 eq per tonne of virgin plastic.192 In another study, Khoo compared various waste disposal scenarios (combinations of mechanical recycling, incineration, pyrolysis, etc.) of mixed plastic and concluded that a combination of mechanical recycling (10%), incineration (83%) and pyrolysis (7%) was environmentally better among the considered scenarios, while the worst combination was mechanical recycling (11%), incineration (71%), and gasification (18%).193 The author also noted that for individual waste disposal of mixed plastics, pyrolysis performed better than incineration but poorer than mechanical recycling or gasification.193 It seems that the mixed plastic waste disposal process plays an important role in the life cycle of plastic. Although the persistent presence of MPs in the ecosystems poses a potential risk to all biota on the earth through soil, water, and food systems, studies on the environmental impacts of MP pollution are scarce. Therefore, scientific studies on the environmental impacts are essential to determine the environmental severity of MP pollution and abate its potential risks.
Table 1 Impacts associated with the primary production of selected plastics/tonne [adapted from ref. 194]a
Types of plastic |
Impact category |
Process energy, MJ |
GWP, kg CO2 eq |
ADP, kg Sb eq |
AP, kg SO2 eq |
EP, kg PO4+ eq |
HTP, kg 1,4-DB eq |
GWP: global warming potential; ADP: abiotic depletion potential (abiotic depletion refers to the depletion of nonliving resources such as fossil fuels, minerals, clay, and peat); AP: acidification potential; EP: eutrophication potential; HTP: human toxicity potential.
|
Polyethylene terephthalate (PET) |
43 336 |
2468 |
33 |
12 |
3 |
735 |
High density polyethylene (HDPE) |
26 399 |
1891 |
33 |
21 |
1 |
67 |
Polypropylene (PP) |
24 396 |
1999 |
33 |
20 |
1 |
50 |
Polystyrene (PS) |
43 587 |
2776 |
38 |
17 |
2 |
55 |
Polyvinyl chloride (PVC) |
38 841 |
1336 |
18 |
10 |
1 |
151 |
Table 2 Environmental impacts of different disposal scenarios of plastic waste/tonne [adapted with permission from ref. 195, Copyright Elsevier, 2021]a
Feedstock |
Scenario |
Impact category/tonne |
Energy, MJ |
GWP, kg CO2 eq |
ODP, kg CFC-11 eq |
ADP, kg Sb eq |
AP, kg SO2 eq |
EP, kg PO4+ eq |
HTP, kg 1,4-DB eq |
TE, kg 1,4-DB eq |
ME, kg 1,4-DB eq |
PO, kg C2H4 eq |
PET: polyethylene terephthalate; PE: polyethylene; A: landfilling without biogas recovery; B: incineration without energy recovery; C: recycling; D: incineration with energy recovery; GWP: global warming potential; ODP: ozone depletion potential; ADP: abiotic depletion potential; AP: acidification potential; EP: eutrophication potential; HTP: human toxicity potential; TE: terrestrial ecotoxicity; ME: marine ecotoxicity; PO: photochemical oxidation.
|
PET |
A |
5.65 × 103 |
2.89 × 103 |
8.34 × 10−6 |
2.58 × 10−4 |
4.33 × 101 |
3.58 |
1.41 × 102 |
1.68 |
8.56 × 105 |
2.39 |
B |
1.62 × 104 |
6.23 × 103 |
2.80 × 10−5 |
8.25 × 10−4 |
5.18 × 101 |
5.42 |
5.13 × 102 |
2.42 |
2.40 × 106 |
2.68 |
C |
7.02 × 103 |
8.73 × 102 |
3.60 × 10−6 |
1.85 × 10−3 |
8.19 × 100 |
1.16 |
1.78 × 102 |
4.73 |
5.05 × 105 |
3.97 × 10−1 |
D |
−1.08 × 104 |
4.94 × 103 |
2.80 × 10−5 |
8.25 × 10−4 |
3.73 × 101 |
4.88 |
4.79 × 102 |
5.71 |
2.33 × 106 |
2.10 |
PE |
A |
1.05 × 104 |
3.82 × 103 |
1.28 × 10−5 |
3.89 × 10−4 |
5.77 × 100 |
1.39 |
2.62 × 102 |
1.23 |
1.23 × 106 |
4.98 × 10−1 |
B |
2.11 × 104 |
6.76 × 103 |
3.30 × 10−5 |
9.65 × 10−4 |
1.30 × 101 |
3.15 |
6.74 × 102 |
1.14 |
2.78 × 106 |
9.45 |
C |
2.96 × 103 |
5.87 × 102 |
5.10 × 10−6 |
5.62 × 10−2 |
2.56 × 100 |
6.25 |
1.07 × 102 |
4.70 |
9.50 × 104 |
1.16 × 10−1 |
D |
−3.93 × 10−4 |
3.94 × 103 |
3.30 × 10−5 |
9.65 × 10−4 |
−1.88 × 101 |
1.95 |
6.01 × 102 |
5.62 |
2.63 × 106 |
8.19
|
Economic impacts
The global market for plastic recycling was $33.0 billion in 2020 and is expected to be $47.3 billion in 2026.196 Waste plastic causes severe economic damage to the world's marine ecosystems and the yearly damage is estimated to be $13.0 billion.44 The waste management cost also depends on the disposal processes. For example, the economic cost of household waste that is burned or dumped is reported to be $375 per tonne; however, in the case of integrated waste management, the cost fell to $50–$100 per tonne.5 In Canada, the tipping fee for plastic waste disposal to landfills varies from $80–$160 per tonne.197
Waste management sectors in Canada employed 29
543 persons, spent $5.9 billion and generated $7.1 billion in 2014;198 however, waste collection costs reached $5.9 billion in 2019.199 In Canada, plastic waste entails a lost opportunity of about $7.8 billion in 2016 and is expected to be $11.1 billion by 2030.9 The Canadian plastic market was about $35 billion in 2017.9 About 2434 industries were engaged in the processing of synthetic resins to produce plastic products, employing 77
400 people and generating a shipment value of $19.6 billion in 2012.200
A hypothetical study noted that the yearly environmental cost of consumer goods made of plastics on the earth was $113 billion, and transportation of plastic goods contributed $53 billion,1 where the environmentally extended input–output (EEI-O) model was used. It is worth mentioning that the results of an input–output model vary with the market price of materials. These environmental costs can be mitigated by adopting an innovative packaging design, fuel-efficient transport, more sustainable electricity, and improved waste management. Improved waste management can save 30% of environmental costs.1 The environmental cost of plastic in-land and water pollutants was $362 and $626 per tonne, respectively.1
Societal impacts
Plastic mulch releases phthalic acid esters (PAEs) into the soil, which are known to be toxic and carcinogenic;201–204 thus, prolonged exposure may severely affect human health124,202,205–207 and pose a threat to ecosystems. However, it is noteworthy to mention that all PAEs may not be carcinogenic to human health. For example, dermal exposure to diethyl phthalate had no carcinogenic effect in rats.208 Growing health and environmental concerns are also leading to increasing consumption of plant-originated food; thus, may increase microplastic (MP) consumption through fish and plant food, which may create a severe health problem in the near future. Hale et al. noted that MPs <20 μm penetrate cell membranes and exposures to MPs compromised the feeding, metabolic and reproduction processes of organisms.28 MPs ingested by many marine species such as fish and shellfish create physiological problems for them.25 Consumption of MP contaminated food and exposure to phthalates in toys resulted in congenital diseases, cancers, and affected neurological and reproductive systems.209,210 In addition, the Trojan horse effect of MPs makes MP contaminated food more hazardous to human health. Interactions between biota and MPs are prevalent in ecosystems. There is growing evidence that exposure to MPs can incite significant health effects.211 At subcellular levels, biochemical changes precede changes to cells and tissues, which affect physiological functions, fitness and ultimately ecosystems.58 Behavioural change at different subcellular levels can be used as an indicator of the effects of MPs on ecosystems and food systems, and thus the human health and social impacts.
MP ingestion by birds, such as chicks, disrupts nutrient absorption, growth, and reproductive systems and ultimately threatens their survival.212 Ingestion of plastic-derived chemicals accumulated in MPs results in various toxicological effects such as metabolic disorders, inhibition of reproduction and growth, inflammatory responses, and even death of aquatic and terrestrial biota.213–216 Wheat grains grown on MP contaminated farmland (containing PAEs 4.1–12.6 mg kg−1) posed a higher carcinogenic risk for adults as they exceeded the recommended intake of PAEs. The contaminated wheat intake also exhibited non-carcinogenic risk, and children were the most sensitive.125 In addition, it is an alarming signal that invasive species such as ryegrass dominate in the presence of MPs in soil under drought; thus, the risk of degrading biodiversity prevails if we fail to address MP pollution. Consequently, it is very important to find potential remedies to MP pollution in our ecosystems to work towards a sustainable society.
Microplastic remediation
Various methods, such as advanced oxidation processes,31,217–219 photocatalysis,220 microwave221 and bioremediation222–226 have been employed to degrade/eliminate microplastics (MPs) from soil and water (Fig. 6). In a photocatalysis process, plastic particles degrade and form cavities around the catalysts initiating oxidation, generating carbonyl and carboxyl groups which are eventually photooxidised into volatile organics, CO2 and H2O.31,227 The microwave-assisted catalytic (iron-based catalyst) process required only 30–90 s to convert ground plastic into hydrogen and predominantly carbon nanotubes.221 On the other hand, the photocatalysis process (Nb2O5) completely converted plastic waste into CO2 in a simulated natural environment and produced CH3COOH without applying sacrificial agents.220
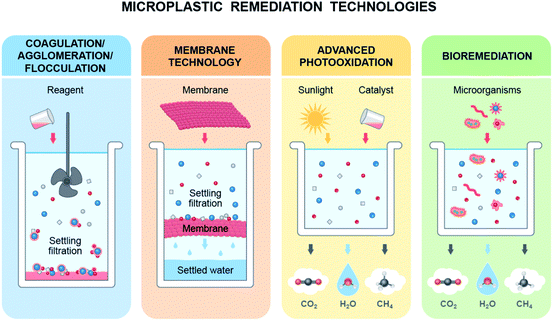 |
| Fig. 6 An overview of microplastic remediation technologies. | |
Kang et al. noted that carbocatalytic oxidation coupled with hydrothermal hydrolysis over carbon nanotubes generated highly reactive radicals and decomposed MPs into harmless organic compounds, which can be a carbon source for algae for complete mineralization of MPs from water.217 Membrane technologies have also been used as a remedy for MP/nanoplastic (NP) pollution in water; however, they need to be improved and employ advanced technology to remove particles smaller than 100 μm.228 Agglomeration and coagulation processes have also been used to form larger particles to facilitate the removal of MPs from water;229–231 however, the removal efficiency depends on the size of MPs.230 It seems that catalytic processes can convert plastics into nanocarbons or hydrocarbons, and other processes can facilitate the removal process, which would not be a suitable remedy for MPs/NPs that are already in our ecosystems due to their wide distribution and particle size.
Several studies have confirmed the ability of microorganisms to remove MPs/NPs (either synthetic or biodegradable) from soil or water.223–226,232 There are four steps in the bioremediation process of plastics: biodeterioration, biofragmentation, assimilation and mineralization. Microbes first enforce physicochemical deterioration, followed by fragmentation of polymers into oligomers and monomers using exoenzymes, integration of molecules into microbial metabolism, and finally, the ejection of oxidized metabolites.223 Exoenzymes (oxygenases) destabilize the long carbon-hydrogen chains of polymers and can add oxygen, forming alcohol, peroxyl, and carboxylic compounds. These compounds are then assimilated and mineralized by the microbial metabolic process.223
It is also noted that low-density polyethylene (LDPE), which can go through the gut of earthworms (bacterial consortium: Lumbricus terrestris), reduces the size of MPs within 4 weeks,224 which indicates that earthworms facilitate the degradation process of plastics. In another development, it was reported that microalgae can synthesize plastic polymers while using them as a carbon source.233 The authors also argued that biodegradable plastics can be produced by using microalgae which can replace synthetic plastics;233,234 the algal cell growth was greater than terrestrial plants.234
Biodegradable plastics are regarded as safer than synthetic plastics225 and more prone to microorganisms;143 thus, adopting biodegradable plastics and microbial degradation of plastic waste would be a potential remedy to MP/NP pollution. In addition, it is also argued that biodegradable microbeads (chito-beads) used in cosmetics exhibited greater cleansing efficiency than polyethylene (PE) microbeads and completely degraded in soil into CO2, H2O and biomass without any toxic effects on plants.235 Consequently, the development of biodegradable plastics and engineered microorganisms which can easily convert plastic particles either from conventional plastics or biodegradable plastics and mineralize them would be the key to MP/NP remediation; thus, they could be environmentally benign. Fig. 7 represents a potential route to eliminate MPs/NPs from the ecosystem and promote the biobased circular economy initiative without interrupting the benefits of plastics. Both terrestrial and aquatic plants absorb MPs/NPs from their surrounding environment. On the other hand, microorganisms convert MPs/NPs into CO2, H2O, and CH4. Some of these products are used by plants; thus, bioremediation coupled with non-edible plant cultivation in the terrestrial ecosystem and algae in the aquatic ecosystem, and producing biodegradable plastic from these biomasses would be a potential route to eliminate MP/NP pollution from the environment.
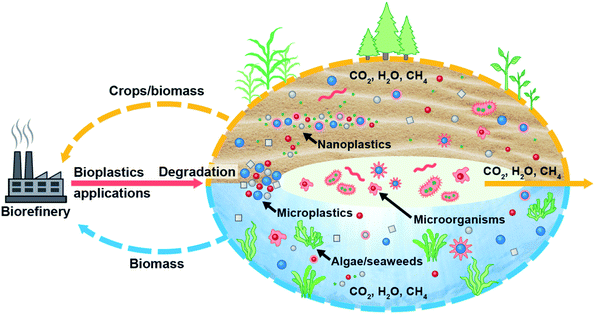 |
| Fig. 7 A potential route of microplastic remediation from ecosystems. | |
Discussion
Plastic particles keep changing and migrating from one ecosystem to another contaminating every sector of human interaction. Ultimately, microplastics (MPs) are affecting biota in various ecosystems and entering our food systems which affect animal and human health. Fig. 8 shows an overview of the sources of MPs, their transportation routes and their interaction among ecosystems. To mitigate the problems associated with waste plastics, numerous efforts are underway to replace conventional plastic with an alternative such as biodegradable plastic. Although biodegradable plastics degrade faster than synthetic plastics, the fate of biodegradable plastics seems to be understudied. Consequently, the fate of MPs from biodegradable plastics and their ecological risks need to be ascertained to control the plastic pollution in the ecosystems, especially in terrestrial ecosystems where biodegradable plastics are gaining more attention.
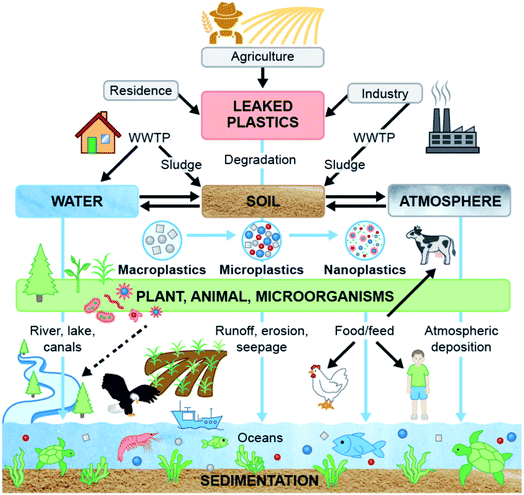 |
| Fig. 8 Schematic of the sources of waste plastics and their transportation routes. | |
A potential option for sustainable management of MPs in biosolid and to mitigate their effects on the terrestrial ecosystem would be its alternative use instead of farm application, such as the use of biosolid in the bio-brick manufacturing process.118 As plant root systems absorbed plastic particles from soil157 and then migrated to leaves,158 growing non-edible plants in contaminated soil and water could also be an option to reduce MPs from contaminated soil and water to abate adverse impacts of MPs on the ecosystem and health.
Despite the fact that MPs are found in every segment of ecosystems, their quantification and remediation methods are yet to be standardized. Consequently, it would be an important task to build consensus on the identification, quantification and remediation of MPs/nanoplastics (NPs) at a national and international level. In addition, impact indicators for MPs/NPs are yet to be developed and standardized for evaluating their environmental impacts. Although MPs/NPs are identified to be an emerging threat to the ecosystems, it seems that huge challenges, such as methods of analysis, need to be addressed before a comprehensive life cycle assessment (LCA) study on MPs/NPs can be conducted.
Although enormous efforts are underway to mitigate plastic pollution such as clean-up drives, design and development, directives/regulations, and awareness programs, which might be useful to reduce some of the plastic pollution through a removal process, it would be difficult to do so because of the wide range of distribution and size of MPs/NPs. Various other efforts are also underway to find a potential remedy to MP/NP pollution in our ecosystems. It seems that bioremediation would be a potential solution to eliminate the MPs/NPs from the environment. However, without joint action (citizen and government, national and international) in design, development, manufacture, use, disposal, best management practice, and stringent policies, plastic pollution will likely worsen. Initiatives can be extended in the following areas to mitigate/eliminate plastic pollution in ecosystems.
• Identify the hotspots in every sector (residential, industrial, agriculture/soil/terrestrial, and aquatic systems).
• Develop more effective and efficient remediation methods.
• Definitive policies/regulations.
• Ensure a system approach to avoid/mitigate plastic pollution from each source.
• Develop alternative products to conventional plastics (compostable plastic, which would not produce any toxic chemicals/MPs). Or even develop an alternative to plastic mulch, which can be used for a certain period without generating/releasing MPs during its use and then disposed of in a way that can be sustainable (controlled distribution & disposal) to abate plastic pollution in agricultural soil and, further, in the ecosystems.
Outlook
Nowadays, microplastic (MP) pollution is recognized as an emerging problem. Environmental, economic, and societal concerns over the effect of microplastic pollution on ecosystems have attracted enormous attention from all sectors (public, policymakers, environmental activists, and scientific communities) for research on alternatives and potential remediation pathways. MPs in terrestrial, aquatic, and food systems absorb hazardous contaminants, which affect soil quality and productivity as well as aquatic and terrestrial animals, plants and human health. Although enormous efforts are underway to replace synthetic plastic with biodegradable plastic to abate plastic pollution in ecosystems, further attention needs to be paid to avoid the adverse impact of MPs/nanoplastics (NPs) from biodegradable plastics in order to avoid any unwanted risk to the environment and human health. Adverse impacts of MPs/NPs were reported for both terrestrial and aquatic ecosystems; however, terrestrial ecosystems seem to be less explored. Consequently, comprehensive studies on terrestrial ecosystems and food systems are important for framing mitigating efforts or even eradicating the problems associated with MPs/NPs. We have compiled information on microplastic pollution in ecosystems and food chains, emphasizing the terrestrial ecosystem, recent technological advances, economic and societal implications, and the remediation of microplastic pollution. From this compilation, a potential remediation pathway has been outlined.
Conclusions
Bioremediation could be a potential solution to the problems associated with MPs/NPs. However, their identification and quantification methods are yet to be standardized and consensus needs to be built on them, which can facilitate the development process of impact indicators for MPs/NPs; thus, evaluating their environmental impacts. Biodegradable plastics that are produced from non-edible biomass such as algae can be a potential pathway to eradicate MP pollution for sustainable ecosystems. In addition, efforts can be as follows, along with minimizing plastic littering and regulatory efforts to mitigate MP pollution, but not limited to them. However, any innovative attempt to mitigate or eradicate MP/NP pollution must be justified with a broader sustainability check to avoid any risk to investment and the environment.
• Design and development of alternatives to conventional plastics that ease plastic waste disposal while avoiding generating MPs/NPs during their applications.
• Employ an integrated approach that can eradicate the evolving problems associated with MPs/NPs.
• Provide evidence of MP/NP free alternatives to conventional plastic to environmental activists, policymakers and end-users, which may accelerate the eradication process of MPs/NPs from ecosystems and resolve some of the evolving problems associated with them.
Abbreviations
ADP | Abiotic depletion potential |
AP | Acidification potential |
BPA | Bisphenol A |
DOC | Dissolved organic carbon |
DOM | Dissolved organic matter |
EDCs | Endocrine-disrupting |
EEI-O | Environmentally extended input–output |
EP | Eutrophication potential |
GHG | Greenhouse gas |
GWP | Global warming potential |
HDPE | High-density polyethylene |
HTP | Human toxicity potential |
LDPE | Low-density polyethylene |
ME | Marine ecotoxicity |
MPs | Microplastics |
MSW | Municipal solid waste |
NPs | Nanoplastics |
ODP | Ozone depletion potential |
PAEs | Phthalic acid esters |
PAHs | Polycyclic aromatic hydrocarbons |
PBAT | Polybutyrate adipate-co-terephthalate |
PBDEs | Polybrominated diphenyl ethers |
PBSA | Polybutylene succinate-co-butylene adipate |
PCBs | Polychlorinated biphenyls |
PCL | Polycaprolactone |
PE | Polyethylene |
PET | Polyethylene terephthalate |
PHA | Polyhydroxyalkanoate |
PLA | Polylactic acid |
PO | Photochemical oxidation |
POM | Particle organic matter |
PP | Polypropylene |
PS | Polystyrene |
PVC | Polyvinyl chloride |
TE | Terrestrial ecotoxicity |
UK | United Kingdom |
USA | United States of America |
WHO | World Health Organization |
WWTPs | Wastewater treatment plants |
Author contributions
Project conceptualization, methodology, administration, funding acquisition and supervision, A. K. M. and M. M.; methodology, investigation, data analysis, writing—original draft preparation, P. R.; writing—review and editing, A. K. M., M. M. and P. R. All authors contributed to the discussion, reviews and approval of the manuscript for publication.
Conflicts of interest
The authors declare that they have no known conflict of interest that could have appeared to influence the work reported in this paper.
Acknowledgements
This study was financially supported by the Ontario Ministry of Agriculture, Food and Rural Affairs (OMAFRA) – University of Guelph, the Bioeconomy Industrial Uses Research Program Theme (Project No. 030351, 030486, and 030578); OMAFRA – University of Guelph Gryphon's Leading to the Accelerated Adoption of Innovative Research (LAAIR) Program (Project No. 030416); OMAFRA – Ontario Agri-Food Research Initiative (Project No. 055217); the Ontario Ministry of Economic Development, Job Creation and Trade ORF-RE09-078 (Project No. 053970, 054345); the Natural Sciences and Engineering Research Council of Canada (NSERC), Canada Research Chair (CRC) program Project No. 460788; and the Agriculture and Agri-Food Canada (AAFC), Maple Leaf Foods, Canada and the Bank of Montreal (BMO), Canada through Bioindustrial Innovation Canada (BIC) Bioproducts AgSci Cluster Program (Project No. 054015, 054449 and 800148).
References
-
R. Lord, Plastics and Sustainability: A Valuation of Environmental Benefits, Costs and Opportunities for Continuous Improvement, https://www.plasticpackagingfacts.org/wp-content/uploads/2016/11/ACC-report-July-2016.pdf, accessed December 13, 2020 Search PubMed.
-
M. Garside, Global Plastic Production 1950-2018, https://www.statista.com/statistics/282732/global-production-of-plastics-since-1950/, accessed October 9, 2020 Search PubMed.
-
H. Ritchie, Plastic Pollution, https://ourworldindata.org/plastic-pollution, accessed January 5, 2021 Search PubMed.
-
Statista, Distribution of Plastic Waste Generation Worldwide in 2018, by Sector, https://www.statista.com/statistics/1166582/global-plastic-waste-generation-by-sector/, accessed October 9, 2020 Search PubMed.
-
S. Wahba, S. Kaza, and K. M. Ionkova, A New Phenomenon – Realizing Economic Growth while Cutting Waste. How?, https://blogs.worldbank.org/sustainablecities/new-phenomenon-realizing-economic-growth-while-cutting-waste-how Search PubMed.
-
I. Tiseo, Weight of U.S. Municipal Solid Plastic Waste Generated 1960-2017, https://www.statista.com/statistics/1097290/us-plastic-waste-generation/, accessed October 9, 2020 Search PubMed.
-
ECCC, Science Assessment of Plastic Pollution, https://www.canada.ca/en/environment-climate-change/services/evaluating-existing-substances/science-assessment-plastic-pollution.html, accessed October 8, 2020 Search PubMed.
-
R. Young, Canada's Plastic Problem: Sorting Fact from Fiction, https://oceana.ca/en/blog/canadas-plastic-problem-sorting-fact-fiction, accessed October 9, 2020 Search PubMed.
-
ECCC, Economic Study of the Canadian Plastic Industry, Markets and Waste: Summary Report to Environment and Climate Change Canada, http://publications.gc.ca/collections/collection_2019/eccc/En4-366-1-2019-eng.pdf, accessed October 10, 2020 Search PubMed.
-
B. Friesen, Agricultural Waste Management in Canada, United States, New Zealand and Australia, 2018 Search PubMed.
- M. B. Anunciado, D. G. Hayes, A. F. Astner, L. C. Wadsworth, C. D. Cowan-Banker, J. E. L. y. Gonzalez and J. M. DeBruyn, J. Polym. Environ., 2021, 1–16 CrossRef CAS.
- B. Xu, F. Liu, Z. Cryder, D. Huang, Z. Lu, Y. He, H. Wang, Z. Lu, P. C. Brookes and C. Tang, Crit. Rev. Environ. Sci. Technol., 2020, 50, 2175–2222 CrossRef CAS.
-
CleanFARMS, Cleanfarma Plastic Recycling Project to be Ramped up, https://www.greenhousecanada.com/cleanfarms-plastic-recycling-project-to-be-ramped-up/, accessed October 14, 2020 Search PubMed.
-
GESAMP, Sources, Fate and Effects of Microplastics in the Marine Environment: a Global Assessment, http://41.89.141.8/kmfri/bitstream/123456789/735/1/GESAMP_microplasticsfullstudy.pdf, accessed October 12, 2020 Search PubMed.
- M. C. Rillig, L. Ziersch and S. Hempel, Sci. Rep., 2017, 7, 1–6 CrossRef CAS PubMed.
- Y. Qi, X. Yang, A. M. Pelaez, E. H. Lwanga, N. Beriot, H. Gertsen, P. Garbeva and V. Geissen, Sci. Total Environ., 2018, 645, 1048–1056 CrossRef CAS PubMed.
- B. Boots, C. W. Russell and D. S. Green, Environ. Sci. Technol., 2019, 53, 11496–11506 CrossRef CAS PubMed.
- P. Alexy, E. Anklam, T. Emans, A. Furfari, F. Galgani, G. Hanke, A. Koelmans, R. Pant, H. Saveyn and B. Sokull Kluettgen, Food Addit. Contam., Part A, 2020, 37, 1–10 CrossRef CAS PubMed.
- B. Toussaint, B. Raffael, A. Angers-Loustau, D. Gilliland, V. Kestens, M. Petrillo, I. M. Rio-Echevarria and G. Van den Eede, Food Addit. Contam., Part A, 2019, 36, 639–673 CrossRef CAS PubMed.
- L. Ding, S. Zhang, X. Wang, X. Yang, C. Zhang, Y. Qi and X. Guo, Sci. Total Environ., 2020, 137525 CrossRef CAS PubMed.
- E. Watt, M. Picard, B. Maldonado, M. A. Abdelwahab, D. F. Mielewski, L. T. Drzal, M. Misra and A. K. Mohanty, RSC Adv., 2021, 11, 21447–21462 RSC.
- J. L. Conkle, C. D. B. Del Valle and J. W. Turner, Environ. Manage., 2018, 61, 1–8 CrossRef PubMed.
- P. Agamuthu, Waste Management & Research: The Journal for a Sustainable Circular Economy, 2018 DOI:10.1177/0734242X18796770.
- E. Mendenhall, Mar. Policy, 2018, 96, 291–298 CrossRef.
- M. Smith, D. C. Love, C. M. Rochman and R. A. Neff, Curr. Environ. Health Rep., 2018, 5, 375–386 CrossRef CAS PubMed.
- M. B. Zobkov and E. E. Esiukova, Oceanology, 2018, 58(1), 137–143 CrossRef.
- B. Zhou, J. Wang, H. Zhang, H. Shi, Y. Fei, S. Huang, Y. Tong, D. Wen, Y. Luo and D. Barceló, J. Hazard. Mater., 2020, 388, 121814 CrossRef CAS PubMed.
- R. C. Hale, M. E. Seeley, M. J. La Guardia, L. Mai and E. Y. Zeng, J. Geophys. Res.: Oceans, 2020, 125, e2018JC014719 Search PubMed.
- F. Yu, C. Yang, Z. Zhu, X. Bai and J. Ma, Sci. Total Environ., 2019, 694, 133643 CrossRef CAS PubMed.
- S. Freeman, A. M. Booth, I. Sabbah, R. Tiller, J. Dierking, K. Klun, A. Rotter, E. Ben-David, J. Javidpour and D. L. Angel, J. Environ. Manage., 2020, 266, 110642 CrossRef CAS PubMed.
- K. Hu, W. Tian, Y. Yang, G. Nie, P. Zhou, Y. Wang, X. Duan and S. Wang, Water Res., 2021, 117144 CrossRef CAS PubMed.
- M. Malankowska, C. Echaide-Gorriz and J. Coronas, Environ. Sci.: Water Res. Technol., 2021, 7, 243–258 RSC.
- Y. Picó and D. Barceló, ACS Omega, 2019, 4, 6709–6719 CrossRef PubMed.
- R. Qi, D. L. Jones, Z. Li, Q. Liu and C. Yan, Sci. Total Environ., 2020, 703, 134722 CrossRef CAS PubMed.
- J.-J. Guo, X.-P. Huang, L. Xiang, Y.-Z. Wang, Y.-W. Li, H. Li, Q.-Y. Cai, C.-H. Mo and M.-H. Wong, Environ. Int., 2020, 137, 105263 CrossRef CAS PubMed.
- J. Wang, X. Liu, Y. Li, T. Powell, X. Wang, G. Wang and P. Zhang, Sci. Total Environ., 2019, 691, 848–857 CrossRef CAS PubMed.
- W. Wang, H. Gao, S. Jin, R. Li and G. Na, Ecotoxicol. Environ. Saf., 2019, 173, 110–117 CrossRef CAS PubMed.
-
E. K. Arora, Macro-Plastics To Micro-Plastics – An Uncomfortable Convenience!, NISCAIR-CSIR, India, 2018 Search PubMed.
-
L. Arreola and J. Fulton, Microplastic Pollution in the Ocean Affecting Marine Life and its Potential Risk to Human Health, 2018 Search PubMed.
- N. J. Beaumont, M. Aanesen, M. C. Austen, T. Börger, J. R. Clark, M. Cole, T. Hooper, P. K. Lindeque, C. Pascoe and K. J. Wyles, Mar. Pollut. Bull., 2019, 142, 189–195 CrossRef CAS PubMed.
- G. Krantzberg, J. Waste Resour. Recycl., 2019, 1(1), 107 Search PubMed.
- B. Carney Almroth and H. Eggert, Rev. Environ. Econ. Policy., 2020, 13(2), 317–326 CrossRef.
- R. Geyer, J. R. Jambeck and K. L. Law, Sci. Adv., 2017, 3, e1700782 CrossRef PubMed.
-
C. Giacovelli, Single-Use Plastics: A Roadmap for Sustainability, 2018 Search PubMed.
- D. Calleja, Field Actions Sci. Rep., 2019, 22–27 Search PubMed.
-
J. Aldag, The last straw: Turning the tide on plastic pollution in Canada, Report of the Standing Committee on Environment and Sustainable Development, House of Commons, Canada, 2019 Search PubMed.
- J. R. Banu, V. G. Sharmila, U. Ushani, V. Amudha and G. Kumar, Sci. Total Environ., 2020, 718, 137287 CrossRef CAS PubMed.
- S. Nanda and F. Berruti, Environ. Chem. Lett., 2020, 1–26 Search PubMed.
-
P. Roy and A. Dutta, in Plastics to Energy, Elsevier, 2019, pp. 377–402 Search PubMed.
- H. W. Ryu, D. H. Kim, J. Jae, S. S. Lam, E. D. Park and Y.-K. Park, Bioresour. Technol., 2020, 123473 CrossRef CAS PubMed.
- M. S. Qureshi, A. Oasmaa, H. Pihkola, I. Deviatkin, A. Tenhunen, J. Mannila, H. Minkkinen, M. Pohjakallio and J. Laine-Ylijoki, J. Anal. Appl. Pyrolysis, 2020, 152, 104804 CrossRef CAS.
- M. Liu, S. Lu, Y. Song, L. Lei, J. Hu, W. Lv, W. Zhou, C. Cao, H. Shi and X. Yang, Environ. Pollut., 2018, 242, 855–862 CrossRef CAS PubMed.
- E.-L. Ng, E. H. Lwanga, S. M. Eldridge, P. Johnston, H.-W. Hu, V. Geissen and D. Chen, Sci. Total Environ., 2018, 627, 1377–1388 CrossRef CAS PubMed.
-
M. Wagner and S. Lambert, Freshwater Microplastics: Emerging Environmental Contaminants?, Springer Nature, 2018 Search PubMed.
-
P. Bose, Microbial Degradation of Plastic Waste and the PETase Enzyme, https://www.azom.com/article.aspx?ArticleID=19280, accessed February 9, 2021 Search PubMed.
- A. Chamas, H. Moon, J. Zheng, Y. Qiu, T. Tabassum, J. H. Jang, M. Abu-Omar, S. L. Scott and S. Suh, ACS Sustainable Chem. Eng., 2020, 8, 3494–3511 CrossRef CAS.
- M. N. Miranda, A. M. T. Silva and M. F. R. Pereira, Sci. Total Environ., 2020, 718, 134968 CrossRef CAS PubMed.
- T. S. Galloway, M. Cole and C. Lewis, Nat. Ecol. Evol., 2017, 1, 1–8 CrossRef PubMed.
- N. Khalid, M. Aqeel and A. Noman, Environ. Pollut., 2020, 115653 CrossRef CAS PubMed.
- M. Pirsaheb, H. Hossini and P. Makhdoumi, Process Saf. Environ. Prot., 2020, 142, 1–4 CrossRef CAS.
- B. Gewert, M. M. Plassmann and M. MacLeod, Environ. Sci.: Processes Impacts, 2015, 17, 1513–1521 RSC.
- A. A. Shah, F. Hasan, A. Hameed and S. Ahmed, Biotechnol. Adv., 2008, 26, 246–265 CrossRef CAS PubMed.
- R. Wei and W. Zimmermann, Microb. Biotechnol., 2017, 10, 1308–1322 CrossRef CAS PubMed.
- J. D. Meeker, S. Sathyanarayana and S. H. Swan, Philos. Trans. R. Soc., B, 2009, 364, 2097–2113 CrossRef CAS PubMed.
- K. Pivnenko, M. K. Eriksen, J. A. Martín-Fernández, E. Eriksson and T. F. Astrup, Waste Manage., 2016, 54, 44–52 CrossRef CAS PubMed.
- H. Zhang, Q. Zhou, Z. Xie, Y. Zhou, C. Tu, C. Fu, W. Mi, R. Ebinghaus, P. Christie and Y. Luo, Sci. Total Environ., 2018, 616, 1505–1512 Search PubMed.
- A. C. Godswill and A. C. Godspel, Int. J. Bioinf. Comput. Biol., 2019, 4, 11–29 Search PubMed.
- J. N. Hahladakis, C. A. Velis, R. Weber, E. Iacovidou and P. Purnell, J. Hazard. Mater., 2018, 344, 179–199 CrossRef CAS PubMed.
- F. Nasser and I. Lynch, J. Proteomics, 2016, 137, 45–51 CrossRef CAS PubMed.
- M. P. Johansen, E. Prentice, T. Cresswell and N. Howell, J. Environ. Radioact., 2018, 190, 130–133 CrossRef PubMed.
- J. T. Turner, Prog. Oceanogr., 2015, 130, 205–248 CrossRef.
- R. Trevisan, D. Uzochukwu and R. T. Di Giulio, Front. Environ. Sci., 2020, 8, 78 CrossRef PubMed.
- M. Zhang and L. Xu, Crit. Rev. Environ. Sci. Technol., 2020, 1–37 Search PubMed.
- X. Guo and J. Wang, Mar. Pollut. Bull., 2019, 142, 1–14 CrossRef CAS PubMed.
- G. Liu, Z. Zhu, Y. Yang, Y. Sun, F. Yu and J. Ma, Environ. Pollut., 2019, 246, 26–33 CrossRef CAS PubMed.
- J. Li, K. Zhang and H. Zhang, Environ. Pollut., 2018, 237, 460–467 CrossRef CAS PubMed.
- M. A. Pascall, M. E. Zabik, M. J. Zabik and R. J. Hernandez, J. Agric. Food Chem., 2005, 53, 164–169 CrossRef CAS PubMed.
- A. P. da Costa Araújo, N. F. S. de Melo, A. G. de Oliveira Junior, F. P. Rodrigues, T. Fernandes, J. E. de Andrade Vieira, T. L. Rocha and G. Malafaia, J. Hazard. Mater., 2020, 382, 121066 CrossRef PubMed.
- G. Renner, T. C. Schmidt and J. Schram, Curr. Opin. Environ. Sci. Health, 2018, 1, 55–61 CrossRef.
- S. Zhang, J. Wang, X. Liu, F. Qu, X. Wang, X. Wang, Y. Li and Y. Sun, TrAC, Trends Anal. Chem., 2019, 111, 62–72 CrossRef CAS.
- E. Dümichen, P. Eisentraut, C. G. Bannick, A.-K. Barthel, R. Senz and U. Braun, Chemosphere, 2017, 174, 572–584 CrossRef PubMed.
- Z. Steinmetz, A. Kintzi, K. Muñoz and G. E. Schaumann, J. Anal. Appl. Pyrolysis, 2020, 147, 104803 CrossRef CAS.
- A. Käppler, D. Fischer, S. Oberbeckmann, G. Schernewski, M. Labrenz, K.-J. Eichhorn and B. Voit, Anal. Bioanal. Chem., 2016, 408, 8377–8391 CrossRef PubMed.
- S. Huppertsberg and T. P. Knepper, Anal. Bioanal. Chem., 2018, 410, 6343–6352 CrossRef CAS PubMed.
-
G. Gourmelon, Global Plastic Production Rises, Recycling Lags, https://www.commondreams.org/newswire/2015/01/28/global-plastic-production-rises-recycling-lags, accessed October 21, 2020 Search PubMed.
- J. R. Jambeck, R. Geyer, C. Wilcox, T. R. Siegler, M. Perryman, A. Andrady, R. Narayan and K. L. Law, Science, 2015, 347, 768–771 CrossRef CAS PubMed.
-
Sloactive, Plastic Pollution, https://sloactive.com/plastic-pollution/, accessed October 21, 2020 Search PubMed.
- E. J. Carpenter, S. J. Anderson, G. R. Harvey, H. P. Miklas and B. B. Peck, Science, 1972, 178, 749–750 CrossRef CAS PubMed.
- A. Isobe, K. Uchida, T. Tokai and S. Iwasaki, Mar. Pollut. Bull., 2015, 101, 618–623 CrossRef CAS PubMed.
- R. W. Obbard, S. Sadri, Y. Q. Wong, A. A. Khitun, I. Baker and R. C. Thompson, Earth's Future, 2014, 2, 315–320 CrossRef.
- S. A. Mason, V. G. Welch and J. Neratko, Front. Chem., 2018, 6, 407 CrossRef PubMed.
- R. K. Naik, M. M. Naik, P. M. D'Costa and F. Shaikh, Mar. Pollut. Bull., 2019, 149, 110525 CrossRef CAS PubMed.
- S. Allen, D. Allen, V. R. Phoenix, G. Le Roux, P. D. Jiménez, A. Simonneau, S. Binet and D. Galop, Nat. Geosci., 2019, 12, 339–344 CrossRef CAS.
- F. Belzagui, M. Crespi, A. Álvarez, C. Gutiérrez-Bouzán and M. Vilaseca, Environ. Pollut., 2019, 248, 1028–1035 CrossRef CAS PubMed.
- C. K. M. Chan, C. Park, K. M. Chan, D. C. W. Mak, J. K. H. Fang and D. M. Mitrano, Environ. Chem., 2021, 8(3), 93–100 CrossRef.
- L. Hou, D. Kumar, C. G. Yoo, I. Gitsov and E. L.-W. Majumder, Chem. Eng. J., 2021, 406, 126715 CrossRef CAS.
- S. Uddin, S. W. Fowler and M. Behbehani, Mar. Pollut. Bull., 2020, 160, 111538 CrossRef CAS PubMed.
- F. De Falco, M. Cocca, V. Guarino, G. Gentile, V. Ambrogi, L. Ambrosio and M. Avella, Polym. Degrad. Stab., 2019, 165, 110–116 CrossRef CAS.
- M. Bergmann, V. Wirzberger, T. Krumpen, C. Lorenz, S. Primpke, M. B. Tekman and G. Gerdts, Environ. Sci. Technol., 2017, 51, 11000–11010 CrossRef CAS PubMed.
- M. Eriksen, L. C. M. Lebreton, H. S. Carson, M. Thiel, C. J. Moore, J. C. Borerro, F. Galgani, P. G. Ryan and J. Reisser, PLoS One, 2014, 9, e111913 CrossRef PubMed.
- I. A. Kane, M. A. Clare, E. Miramontes, R. Wogelius, J. J. Rothwell, P. Garreau and F. Pohl, Science, 2020, 368, 1140–1145 CrossRef CAS PubMed.
- C. Jiang, L. Yin, Z. Li, X. Wen, X. Luo, S. Hu, H. Yang, Y. Long, B. Deng and L. Huang, Environ. Pollut., 2019, 249, 91–98 CrossRef CAS PubMed.
- G. De Bhowmick, A. K. Sarmah and B. Dubey, Case Stud. Chem. Environ. Eng., 2021, 3, 100076 CrossRef.
- S. Ziajahromi, P. A. Neale, I. T. Silveira, A. Chua and F. D. L. Leusch, Chemosphere, 2021, 263, 128294 CrossRef CAS PubMed.
- R. M. Blair, S. Waldron, V. R. Phoenix and C. Gauchotte-Lindsay, Environ. Sci. Pollut. Res., 2019, 26, 12491–12504 CrossRef PubMed.
- K. R. Vanapalli, B. K. Dubey, A. K. Sarmah and J. Bhattacharya, Case Stud. Chem. Environ. Eng., 2021, 3, 100071 CrossRef.
- P. J. Thomas, R. Oral, G. Pagano, S. Tez, M. Toscanesi, P. Ranieri, M. Trifuoggi and D. M. Lyons, Mar. Environ. Res., 2020, 161, 105132 CrossRef CAS PubMed.
- L.-Z. Zuo, H.-X. Li, L. Lin, Y.-X. Sun, Z.-H. Diao, S. Liu, Z.-Y. Zhang and X.-R. Xu, Chemosphere, 2019, 215, 25–32 CrossRef CAS PubMed.
- A. A. Horton, A. Walton, D. J. Spurgeon, E. Lahive and C. Svendsen, Sci. Total Environ., 2017, 586, 127–141 CrossRef CAS PubMed.
- L. Nizzetto, M. Futter and S. Langaas, Environ. Sci. Technol., 2016, 10777–10779 CrossRef CAS PubMed.
- C. M. Rochman, Science, 2018, 360, 28–29 CrossRef CAS PubMed.
- F. Corradini, P. Meza, R. Eguiluz, F. Casado, E. Huerta-Lwanga and V. Geissen, Sci. Total Environ., 2019, 671, 411–420 CrossRef CAS PubMed.
- R. R. Hurley and L. Nizzetto, Curr. Opin. Environ. Sci. Health, 2018, 1, 6–11 CrossRef.
- P. van den Berg, E. Huerta-Lwanga, F. Corradini and V. Geissen, Environ. Pollut., 2020, 261, 114198 CrossRef CAS PubMed.
- C. M. Rochman and T. Hoellein, Science, 2020, 368, 1184–1185 CrossRef CAS PubMed.
- J. Crossman, R. R. Hurley, M. Futter and L. Nizzetto, Sci. Total Environ., 2020, 724, 138334 CrossRef CAS PubMed.
- X. Ren, J. Tang, C. Yu and J. He, J. Agro-Environ. Sci., 2018, 37, 1045–1058 Search PubMed.
- A. Mohajerani and B. Karabatak, Waste Manage., 2020, 107, 252–265 CrossRef CAS PubMed.
- Y. Changrong, H. Wenqing and C. Neil, World Agric., 2014, 4, 32–36 Search PubMed.
- M. Scheurer and M. Bigalke, Environ. Sci. Technol., 2018, 52, 3591–3598 CrossRef CAS PubMed.
- S. Zhang, X. Yang, H. Gertsen, P. Peters, T. Salánki and V. Geissen, Sci. Total Environ., 2018, 616, 1056–1065 CrossRef PubMed.
- Y. Huang, Q. Liu, W. Jia, C. Yan and J. Wang, Environ. Pollut., 2020, 260, 114096 CrossRef CAS PubMed.
- D. He, Y. Luo, S. Lu, M. Liu, Y. Song and L. Lei, TrAC, Trends Anal. Chem., 2018, 109, 163–172 CrossRef CAS.
- L. He, G. Gielen, N. S. Bolan, X. Zhang, H. Qin, H. Huang and H. Wang, Agron. Sustainable Dev., 2015, 35, 519–534 CrossRef CAS.
- M. Shi, Y. Sun, Z. Wang, G. He, H. Quan and H. He, Environ. Pollut., 2019, 250, 1–7 CrossRef CAS PubMed.
- S. Piehl, A. Leibner, M. G. J. Löder, R. Dris, C. Bogner and C. Laforsch, Sci. Rep., 2018, 8, 1–9 Search PubMed.
- X. J. Jiang, W. Liu, E. Wang, T. Zhou and P. Xin, Soil Tillage Res., 2017, 166, 100–107 CrossRef.
- M. Zhang, B. Dong, Y. Qiao, H. Yang, Y. Wang and M. Liu, Field Crops Res., 2018, 225, 130–140 CrossRef.
- J. Wang, A. Taylor, C. Xu, D. Schlenk and J. Gan, Environ. Pollut., 2018, 238, 462–470 CrossRef CAS PubMed.
- M. C. Rillig and A. Lehmann, Science, 2020, 368, 1430–1431 CrossRef CAS PubMed.
- P. He, L. Chen, L. Shao, H. Zhang and F. Lü, Water Res., 2019, 159, 38–45 CrossRef CAS PubMed.
- H. Liu, X. Yang, G. Liu, C. Liang, S. Xue, H. Chen, C. J. Ritsema and V. Geissen, Chemosphere, 2017, 185, 907–917 CrossRef CAS PubMed.
- M. C. Rillig, Environ. Sci. Technol., 2018, 52, 6079–6080 CrossRef CAS PubMed.
- M. Brodhagen, J. R. Goldberger, D. G. Hayes, D. A. Inglis, T. L. Marsh and C. Miles, Environ. Sci. Policy, 2017, 69, 81–84 CrossRef.
- E. K. Liu, W. Q. He and C. R. Yan, Environ. Res. Lett., 2014, 9, 91001 CrossRef.
- M. Karamanlioglu and G. D. Robson, Polym. Degrad. Stab., 2013, 98, 2063–2071 CrossRef CAS.
- S. Kubowicz and A. M. Booth, Environ. Sci. Technol., 2017, 51(21), 12058–12060 CrossRef CAS PubMed.
- A. F. Astner, D. G. Hayes, H. O'Neill, B. R. Evans, S. V Pingali, V. S. Urban and T. M. Young, Sci. Total Environ., 2019, 685, 1097–1106 CrossRef CAS PubMed.
- M. González-Pleiter, M. Tamayo-Belda, G. Pulido-Reyes, G. Amariei, F. Leganés, R. Rosal and F. Fernández-Piñas, Environ. Sci.: Nano, 2019, 6, 1382–1392 RSC.
- A. S. Al Hosni, J. K. Pittman and G. D. Robson, Waste Manage., 2019, 97, 105–114 CrossRef CAS PubMed.
- A. Pischedda, M. Tosin and F. Degli-Innocenti, Polym. Degrad. Stab., 2019, 170, 109017 CrossRef CAS.
- D. Iram, R. Riaz and R. K. Iqbal, Open J. Environ. Biol., 2019, 4, 7–15 Search PubMed.
- J. Liao and Q. Chen, J. Hazard. Mater., 2021, 126329 CrossRef CAS PubMed.
- J. Rüthi, D. Bölsterli, L. Pardi-Comensoli, I. Brunner and B. Frey, Front. Environ. Sci., 2020, 8, 173 Search PubMed.
- S. Pignattelli, A. Broccoli and M. Renzi, Sci. Total Environ., 2020, 727, 138609 CrossRef CAS PubMed.
- K. Yokota and M. Mehlrose, Water, 2020, 12, 2650 CrossRef CAS.
- H. Zang, J. Zhou, M. R. Marshall, D. R. Chadwick, Y. Wen and D. L. Jones, Soil Biol. Biochem., 2020, 148, 107926 CrossRef CAS.
- F. Wang, X. Zhang, S. Zhang, S. Zhang and Y. Sun, Chemosphere, 2020, 254, 126791 CrossRef CAS PubMed.
- Y. Zhou, X. Liu and J. Wang, Sci. Total Environ., 2019, 694, 133798 CrossRef CAS PubMed.
- H. Tong, X. Hu, X. Zhong and Q. Jiang, Environ. Toxicol. Chem., 2021, 40, 72–78 CrossRef CAS PubMed.
- Y. M. Lozano and M. C. Rillig, Environ. Sci. Technol., 2020, 54, 6166–6173 CrossRef CAS PubMed.
- A. A. de Souza Machado, C. W. Lau, W. Kloas, J. Bergmann, J. B. Bachelier, E. Faltin, R. Becker, A. S. Görlich and M. C. Rillig, Environ. Sci. Technol., 2019, 53, 6044–6052 CrossRef CAS PubMed.
- J. Ru, Y. Huo and Y. Yang, Front. Microbiol., 2020, 11, 442 CrossRef PubMed.
- S. Miri, R. Saini, S. M. Davoodi, R. Pulicharla, S. K. Brar and S. Magdouli, Chemosphere, 2021, 131670 Search PubMed.
- J. C. Sanchez-Hernandez, Y. Capowiez and K. S. Ro, ACS Sustainable Chem. Eng., 2020, 8, 4292–4316 CrossRef CAS.
- M. Llorca, D. Álvarez-Muñoz, M. Ábalos, S. Rodríguez-Mozaz, L. H. Santos, V. M. León, J. A. Campillo, C. Martínez-Gómez, E. Abad and M. Farré, Trends Environ. Anal. Chem., 2020, e00090 CrossRef CAS.
- L. Li, Q. Zhou, N. Yin, C. Tu and Y. Luo, Chin. Sci. Bull., 2019, 64, 928–934 CrossRef.
- Y. Su, V. Ashworth, C. Kim, A. S. Adeleye, P. Rolshausen, C. Roper, J. White and D. Jassby, Environ. Sci.: Nano, 2019, 6, 2311–2331 RSC.
-
L. Li, J. Yang, Q. Zhou, W. J. G. M. Peijnenburg and Y. Luo, Uptake of microplastics and their effects on plants, in Microplastics in Terrestrial Environments. The Handbook of Environmental Chemistry, vol. 95, Springer, Cham, 2020 Search PubMed.
- E. C. Ebere, V. A. Wirnkor and V. E. Ngozi, World Sci. News, 2019, 131, 256–267 CAS.
- L. G. A. Barboza, C. Lopes, P. Oliveira, F. Bessa, V. Otero, B. Henriques, J. Raimundo, M. Caetano, C. Vale and L. Guilhermino, Sci. Total Environ., 2020, 717, 134625 CrossRef CAS PubMed.
- T. Saeed, N. Al-Jandal, A. Al-Mutairi and H. Taqi, Mar. Pollut. Bull., 2020, 152, 110880 CrossRef CAS PubMed.
- A. Lusher, P. Hollman and J. Mendoza-Hill, FAO Fish. Aquac. Tech. Pap., 2017, 9(9), 1346–1360 Search PubMed.
- Z. Cheng, J.-R. Chen, C. Zheng, Z.-B. Yang, X.-X. Xu and M.-H. Wong, Chemosphere, 2021, 276, 130189 CrossRef CAS PubMed.
-
P. Marsden, A. A. Koelmans, J. Bourdon-Lacombe, T. Gouin, L. D'Anglada, D. Cunliffe, P. Jarvis, J. Fawell and J. De France, Microplastics in Drinking Water, World Health Organization, 2019 Search PubMed.
- D. Schymanski, C. Goldbeck, H.-U. Humpf and P. Fürst, Water Res., 2018, 129, 154–162 CrossRef CAS PubMed.
- S. V. Panno, W. R. Kelly, J. Scott, W. Zheng, R. E. McNeish, N. Holm, T. J. Hoellein and E. L. Baranski, Groundwater, 2019, 57, 189–196 CrossRef CAS PubMed.
- A. A. Koelmans, N. H. M. Nor, E. Hermsen, M. Kooi, S. M. Mintenig and J. De France, Water Res., 2019, 155, 410–422 CrossRef CAS PubMed.
-
WHO, Microplastics in Drinking-Water, https://apps.who.int/iris/bitstream/handle/10665/326499/9789241516198-eng.pdf?ua=1, accessed January 19, 2021 Search PubMed.
- E. H. Lwanga, J. M. Vega, V. K. Quej, J. de los Angeles Chi, L. S. Del Cid, C. Chi, G. E. Segura, H. Gertsen, T. Salánki and M. van der Ploeg, Sci. Rep., 2017, 7, 1–7 CrossRef PubMed.
- Y. Song, C. Cao, R. Qiu, J. Hu, M. Liu, S. Lu, H. Shi, K. M. Raley-Susman and D. He, Environ. Pollut., 2019, 250, 447–455 CrossRef CAS PubMed.
- Y. Deng, Y. Zhang, B. Lemos and H. Ren, Sci. Rep., 2017, 7, 1–10 CrossRef PubMed.
- Y. Cho, W. J. Shim, M. Jang, G. M. Han and S. H. Hong, Environ. Pollut., 2019, 245, 1107–1116 CrossRef CAS PubMed.
-
B. Liebmann, S. Köppel, P. Königshofer, T. Bucsics, T. Reiberger and P. Schwabl, in Conference on Nano and Microplastics in Technical and Freshwater Systems, 2018 Search PubMed.
- P. Schwabl, S. Köppel, P. Königshofer, T. Bucsics, M. Trauner, T. Reiberger and B. Liebmann, Ann. Intern. Med., 2019, 171(7), 453–457 CrossRef PubMed.
- M. E. Iñiguez, J. A. Conesa and A. Fullana, Sci. Rep., 2017, 7, 1–7 CrossRef PubMed.
- A. Karami, A. Golieskardi, C. K. Choo, V. Larat, T. S. Galloway and B. Salamatinia, Sci. Rep., 2017, 7, 46173 CrossRef CAS PubMed.
- F. Zhang, Y. B. Man, W. Y. Mo, K. Y. Man and M. H. Wong, Crit. Rev. Environ. Sci. Technol., 2020, 50, 2109–2143 CrossRef.
- A. Mathalon and P. Hill, Mar. Pollut. Bull., 2014, 81, 69–79 CrossRef CAS PubMed.
- X. Qu, L. Su, H. Li, M. Liang and H. Shi, Sci. Total Environ., 2018, 621, 679–686 CrossRef CAS PubMed.
- S. Karbalaei, P. Hanachi, T. R. Walker and M. Cole, Environ. Sci. Pollut. Res., 2018, 25, 36046–36063 CrossRef CAS PubMed.
- G. Liebezeit and E. Liebezeit, Pol. J. Food Nutr. Sci., 2015, 65, 143–147 CrossRef CAS.
- A. Khalid, A. Zalouk-Vergnoux, S. Benali, R. Mincheva, J.-M. Raquez, S. Bertrand and L. Poirier, Mar. Pollut. Bull., 2021, 167, 112295 CrossRef CAS PubMed.
- D. M. Mitrano and W. Wohlleben, Nat. Commun., 2020, 11, 1–12 CrossRef PubMed.
-
Canada, Microbeads in Toiletries Regulations, http://www.gazette.gc.ca/rp-pr/p2/2017/2017-06-14/html/sor-dors111-eng.html, accessed February 10, 2021 Search PubMed.
- A. F. Herbort, M. T. Sturm and K. Schuhen, Environ. Sci. Pollut. Res., 2018, 25, 15226–15234 CrossRef CAS PubMed.
-
Blueair, Blueair Air Purifiers Remove Microplastics from the Air, https://www.blueair.com/ca/blue-family-page.html?, accessed January 29, 2021 Search PubMed.
- M. I. Ali, S. Ahmed, G. Robson, I. Javed, N. Ali, N. Atiq and A. Hameed, J. Basic Microbiol., 2014, 54, 18–27 CrossRef CAS PubMed.
- J. C. Prata, Environ. Pollut., 2018, 234, 115–126 CrossRef CAS PubMed.
-
L. Wuennenberg and C. M. Tan, Plastic Waste in Canada: A daunting economic and environmental threat or an opportunity for sustainable public procurement?, 2019, https://www.iisd.org/articles/plastic-waste-canada, accessed on January 23, 2021 Search PubMed.
-
CleanFARMS, Ontario Agricultural Waste Study: Environmental Impacts of Open-Burning Agricultural Plastics, 2011 Search PubMed.
- H. Jeswani, C. Krüger, M. Russ, M. Horlacher, F. Antony, S. Hann and A. Azapagic, Sci. Total Environ., 2021, 769, 144483 CrossRef CAS PubMed.
- H. H. Khoo, Resour., Conserv. Recycl., 2019, 145, 67–77 CrossRef.
-
UK-Government, LCA of Management Options for Mixed Waste Plastics, https://www.wrap.org.uk/sites/files/wrap/LCA%20of%20Management%20Options%20for%20Mixed%20Waste%20Plastics.pdfs#0, accessed December 25, 2020 Search PubMed.
- Y. Aryan, P. Yadav and S. R. Samadder, J. Cleaner Prod., 2019, 211, 1268–1283 CrossRef CAS.
-
L. Wood, Global Plastic Recycling Market Report 2021: Market to Reach $47.3 Billion by 2026 from $33 Billion in 2020 – ResearchAndMarkets.com, https://www.businesswire.com/news/home/20211130005878/en/Global-Plastic-Recycling-Market-Report-2021-Market-to-Reach-47.3-Billion-by-2026-from-33-Billion-in-2020---ResearchAndMarkets.com, accessed December 3, 2021 Search PubMed.
-
OMAFRA, Recycling Farm Plastic Films, http://www.omafra.gov.on.ca/english/engineer/facts/95-019.htm#11, accessed October 14, 2020 Search PubMed.
-
StatisticsCanada, Canada at a Glance, Environment Edition, https://www150.statcan.gc.ca/n1/pub/12-581-x/2017001/sec-5-eng.htm, accessed October 14, 2020 Search PubMed.
-
IBISWorld, Waste Collection Services in Canada – Market Research Report, https://www.ibisworld.com/canada/market-research-reports/waste-collection-services-industry/, accessed October 14, 2020 Search PubMed.
-
Canada, NAICS 3261 Plastic Products Industry (Total), https://www.ic.gc.ca/eic/site/plastics-plastiques.nsf/eng/pl00312.html, accessed February 1, 2021 Search PubMed.
- W.-L. Wang, Q.-Y. Wu, C. Wang, T. He and H.-Y. Hu, Environ. Sci. Pollut. Res., 2015, 22, 3620–3630 CrossRef CAS PubMed.
- O. Edjere, A. C. Ibezute and O. E. Oghama, Int. Res. J. Pure Appl. Chem., 2020, 18–28 CrossRef.
- L. Wei, Z. Li, J. Sun and L. Zhu, Sci. Total Environ., 2020, 726, 137978 CrossRef CAS PubMed.
- B. Zhou, L. Zhao, Y. Sun, X. Li, L. Weng and Y. Li, Sci. Total Environ., 2021, 778, 146281 CrossRef CAS PubMed.
- Z. Steinmetz, C. Wollmann, M. Schaefer, C. Buchmann, J. David, J. Tröger, K. Muñoz, O. Frör and G. E. Schaumann, Sci. Total Environ., 2016, 550, 690–705 CrossRef CAS PubMed.
- J. Wang, S. Lv, M. Zhang, G. Chen, T. Zhu, S. Zhang, Y. Teng, P. Christie and Y. Luo, Chemosphere, 2016, 151, 171–177 CrossRef CAS PubMed.
- X. Li, W. Zhang, J. Lv, W. Liu, S. Sun, C. Guo and J. Xu, Environ. Sci. Eur., 2021, 33, 1–14 CrossRef.
-
WHO, Concise International Chemical Assessment Document 52. Geneva, Switz World Heal Organ, 2003, https://www.who.int/ipcs/publications/cicad/en/cicad52.pdf, accessed on March 1, 2021 Search PubMed.
- R. Kavlock, K. Boekelheide, R. Chapin, M. Cunningham, E. Faustman and P. Foster, Reprod. Toxicol., 2002, 16, 529–653 CrossRef CAS PubMed.
- K. V. S. Rajmohan, C. Ramya, M. R. Viswanathan and S. Varjani, Curr. Opin. Environ. Sci. Health, 2019, 12, 72–84 CrossRef.
- A. L. Lusher, N. A. Welden, P. Sobral and M. Cole, Anal. Methods, 2017, 9, 1346–1360 RSC.
-
N. K. Y. Susanti, A. Mardiastuti and Y. Wardiatno, in IOP Conference Series: Earth and Environmental Science, IOP Publishing, 2020, vol. 528, p. 12013 Search PubMed.
- E. Besseling, A. Wegner, E. M. Foekema, M. J. Van Den Heuvel-Greve and A. A. Koelmans, Environ. Sci. Technol., 2013, 47, 593–600 CrossRef CAS PubMed.
- L. Lei, S. Wu, S. Lu, M. Liu, Y. Song, Z. Fu, H. Shi, K. M. Raley-Susman and D. He, Sci. Total Environ., 2018, 619, 1–8 CrossRef PubMed.
- Y. Ma, A. Huang, S. Cao, F. Sun, L. Wang, H. Guo and R. Ji, Environ. Pollut., 2016, 219, 166–173 CrossRef CAS PubMed.
- W. Wang, J. Ge, X. Yu and H. Li, Sci. Total Environ., 2020, 708, 134841 CrossRef CAS PubMed.
- J. Kang, L. Zhou, X. Duan, H. Sun, Z. Ao and S. Wang, Matter, 2019, 1, 745–758 CrossRef CAS.
- H. Ye, Y. Wang, X. Liu, D. Xu, H. Yuan, H. Sun, S. Wang and X. Ma, J. Colloid Interface Sci., 2021, 588, 510–521 CrossRef CAS PubMed.
- I. A. Ricardo, E. A. Alberto, A. H. S. Júnior, D. L. P. Macuvele, N. Padoin, C. Soares, H. G. Riella, M. C. V. M. Starling and A. G. Trovó, Chem. Eng. J., 2021, 130282 CrossRef CAS.
- X. Jiao, K. Zheng, Q. Chen, X. Li, Y. Li, W. Shao, J. Xu, J. Zhu, Y. Pan and Y. Sun, Angew. Chem., Int. Ed., 2020, 59, 15497–15501 CrossRef CAS PubMed.
- X. Jie, W. Li, D. Slocombe, Y. Gao, I. Banerjee, S. Gonzalez-Cortes, B. Yao, H. AlMegren, S. Alshihri and J. Dilworth, Nat. Catal., 2020, 3, 902–912 CrossRef CAS.
-
J. Nikiema, J. Mateo-Sagasta, Z. Asiedu, D. Saad and B. Lamizana, Water pollution by plastics and microplastics: a review of technical solutions from source to sea, 2020, https://www.unep.org/resources/report/water-pollution-plastics-and-microplastics-review-technical-solutions-source-sea, accessed on January 15, 2021 Search PubMed.
- C. Dussud and J.-F. Ghiglione, CIESM Workshop Monogr., 2014, 46, 49–54 Search PubMed.
- E. H. Lwanga, B. Thapa, X. Yang, H. Gertsen, T. Salánki, V. Geissen and P. Garbeva, Sci. Total Environ., 2018, 624, 753–757 CrossRef PubMed.
- P. Bhatt, V. M. Pathak, A. R. Bagheri and M. Bilal, Environ. Res., 2021, 111762 CrossRef CAS PubMed.
- J. Fojt, J. David, R. Přikryl, V. Řezáčová and J. Kučerík, Sci. Total Environ., 2020, 745, 140975 CrossRef CAS PubMed.
- X. u Zhao, Z. Li, Y. Chen, L. Shi and Y. Zhu, J. Mol. Catal. A: Chem., 2007, 268, 101–106 CrossRef.
- Y. Hu, M. Gong, J. Wang and A. Bassi, Rev. Environ. Sci. Bio/Technol., 2019, 18, 207–230 CrossRef.
- B. Ma, W. Xue, Y. Ding, C. Hu, H. Liu and J. Qu, J. Environ. Sci., 2019, 78, 267–275 CrossRef PubMed.
- J. W. Park, S. J. Lee, D. Y. Hwang and S. Seo, RSC Adv., 2021, 11, 3556–3566 RSC.
- M. Sarcletti, H. Park, J. Wirth, S. Englisch, A. Eigen, D. Drobek, D. Vivod, B. Friedrich, R. Tietze and C. Alexiou, Mater. Today., 2021, 48, 38–46 CrossRef CAS.
- N. Mohanan, Z. Montazer, P. K. Sharma and D. B. Levin, Front. Microbiol., 2020, 11, 2837 Search PubMed.
- W. Y. Chia, D. Y. Y. Tang, K. S. Khoo, A. N. K. Lup and K. W. Chew, Environ. Sci. Ecotechnol., 2020, 100065 CrossRef.
- A. Nakanishi, K. Iritani and Y. Sakihama, J. Nanotechnol. Nanomater., 2020, 1(2), 72–85 Search PubMed.
- S. Ju, G. Shin, M. Lee, J. M. Koo, H. Jeon, Y. S. Ok, D. S. Hwang, S. Y. Hwang, D. X. Oh and J. Park, Green Chem., 2021, 23(8), 6953–6965 RSC.
Footnote |
† Electronic supplementary information (ESI) available. See DOI: 10.1039/d1va00012h |
|
This journal is © The Royal Society of Chemistry 2022 |