DOI:
10.1039/D0QM00090F
(Review Article)
Mater. Chem. Front., 2020,
4, 1586-1613
Advances in carbon dots: from the perspective of traditional quantum dots
Received
17th February 2020
, Accepted 16th March 2020
First published on 17th March 2020
Abstract
Quantum dots (QDs) have been the core concept of nanoscience and nanotechnology since their inception, and play a dominant role in the development of the nano-field. Carbon dots (CDots), defined by a feature size of <10 nm, have become a rising star in the crossover field of carbon materials and traditional QDs (TQDs). CDots possess many unique structural, physicochemical and photochemical properties that render them a promising platform for biology, devices, catalysis and other applications. However, due to the complex nature of CDots, to gain a profound understanding of the physical and chemical properties of CDots is still a great challenge. Many key issues including structure, synthesis, and optical properties are unclear, leaving open arguments and nonuniform description of their basics. The achievements and experience of TQDs in the past four decades are expected to provide crucial values for further development of CDots. Here, we will compare the research outputs of TQDs and CDots and try to give a comprehensive picture of their differences and correlations in structure, synthesis, properties and applications. Along this line, further perspectives are given on future directions and key issues toward a better understanding of the basic properties of TQDs and CDots in a unified manner. We expect the researchers in the community to foresee the great potential of CDots, and focus on the critical results obtained during the development of TQDs, paying special attention to the profound principles behind the synthetic chemistry, luminescence mechanisms and applications.
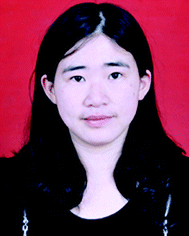
Yanhong Liu
| Yanhong Liu is currently an assistant professor at the School of Chemistry and Chemical Engineering, Jiangsu University, P. R. China. Her research interest focuses on the design, fabrication and catalytic application of hybrid nanostructures based on quantum dots and 2D materials. |
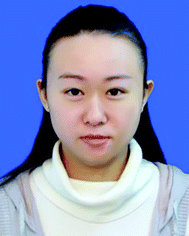
Hui Huang
| Hui Huang is currently an associate professor at the Institute of Functional Nano & Soft Materials and the Jiangsu Key Laboratory for Carbon-Based Functional Materials & Devices in Soochow University, P. R. China. She obtained her PhD in 2013 from Soochow University. Her research interests include design and synthesis of composite nanomaterials based on carbon, metals, semiconductors and polyoxometallates as well as their application. |
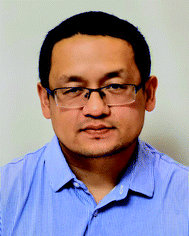
Baodong Mao
| Baodong Mao is currently a professor at the School of Chemistry and Chemical Engineering, Jiangsu University, P. R. China. He obtained his PhD in 2012 from Case Western Reserve University, USA. His current research interest mainly focuses on the spectroscopic understanding and photocatalytic and electrocatalytic applications of environment-friendly quantum dots. |
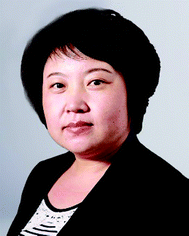
Yang Liu
| Yang Liu is currently a professor at the Institute of Functional Nano & Soft Materials and the Jiangsu Key Laboratory for Carbon-Based Functional Materials & Devices in Soochow University, P. R. China. Her main research field is dedicated to the synthesis and assembly of morphologically unique nanostructures and nanocomposites, and their applications in catalysis and energy conversion. |
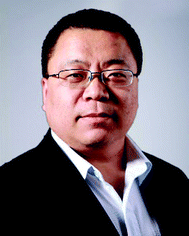
Zhenhui Kang
| Zhenhui Kang is currently a professor at the Institute of Functional Nano & Soft Materials and the Jiangsu Key Laboratory for Carbon-Based Functional Materials & Devices in Soochow University, P. R. China. His main research interests are in the fields of the synthesis and surface chemistry of carbon dots, as well as exploring their applications in nanocatalysis, new energy and biology. |
1. Introduction
Quantum dots (QDs) are a concept explicitly proposed in the 1980s, in which the charge carrier transportation is limited in three dimensions and the continuous band structure in the bulk becomes discrete energy levels, exhibiting many physicochemical properties dramatically different from their bulk counterparts.1 Since the birth of QDs, they have been the core concept of nanoscience, and have been guiding the development of physics, chemistry and materials science in related fields.2–4 They still play an important role in nanoscience today after nearly four decades. Many milestones in nanoscience and nanotechnology actually come from QDs related research, such as the discovery of the quantum confinement effect, size-controlled synthesis, biological and device applications etc., which have played a crucial role in the establishment of basic concepts and principles in the nanofield.5–9 In addition to the traditional cadmium-based II–VI and lead-based IV–VI QDs, environment-friendly carbon and other two-dimensional materials have attracted extensive research interest.10–12 Carbon dots (CDots) were naturally named QDs since their introduction in 2004.13,14 As a subgroup of QDs, the novel structure, synthetic chemistry, and optical properties of CDots are all different from traditional QDs (TQDs), which poses a series of new challenges for the development and understanding of the QDs family. CDots not only exhibit strong luminescence and small size characteristics similar to TQDs, but also own the unique advantages of good aqueous dispersibility, low toxicity and high conductivity that are usually absent in TQDs.15–20 CDots have received great attention in the fields of electronics, optics, photoelectrochemistry, catalysis and biology. After a decade of rapid development, a large number of publications have emerged in the CDots field. However, there are still many ongoing debates and non-unified understanding of CDots on their synthesis, optical properties and applications, where many critical basic issues remain unclear.21–24
In popular application fields of TQDs, such as solar cells, light-emitting diodes (LEDs), bioimaging and photocatalysis, CDots have started to play an increasingly important role in recent years (Fig. 1). In these applications, researchers have accumulated a lot of experience in the preparation, structure manipulation and particle processing of TQDs, which are worthy learning or consulting for CDots.25–29 For example, TQDs have made great progress and reached extraordinary merits in terms of structure control and performances, such as the size distribution of <5%, PL full width at half maximum (FWHM) of <20 nm, bad-QD ratio of <1% in devices, and high carrier mobility near the theoretical values.6,9 Besides the well-established fields of TQDs, CDots have also started to play a decisive role in a number of emerging new fields, including but not limited to electrocatalysis, energy storage and agriculture, owing to their own structural advantages.23,24 However, CDots still have some disadvantages in terms of key properties, such as the difficulty in obtaining consistent standard samples, characterization, and in-depth mechanistic studies. On the other hand, recent work has demonstrated several unique advantages of CDots transcending TQDs in optical, catalytic, and biological applications. Some promising characteristics, such as multi-electron accepting and donating, may bring about excellent device and catalytic performances in the near future.
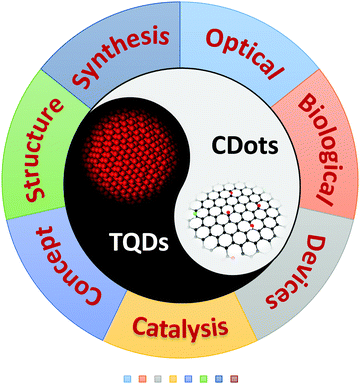 |
| Fig. 1 Introduction of TQDs and CDots. | |
Although there have been some reviews summarizing the synthesis, optical properties, biology and device applications of CDots, it is now necessary to clarify some basic concepts by systematically comparing CDots with TQDs and to re-examine the unique characteristics of CDots from the perspective of TQDs,30,31 especially the growth mechanism and optical properties. We hope that a comprehensive clarification of CDots from the perspective of TQDs could provide a useful guidance at the basic concept level, and subsequently a deeper understanding of the challenges, advantages and disadvantages of CDots.
2. Concepts and history
2.1 TQDs
TQDs technology dates back to the 1970s in an effort to solve the global energy crisis. TQDs are defined as semiconductor nanostructures in which the conduction band (CB) electrons, valence band (VB) holes, and excitons (electron–hole pairs) are all constrained in three dimensions. The concept of QDs was explicitly proposed in the 1980s based on the experimental and theoretical research of CdS nanocrystals.1,32,33 In terms of structure, TQDs are quasi-zero-dimensional particles composed of a small number of atoms, usually with sizes of 1–10 nm for lots of TQDs, which are less than or close to the exciton Bohr radius of the corresponding semiconductors. TQDs are generally made of II–VI (CdS, CdSe and CdTe), IV–VI (PbS and PbSe) and III–V (InP and InAs) semiconductors. Since the motion of electrons and holes is limited, the continuous band structure becomes discrete, which is called the quantum confinement effect or quantum size effect.4 This results in the band gap enlargement with size decrease as well as other subsequent phenomena. TQDs often exhibit molecular size-dependent electronic and physicochemical properties different from bulk materials, namely the quantum confinement effect, surface effect, dielectric confinement effect, quantum tunneling effect and Coulomb blockade effect.2,3 One of the most prominent properties of TQDs is that size-dependent photoluminescence (PL) with different colors can occur after being excited. Here are several milestones in TQDs research: (1) differently sized CdS particles producing different colors, and accordingly proposal of the “quantum confinement effect”;1 (2) the organometallic hot-injection method in 1993 enabling delicate size control,25 and later CdO-based hot-injection method to replace the explosive dimethyl cadmium around 2001;26 (3) decisive wave function engineering for photophysical and electronic device research; (4) the use of TQDs as fluorescent markers in living cell systems in 1998.34,35 After more than 30 years of development, TQDs have evolved from the original single-component particles to currently complex structures with different components and heterojunctions. In some ways, TQDs can be considered as a bridge between small molecules and bulk semiconductor crystals.
2.2 CDots
In general, CDots can be defined as spherical carbon particles (graphite or amorphous fragments) with sizes <10 nm. To some extent, CDots are known as a subgroup of QDs, as well as a crossover of the luminescent semiconductor QDs and carbon materials.31,36 Historically, CDots were first discovered in arc-discharged soot in 2004, from which the carbon-based PL attracted tremendous attention.13,14 In 2006, surface passivation with polymers (i.e. polyethylene glycol, PEG) was employed to enhance their PL emissions.15 In 2010, highly crystalline CDots were synthesized from graphite and purified, showing size-dependent blue, yellow and red PL.20 Currently, CDots with well-defined chemical structures and controlled morphology are being pursued, such as carbonized polymer dots, chiral CDots, triangular CDots and crystalline C3N CDots.37–40 To date, CDots have exhibited many unique properties, such as tunable PL, good dispersibility, low toxicity, high biocompatibility, and low cost. Researchers in the community have realized the urgency of the standardization and deepening of CDots research,23,24 with reference to the concepts and development history of TQDs. In this field, an unavoidable question is the diversity and complexity of the core structure ranging from amorphous to crystalline. In terms of structure and catalytic properties, the highly crystalline CDots seem superior to the amorphous ones.20 This also brought various names and descriptions for CDots in the past decade, such as “carbon dots”, “carbon nanodots”, “carbon quantum dots”, “graphene dots”, “graphene nanodots”, “graphene quantum dots”, “graphite dots”, “graphite nanodots”, “polymer dots”, and “carbonized polymer dots”.21–23,37,41 It is worth noting that ‘quantum dots’ or even ‘quantum size effect’ is appearing more and more frequently in related reports as the keywords, e.g. there is a clear trend for the concern of ‘quantum size effect’ in CDots research. Thus, for CDots, it is still necessary to understand and discuss their basic structure and properties from the perspective of TQDs. In the meantime, the use of the “quantum size effect” on CDots should be very careful since the spectral shifts of CDots do not necessarily represent a real size-dependant quantum confinement effect and are often related to surface states or doping.42,43 Considering the close relevance of TQDs and CDots research, we hope that the perspective of discussion here is not limited to the quantum confinement effect and optical properties, but extends to a broader view of the basics of CDots in different aspects.
3. Structure
3.1 TQDs
TQDs are usually faceted or quasi-spherical crystalline particles with sizes of about 1–10 nm depending on the material (Fig. 2A).44 They typically contain an inorganic crystalline core coated with various ligands, such as surfactant molecules, and are usually fabricated as a colloid suspended in a solution (Fig. 2B).9 Surface ligands play a crucial role in the synthesis, processing, and application of TQDs. In practice, the dangling bonds that expose the small crystal facet are passivated by ligands via atomic or molecular bonding to minimize the intra-band surface states and to reduce surface atom reconstruction. It is also beneficial to coat TQDs with a wide-bandgap inorganic shell to stabilize the surface, which not only deactivates the surface bonds, but also keeps the photogenerated charge carriers away from the surface and environment.6 Depending on the number of electrons involved and the electron donating/accepting groups, the capping ligands of TQDs can be classified into three categories:27,46 (1) The L-type ligands are neutral two-electron donors with a lone pair of electrons that can coordinate with surface metal atoms, including amines (RNH2), phosphines (R3P) and phosphine oxide (R3PO); (2) X-type ligands have an odd number of valence electrons that can form a two-electron covalent bond, such as carboxylates (RCOO−), thiolates (RS−), phosphonates (RPO(OH)O−), simple inorganic ions (Cl−, Br−, SCN−, etc.), or a combination of these; (3) Z-type ligands, such as Pb(OOCR)2 or CdCl2, can interact with surface metal atoms of metal chalcogenides and oxides with electron-rich Lewis basic sites, forming a two-electron acceptor. Surface ligands with L- or X-type head groups and long hydrocarbon-chain tails are often used to control the nucleation and growth kinetics of QDs during synthesis. However, in many cases, these original or natural ligands must be replaced with other suitable ligands for device and biological applications considering charge transfer or biocompatibility.46 The size and shape control of the inorganic core has played a critical role in the property manipulation of TQDs.5,47 A large number of studies have also been devoted to the doping, alloying, lattice stress tuning, and heterostructure design of TQDs to adjust their band gap and optical properties.48–50 The heterostructures, i.e. QDs containing two or more regions of different materials in close contact, provide great opportunities for achieving novel optoelectronic properties and charge carrier behaviors, including type-I, type-II and quasi-type-II heterostructures based on the band alignment.6,9
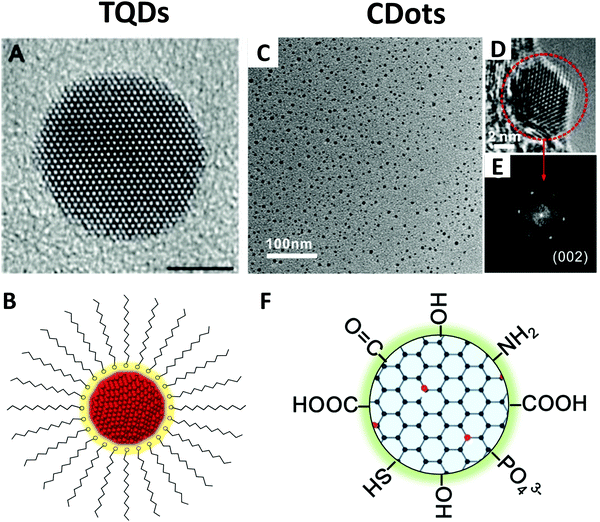 |
| Fig. 2 Structure of TQDs and CDots. Left: HRTEM image (A) and the schematic core/ligand structure (B) of TQDs; Reprinted with permission from ref. 44, Copyright 2003, The Royal Society of Chemistry. Right: TEM (C), HRTEM (D), SAED pattern (E) and the scheme for core/surface group structure (F) of CDots. Reprinted with permission from ref. 45, Copyright 2017, American Chemical Society. | |
TQDs can be considered as a bridge between small molecules and bulk crystals, showing discrete energy levels like isolated atoms and molecules, as well as the original properties of crystalline bulk semiconductors.4,47,51 TQDs of uniform size and shapes are also referred to as “artificial atoms” and can self-assembly to super lattices acting as building blocks.52 The self-assembly of TQDs to form a perfect “crystal phase material” may be a key to bring about a revolution in optoelectronic properties, and push TQD devices into practical applications.
3.2 CDots
CDots can be considered as a special “core–shell” nanostructure with a carbon core of less than 10 nm and a functional group shell (Fig. 2C–F).45 A typical TEM image of CDots shows two lattice fringes, namely, (002) and (110) lattices of graphite. It is worth noticing that, different from TQDs, CDots are not always crystalline, and the amorphous CDots are usually from carbonization of organic precursors.53 According to the structure of carbon cores, CDots have also been named with different origins, such as graphene QDs, carbon nanodots and carbonized polymer dots.37,54 Single-layer CDots have one layer of graphene fragments as the carbon core, while multilayer CDots have graphite nanocrystal structures.55,56 More frequently, CDots are roughly defined or referred to as quasi-spherical carbon nanoparticles mainly consisting of sp2/sp3-hybrid carbon or sp2-domains embedded in amorphous carbon.21,23 CDots usually have various functional groups on the surface, such as hydroxyl, epoxy/ether, carbonyl and carboxylic groups (Fig. 2F), which are actually quite different from the concept of surface ligands in TQDs with a functional head group and a long hydrocarbon tail (Fig. 2B). The surface functional groups in CDots provide a rich oxygen-containing moiety on their surface and are favorable for water solubility and further functionalization for various applications.57–61 In terms of surface ligand bonding, CDots actually have a completely different core/surface structure from TQDs. The surface functional groups are covalently bonded to the carbon core via C–C or other C–X bonds (X refers to O, N, S etc.), different from the coordination interaction of the capping ligands and surface metal atoms in TQDs (Fig. 2Bvs.Fig. 2F).27 However, due to their complex synthetic chemistry, the detailed chemical structure of CDots is still unclear, especially the surface defects, doping atoms or covalently bonded functional groups. Although heteroatom doping and surface modification have been used to adjust the chemical structure and properties of CDots, there is still no report on the undebated bandgap engineering and wave function engineering strategies.43,62 It is worth noting that CDot-related doping, epitaxial modification and heterojunction design have great difficulties due to the structure characteristics of the carbon cores, which show dramatically different behavior from TQDs. We should realize that the doping of CDots employs chemical knowledge completely different from TQDs, and more related research studies should be designed from the perspective of organic synthesis. Along this line, surface functionalization based on delicate organic synthesis may be more preferred rather than heteroatom doping for CDots.22,24,63
One of the greatest advantages of CDots is that they bridge traditional carbon materials and organic molecules.24,62 The carbon-like core and the organic-like surface together determine the nature of CDots, which blend the properties of carbon materials with small organic molecules, giving great flexibility in the manipulation of structure and physiochemical properties.36 We should pay attention to the fact that the clarification of the CDot structure is not an isolated problem, but should be comprehensively considered in correlation with the structures of inorganic TQDs, nanographites, graphene, and polycyclic aromatic hydrocarbons.62–66
4. Synthesis and purification
4.1 TQDs
Although several kinds of methods were developed for the preparation of TQDs, currently it is mainly focused on the organometallic hot-injection method (Fig. 3A).12,27–29,67 In the early 1980s, the synthesis of QDs was based on traditional chemical methods for preparing colloidal particles, such as precipitation, microemulsions and micelles.68 In 1993, the first monodispersed CdS, CdSe and CdTe QDs were fabricated by using trioctylphosphine oxide (TOPO) as a coordinating solvent to treat dimethyl cadmium (CdMe2) and trioctylphosphine selenium with controlled temperature at 300–360 °C under an argon atmosphere.25 This method, commonly referred to as the ‘high temperature metal organic-coordinating solvent route’, successfully achieved the continuous regulation of the size and optical properties of QDs for the first time. Synthetic principles established in this experiment are still widely used today, such as ligand-capped metal precursors, phosphine chalcogen precursors, fast nucleation via injection, separation of nucleation and growth processes, and purification of QDs after synthesis. It has been widely explored for different kinds of QDs, including II–VI, IV–VI and III–V semiconductors.29 The method was then further improved, and CdO was used instead of the highly toxic and explosive CdMe2 to form the cadmium phosphate precursor, which is more widely employed in the state-of-the-art procedures and even the commercial production of QDs.26 In general, capping ligands have several key functions in the synthesis of QDs: (1) forming molecular precursors and further evolving into “monomers”; (2) preventing uncontrolled aggregation of monomers and nuclei; (3) regulating the nucleation and growth kinetics for the size and shape control of QDs; (4) stabilizing the QDs.46 Obviously, it is difficult to find a single molecule that meets all these requirements, so two or more ligands are often used simultaneously in most synthetic routes. Alloying, doping and heterostructure design also imposes new challenges for QD synthesis, for which a series of new methods were developed, such as seeded-growth, successive ionic layer adsorption and reaction (SILAR), nucleation-/growth-doping, gradient interfacial alloying, and selective cation exchange.5,29,48–50,69
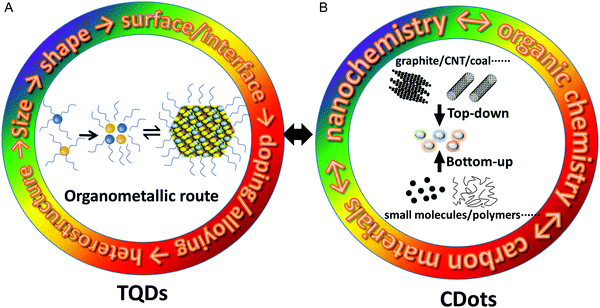 |
| Fig. 3 Schematic synthetic strategies of TQDs and CDots. | |
Large effort has been devoted to the growing kinetics of the QDs, where the size distribution is largely determined by the uniformity of the core during nucleation.70 The LaMer model is widely used for describing the growth mechanism of QDs based on thermodynamic principles.71–73 The basic idea is that the metal precursors and chalcogenide precursors first react to form monomers that coalesce into larger nuclei, which then grow into QDs by monomer “addition” (e.g. Precursor → Monomer ⇌ QDs, Fig. 3A). Since the growing rate caused by the “addition” of the monomer is the reciprocal of the radius R of the QDs, smaller particles grow faster than larger ones. As the monomer is consumed, it is impossible to continue monomer addition to obtain larger QDs. One of the main drawbacks of the LaMer model is that it does not consider changes in the particle size distribution during the growing stage. In addition, the QDs’ growth process in solution can be accompanied by the Ostwald ripening process, which is caused by the larger solubility of small particles throughout the system (Kelvin effect) and leads to a wider size distribution.74In situ characterization techniques, such as liquid transmission electron microscopy and small-angle X-ray scattering, can be used to understand the reaction kinetics by obtaining crystal structures of the precursors and intermediate species during nucleation and growth stages at different reaction times.75–78 Another critical aspect is the synthetic chemistry, for which the amine/phosphine chalcogenides and metal salts as reactive precursors have evolved for more than two decades and render good control of the size, shape, composition and crystal structure of QDs. It is worth noting that the synthetic mechanism, in particular, relies on the molecular level understanding of the formation of metal–chalcogenide bonds in the initial stage, which is also an important process for CDots, but with totally different chemistry.28
4.2 CDots
The fabrication methods of CDots can be generally divided into two broad categories: top-down and bottom-up approaches (Fig. 3B).21–23 The top-down approaches involve “crushing” larger carbon structures (graphite, graphene, carbon nanotubes (CNTs), carbon black, coal etc.) into smaller nanoscale particles under harsh conditions, such as arc discharge, laser ablation, electrochemical etching and chemical oxidation.15,17,79,80 The bottom-up approaches are based on the synthesis and carbonization from small molecules (carbohydrates, organic acids and amines) or polymer precursors under relatively simple and mild conditions, such as hydrothermal treatment, ultrasonic reactions and microwave-assisted pyrolysis.39,53,81,82 Generally speaking, the top-down approaches facilitate the fabrication of CDots with high crystallinity and a relatively intact structure,20 while the bottom-up approaches tend to produce CDots containing amorphous carbon cores with abundant doping sites and functional groups. In the bottom-up synthesis, the key factors affecting the chemical structure and size of CDots include the molecular structure of precursors, solvent, reaction time and temperature.39,81,82 The selected reaction conditions are critical because of their strong influence on the reactants as well as the highly random nucleation and growing processes of CDots, though size- and composition-controlled CDots have been reported by careful optimization of reaction parameters. In terms of synthetic manipulation, the top-down approaches of CDots are totally different from those of TQDs, for which the size-selective separation and post-synthesis processing are the main considerations.20 In contrast, the bottom-up approaches have a certain correlation with the synthetic chemistry of TQDs, where the use of suitable ligands, regulation of precursor reactivity, and separation of the nucleation and growing stages play important roles for the size and composition of CDots.25,26,28 As mentioned above, the concept of capping ligands is rarely used in CDot synthesis, whereas surface functional groups are more appropriate. Preparation of CDots with monodispersed size, shape and subsequently narrow PL is far from that of TQDs. Fortunately, high-quality triangular CDots with super narrow PL have made some progress recently.40,83 Organic chemistry methods of large conjugated molecules are also used to synthesize monodispersed CDots with the exact molecular structure of conjugated sp2 cores (containing more than 100 carbon atoms) and alkyl edges (serving as stabilizing moieties).24,62,63
For the synthetic mechanism of CDots, there is still a lack of detailed studies on the regulation of precursor reactivity and the separation of nucleation and growth stages as those employed in TQD syntheses, which are critical for the size and shape control.25,26,28 This is of course due to the fact that the carbonization reaction itself, a radical reaction, is more difficult to control than the ionic reactions for TQDs. Thus, we should also explore new and more suitable reaction precursors to form CDots.39,40,84,85 Frequently, the required harsh conditions (e.g., high temperature and high pressure) in CDot synthesis result in complex multi-step organic reactions and various intermediates, which severely complicate the formation mechanism study.37,86,87 On one hand, the rich chemistry involved in CDot synthesis brings a large variety of CDots with useful properties, but critical review and rationalization of the synthetic processes are missing. For example, the use of various cheap biomass precursors in CDot synthesis may result in the unintentional introduction of trace non-metallic or metallic doping, and subsequently great changes in their structure and properties. On the other hand, one of the odd considerations is the industrial availability of the raw materials, where biowaste, like leaves and grass, seems less attractive.87–90 According to the development history of TQDs, we should realize that the synthetic methods applicable in future scale-up applications are more likely to be different from laboratory systems, where the product quality and consistency are more important parameters.91–93 It is worthwhile to note that CDot synthesis is actually at the crossroad of nano-, organic and materials chemistry (Fig. 3B). Along this line, some potential explorations may include: graphite electrochemical etching and surface functionalization, organic total synthesis, and controllable conversion of polymers with monodisperse molecular weight.37,63,89
4.3 Purification and separation
Purification is very important for the synthesis of colloidal particles, but is often overlooked.94–96 A relatively mature purification procedure has been established for TQDs to remove the excessive ligands and unreacted precursors based on polar solvent induced aggregation and centrifugation cycles.25,26 Usually, an excess of a polar solvent (such as methanol, ethanol, acetone, acetonitrile, etc.) is added to the colloidal TQD dispersion in a non-polar solvent to precipitate the QDs. After centrifugation, the QDs are located at the bottom of the centrifugation tube and the free ligands remain in solution. This procedure works well for most II–VI and IV–VI QDs. The efficiency of this method primarily depends on the solubility of the free ligands or other impurities in the solvent. For example, for InAs QDs, the usual centrifugation purification method cannot effectively remove the excess indium precursor because of its poor solubility.97 Poorly dispersed TQDs require a greater number of purification cycles, but there is a lack of quantitative description and discussion of this phenomenon. Another major drawback of centrifugation purification is the difficulty in controlling the surface ligand coverage and optical properties. For example, short-chain alcohols (such as methanol and ethanol) used for purification typically induce dissociation and release of the ligands (such as oleates) from the surface of TQDs, accompanied by a decrease in PL quantum yield.98 Other optional purification techniques include chromatography and electrophoresis, but are much less used in the preparation of TQDs due to the complicated operation, high cost, and the lack of suitable stationary phase compatible with TQDs in non-polar solvents.
Compared with TQDs, the separation and purification of CDots are actually more challenging due to the large number of hydrophilic functional groups and the small density of the carbon cores.15,20 Most of the synthesized CDots are accompanied by various reactants and by-products, which need to be removed by combining filtration/centrifugation to remove larger particles and dialysis to remove small molecules or ions.53,87,99 In some cases, CDots obtained after simple purification still cannot meet the high standards of characterization and practical applications due to the diversity in size, defects and surface functional groups. Further development of post-synthesis separation techniques is necessary for high-quality CDots. Dialysis, ultrafiltration, electrophoresis and column chromatography have been successfully used to obtain purified and size-adjustable CDs. The use of high-resolution separation techniques, namely, anion exchange high performance liquid chromatography or the combination of dialysis tubes with different cutoff molecular weights (Mw), has shown the potential to obtain CDots of different sizes with a narrow size distribution.100 In addition, similar to the simple aggregation/centrifugation procedure of TQDs, recent work has also demonstrated the separation of CDots based on solvent polarity tuning in chromatographic purification. In addition to these relatively complicated purification procedures of CDots, an alternative electrochemical method has also been developed for scale production of high-quality CDots using only graphite rods and ultrapure water, where the as-obtained CDots in pure water with no other impurities are feasible for lots of applications directly.89 It is worth noting that, besides size distribution, a few studies have paid attention to the phase, structure and surface uniformity of CDots, in which impurities such as amorphous carbon, oligomers and carbon particles are inevitably present. Further development of new separation and purification methods of CDots, such as hydrophilicity gradient ultracentrifugation,96,101 is still required with the consideration of the size, morphology, crystallinity and surface-specific factors,40 which are of particular importance for optical property regulation and biomedical and device applications, as observed for TQDs.8,9,102
5. Optical properties
5.1 TQDs
One of the most prominent properties of TQDs is the size-dependent emission colors resulting from the quantum confinement size effect. In general, the quantum confinement upon size decrease not only causes an increase in the band gap and a blue shift in absorption and PL, but also causes a change in the density of states (DOS).1,3,4 The quantum size effect together with composition change have endowed TQDs with a wide PL tuning range from UV to NIR (Fig. 4A).29,103 For direct bandgap semiconductors, the bandgap can be estimated from the first exciton peak by ignoring other sources of absorption other than VB to CB transitions. For indirect band gap ones, there is usually no sharp or well-defined exciton peaks and it is relatively difficult to observe the quantum size effect. The absorbance of TQDs follows Lambert–Beer's law, e.g. the molar extinction coefficient is a function of the particle concentration and is dependent on the size.104 Empirical fitting formulas of size vs. first exciton peak and the extinction coefficient vs. size were first reported for various QDs of CdS, CdSe, and CdTe, and have also been expanded to IV–VI and III–V QDs. The PL spectrum of TQDs contains many useful information and has become one of the most important ways to study the discrete band structures. The commonly reported parameters include the emission wavelength, PL quantum yield, and full width at half maximum (FWHM) that are critical for optical applications (Fig. 4B).29,103 Owing to the fact that the electron and hole wave functions are confined in a limited space as well as the continuously improved synthetic procedure, an optimized PL quantum yield of 100% has been reported in CdSe/CdS core/shell systems with super narrow FWHM.105,106
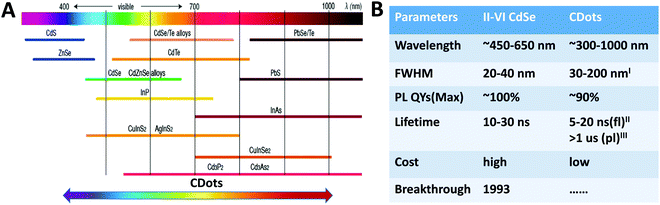 |
| Fig. 4 PL tuning range (A) and comparison of optical properties (B) of TQDs vs. CDots. Reproduced with permission from ref. 29 and 103, Copyright 2016 and 2018, American Chemical Society (IFWHM of 30 nm was only reported in ref. 40; IIfl: fluorescence; IIIpl: phosphorescence). | |
Besides steady-state optical properties, a profound understanding of the exciton dynamics plays a crucial role in TQDs research, which relies heavily on the direct detection of their lifetimes using ultrafast transient spectroscopy (Fig. 5A).6,102,107 Due to the relatively short lifetime of excitons, different methods based on transient absorption, time-resolved PL and spin dynamics have made exciton dynamics studies possible over the past two decades.108,109 Although the interpretation of ultrafast spectroscopy data is not as straightforward as experimental measurements (also difficult), a consistent and clear understanding of the exciton dynamics has gradually emerged over the past decades. The time scale of different kinetic processes depends on the variation of defects and surface properties determined by the quality of a particular QD sample. Some common key processes can be identified for all materials, including dephasing, intraband relaxation, trapping, and inter-band recombination of both free and trapped charge carriers (e.g. electrons and holes).3,47,109 In principle, hot electrons and holes (before relaxation to the band edges) can also undergo radiative or non-radiative recombinations, though the probability is usually very low. High-quality or near-perfect TQDs with a low density of trap states primarily experience radiative band-edge recombination, in which the PL quantum yield is very high and the associated PL lifetimes of direct-bandgap TQDs are typically on the order of a few to tens of nanoseconds. In typical TQDs with relatively high-density intraband defect states, non-radiative recombination becomes important where electrons or holes are trapped in these states, usually occurring on time scales of a few to tens of picoseconds. Therefore, the time scale of charge carrier capturing is usually faster than that of the band-edge radiative recombination. Subsequently, a typical result of high-density trap states is that the band edge PL is reduced and the overall PL quantum yield is usually low. After capturing, the charge carriers may still undergo additional capturing, such as transferring from shallow trap states to deeper ones, followed by subsequent radiative or non-radiative recombination. The time scale for further capturing strongly depends on the nature of the trap states involved, ranging from a few to hundreds of picoseconds. The lifetime of a trapped charge carrier can be very long, from a few hundred ps to ns, or even longer. If radiative recombination occurs from these trap states, a PL red shift (relative to the band edge emissions) will be observed, which can be easily distinguished. For dipole forcing or indirect bandgap transitions, the lifetime can be as long as ms or even longer.3,47,109
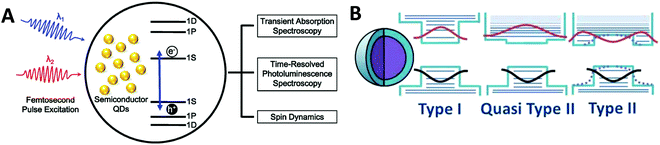 |
| Fig. 5 (A) Schemes of ultrafast spectroscopy studies of TQDs. Reprinted with permission from ref. 108, Copyright 2012, American Chemical Society. (B) Scheme of wave function engineering and heterostructure design of TQDs. Reprinted with permission from ref. 9, Copyright 2016, American Association for the Advancement of Science. | |
Bandgap engineering and wave function engineering have been feature concepts in the field of TQDs. The structure-dependent and composition-dependent properties developed beyond the quantum size effect have begun to play an important role in practical applications. In addition to size and shape control, a large number of studies have been devoted to alloying, doping, lattice stress tuning, and heterostructure design of TQDs to adjust their band gap and other optical properties (Fig. 5B).6,48–50,110–112 Briefly, alloying changes the band gap by mixing the two components together, while doping does not change the band gap, but adds new intra-band energy levels.48–50 Heterojunctions, including type-I, type-II and quasi type-II, have been employed according to the band alignment (Fig. 5B).6,9 Type-I heterojunctions have a nested band arrangement, causing electrons and holes to be mainly located in the core (normal type-I) or shell (inverted type-I). In type-II heterojunctions, the staggered arrangement of the conduction band and the valence band pulls the band edge electrons and holes into the core or the shell portion. In quasi-type-II heterojunctions, one carrier is confined in one component of the heterojunction and the other carrier is delocalized throughout the whole heterojunction, giving novel carrier dynamics and optical properties. Moreover, these quantum-confined TQDs exhibit other unique optical properties, especially PL blinking and multiple exciton generation (MEG).6,9,113 PL blinking refers to the intermittent luminescence shutdown of some TQDs observed in the single-particle spectrum, which is harmful to the PL quantum yields and LED applications, but is potentially useful for super-resolution bioimaging.114,115 It can be eliminated by forming a core/shell structure with a smooth gradient interface to prevent the Auger recombination and charged/ionized particles. When TQDs are excited by a photon with energy at least twice of the band gap, the generated electrons and holes can release their excessive kinetic energy by exciting a second electron in the collision-type Auger process, resulting in a double or even more excitons and an internal quantum efficiency greater than 100%, known as MEG. A profound understanding and manipulation of the MEG process plays an important role in the development of next-generation photovoltaics and lasers using TQDs.6,113
5.2 CDots
The quasi-bandgap emission and color regulation of the carbonaceous structures were observed in CDots that covers the deep UV to NIR region (Fig. 4A),116–122 but are still debating in terms of the quantum size effect.20,123 Compared with TQDs, the PL quantum yield and FWHM of CDots are still far behind (Fig. 4B).29,103 For TQDs, PL is usually used where the fluorescence and phosphoresce are not strictly distinguished, whereas both of the molecule-like fluorescence and phosphoresce behaviors may occur in CDots.16,20,37,123–125 Although CDots have highly variable chemical structures, most have similar absorption features with a strong absorption band in the UV region (230–320 nm) and a tail extends into the visible region. For the carbon core, the strong absorption peak at ∼230 nm is ascribed to the π–π* transition of the aromatic C–C bond, while the shoulder around 300 nm to the n–π* transition of C
O or other surface groups.123 Of course, accurate determination of extinction coefficients and quantum confinement is still controversial and far behind that for TQDs.104,126 This would first rely on the preparation of size-uniform and surface-well-controlled CDots, which is also an important part of CDots research to be supplemented.22,23 For the quantum confinement effect, density function theory (DFT) calculations have indicated that the band gap can be decreased from 7 eV of benzene to approximately 2 eV of CDots composed of 20 aromatic rings with increased sp2 domain size.21 However, such a perfect CDot structure with precisely tailored dimensions, is very difficult to obtain experimentally or to correlate with actual samples, in which the surface groups and defects often play a crucial role.61 Experimentally, Kang et al. demonstrated enhanced redshifted emission by manipulating the size and nature of electrochemically prepared and size-separated CDots. The bright blue, green, yellow and red fluorescence were produced as the size of the CDots increases. However, the size distribution of CDots made by different groups fluctuated significantly that increases the difficulty on the study of the quantum confinement effect. Moreover, most of the reported CDots are greater than 5 nm in diameter and have a PL emission in the visible range, whereas the theoretical band gap of CDots with this size is below 1.0 eV (with PL > 1240 nm) based on DFT calculations.127 Generally speaking, top-down routes often result in a relatively low quantum yield of CDots compared to the bottom-up routes, though specific precursors and technical conditions may bring different results. Many CDots exhibit an excitation-dependent PL with strong emission in the blue region that decreases rapidly in the red region, possibly due to the widespread trap states or recombination pathways related to different sizes and surface groups of CDots, for which the mechanism is currently unresolved. In practice, an in-depth and comprehensive insight into the mechanism of excitation-dependent PL is very beneficial for modulating their optical properties.22,23 Due to their structural diversity, CDots are also reported to exhibit other novel tunable optical properties, such as up-conversion PL,20 solid-state phosphorescence,88 lasing,128 and aggregation induced emission (AIE).129,130 However, correct index of these phenomena remains a major challenge because they are strongly influenced by the surface and overall nature of the CDot samples.61,127 For example, under the excitation of the Xe lamp, the so-called “up-conversion PL” in CDots in the early studies may be due to the second-order diffracted light (λex/2), which co-exists with the selected first-order light (λex) from the fluorescence spectrometer.20 Besides the PL properties, other photoelectrical properties from the carbonic nature of CDots have also attracted great interest due to their potential applications in biology, devices and catalysis.21,23 CDots have been reported to be able to accept/donate multiple electrons, produce photogenerated protons, and mediate photoinduced charge transfer, beyond the photogenerated electrons and holes.23 The conjugated sp2 domain of the carbon core renders great electron accepting/donating capability that is useful for charge transporting layers in solar cells and LEDs and for storing photogenerated electrons with a higher reduction potential in photocatalysis. The unique photoelectrical properties combined with versatile surface active sites provide great opportunities for design of multifunctional photocatalysts and electrocatalysts. Some CDots have also shown different toxicity under light illumination and in the dark, indicating special biological photoelectrical processes.
Different from TQDs, the decisive PL mechanism in CDots is still an open debate and requires in-depth clarification. To date, several PL mechanisms have been proposed: quantum confinement effect of the conjugated sp2-domains, defect states, molecular states, cross-linking-enhanced emission effects, and the core-surface electron coupling mechanism.16,20,37,123–125 A large number of studies have tested the PL lifetime of CDots with some reasonable explanations, where multiple decay pathways are generally included. However, the clarification of many intrinsic optical properties of TQDs comes from ultrafast photophysics study,108,109,131,132 which is still rare in CDots research and lacks a universal picture of the exciton dynamics for the whole system.132–135 It was reported that the characteristics of three kinds of CDots can be attributed to the same mode, where the main difference is the intensity of the stimulated emission. The photogenerated charge carriers are first stored in the carbon skeleton as a temporary reservoir, which then quickly transferred to any possible discharge state (hybrid structure of carbon skeleton and surface groups) and trap states (amorphous structures).19,21,37,42 However, some experimental results indicate a completely opposite behavior, indicating a size-independent PL. A more profound and systematic ultrafast spectroscopy study is required along with high-quality CDots from largely improved synthetic approaches.
In the structural regulation of TQDs, band gap engineering and wave function engineering are two key concepts and a series of regulation strategies have been developed, such as doping, alloying, heterostructures, etc.6,48–50 For CDots, doping and surface modification have brought fruitful though complicated results for the tuning of optical and electrochemical properties, but there are much fewer reports on the undebated bandgap engineering strategies.16,20,124,125,136,137 The precise surface control, doping, epitaxial growth, and heterojunction design have shown great difficulties due to the structure characteristics of the carbon core, different from TQDs.125,138 The earliest CDots were obtained by acid reflux oxidation, and the PL signal can only be detected after surface passivation by polymers.15 Various methods for surface modification of CDots have been developed by surface chemistry or strong molecular interactions (i.e. covalent bonding, coordination, and π–π interaction) to improve PL and regulate the band gap of CDots.21,58,84 For doping of the carbon core, there is still poor controllability, and the influence of doping elements on optical properties is unclear.43 It is worth noting that the doping and surface modification of CDots requires chemical knowledge completely different from TQDs, and should be considered from the perspective of organic chemistry.24,62,63 In terms of heterojunctions, the structure of copying type-I core–shell structure of TQDs, such as direct ZnS coating on CDots, did not yield good results. In contrast, surface passivation by sophisticated organic reactions is more controllable, where surface functional group can be selectively formed, eliminated or converted. CDot-based type-II heterojunctions are rarely seen. Actually, even in the composites of CDots and other semiconductors (such as TiO2), the concept of heterojunction is generally not favored because of the lack of explicit band alignment information, where CDots are simply referred to as an electron donor or acceptor, or a cocatalyst.139 An interesting phenomenon is the built-in internal heterojunction of CDots, where the functional oxygen species act as the p-type domain and the N-containing groups as the n-type domain embedded in the CDot framework.140 In addition, a combination of the heterojunction concept of TQDs and the electron donor/acceptor fragment design in organic electronics may bring more rational strategies for the design and construction of engineered CDots.62
Although the exact origin of fluorescence in CDots is still controversial, more in-depth research is undertaken towards a more clear and comprehensive understanding of the mechanism. CDots have significantly different optoelectronic properties from the traditional TQDs,20,88,129,130 and the elaboration of related excited state photophysics processes is critical for materials design and application, especially the excited state mechanistic studies.6,48–50 It would be beneficial for perusing the PL mechanism of CDots to consider at the crossroad of TQDs, graphene, small molecules and large conjugated systems (like polycyclic aromatic hydrocarbons and porphyrins).24,30,64,66,141,142 In addition, the optical properties of CDots are closely related to their synthetic route, and currently there are still no uncontroversial standard samples that can be widely adopted by different research groups.39,84,85
6. Biological applications
6.1 TQDs
As a new class of fluorophores, TQDs have multiple advantages for biological applications, such as strong and tunable emission, larger light absorbing interface, single particle level detection, and negligible photobleaching properties,7,8 making them superior to organic dyes or fluorescent proteins.143,144 Since the pioneering works in 1998,34,35 TQDs have been widely studied as important fluorescent biological probes, which have achieved a series of remarkable results in the fields of tissue imaging, in vivo imaging, targeted tracing in living cells and animals, as well as drug delivery and therapy (Fig. 6).7,8,145,146 For in vitro imaging, TQDs have made great progress in intracellular imaging of many living cells as well as components in fixed cells, such as proteins, nucleus, mitochondria, microtubules, microfilament proteins, cytokeratin, etc.7,8 At the same time, TQDs are also used to study cell membrane proteins and receptors, including prostate specific membrane antigen, HER kinase, glycine receptor, serotonin transport protein, p-glycoprotein, band III protein and sodium–potassium ATPase. For immunofluorescence applications, the capability of detecting trace signals represents one of the most important advantages, which can reach single-molecule-level detection employing their blinking characteristic. For in vivo imaging, TQDs emitting in the NIR range are very important because of the low absorption coefficients of water and hemoglobin in this range (650–900 nm, the NIR window), allowing for deeper tissue penetration (up to ∼10 mm).147–149 TQD probes can also be excited with the two-photon techniques using NIR light and thus allow deeper tissue penetration depths. The most prominent advantages of TQDs relies on super-resolution microscopy and single-particle tracking (Fig. 6).7,150,151 Super-resolution imaging can take full advantage of the PL blinking property of TQDs, which can be used to resolve closely-distributed QDs due to the complete PL on–off intermittence of individual QDs that can be detected (different QDs are unlikely to blink at the same time).115
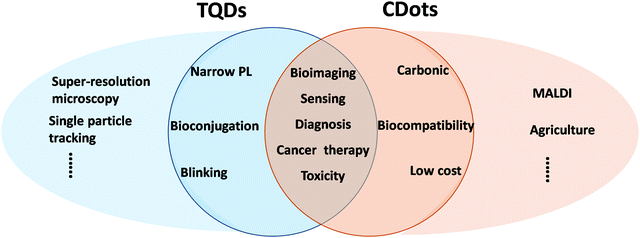 |
| Fig. 6 Schematic comparison of biological applications of TQDs and CDots. | |
For sensing applications, the TQD probes mainly include fluorescent probes and electrochemical probes.8,152–154 The characteristics of narrow PL peak, tunable bandgap, and multi-type electron transfer mechanisms have made TQDs one of the most favored fluorescent probes in the field of analysis based on PL quenching or recovery.7,8,153,155,156 Bioluminescence resonance energy transfer can be used to promote TQDs in an excited state, which is applicable to the excitation of TQDs of different colors.153,155,156 TQD-based fluorescence sensors have lower detection limits than commonly used enzyme-linked immunosorbent assays. For all these applications, surface ligands are the bridge between the TQD core and the biological environment, and play an important role in targeted recognition, detection, and drug delivery.27,46 Biomedical ligand design of TQDs relies on three aspects: robust anchoring of hydrophilic molecules on the surface, efficient rejection of proteins in the blood and cytoplasm, and controllable attachment of targeted bio-functional units. In addition to providing colloidal stability, a variety of different molecules (dyes, polymers, peptides, proteins, DNA, carbohydrates, etc.) have been attached as functional ligands to the surface of TQDs. Effective coupling of biomolecules to the surface of QDs while maintaining the optical properties and bioactivity is crucial for sensing and bioimaging applications.35,157,158
Another important topic is the potential toxic effects of TQDs, including the chemical composition (heavy metal ions), size (nanoscale) and the interference on biological systems.102,159–162 Thus, TQDs have not yet been implemented in clinical human imaging or drug delivery applications. Living organisms may have many interactions with TQDs (e.g. via proteins and ions), where the synergistic effects need to be carefully considered for in vivo applications. For example, the size of TQDs plays an important role in their distribution throughout the organisms.161,162 TQDs with a hydration radius of 5.5 nm or smaller and zwitterionic or neutral capping ligands can be rapidly cleared by the kidney.163 However, smaller size TQDs may have the potential danger of passing through important barriers in the body, such as the blood–brain barrier.164 In some cases, different types of QDs may show similar toxic effects that are related to the size.165 TQDs have shown obvious toxic effects on various cells and plant growth. However, in vitro and in vivo toxicity studies sometimes show controversial results,166,167 due to the fact that the toxic effects of TQDs depend on many factors, such as composition, concentration, size, surface modification, and biological systems they interact with and the interaction time. In the in vivo environment, TQDs are not directly exposed to target cells, but rather go through considerably complex processes in different transportation systems within the body. The exact mechanism of how TQDs interact with the body is still unclear, especially their distribution, metabolism and excretion mechanisms. In the cases where toxicity plays an important role, careful and long-term systematic studies are needed to demonstrate the intrinsic advantages of TQDs.102,159,162,168
6.2 CDots
CDots with strong PL and good biocompatibility has become an emerging class of promising fluorescent probes for bioimaging and biosensing.19,41,169–171 Although the toxic heavy metals in TQDs result in a natural disadvantage in biology, related biomedical studies provide useful guidelines for other nanomaterials.102,159 Systematic comparison of CDots with TQDs is of great interest for further development of their biological applications (Fig. 6).31 Moreover, CDots show great potential in emerging new areas, such as biochips, food science and agriculture, beyond that of TQDs.172–175In vitro cell imaging was one of the most popular topics in early CDots research,16 but increasing attention has turned to in vivo bioimaging, drug delivery, cancer therapy and antibacterial application soon after.125,171,176–179 Based on their structural diversity, CDots have also been used to regulate enzyme activity, cell membrane permeability, and gene expression. For example, CDots with special functional groups have been customized for use as PL probes for cancer cells and bacteria. CDots have also been chemically complexed or intramolecularly interacted with anticancer drugs to achieve high-loading drug delivery to improve the treatment effect. Some CDots show antibacterial activity in membranes due to the formation of reactive oxygen species.180–182 Different CDots have been developed to modulate the catalytic activity of enzymes, including laccase and porcine pancreatic lipase.183–187 In combination with phosphate functionalized CDots, the laccase activity increased by 47% in the dark due to enhanced binding capacity of the substrate and by 92% under illumination due to accelerated electron transfer.188
For biosensing, in addition to the fluorescence on/off methods, PL peak shift, lifetime variation, ratio sensing and dual-mode detection have also been explored in CDot-based sensing systems.41,55,59,170,171,174,175,189 CDot-based high-performance laboratory chip devices hold great potential for critical sensing/detection fields, especially the early detection of diseases. Besides these overlapping areas with TQDs, recently CDots have been explored in emerging new areas, showing great promise. Hydroxyl-based CDots were used as a matrix to enable matrix-assisted laser desorption ionization mass spectrometry (MALDI MS) for quick analysis of small biomolecules with high sensitivity.45 Moreover, one of the most promising applications of CDots might be in agriculture due to their high biocompatibility and low cost (Fig. 6).23,172,190,191 Recent studies have shown the permeability of CDots in different plants and their beneficial effects on plant growth with proven biosafety.173,186,192 Retrieving the long history of slash-and-burn produced plant ash in agriculture, the effects of ash on plant growth should include the key functions of the fire-induced carbon nanoparticles (CDots could be a useful model here) that are involved in the life cycle of the entire plant.172,186,192 Along this line, the effects of CDots on plants in recent studies can be summarized as follows: (1) accelerating the germination and root elongation process by increasing the water absorption capacity of the seeds with various hydrophilic functional groups; (2) improving the disease resistance via enhancing the expression of the thiopurine (Os06g32600) gene by inserting a DNA groove; (3) promoting plant growth by degrading phytohormone analogs to CO2 (further converted to carbohydrates by the Calvin cycle of photosynthesis).191 More importantly, research on the agriculture application of CDots has a Janus nature, focusing on both the past and the future. The CDot-initiated photocatalytic protein formation from amino acids may bring an alternative view for the chemical origin of life,193 whereas the revelation of old puzzles in wildfire and slash-and-burn agriculture may open a new avenue for the application of CDots and biosafety of carbon nanomaterials in modern agriculture.172,194
Low toxicity and good biocompatibility of CDots are their greatest advantages for successful biological applications, but the research is far from clear and sufficiently profound.171,177,179,195–197 For example, most CDots have low toxicity, but several CDots are highly toxic.194 Most CDots exhibit low toxicity at low concentrations (e.g., 10 mg ml−1), mainly from the carbon core, and in that stage, can be either degraded or directly cleared by the system. However, increasing concentrations may enhance cytotoxicity and impair organ development.198 In some cases of external stimuli, toxicity of CDots was significantly increased. For example, CDots prepared by hydrothermal treatment of graphite are non-toxic in the absence of light, but exhibit phototoxicity due to the generation of reactive oxygen species under light irradiation.199 Here, the formation of the reactive oxygen species can be suppressed by surface chemical modification. It should be noted that the widely claimed non-toxicity of CDots is based on a simple cognition that the carbon element of the core is nontoxic, while ignoring the surface containing a large number of bioactive organic groups.59,171,197,200 We should also keep in mind that, in fact, the ideal structure model of the CDot core is not far from polycyclic aromatic hydrocarbons, a notorious family of carcinogens.63,201 Therefore, more detailed and in-depth research is necessary before declaring the low toxicity of CDots.171,177,179,195–197 Overall, the number of studies on biological applications of CDots are huge, but it still lacks in-depth and comprehensive works, which may restrain further development of the entire field. CDots still need to delve deeper into their own structural and property advantages, and subsequently find their own way to go beyond TQDs.31,172
7. Device applications
7.1 TQDs
TQDs have been widely used in various electronic devices, including transistors, solar cells, LEDs and photodetectors (Fig. 7 and 8A).9 TQDs with a layer of capping ligands can be used as “QD inks” to form thin film devices by low-cost, room temperature, large area, solution processing methods, such as spin coating and printing, rather than expensive vacuum, high temperature device manufacturing processes. This provides the opportunity to integrate inorganic semiconductors with other organic materials into high performance flexible devices.9,107,202 The size, shape, and heterostructure design of TQDs also provide powerful regulation tools for the electronic properties not found in conventional bulk semiconductors.6,9,107 For example, type-I core/shell TQDs are beneficial for achieving high PL quantum yield required for efficient electroluminescence, while type-II heterostructures can provide efficient charge separation beneficial to photovoltaic and photodetection devices (Fig. 4B).203 In addition, the multiple exciton generation process (MEG) in a single TQD can increase the number of photogenerated charge carriers, which is of great significance for photovoltaics and photodetectors that aim to boost the photoelectric conversion efficiency beyond theoretical limits.113,204 However, the strong interaction of multiple excitons or multiple charge carriers may cause particle charging and PL blinking that severely hinder the efficiency of optoelectronic devices.115 In addition to the structure and property tuning of individual TQDs, device applications also need to consider the preparation of perfect QD solids,205,206 where surface/interface modification, band alignment, charge transportation, carrier migration, exciton diffusion and heat transfer between TQDs all need to be well controlled.9 Typical natural ligands with long hydrocarbon chains (C8–C18) do not allow for efficient charge transfer and are generally disadvantageous for TQD-based devices.46,207,208 The exchange of these insulating long-chain ligands with small molecules or specially designed conductive materials is a critical step.209 For example, mobility is one of the most important parameters for TQD devices, where high mobility (>10 cm2 V−1 s−1) can be achieved by a combination of tight ligand exchange, surface passivation and appropriate doping.210 Novel inorganic ligands, such as metal chalcogenide anions, can serve as molecular “solders” for the conversion of these TQDs to thin films with ultra-high mobility (>400 cm2 V−1 s−1).211 Moreover, TQDs offer advantages over organic and polymeric materials because their PL can be extended into the NIR region.49,50 Other frontier studies of TQD devices include increased light extraction efficiency through photon and plasmonic structures and refractive index control via TQD matrix engineering.6
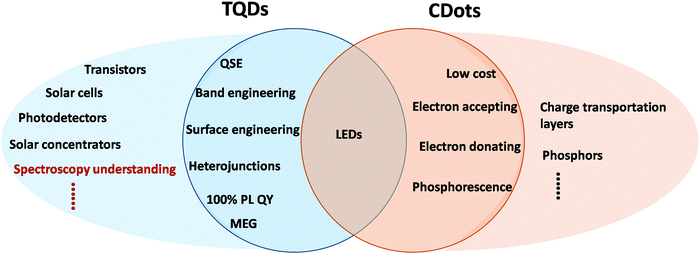 |
| Fig. 7 Schematic comparison of device applications of TQDs and CDots. QSE: quantum size effect, MEG: multiple exciton generation. | |
The field of TQD electroluminescent (EL) devices has been progressing rapidly since 1994,212 with a recent 20.5% external quantum efficiency (EQE) record.213 Another important goal is to enable the EL devices to go beyond display applications into the lighting and laser fields. Single particle spectroscopy indicates that the FWHM of the PL peak of TQDs can reach approximately 20 nm (Fig. 4B).105,106 This unique characteristic along with high PL quantum yield is extremely important in extended color gamut display applications. Another advantage of TQDs over organic molecules is that there is no inherent limitation of the maximum EL efficiency imposed by the spin selection law.214–216 Currently, high-performance TQD-based LEDs are usually combined with hybrid charge transporting layers, such as an inorganic electron transporting layer and an organic hole transporting layer.6,217 This kind of device structure provides high efficiency and brightness comparable with the state-of-the-art organic LEDs. However, considerable obstacles have been encountered in the development of device structures compatible with TQDs especially that can allow for the injection of electrons or holes in a wide range of operating currents.9 To improve the EQE of LEDs, the main factors include non-radiative recombination caused by defects and internal energy conversion within the luminescent layer of TQDs (radiative attenuation), and Auger recombination of the charged TQDs (Fig. 8B).6,9 Along this line, a critical issue of TQDs is the spectroscopic understanding of the devices in terms of inquiry mechanism, problem induction, application-guided synthesis and post-processing strategies.6
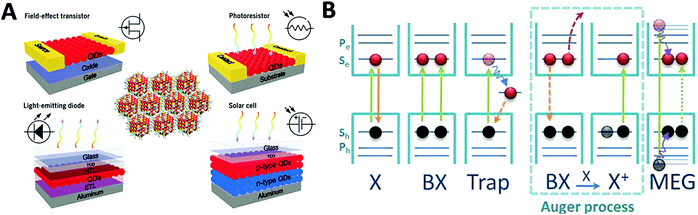 |
| Fig. 8 Schematic representation of various devices using TQDs (A) and the spectroscopic understanding of charge separation and recombination processes involving trapping, particle charging, Auger recombination, and MEG that are crucial in the devices (B). X: exciton, BX: biexciton, MEG: multiple exciton generation. Adapted with permission from ref. 9, copyright 2016, the American Association for the Advancement of Science. | |
For photovoltaics, TQDs have the advantages of high optical absorption efficiency, unique band gap engineering, hot electron extraction and the MEG effect, which give a theoretical power conversion efficiency of up to 44% in a single-junction device, able to break through the traditional Shockley–Queisser limit of 31%.6,218,219 Compared to conventional silicon and organic photovoltaic materials, TQD solids, such as PbS and PbSe, are particularly well suited for efficient capturing of photons in the near and mid infrared regions.220,221 All inorganic thin film solar cells made from TQDs can avoid the use of unstable organic components, while maintaining the low-cost advantages of solution-based methods for device construction. From the first demonstration in 2005 to the recently certified power conversion efficiency of over 16%, TQD solar cells have experienced a quick development involving combination of TQDs with other materials, the construction of solid films to overcome single-junction efficiency limitation, as well as the advanced concepts based on the unique quantum size effect.6,9,219
7.2 CDots
TQDs have been widely used in various optoelectronic devices (Fig. 8A), especially in displays and lighting, which is also the first commercialization field of TQDs with valuable experiences worth learning by CDots.9 CDots have been widely explored as candidates for various solution-processed optoelectronic devices (Fig. 7),222–224 but mainly focused on photoluminescent and electroluminescent LEDs. In early studies, many CDots were used as low-cost phosphors for photoluminescent LEDs, which still occupies a large part of CDots research today.137,224,225 It usually consists of a UV or blue emitting GaN LED chip and color-converting phosphor coating made of CDot composites. In order to avoid solid state annihilation of PL, it is recommended that the CDots should be surface modified and dispersed in the matrix before being used for LEDs.40,116,226,227 Recently, EL LEDs consisting of a CDot luminescent layer sandwiched between an organic hole transporting layer and an organic or inorganic electron transporting layer exhibit switchable EL color by varying the applied voltage. This voltage-dependent EL behavior may be due to multiple recombination pathways of different excited states coexisting in each CDot. Besides working as the active layer, CDots have also been used as a dopant in organic LEDs showing high EQE, even comparable to TQD-based LEDs. However, CDot-based LEDs typically provide wide EL peaks with FWHM over 80 nm, limiting their use in high performance devices. Recently, narrow-bandwidth (FWHM of 30 nm) CDots with multi-color PL were synthesized by a simple hydrothermal method with high quantum yield.40 The multi-color LEDs (from blue to red) based on these CDots show high color purity and high performance with a brightness (Lmax) of 4762 cd m−2 and current efficiency of 5.11 cd A−1. CDots with high PL quantum yield and narrow bandwidth will play an important role in next generation displays with wide color gamut, which still has a long way to go in terms of structure and property control comparable to TQDs. For solar cells, CDots with a high specific surface area, high mobility and adjustable bandgap are promising as electron or hole accepting materials.223,225,228–230 CDots have been successfully used as a charge transporting material or to modify the active layer, showing great potential for the photovoltaic performance enhancement.222
For CDots, the absence of toxic elements is one of the greatest advantages for large-scale application in devices. However, studies of other key electronic parameters of CDot devices are still lacking.6,9 For example, 100% PL quantum yields may be of particular importance for LEDs and solar concentrating panels because photon intensity decays exponentially with thickness,6,231 which has not been achieved for CDots. Another example is the pursuit of ultra-high mobility that are crucial for lots of high-performance devices.211 Improvement of mobility may rely on the profound understanding and simultaneous manipulation of the carbon core and the surface of CDots, which may be correlated with graphene.232,233 It also requires continuous advances in the synthetic procedure and the control of the charge transportation, energy and heat transfer for specific device applications. For device applications, CDots are often used directly as interfacial layers, and the interface engineering between the remaining TQDs or other active layers or device components is still in its infancy, which is important for optimizing device performance.6,9,210,222,223
8. Catalysis applications
8.1 TQDs
Photocatalysis is one of the most promising processes for solar energy conversion and storage and has shown great potential in photocatalytic hydrogen production, CO2 reduction and environmental remediation. The photocatalytic reaction mainly includes three important processes, e.g. light harvesting, charge generation and separation and surface redox reactions of the photogenerated electrons and holes, as shown in the middle of Fig. 9. TQDs are expected to meet the requirements of high light absorption, long-lived charge separation and efficient catalysis in artificial photosynthetic systems due to the property tuning capability through size, shape and composition. Also, TQDs exhibit the advantages of apparent homogeneous catalysis (excellent dispersibility, activity and selectivity) combined with heterogeneous catalysis (easy separation). These characteristics make TQDs and their composites uniquely attractive in photocatalytic applications and have attracted widespread attention.235,237,240–243 The multifunctional heterojunctions of TQDs can be used to efficiently separate photogenerated electron–hole pairs, to harvest plasmonic hot electrons237,244 and to catalyze the redox reactions.234,245
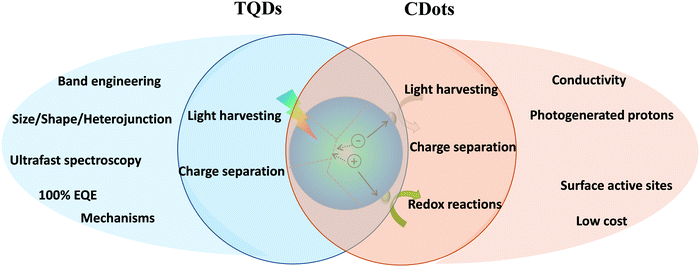 |
| Fig. 9 Schematic comparison of photocatalytic applications of TQDs and CDots. | |
TQD-based heterojunctions with well-defined domains and compositions play a crucial role in the mechanistic study of semiconductor photocatalysis (Fig. 10A).234 Transient absorption and time-resolved PL studies have provided valuable information on the temporal variation of charge carrier decay characteristics in photocatalytic systems (Fig. 10B).235,246–248 For example, photogenerated electron injection into the Pt tip in CdS/Pt nanorods has been shown to occur within 3–4 ps. This process is facilitated by the capture of photogenerated holes on the CdS surface (τ ≈ 1 ps), which prevents the transfer of excitons to the Pt particles. Therefore, the holes on CdS and the electrons on Pt form a catalytically active charge separation state, and their lifetime exceeds 1 μs. However, if the photogenerated holes cannot be captured quickly (τ > 4 ps), the excitons will be able to diffuse to the Pt tip, resulting in the recombination of the electrons and holes and subsequently decrease of the catalytic activity.249 To reduce exciton quenching, pristine CdS nanorods can be replaced with type-II heterojunctions to improve separation of photogenerated charge carriers by promoting the confinement of holes in ZnSe or CdSe, while ZnSe or CdSe has a clear spatial separation from the Pt tip separated by CdS in a “dot@rod” structure (Fig. 10A). Such an arrangement allows the generated charge-separated excitons to be confined near p-type QDs (ZnSe or CdSe) and they do not diffuse as easily into the Pt tip as that in CdS/Pt. This phenomenon has been demonstrated in other studies by ultrafast spectroscopy of CdSe/CdS and ZnSe/CdS nanorods. Introducing a hole confinement domain (ZnSe or CdSe) can reduce the surface hole trapping rate, which helps to suppress the surface-defect-mediated charge recombination.250,251 Moreover, the unique advantage of the “dot@rod” catalyst is its ability to drive efficient separation of photogenerated charge carriers employing electron/hole dissociation and random electron hopping (Fig. 10C–E).236,237 Ideally, however, both the electron-rich and hole-rich components of the TQD-based catalysts should be exposed to better use the absorbed energy via ligand or cocatalyst-mediated charge transfer. Such a morphology can be achieved by etching the CdS layer outside the CdSe/CdS “dot@rod” structure, in which the hole-rich and electron-rich components will be both in direct contact with the external environment.252
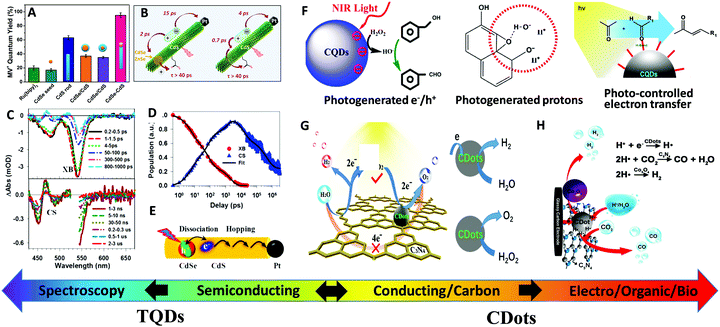 |
| Fig. 10 Catalytic applications of TQDs and CDots. (A) Photocatalytic activity of various TQD structures with different charge separation arrangements for the quantum yield of photogenerated MV+˙ (methyl viologen) radicals. Reprinted with permission from ref. 234, Copyright 2012, American Chemical Society. (B) Photogenerated charge carrier decay dynamics in the CdSe@CdS–Pt dot@rod (left) and CdS/Pt nanorods (right) obtained from transient absorption measurements. Reprinted with permission from ref. 235, Copyright 2015, American Chemical Society. (C) Representative transient absorption spectra of CdSe@CdS–Pt nanorods upon excitation of the CdSe core. (D) Kinetics of exciton bleach (XB) and charge separated states (CS) from 1 to 3000 ns. Reprinted with permission from ref. 236, Copyright 2014, American Chemical Society. (E) Plausible mechanism for the long-range charge transfer in CdSe@CdS–Pt NRs, including electron/hole dissociation and random electron hopping. Reprinted with permission from ref. 237, Copyright 2016, The Royal Society of Chemistry. (F) Schematic representation of versatile functions of CDots for catalysis: photogenerated e−/h+, photogenerated protons and photo-controlled electron transfer. (G) Schematic mechanism of the two-step 2e−–2e− photocatalytic water splitting process in the g-C3N4/CDots system. Adapted with permission from ref. 238, Copyright 2015, the American Association for the Advancement of Science. (H) Schematic three-component electrocatalyst design for syngas production by coupling the HER catalyst (Co3O4) with the CO2 reduction catalyst (CDots-C3N4), employing the adsorption capacity of CDots for CO2 molecules and protons. Reprinted with permission from ref. 239, Copyright 2017, Nature Publishing Group. | |
It has been reported that increasing the hole transfer rate is an important factor in increasing the photocatalytic activity, which can be achieved by finding better hole extracting agents and TQD structural design. A sacrificial agent with a more negative redox potential as electron donors (hole acceptors) can provide a larger hole extraction driving force, resulting in a higher hole transfer rate. For example, the use of S2−/SO32− as a sacrificial agent can result in CdS hydrogen production efficiencies exceeding 50%.253–257 Under this guidance, another way to increase the rate of hole transportation is to utilize a hole extracting agent that is more accessible to the surface of the photocatalysts, as the recently reported redox shuttle mechanism in the CdS nanorods/Ni system at high pH.258 The photogenerated holes oxidize small hydroxides (OH−) to form hydroxyl radicals (OH˙) without direct oxidation of the ethanol molecule, mainly because the hydroxide is small in size and can easily diffuse into the holes on surface of the catalyst. Subsequently, OH˙ is shuttled and diffused into the solution to rapidly oxidize the ethanol molecules to acetaldehyde. Along this line, the OH−/OH˙ redox shuttle strategy was further applied to the CdSe@CdS–Pt nanorod system with a near-unity quantum efficiency at 420 nm.259 All these exquisite nanostructures may not represent the most efficient photocatalysts, but bring a revolutionary profound understanding of catalytic mechanisms and catalyst design principles.
8.2 CDots
CDots combine the advantages of QDs and carbon materials in catalysis, which are actually superior to and more widely used than TQDs.20,139,238,260 For comparison, TQDs were mainly investigated focusing on light harvesting via bandgap engineering and charge separation via heterojunction design, whereas CDots can be used as multifunctional components (light absorber/sensitizer, charge transfer mediator and cocatalyst) for high-performance photocatalysts and other catalysts (Fig. 9).239,261,262 For photocatalysis, CDots have demonstrated versatile functions including improved light absorption, charge separation and surface co-catalysis effect, which have shown great potential in the fields of photocatalytic environmental remediation, hydrogen production and carbon dioxide reduction. Following the pioneering work of photocatalyst design in 2010, research in CDots' catalytic applications has grown rapidly.20 Unique structural advantages make CDots able to provide not only photogenerated e−/h+, but also photogenerated protons and photo-controlled electron transfer under illumination (Fig. 10F), which endow them with great potential in various catalytic reactions beyond photocatalysis.263–265 Moreover, CDots are excellent electron acceptors and donors,266 which can be used as critical components for efficient photocatalysts acting as charge separation mediators. The photocatalytic properties of CDots are determined by structure and interfacial interactions that can be optimized by size, heteroatom doping, and surface functional groups.140,267–270 It has been reported that nitrogen doping reduces the work function of CDots. Hydrogen bonding on the surface of CDots has been shown to cause the upward band bending, which is beneficial to promote the electron transfer and photocatalytic performance. In particular, nitrogen-doped CDots with oxygen-containing functional groups have been successfully prepared as effective photocatalysts for water splitting under visible light, in which the fine p–n junction is composed of a nitrogen-doped core (as an n-domain) and an oxygen-rich edge (as a p-domain) within one dot.140 CDots also showed great potential forming efficient composites to enhance the photocatalytic or other photoelectrochemical performance of traditional semiconductors.139,238,260,271–273 For example, CDots can act as photosensitizers for TiO2, or promote electron–hole separation mediated by electron or energy transfer mechanisms.20,271,274 In these cases, interfacial interactions, such as covalent bonds and hydrogen bonds, are crucial for charge separation and utilization because of the strong influence on charge carrier dynamics. Besides working as photo-active components, CDots have also shown great potential in manipulation of the fundamental reaction pathways for water splitting.139,238,260,275,276 Metal-free CDot-based photocatalysts have been developed to manipulate the one-step four-electron or two-step two-electron pathways throughout the photocatalytic overall water splitting (Fig. 10G).238,275 For PEC water splitting, CDots were deposited on various oxide photoanodes for water oxidation to improve the charge transfer kinetics and provide synergistic cocatalytic effects.277–280 CDots have also been used in the photochemical reduction of Ag+ to Ag0, selective photooxidation of benzyl alcohol to benzaldehyde,264,281 and photocatalytic CO2 reduction.275,282 Moreover, the photogenerated protons allow CDots to be used in a variety of acid catalyzed reactions, including Beckmann rearrangement, esterification and aldol condensation.283 Hydroxyl-functionalized CDots can enhance hydrogen bonding effects through the electron acceptor property of CDots, which were used in aldehyde–alcohol condensation reactions with high conversion efficiency.264
In addition to the successful application in photocatalysis, CDot based materials have become competing candidates for electrocatalysis due to their high stability and electrical conductivity. CDots show excellent application prospects in the fields of electrocatalytic hydrogen evolution reaction (HER), oxygen evolution reaction (OER), oxygen reduction reaction (ORR), CO2 reduction reaction, etc.21,23,172,284 The surface modification of pure CDots may be a promising versatile design strategy for various electrocatalytic applications.262,285 Regarding one of the pioneering reports, the electrochemically prepared N-CDots with oxygen-rich functional groups have shown great electrocatalytic ORR activity.286 Specifically, phosphonate-modified CDots show ORR activity close to Pt/C, while NH2-terminated CDots show a slightly better OER activity than IrO2/C.285 The subsequent work showed that CDot-based ORR model catalysts could be promising metal-free carbon catalysts, superior to 20% Pt/C under alkaline conditions. Design and preparation of composite electrocatalysts have also attracted lots of attention by coupling CDots with NiFe layered double hydroxide (NiFe-LDH), MoS2, ruthenium nanoparticles and so on, showing a great synergistic effect.287–291 For CO2 reduction, a new three-component catalyst design concept was proposed for the tunable and efficient production of syngas (H2 and CO), coupling the HER catalyst (Co3O4) with the CO2 reduction catalyst (CDots–C3N4).239 The enhanced activity is mainly attributed to the unique adsorption capacity of CDots for both CO2 molecules and protons, whereas C3N4 and Co3O4 are the active sites of CO2 reduction and HER, respectively (Fig. 10H). CDots are the sites for active hydrogen production required to initiate the CO2 reduction to CO as well as the HER processes.
As mentioned above, TQDs represent one of the most promising photocatalytic systems for solar fuels, which rely on the bandgap manipulation for effective light absorption, as well as high-quality semiconductor/catalyst interfaces to facilitate charge separation and utilization.235,237,240,244 The near-unity EQE of the TQD-based photocatalysts is still not the final meaning of these studies,258,259 whereas the fundamental value is more likely to provide a deep understanding of traditional catalysts at the nano- and atomic scales, enriching the knowledge of inorganic chemistry, material chemistry and catalytic chemistry to provide useful guidelines for the development of industrial catalysts.237,292 Similarly, the development of CDot-based catalysts does not necessarily lie in high-efficiency catalysts, but is more likely to be a deeper understanding of the surface chemistry and catalytic behavior of carbon particles in terms of model catalysts, in the crossroad of graphene, organic chemistry and biochemistry (Fig. 10).193,238,261,262,293,294 For example, based on their catalytic properties, the introduction of CDots into the polymer matrix has been demonstrated to be a simple and cost-effective method for inducing healing of bulk polymers, that is useful in self-healing and corrosion resistance of polymer coatings. The self-healing is initiated by CDot-catalyzed interfacial amino bonding between the CDots and the polymer matrix, which can be optimized by the surface functional groups of CDots. In addition, recently CDots have been used for the photocatalytic polymerization of amino acids into polypeptides and proteins, even with tertiary structures under very mild conditions, which provides an interesting view for chemical origins of life.193 It can be seen that CDots with a crystalline nature of the core and a functionalized surface are considered to be a promising multifunctional subunit for the design and construction of various hybrid composites and model catalysts.21,23,24 Above all, it is still necessary to further optimize the synthesis of CDots, regulate the types and number of surface functional groups, improve the light-harvesting ability, and optimize and regulate the electron storage and transfer ability.21,23 In addition, a more in-depth understanding of the structure–activity relationship in the CDot-based catalytic systems is inevitable, where the molecular-level mechanisms and interface design should be the key.
9. Challenges and perspectives
In summary, CDots are similar to TQDs in several aspects, but there are a number of significant differences in structure and properties. CDots combine the advantages of the carbon core with a potential application-oriented surface and provide high freedom to manipulate their physicochemical properties, which significantly extends their potential applications beyond TQDs. However, some major challenges still remain for further development of CDots, including precise synthetic control, chemical structure clarification and structure–property relationships. The CDots research presents a typical paradox in terms of the number of publications and the depth of the field compared to that of TQDs.
(1) For basic concepts, we should still be cautious about the “quantum size effect” of CDots, which lacks clear undebated experimental evidence. The spectral shift of CDots does not necessarily represent a true size effect, but may relate to doping or surface groups. In terms of a model system for fundamental research, crystalline CDots with a graphite core appear to be less controversial than amorphous ones, which would be more interesting to dig in from the perspective of “QDs”.
(2) For precise control of synthesis, the reproducible preparation of CDots with uniform size, composition and surface is still challenging, which relies on the profound understanding of the synthetic chemistry, growth mechanism and in situ characterization. What can be learned from the history of TQDs is the emerging of revolutionary procedures (e.g. the organometallic hot-injection method), and a continuous review and improvement of every aspect over decades, including precursors, mechanisms and even mistakes. There is no experimental evidence whether the separated dots are what we want, and whether the beautiful dots in TEM and the colorful PL are from the same subgroup or a mixture of all these particles. Under this consideration, the synthesis of CDots is more likely to be in the “Prehistoric Age” and requires high priority before everything else.
(3) For optical properties, CDots as a new class of luminescent materials show not only size, composition and surface-dependent PL (similar to TQDs), but also show novel excitation-dependent and up-conversion PL, which are not common in TQDs. The optical properties of CDots rely heavily on the synthetic route, and currently there is still no noncontroversial standard sample due to the complex nature of CDots. The exact origin and mechanism of their PL is still controversial, where extensive ultrafast spectroscopy study plays a crucial role, as seen in the history of TQDs. The organic-molecule-like structural characteristic increases the difficulty of CDot synthesis, but gives extra tunability of their optical properties in terms of crossover of inorganic QDs, organic molecules and graphene.
(4) For biological applications, CDots hold great potential due to their low toxicity and good biocompatibility. However, to some extent, the widespread and versatile biomedical studies of CDots have not found the irreplaceable role in this field, comparable to the development of super-resolution imaging by TQDs. It is worth noting that CDots are promising in integrating the advantages of TQDs and small-molecule dyes or drugs. The tight core/surface bonding and small surface groups also brings advantages on biocompatibility in terms of the effective particle size and renal clearance. However, the commonly claimed low toxicity of CDots still needs systematic studies and detailed molecular understanding.
(5) For device applications, the unique core/surface groups structure, versatile optical properties and electron donating/accepting capabilities of CDots provide new opportunities in various devices, but great challenges still exist. Despite their potential beyond TQDs, CDots have only shown limited success in LEDs, in contrast to the booming of TQD devices in all fields. From the history and experience of TQDs, CDots still have a long way to go on the synthesis of perfect CDots, the formation of high-quality films via interfacial engineering, and the profound spectral understanding of performance-limiting factors.
(6) For catalysis applications, TQDs have played a key role in the mechanistic study and photocatalyst design, whereas CDots showed much more versatile use in photocatalysts and electrocatalysts, by providing enhanced light absorption, charge transfer, conductivity and co-catalytic sites. The catalytic performance of CDots can be adjusted by carefully designing the core structure and surface, which are still limited by the lack of precise control and detailed characterization at the atomic level and in situ catalysis kinetics study. Note that three typical catalytic systems (heterogeneous, homogeneous and enzyme catalysis) exhibit similar patterns at the molecular level ultimately, from which we should see that CDots provide a potentially ideal catalyst structural model as the bridge of traditional carbon materials and organic chemistry.
Overall, it is now the time to reconsider the unique characteristics, advantages and disadvantages of CDots from the perspective of TQDs. Understanding CDots from the perspective of TQDs provides guidance at the basic conceptual level. After a comparison with the TQD system, it is expected to deepen the mechanistic studies on synthetic chemistry and optoelectronic properties to promote the development of this field. The rapid development of CDots in the fields of catalysis and biology does not obscure their shortcomings in synthesis and spectral understanding of high-quality samples, which is still the biggest obstacle to their further development. From the experience of TQDs, the close cooperation of in-depth theoretical study, precise synthesis, in situ characterization and spectroscopic understanding of the devices and biosystems together is inevitable to further promote their applications. We should believe and expect the great potential of CDots, and focus on the critical and tough issues for their further development.
Conflicts of interest
The authors have no conflicts to declare.
Acknowledgements
This work was supported by the National Natural Science Foundation of China (21908081, 21501072, 51972216, 51725204, 21771132, and 52041202), the National MCF Energy R&D Program (2018YFE0306105), Innovative Research Group Project of the National Natural Science Foundation of China (51821002), the Jiangsu Specially-Appointed Professors Program, the Natural Science Foundation of Jiangsu Province (BK20190041, BK20190828 and BK20150489), Key-Area Research and Development Program of GuangDong Province (2019B010933001), Collaborative Innovation Center of Suzhou Nano Science & Technology, the Priority Academic Program Development of Jiangsu Higher Education Institutions (PAPD), and the 111 Project.
References
- L. E. Brus, Electron–electron and electron–hole interactions in small semiconductor crystallites: the size dependence of the lowest excited electronic state, J. Chem. Phys., 1984, 80, 4403–4409 CrossRef CAS.
-
S. V. Gaponenko, Optical Properties of Semiconductor Nanoparticles, Cambridge University Press, Cambridge, UK, 1998 Search PubMed.
-
V. I. Klimov, Nanocrystal Quantum Dots, 2nd edn, CRC Press, 2010 Search PubMed.
- A. P. Alivisatos, Semiconductor clusters, nanocrystals, and quantum dots, Science, 1996, 271, 933–937 CrossRef CAS.
- C. Burda, X. Chen, R. Narayanan and M. A. El-Sayed, Chemistry and Properties of Nanocrystals of Different Shapes, Chem. Rev., 2005, 105, 1025–1102 CrossRef CAS PubMed.
- J. M. Pietryga, Y.-S. Park, J. Lim, A. F. Fidler, W. K. Bae, S. Brovelli and V. I. Klimov, Spectroscopic and Device Aspects of Nanocrystal Quantum Dots, Chem. Rev., 2016, 116, 10513–10622 CrossRef CAS PubMed.
- K. D. Wegner and N. Hildebrandt, Quantum dots: bright and versatile in vitro and in vivo fluorescence imaging biosensors, Chem. Soc. Rev., 2015, 44, 4792–4834 RSC.
- P. D. Howes, R. Chandrawati and M. M. Stevens, Colloidal nanoparticles as advanced biological sensors, Science, 2014, 346, 1247390 CrossRef PubMed.
- C. R. Kagan, E. Lifshitz, E. H. Sargent and D. V. Talapin, Building devices from colloidal quantum dots, Science, 2016, 353, aac5523 CrossRef PubMed.
- S. Y. Lim, W. Shen and Z. Gao, Carbon quantum dots and their applications, Chem. Soc. Rev., 2015, 44, 362–381 RSC.
- M. Nasilowski, B. Mahler, E. Lhuillier, S. Ithurria and B. Dubertret, Two-Dimensional Colloidal Nanocrystals, Chem. Rev., 2016, 116, 10934–10982 CrossRef CAS PubMed.
- X. Wang, G. Sun, N. Li and P. Chen, Quantum dots derived from two-dimensional materials and their applications for catalysis and energy, Chem. Soc. Rev., 2016, 45, 2239–2262 RSC.
- X. Y. Xu, R. Ray, Y. L. Gu, H. J. Ploehn, L. Gearheart, K. Raker and W. A. Scrivens, Electrophoretic analysis and purification of fluorescent single-walled carbon nanotube fragments, J. Am. Chem. Soc., 2004, 126, 12736–12737 CrossRef CAS PubMed.
- Actually, in 2002, Mao and Kang's advisors have proposed the quasi-QDs emission behavior in their discussion of the fluorescence properties of graphite nanofragments fabricated from electrochemical etching of graphite rods, during their graduate studies. Unfortunately, further in-depth study was unable to finish at that time. Here we would like to acknowledge Profs. Enbo Wang and Lin Xu for their pristine but profound discussion a decade ago that brought us a career in CDots research today.
- Y.-P. Sun, B. Zhou, Y. Lin, W. Wang, K. A. S. Fernando, P. Pathak, M. J. Meziani, B. A. Harruff, X. Wang, H. Wang, P. G. Luo, H. Yang, M. E. Kose, B. Chen, L. M. Veca and S.-Y. Xie, Quantum-sized carbon dots for bright and colorful photoluminescence, J. Am. Chem. Soc., 2006, 128, 7756–7757 CrossRef CAS PubMed.
- L. Cao, X. Wang, M. J. Meziani, F. Lu, H. Wang, P. G. Luo, Y. Lin, B. A. Harruff, L. M. Veca, D. Murray, S.-Y. Xie and Y.-P. Sun, Carbon dots for multiphoton bioimaging, J. Am. Chem. Soc., 2007, 129, 11318–11319 CrossRef CAS PubMed.
- J. Zhou, C. Booker, R. Li, X. Zhou, T.-K. Sham, X. Sun and Z. Ding, An electrochemical avenue to blue luminescent nanocrystals from multiwalled carbon nanotubes (MWCNTs), J. Am. Chem. Soc., 2007, 129, 744–745 CrossRef CAS PubMed.
- A. B. Bourlinos, A. Stassinopoulos, D. Anglos, R. Zboril, V. Georgakilas and E. P. Giannelis, Photoluminescent carbogenic dots, Chem. Mater., 2008, 20, 4539–4541 CrossRef CAS.
- S. N. Baker and G. A. Baker, Luminescent carbon nanodots: emergent nanolights, Angew. Chem., Int. Ed., 2010, 49, 6726–6744 CrossRef CAS PubMed.
- H. Li, X. He, Z. Kang, H. Huang, Y. Liu, J. Liu, S. Lian, C. H. A. Tsang, X. Yang and S.-T. Lee, Water-Soluble Fluorescent Carbon Quantum Dots and Photocatalyst Design, Angew. Chem., Int. Ed., 2010, 49, 4430–4434 CrossRef CAS PubMed.
- C. Hu, M. Li, J. Qiu and Y. P. Sun, Design and fabrication of carbon dots for energy conversion and storage, Chem. Soc. Rev., 2019, 48, 2315–2337 RSC.
- F. Arcudi, L. Dordevic and M. Prato, Design, Synthesis, and Functionalization Strategies of Tailored Carbon Nanodots, Acc. Chem. Res., 2019, 52, 2070–2079 CrossRef CAS PubMed.
- B. Yao, H. Huang, Y. Liu and Z. Kang, Carbon Dots: A Small Conundrum, Trends Chem., 2019, 1, 235–246 CrossRef.
- N. Martin and G. Bodwell, Advanced Molecular Nanocarbons: Fertile Ground for Discovery, Creation, and Invention, Acc. Chem. Res., 2019, 52, 2757–2759 CrossRef CAS PubMed.
- C. B. Murray, D. J. Norris and M. G. Bawendi, Synthesis and characterization of nearly monodisperse CdE (E = sulfur, selenium, tellurium) semiconductor nanocrystallites, J. Am. Chem. Soc., 1993, 115, 8706–8715 CrossRef CAS.
- Z. A. Peng and X. G. Peng, Formation of high-quality CdTe, CdSe, and CdS nanocrystals using CdO as precursor, J. Am. Chem. Soc., 2001, 123, 183–184 CrossRef CAS PubMed.
- J. Owen, The coordination chemistry of nanocrystal surfaces, Science, 2015, 347, 615–616 CrossRef CAS PubMed.
- K. L. Sowers, B. Swartz and T. D. Krauss, Chemical Mechanisms of Semiconductor Nanocrystal Synthesis, Chem. Mater., 2013, 25, 1351–1362 CrossRef CAS.
- P. Reiss, M. Carrière, C. Lincheneau, L. Vaure and S. Tamang, Synthesis of Semiconductor Nanocrystals, Focusing on Nontoxic and Earth-Abundant Materials, Chem. Rev., 2016, 116, 10731–10819 CrossRef CAS PubMed.
- A. Cayuela, M. L. Soriano, C. Carrillo-Carrion and M. Valcarcel, Semiconductor and carbon-based fluorescent nanodots: the need for consistency, Chem. Commun., 2016, 52, 1311–1326 RSC.
- A. Rakovich and T. Rakovich, Semiconductor versus graphene quantum dots as fluorescent probes for cancer diagnosis and therapy applications, J. Mater. Chem. B, 2018, 6, 2690–2712 RSC.
- R. Rossetti, S. Nakahara and L. E. Brus, Quantum size effects in the redox potentials, resonance Raman spectra, and electronic spectra of CdS crystallites in aqueous solution, J. Chem. Phys., 1983, 79, 1086 CrossRef CAS.
- L. Brus, A simple model for the ionization potential, electron affinity, and aqueous redox potentials of small semiconductor crystallites, J. Chem. Phys., 1983, 79, 5566–5571 CrossRef CAS.
- M. Bruchez, M. Moronne, P. Gin, S. Weiss and A. P. Alivisatos, Semiconductor Nanocrystals as Fluorescent Biological Labels, Science, 1998, 281, 2013–2016 CrossRef CAS PubMed.
- W. C. W. Chan and S. Nie, Quantum Dot Bioconjugates for Ultrasensitive Nonisotopic Detection, Science, 1998, 281, 2016–2018 CrossRef CAS PubMed.
- A. Kelarakis, Graphene quantum dots: In the crossroad of graphene, quantum dots and carbogenic nanoparticles, Curr. Opin. Colloid Interface Sci., 2015, 20, 354–361 CrossRef CAS.
- C. Xia, S. Zhu, T. Feng, M. Yang and B. Yang, Evolution and Synthesis of Carbon Dots: From Carbon Dots to Carbonized Polymer Dots, Adv. Sci., 2019, 6, 1901316 CrossRef CAS PubMed.
- S. Bhattacharyya, F. Ehrat, P. Urban, R. Teves, R. Wyrwich, M. Doblinger, J. Feldmann, A. S. Urban and J. K. Stolarczyk, Effect of nitrogen atom positioning on the trade-off between emissive and photocatalytic properties of carbon dots, Nat. Commun., 2017, 8, 1401 CrossRef PubMed.
- L. Dordevic, F. Arcudi, A. D'Urso, M. Cacioppo, N. Micali, T. Buergi, R. Purrello and M. Prato, Design principles of chiral carbon nanodots help convey chirality from molecular to nanoscale level, Nat. Commun., 2018, 9, 3442 CrossRef PubMed.
- F. Yuan, T. Yuan, L. Sui, Z. Wang, Z. Xi, Y. Li, X. Li, L. Fan, Z. A. Tan, A. Chen, M. Jin and S. Yang, Engineering triangular carbon quantum dots with unprecedented narrow bandwidth emission for multicolored LEDs, Nat. Commun., 2018, 9, 2249 CrossRef PubMed.
- S. Y. Lim, W. Shen and Z. Gao, Carbon quantum dots and their applications, Chem. Soc. Rev., 2015, 44, 362–381 RSC.
- Z. Gan, H. Xu and Y. Hao, Mechanism for excitation-dependent photoluminescence from graphene quantum dots and other graphene oxide derivates: consensus, debates and challenges, Nanoscale, 2016, 8, 7794–7807 RSC.
- Q. Xu, T. Kuang, Y. Liu, L. Cai, X. Peng, T. Sreenivasan Sreeprasad, P. Zhao, Z. Yu and N. Li, Heteroatom-doped carbon dots: synthesis, characterization, properties, photoluminescence mechanism and biological applications, J. Mater. Chem. B, 2016, 4, 7204–7219 RSC.
- E. C. Scher, L. Manna and A. P. Alivisatos, Shape control and applications of nanocrystals, Philos. Trans., 2003, 361, 241–257 CrossRef CAS PubMed.
- R. Shi, X. Dai, W. Li, F. Lu, Y. Liu, H. Qu, H. Li, Q. Chen, H. Tian, E. Wu, Y. Wang, R. Zhou, S.-T. Lee, Y. Lifshitz, Z. Kang and J. Liu, Hydroxyl-Group-Dominated Graphite Dots Reshape Laser Desorption/Ionization Mass Spectrometry for Small Biomolecular Analysis and Imaging, ACS Nano, 2017, 11, 9500–9513 CrossRef CAS PubMed.
- M. A. Boles, D. Ling, T. Hyeon and D. V. Talapin, The surface science of nanocrystals, Nat. Mater., 2016, 15, 364 CrossRef CAS PubMed.
- M. A. El-Sayed, Small Is Different:
Shape-, Size-, and Composition-Dependent Properties of Some Colloidal Semiconductor Nanocrystals, Acc. Chem. Res., 2004, 37, 326–333 CrossRef CAS PubMed.
- X. G. Peng, Band Gap and Composition Engineering on a Nanocrystal (BCEN) in Solution, Acc. Chem. Res., 2010, 43, 1387–1395 CrossRef CAS PubMed.
- M. D. Regulacio and M.-Y. Han, Composition-Tunable Alloyed Semiconductor Nanocrystals, Acc. Chem. Res., 2010, 43, 621–630 CrossRef CAS PubMed.
- A. M. Smith and S. Nie, Semiconductor Nanocrystals: Structure, Properties, and Band Gap Engineering, Acc. Chem. Res., 2010, 43, 190–200 CrossRef CAS PubMed.
- M. Nirmal and L. Brus, Luminescence Photophysics in Semiconductor Nanocrystals, Acc. Chem. Res., 1999, 32, 407–414 CrossRef CAS.
- M. A. Boles, M. Engel and D. V. Talapin, Self-Assembly of Colloidal Nanocrystals: From Intricate Structures to Functional Materials, Chem. Rev., 2016, 116, 11220–11289 CrossRef CAS PubMed.
- H. Zhu, X. Wang, Y. Li, Z. Wang, F. Yang and X. Yang, Microwave synthesis of fluorescent carbon nanoparticles with electrochemiluminescence properties, Chem. Commun., 2009, 5118–5120 RSC.
- H. Li, Z. Kang, Y. Liu and S.-T. Lee, Carbon nanodots: synthesis, properties and applications, J. Mater. Chem., 2012, 22, 24230 RSC.
- J. Peng, W. Gao, B. K. Gupta, Z. Liu, R. Romero-Aburto, L. Ge, L. Song, L. B. Alemany, X. Zhan, G. Gao, S. A. Vithayathil, B. A. Kaipparettu, A. A. Marti, T. Hayashi, J.-J. Zhu and P. M. Ajayan, Graphene Quantum Dots Derived from Carbon Fibers, Nano Lett., 2012, 12, 844–849 CrossRef CAS PubMed.
- Z. Zhang, J. Zhang, N. Chen and L. Qu, Graphene quantum dots: an emerging material for energy-related applications and beyond, Energy Environ. Sci., 2012, 5, 8869 RSC.
- A. B. Bourlinos, A. Stassinopoulos, D. Anglos, R. Zboril, M. Karakassides and E. P. Giannelis, Surface functionalized carbogenic quantum dots, Small, 2008, 4, 455–458 CrossRef CAS PubMed.
- S. Zhu, J. Zhang, S. Tang, C. Qiao, L. Wang, H. Wang, X. Liu, B. Li, Y. Li, W. Yu, X. Wang, H. Sun and B. Yang, Surface Chemistry Routes to Modulate the Photoluminescence of Graphene Quantum Dots: From Fluorescence Mechanism to Up-Conversion Bioimaging Applications, Adv. Funct. Mater., 2012, 22, 4732–4740 CrossRef CAS.
- C. Ding, A. Zhu and Y. Tian, Functional Surface Engineering of C-Dots for Fluorescent Biosensing and in Vivo Bioimaging, Acc. Chem. Res., 2014, 47, 20–30 CrossRef CAS PubMed.
- W. Liu, C. Li, Y. Ren, X. Sun, W. Pan, Y. Li, J. Wang and W. Wang, Carbon dots: surface engineering and applications, J. Mater. Chem. B, 2016, 4, 5772–5788 RSC.
- L. Li and T. Dong, Photoluminescence tuning in carbon dots: surface passivation or/and functionalization, heteroatom doping, J. Mater. Chem. C, 2018, 6, 7944–7970 RSC.
- X.-Y. Wang, X. Yao, A. Narita and K. Muellen, Heteroatom-Doped Nanographenes with Structural Precision, Acc. Chem. Res., 2019, 52, 2491–2505 CrossRef CAS PubMed.
- I. Pozo, E. Guitian, D. Perez and D. Pena, Synthesis of Nanographenes, Starphenes, and Sterically Congested Polyarenes by Aryne Cyclotrimerization, Acc. Chem. Res., 2019, 52, 2472–2481 CrossRef CAS PubMed.
- M. Fu, F. Ehrat, Y. Wang, K. Z. Milowska, C. Reckmeier, A. L. Rogach, J. K. Stolarczyk, A. S. Urban and J. Feldmann, Carbon Dots: A Unique Fluorescent Cocktail of Polycyclic Aromatic Hydrocarbons, Nano Lett., 2015, 15, 6030–6035 CrossRef CAS PubMed.
- X.-Y. Wang, X. Yao and K. Muellen, Polycyclic aromatic hydrocarbons in the graphene era, Sci. China: Chem., 2019, 62, 1099–1144 CrossRef CAS.
- V. Georgakilas, J. A. Perman, J. Tucek and R. Zboril, Broad Family of Carbon Nanoallotropes: Classification, Chemistry, and Applications of Fullerenes, Carbon Dots, Nanotubes, Graphene, Nanodiamonds, and Combined Superstructures, Chem. Rev., 2015, 115, 4744–4822 CrossRef CAS PubMed.
- S. G. Kwon and T. Hyeon, Formation Mechanisms of Uniform Nanocrystals via Hot-Injection and Heat-Up Methods, Small, 2011, 7, 2685–2702 CrossRef CAS PubMed.
- L. Jing, S. V. Kershaw, Y. Li, X. Huang, Y. Li, A. L. Rogach and M. Gao, Aqueous Based Semiconductor Nanocrystals, Chem. Rev., 2016, 116, 10623–10730 CrossRef CAS PubMed.
- L. De Trizio and L. Manna, Forging Colloidal Nanostructures via Cation Exchange Reactions, Chem. Rev., 2016, 116, 10852–10887 CrossRef CAS PubMed.
- H. Reiss, The Growth of Uniform Colloidal Dispersions, J. Chem. Phys., 2004, 19, 482–487 CrossRef.
- X. G. Peng, L. Manna, W. D. Yang, J. Wickham, E. Scher, A. Kadavanich and A. P. Alivisatos, Shape control of CdSe nanocrystals, Nature, 2000, 404, 59–61 CrossRef CAS PubMed.
- V. K. LaMer and R. H. Dinegar, Theory, production and mechanism of formation of monodispersed hydrosols, J. Am. Chem.
Soc., 1950, 72, 4847–4854 CrossRef CAS.
- J. Park, J. Joo, S. G. Kwon, Y. Jang and T. Hyeon, Synthesis of monodisperse spherical nanocrystals, Angew. Chem., Int. Ed., 2007, 46, 4630–4660 CrossRef CAS PubMed.
- W. Ostwald, Studien über die Bildung und Umwandlung fester Körper, Z. Phys. Chem., 2017, 22, 289–330 Search PubMed.
- J. M. Yuk, J. Park, P. Ercius, K. Kim, D. J. Hellebusch, M. F. Crommie, J. Y. Lee, A. Zettl and A. P. Alivisatos, High-Resolution EM of Colloidal Nanocrystal Growth Using Graphene Liquid Cells, Science, 2012, 336, 61–64 CrossRef CAS PubMed.
- T. J. Woehl, J. E. Evans, L. Arslan, W. D. Ristenpart and N. D. Browning, Direct in Situ Determination of the Mechanisms Controlling Nanoparticle Nucleation and Growth, ACS Nano, 2012, 6, 8599–8610 CrossRef CAS PubMed.
- Y. Sun and Y. Ren, In Situ Synchrotron X-Ray Techniques for Real-Time Probing of Colloidal Nanoparticle Synthesis, Part. Part. Syst. Charact., 2013, 30, 399–419 CrossRef CAS.
- Q. Liu, Z. Li, J. S. Okasinski, Y. Ren and Y. Sun, In situ high-energy synchrotron X-ray diffraction revealing precipitation reaction kinetics of silver ions with mixed halide ions, J. Mater. Chem. C, 2015, 3, 7492–7498 RSC.
- L. Zheng, Y. Chi, Y. Dong, J. Lin and B. Wang, Electrochemiluminescence of Water-Soluble Carbon Nanocrystals Released Electrochemically from Graphite, J. Am. Chem. Soc., 2009, 131, 4564–11309 CrossRef CAS PubMed.
- H. Liu, T. Ye and C. Mao, Fluorescent carbon nanoparticles derived from candle soot, Angew. Chem., Int. Ed., 2007, 46, 6473–6475 CrossRef CAS PubMed.
- S. Hu, A. Trinchi, P. Atkin and I. Cole, Tunable Photoluminescence Across the Entire Visible Spectrum from Carbon Dots Excited by White Light, Angew. Chem., Int. Ed., 2015, 54, 2970–2974 CrossRef CAS PubMed.
- F. Li, Y. Li, X. Yang, X. Han, Y. Jiao, T. Wei, D. Yang, H. Xu and G. Nie, Highly Fluorescent Chiral N-S-Doped Carbon Dots from Cysteine: Affecting Cellular Energy Metabolism, Angew. Chem., Int. Ed., 2018, 57, 2377–2382 CrossRef CAS PubMed.
- S. Yang, W. Li, C. Ye, G. Wang, H. Tian, C. Zhu, P. He, G. Ding, X. Xie, Y. Liu, Y. Lifshitz, S.-T. Lee, Z. Kang and M. Jiang, C3N-A 2D Crystalline, Hole-Free, Tunable-Narrow-Bandgap Semiconductor with Ferromagnetic Properties, Adv. Mater., 2017, 29, 1605625 CrossRef PubMed.
- L. Dordevic, F. Arcudi and M. Prato, Preparation, functionalization and characterization of engineered carbon nanodots, Nat. Protoc., 2019, 14, 2931–2953 CrossRef PubMed.
- N. C. Verma, A. Yadav and C. K. Nandi, Paving the path to the future of carbogenic nanodots, Nat. Commun., 2019, 10, 2391 CrossRef PubMed.
- X. Miao, D. Qu, D. Yang, B. Nie, Y. Zhao, H. Fan and Z. Sun, Synthesis of Carbon Dots with Multiple Color Emission by Controlled Graphitization and Surface Functionalization, Adv. Mater., 2018, 30, 1704740 CrossRef PubMed.
- J. Zhang, Y. Yuan, G. Liang and S.-H. Yu, Scale-Up Synthesis of Fragrant Nitrogen-Doped Carbon Dots from Bee Pollens for Bioimaging and Catalysis, Adv. Sci., 2015, 2, 1500002 CrossRef PubMed.
- K. Jiang, Y. Wang, X. Gao, C. Cai and H. Lin, Facile, Quick, and Gram-Scale Synthesis of Ultralong-Lifetime Room-Temperature-Phosphorescent Carbon Dots by Microwave Irradiation, Angew. Chem., Int. Ed., 2018, 57, 6216–6220 CrossRef CAS PubMed.
- H. Ming, Z. Ma, Y. Liu, K. Pan, H. Yu, F. Wang and Z. Kang, Large scale electrochemical synthesis of high quality carbon nanodots and their photocatalytic property, Dalton Trans., 2012, 41, 9526–9531 RSC.
- J. Zhang and S.-H. Yu, Carbon dots: large-scale synthesis, sensing and bioimaging, Mater. Today, 2016, 19, 382–393 CrossRef CAS.
- W. J. Stark, P. R. Stoessel, W. Wohlleben and A. Hafner, Industrial applications of nanoparticles, Chem. Soc. Rev., 2015, 44, 5793–5805 RSC.
- L. Zhang and Y. Xia, Scaling up the Production of Colloidal Nanocrystals: Should We Increase or Decrease the Reaction Volume, Adv. Mater., 2014, 26, 2600–2606 CrossRef CAS PubMed.
- Y. Pu, F. Cai, D. Wang, J.-X. Wang and J.-F. Chen, Colloidal Synthesis of Semiconductor Quantum Dots toward Large Scale Production: A Review, Ind. Eng. Chem. Res., 2018, 57, 1790–1802 CrossRef CAS.
- A. J. Morris-Cohen, M. D. Donakowski, K. E. Knowles and E. A. Weiss, The Effect of a Common Purification Procedure on the Chemical Composition of the Surfaces of CdSe Quantum Dots Synthesized with Trioctylphosphine Oxide, J. Phys. Chem. C, 2010, 114, 897–906 CrossRef CAS.
- A. M. Munro, I. Jen-La Plante, M. S. Ng and D. S. Ginger, Quantitative study of the effects of surface ligand concentration on CdSe nanocrystal photoluminescence, J. Phys. Chem. C, 2007, 111, 6220–6227 CrossRef CAS.
- W. Cao, Y. Qin, H. Huang, B. Mao, Y. Liu and Z. Kang, Extraction of High-Quality Quantum Dot Photocatalysts via Combination of Size Selection and Electrochemiluminescence, ACS Sustainable Chem. Eng., 2019, 7, 20043–20050 CrossRef CAS.
- P. R. Yu, M. C. Beard, R. J. Ellingson, S. Ferrere, C. Curtis, J. Drexler, F. Luiszer and A. J. Nozik, Absorption cross-section and related optical properties of colloidal InAs quantum dots, J. Phys. Chem. B, 2005, 109, 7084–7087 CrossRef CAS PubMed.
- A. Hassinen, I. Moreels, K. De Nolf, P. F. Smet, J. C. Martins and Z. Hens, Short-Chain Alcohols Strip X-Type Ligands and Quench the Luminescence of PbSe and CdSe Quantum Dots, Acetonitrile Does Not, J. Am. Chem. Soc., 2012, 134, 20705–20712 CrossRef CAS PubMed.
- Y. Dong, H. Pang, H. B. Yang, C. Guo, J. Shao, Y. Chi, C. M. Li and T. Yu, Carbon-Based Dots Co-doped with Nitrogen and Sulfur for High Quantum Yield and Excitation-Independent Emission, Angew. Chem., Int. Ed., 2013, 52, 7800–7804 CrossRef CAS PubMed.
- F. Arcudi, L. Dordevic and M. Prato, Synthesis, Separation, and Characterization of Small and Highly Fluorescent Nitrogen-Doped Carbon NanoDots, Angew. Chem., Int. Ed., 2016, 55, 2107–2112 CrossRef CAS PubMed.
- L. Deng, X. Wang, Y. Kuang, C. Wang, L. Luo, F. Wang and X. Sun, Development of hydrophilicity gradient ultracentrifugation method for photoluminescence investigation of separated non-sedimental carbon dots, Nano Res., 2015, 8, 2810–2821 CrossRef CAS.
- J. Zhou, Y. Yang and C.-y. Zhang, Toward Biocompatible Semiconductor Quantum Dots: From Biosynthesis and Bioconjugation to Biomedical Application, Chem. Rev., 2015, 115, 11669–11717 CrossRef CAS PubMed.
- B. Chen, N. Pradhan and H. Zhong, From Large-Scale Synthesis to Lighting Device Applications of Ternary I–III–VI Semiconductor Nanocrystals: Inspiring Greener Material Emitters, J. Phys. Chem. Lett., 2018, 9, 435–445 CrossRef CAS PubMed.
- W. W. Yu, L. H. Qu, W. Z. Guo and X. G. Peng, Experimental determination of the extinction coefficient of CdTe, CdSe, and CdS nanocrystals, Chem. Mater., 2003, 15, 2854–2860 CrossRef CAS.
- J. Zhou, M. Zhu, R. Meng, H. Qin and X. Peng, Ideal CdSe/CdS Core/Shell Nanocrystals Enabled by Entropic Ligands and Their Core Size-, Shell Thickness-, and Ligand-Dependent Photoluminescence Properties, J. Am. Chem. Soc., 2017, 139, 16556–16567 CrossRef CAS PubMed.
- O. Chen, J. Zhao, V. P. Chauhan, J. Cui, C. Wong, D. K. Harris, H. Wei, H.-S. Han, D. Fukumura, R. K. Jain and M. G. Bawendi, Compact high-quality CdSe-CdS core-shell nanocrystals with narrow emission linewidths and suppressed blinking, Nat. Mater., 2013, 12, 445–451 CrossRef CAS PubMed.
- D. V. Talapin, J.-S. Lee, M. V. Kovalenko and E. V. Shevchenko, Prospects of Colloidal Nanocrystals for Electronic and Optoelectronic Applications, Chem. Rev., 2010, 110, 389–458 CrossRef CAS PubMed.
- C.-H. Chuang and C. Burda, Contribution of Femtosecond Laser Spectroscopy to the Development of Advanced Optoelectronic Nanomaterials, J. Phys. Chem. Lett., 2012, 3, 1921–1927 CrossRef CAS PubMed.
- D. A. Wheeler and J. Z. Zhang, Exciton Dynamics in Semiconductor Nanocrystals, Adv. Mater., 2013, 25, 2878–2896 CrossRef CAS PubMed.
- B. Mao, C.-H. Chuang, F. Lu, L. Sang, J. Zhu and C. Burda, Study of the partial Ag-to-Zn cation exchange in AgInS2/ZnS nanocrystals, J. Phys. Chem. C, 2013, 117, 648–656 CrossRef CAS.
- B. Mao, C.-H. Chuang, C. McCleese, J. Zhu and C. Burda, Near-infrared emitting AgInS2/ZnS nanocrystals, J. Phys. Chem. C, 2014, 118, 13883–13889 CrossRef CAS.
- B. Mao, C.-H. Chuang, J. Wang and C. Burda, Synthesis and photophysical properties of ternary I-III-VI AgInS2 nanocrystals: intrinsic versus surface states, J. Phys. Chem. C, 2011, 115, 8945–8954 CrossRef CAS.
- A. J. Nozik, M. C. Beard, J. M. Luther, M. Law, R. J. Ellingson and J. C. Johnson, Semiconductor Quantum Dots and Quantum Dot Arrays and Applications of Multiple Exciton Generation to Third-Generation Photovoltaic Solar Cells, Chem. Rev., 2010, 110, 6873–6890 CrossRef CAS PubMed.
- A. A. Cordones and S. R. Leone, Mechanisms for charge trapping in single semiconductor nanocrystals probed by fluorescence blinking, Chem. Soc. Rev., 2013, 42, 3209–3221 RSC.
- A. L. Efros and D. J. Nesbitt, Origin and control of blinking in quantum dots, Nat. Nanotechnol., 2016, 11, 661–671 CrossRef CAS PubMed.
- S. Y. Song, K. K. Liu, J. Y. Wei, Q. Lou, Y. Shang and C. X. Shan, Deep-Ultraviolet Emissive Carbon Nanodots, Nano Lett., 2019, 19, 5553–5561 CrossRef CAS PubMed.
- K. Hola, Y. Zhang, Y. Wang, E. P. Giannelis, R. Zboril and A. L. Rogach, Carbon dots—Emerging light emitters for bioimaging, cancer therapy and optoelectronics, Nano Today, 2014, 9, 590–603 CrossRef CAS.
- D. Li, P. Jing, L. Sun, Y. An, X. Shan, X. Lu, D. Zhou, D. Han, D. Shen, Y. Zhai, S. Qu, R. Zboril and A. L. Rogach, Near-Infrared Excitation/Emission and Multiphoton-Induced Fluorescence of Carbon Dots, Adv. Mater., 2018, 30, 1705913 CrossRef PubMed.
- K. K. Liu, S. Y. Song, L. Z. Sui, S. X. Wu, P. T. Jing, R. Q. Wang, Q. Y. Li, G. R. Wu, Z. Z. Zhang, K. J. Yuan and C. X. Shan, Efficient Red/Near-Infrared-Emissive Carbon Nanodots with Multiphoton Excited Upconversion Fluorescence, Adv. Sci., 2019, 6, 1900766 CrossRef PubMed.
- Z. Zhu, Y. Zhai, Z. Li, P. Zhu, S. Mao, C. Zhu, D. Du, L. A. Belfiore, J. Tang and Y. Lin, Red carbon dots: optical property regulations and applications, Mater. Today, 2019, 30, 52–79 CrossRef CAS.
- M. Lan, S. Zhao, Z. Zhang, L. Yan, L. Guo, G. Niu, J. Zhang, J. Zhao, H. Zhang, P. Wang, G. Zhu, C.-S. Lee and W. Zhang, Two-photon-excited near-infrared emissive carbon dots as multifunctional agents for fluorescence imaging and photothermal therapy, Nano Res., 2017, 10, 3113–3123 CrossRef CAS.
- K. Jiang, S. Sun, L. Zhang, Y. Lu, A. Wu, C. Cai and H. Lin, Red, Green, and Blue Luminescence by Carbon Dots: Full-Color Emission Tuning and Multicolor Cellular Imaging, Angew. Chem., Int. Ed., 2015, 54, 5360–5363 CrossRef CAS PubMed.
- Y. Xiong, J. Schneider, E. V. Ushakova and A. L. Rogach, Influence of molecular fluorophores on the research field of chemically synthesized carbon dots, Nano Today, 2018, 23, 124–139 CrossRef CAS.
- L. Bao, Z.-L. Zhang, Z.-Q. Tian, L. Zhang, C. Liu, Y. Lin, B. Qi and D.-W. Pang, Electrochemical Tuning of Luminescent Carbon Nanodots: From Preparation to Luminescence Mechanism, Adv. Mater., 2011, 23, 5801–5806 CrossRef CAS PubMed.
- H. Ding, S.-B. Yu, J.-S. Wei and H.-M. Xiong, Full-Color Light-Emitting Carbon Dots with a Surface-State-Controlled Luminescence Mechanism, ACS Nano, 2016, 10, 484–491 CrossRef CAS PubMed.
- C. Xia, W. Wu, T. Yu, X. Xie, C. van Oversteeg, H. C. Gerritsen and C. d. M. Donega, Size-Dependent Band-Gap and Molar Absorption Coefficients of Colloidal CuInS2 Quantum Dots, ACS Nano, 2018, 12, 8350–8361 CrossRef CAS PubMed.
- Z. Gan, X. Wu, G. Zhou, J. Shen and P. K. Chu, Is There Real Upconversion Photoluminescence from Graphene Quantum Dots?, Adv. Opt. Mater., 2013, 1, 554–558 CrossRef.
- A. Yadav, L. Bai, Y. Yang, J. Liu, A. Kaushik, G. J. Cheng, L. Jiang, L. Chi and Z. Kang, Lasing behavior of surface functionalized carbon quantum dot/RhB composites, Nanoscale, 2017, 9, 5049–5054 RSC.
- K. Jiang, X. Gao, X. Feng, Y. Wang, Z. Li and H. Lin, Carbon Dots with Dual-Emissive, Robust, and Aggregation-Induced Room-Temperature Phosphorescence Characteristics, Angew. Chem., Int. Ed., 2020, 59, 1263–1269 CrossRef CAS PubMed.
- H. Yang, Y. Liu, Z. Guo, B. Lei, J. Zhuang, X. Zhang, Z. Liu and C. Hu, Hydrophobic carbon dots with blue dispersed emission and red aggregation-induced emission, Nat. Commun., 2019, 10, 1789 CrossRef PubMed.
- G. D. Scholes and G. Rumbles, Excitons in nanoscale systems, Nat. Mater., 2006, 5, 683–696 CrossRef CAS PubMed.
- S. K. Das, Y. Liu, S. Yeom, D. Y. Kim and C. I. Richards, Single-Particle Fluorescence Intensity Fluctuations of Carbon Nanodots, Nano Lett., 2014, 14, 620–625 CrossRef CAS PubMed.
- A. Cadranel, J. T. Margraf, V. Strauss, T. Clark and D. M. Guldi, Carbon Nanodots for Charge-Transfer Processes, Acc. Chem. Res., 2019, 52, 955–963 CrossRef CAS PubMed.
- L. Vallan, R. Canton-Vitoria, H. B. Gobeze, Y. Jang, R. Arenal, A. M. Benito, W. K. Maser, F. D'Souza and N. Tagmatarchis, Interfacing Transition Metal Dichalcogenides with Carbon Nanodots for Managing Photoinduced Energy and Charge-Transfer Processes, J. Am. Chem. Soc., 2018, 140, 13488–13496 CrossRef CAS PubMed.
- V. Strauss, J. T. Margraf, C. Dolle, B. Butz, T. J. Nacken, J. Walter, W. Bauer, W. Peukert, E. Spiecker, T. Clark and D. M. Guldi, Carbon Nanodots: Toward a Comprehensive Understanding
of Their Photoluminescence, J. Am. Chem. Soc., 2014, 136, 17308–17316 CrossRef CAS PubMed.
- L. Li, G. Wu, G. Yang, J. Peng, J. Zhao and J. J. Zhu, Focusing on luminescent graphene quantum dots: current status and future perspectives, Nanoscale, 2013, 5, 4015–4039 RSC.
- L. Tang, R. Ji, X. Cao, J. Lin, H. Jiang, X. Li, K. S. Teng, C. M. Luk, S. Zeng, J. Hao and S. P. Lau, Deep Ultraviolet Photoluminescence of Water-Soluble Self-Passivated Graphene Quantum Dots, ACS Nano, 2012, 6, 5102–5110 CrossRef CAS PubMed.
- L. Bao, C. Liu, Z.-L. Zhang and D.-W. Pang, Photoluminescence-Tunable Carbon Nanodots: Surface-State Energy-Gap Tuning, Adv. Mater., 2015, 27, 1663–1667 CrossRef CAS PubMed.
- H. Yu, R. Shi, Y. Zhao, G. I. Waterhouse, L. Z. Wu, C. H. Tung and T. Zhang, Smart Utilization of Carbon Dots in Semiconductor Photocatalysis, Adv. Mater., 2016, 28, 9454–9477 CrossRef CAS PubMed.
- T.-F. Yeh, C.-Y. Teng, S.-J. Chen and H. Teng, Nitrogen-Doped Graphene Oxide Quantum Dots as Photocatalysts for Overall Water-Splitting under Visible Light Illumination, Adv. Mater., 2014, 26, 3297–3303 CrossRef CAS PubMed.
- A. Cadranel, V. Strauss, J. T. Margraf, K. A. Winterfeld, C. Vogl, L. Dordevic, F. Arcudi, H. Hoelzel, N. Jux, M. Prato and D. M. Guldi, Screening Supramolecular Interactions between Carbon Nanodots and Porphyrins, J. Am. Chem. Soc., 2018, 140, 904–907 CrossRef CAS PubMed.
- F. Arcudi, V. Strauss, L. Dordevic, A. Cadranel, D. M. Guldi and M. Prato, Porphyrin Antennas on Carbon Nanodots: Excited State Energy and Electron Transduction, Angew. Chem., Int. Ed., 2017, 56, 12097–12101 CrossRef CAS PubMed.
- M. Dahan, T. Laurence, F. Pinaud, D. S. Chemla, A. P. Alivisatos, M. Sauer and S. Weiss, Time-gated biological imaging by use of colloidal quantum dots, Opt. Lett., 2001, 26, 825–827 CrossRef CAS PubMed.
- H. E. Grecco, K. A. Lidke, R. Heintzmann, D. S. Lidke, C. Spagnuolo, O. E. Martinez, E. A. Jares-Erijman and T. M. Jovin, Ensemble and single particle photophysical properties (two-photon excitation, anisotropy, FRET, lifetime, spectral conversion) of commercial quantum dots in solution and in live cells, Microsc. Res. Tech., 2004, 65, 169–179 CrossRef CAS PubMed.
- S.-L. Liu, Z.-G. Wang, Z.-L. Zhang and D.-W. Pang, Tracking single viruses infecting their host cells using quantum dots, Chem. Soc. Rev., 2016, 45, 1211–1224 RSC.
- T. L. Doane and C. Burda, The unique role of nanoparticles in nanomedicine: imaging, drug delivery and therapy, Chem. Soc. Rev., 2012, 41, 2885–2911 RSC.
- M. E. Akerman, W. C. W. Chan, P. Laakkonen, S. N. Bhatia and E. Ruoslahti, Nanocrystal targeting in vivo, Proc. Natl. Acad. Sci. U. S. A., 2002, 99, 12617–12621 CrossRef CAS PubMed.
- B. Dubertret, P. Skourides, D. J. Norris, V. Noireaux, A. H. Brivanlou and A. Libchaber, In vivo imaging of quantum dots encapsulated in phospholipid micelles, Science, 2002, 298, 1759–1762 CrossRef CAS PubMed.
- S. Rieger, R. P. Kulkarni, D. Darcy, S. E. Fraser and R. W. Koster, Quantum dots are powerful multipurpose vital labeling agents in zebrafish embryos, Dev. Dyn., 2005, 234, 670–681 CrossRef CAS PubMed.
- M. M. Barroso, Quantum dots in cell biology, J. Histochem. Cytochem., 2011, 59, 237 CrossRef CAS PubMed.
- K. A. Lidke, B. Rieger, T. M. Jovin and R. Heintzmann, Superresolution by localization of quantum dots using blinking statistics, Opt. Express, 2005, 13, 7052–7062 CrossRef PubMed.
- M. De, P. S. Ghosh and V. M. Rotello, Applications of Nanoparticles in Biology, Adv. Mater., 2008, 20, 4225–4241 CrossRef CAS.
- R. Freeman and I. Willner, Optical molecular sensing with semiconductor quantum dots (QDs), Chem. Soc. Rev., 2012, 41, 4067–4085 RSC.
- P. Wu and X.-P. Yan, Doped quantum dots for chemo/biosensing and bioimaging, Chem. Soc. Rev., 2013, 42, 5489–5521 RSC.
- N. Hildebrandt, C. M. Spillmann, W. R. Algar, T. Pons, M. H. Stewart, E. Oh, K. Susumu, S. A. Díaz, J. B. Delehanty and I. L. Medintz, Energy Transfer with Semiconductor Quantum Dot Bioconjugates: A Versatile Platform for Biosensing, Energy Harvesting, and Other Developing Applications, Chem. Rev., 2016, 536–711 Search PubMed.
- S. Silvi and A. Credi, Luminescent sensors based on quantum dot-molecule conjugates, Chem. Soc. Rev., 2015, 44, 4275–4289 RSC.
- V. Biju, T. Itoh and M. Ishikawa, Delivering quantum dots to cells: bioconjugated quantum dots for targeted and nonspecific extracellular and intracellular imaging, Chem. Soc. Rev., 2010, 39, 3031–3056 RSC.
- G. Palui, F. Aldeek, W. Wang and H. Mattoussi, Strategies for interfacing inorganic nanocrystals with biological systems based on polymer-coating, Chem. Soc. Rev., 2015, 44, 193–227 RSC.
- G. Xu, S. Zeng, B. Zhang, M. T. Swihart, K.-T. Yong and P. N. Prasad, New Generation Cadmium-Free Quantum Dots for Biophotonics and Nanomedicine, Chem. Rev., 2016, 116, 12234–12327 CrossRef CAS PubMed.
- M. X. Yu and J. Zheng, Clearance Pathways and Tumor Targeting of Imaging Nanoparticles, ACS Nano, 2015, 9, 6655–6674 CrossRef CAS PubMed.
- K.-T. Yong, W.-C. Law, R. Hu, L. Ye, L. Liu, M. T. Swihart and P. N. Prasad, Nanotoxicity assessment of quantum dots: from cellular to primate studies, Chem. Soc. Rev., 2013, 42, 1236–1250 RSC.
- S. Sharifi, S. Behzadi, S. Laurent, M. L. Forrest, P. Stroeve and M. Mahmoudi, Toxicity of nanomaterials, Chem. Soc. Rev., 2012, 41, 2323–2343 RSC.
- H. S. Choi, W. Liu, P. Misra, E. Tanaka, J. P. Zimmer, B. I. Ipe, M. G. Bawendi and J. V. Frangioni, Renal clearance of quantum dots, Nat. Biotechnol., 2007, 25, 1165–1170 CrossRef CAS PubMed.
- H. S. Choi, W. Liu, F. Liu, K. Nasr, P. Misra, M. G. Bawendi and J. V. Frangioni, Design considerations for tumour-targeted nanoparticles, Nat. Nanotechnol., 2010, 5, 42–47 CrossRef CAS PubMed.
- C. E. Bradburne, J. B. Delehanty, K. B. Gemmill, B. C. Mei, H. Mattoussi, K. Susumu, J. B. Blanco-Canosa, P. E. Dawson and I. L. Medintz, Cytotoxicity of Quantum Dots Used for In Vitro Cellular Labeling: Role of QD Surface Ligand, Delivery Modality, Cell Type, and Direct Comparison to Organic Fluorophores, Bioconjugate Chem., 2013, 24, 1570–1583 CrossRef CAS PubMed.
- T. S. Hauck, R. E. Anderson, H. C. Fischer, S. Newbigging and W. C. W. Chan, In vivo Quantum-Dot Toxicity Assessment, Small, 2010, 6, 138–144 CrossRef CAS PubMed.
- Y.-K. Hsieh, H.-A. Hsieh, H.-F. Hsieh, T.-H. Wang, C.-C. Ho, P.-P. Lin and C.-F. Wang, Using laser ablation inductively coupled plasma mass spectrometry to characterize the biointeractions of inhaled CdSe quantum dots in the mouse lungs, J. Anal. At. Spectrom., 2013, 28, 1396–1401 RSC.
- M. Longmire, P. L. Choyke and H. Kobayashi, Clearance properties of nano-sized particles and molecules as imaging agents: considerations and caveats, Nanomedicine, 2008, 3, 703–717 CrossRef CAS PubMed.
- K. Yang, L. Feng, X. Shi and Z. Liu, Nano-graphene in biomedicine: theranostic applications, Chem. Soc. Rev., 2013, 42, 530–547 RSC.
- K. Nekoueian, M. o. Amiri, M. Sillanpaa, F. Marken, R. Boukherroub and S. Szunerits, Carbon-based quantum particles: an electroanalytical and biomedical perspective, Chem. Soc. Rev., 2019, 48, 4281–4316 RSC.
- N. Panwar, A. M. Soehartono, K. K. Chan, S. Zeng, G. Xu, J. Qu, P. Coquet, K.-T. Yong and X. Chen, Nanocarbons for Biology and Medicine: Sensing, Imaging, and Drug Delivery, Chem. Rev., 2019, 119, 9559–9656 CrossRef CAS PubMed.
- Z. H. Kang and S. T. Lee, Carbon dots: advances in nanocarbon applications, Nanoscale, 2019, 11, 19214–19224 RSC.
- C. Pang and Y. Gong, Current Status and Future Prospects of Semiconductor Quantum Dots in Botany, J. Agric. Food Chem., 2019, 67, 7561–7568 CrossRef CAS PubMed.
- X. Shi, W. Wei, Z. Fu, W. Gao, C. Zhang, Q. Zhao, F. Deng and X. Lu, Review on carbon dots in food safety applications, Talanta, 2019, 194, 809–821 CrossRef CAS PubMed.
- Y. Dong, J. Cai, X. You and Y. Chi, Sensing applications of luminescent carbon based dots, Analyst, 2015, 140, 7468–7486 RSC.
- S.-T. Yang, L. Cao, P. G. Luo, F. Lu, X. Wang, H. Wang, M. J. Meziani, Y. Liu, G. Qi and Y.-P. Sun, Carbon Dots for Optical Imaging in Vivo, J. Am. Chem. Soc., 2009, 131, 11308–11309 CrossRef CAS PubMed.
- S.-T. Yang, X. Wang, H. Wang, F. Lu, P. G. Luo, L. Cao, M. J. Meziani, J.-H. Liu, Y. Liu, M. Chen, Y. Huang and Y.-P. Sun, Carbon Dots as Nontoxic and High-Performance Fluorescence Imaging Agents, J. Phys. Chem. C, 2009, 113, 18110–18114 CrossRef CAS PubMed.
- J. Ge, M. Lan, B. Zhou, W. Liu, L. Guo, H. Wang, Q. Jia, G. Niu, X. Huang, H. Zhou, X. Meng, P. Wang, C.-S. Lee, W. Zhang and X. Han, A graphene quantum dot photodynamic therapy agent with high singlet oxygen generation, Nat. Commun., 2014, 5, 4596 CrossRef CAS PubMed.
- J. Du, N. Xu, J. Fan, W. Sun and X. Peng, Carbon Dots for In Vivo Bioimaging and Theranostics, Small, 2019, e1805087 CrossRef PubMed.
- H. Li, J. Huang, Y. Song, M. Zhang, H. Wang, F. Lu, H. Huang, Y. Liu, X. Dai, Z. Gu, Z. Yang, R. Zhou and Z. Kang, Degradable Carbon Dots with Broad-Spectrum Antibacterial Activity, ACS Appl. Mater. Interfaces, 2018, 10, 26936–26946 CrossRef CAS PubMed.
- Q. Xin, H. Shah, A. Nawaz, W. Xie, M. Z. Akram, A. Batool, L. Tian, S. U. Jan, R. Boddula, B. Guo, Q. Liu and J. R. Gong, Antibacterial Carbon-Based Nanomaterials, Adv. Mater., 2019, 31, 1804838 CrossRef CAS PubMed.
- P. Devi, S. Saini and K.-H. Kim, The advanced role of carbon quantum dots in nanomedical applications, Biosens. Bioelectron., 2019, 141, 111158 CrossRef CAS PubMed.
- H. Li, W. Kong, J. Liu, M. Yang, H. Huang, Y. Liu and Z. Kang, Carbon dots for photoswitching enzyme catalytic activity, J. Mater. Chem. B, 2014, 2, 5652–5658 RSC.
- Y. Wang and Y. Xia, Optical, electrochemical and catalytic methods for in-vitro diagnosis using carbonaceous nanoparticles: a review, Mikrochim. Acta, 2019, 186, 50 CrossRef PubMed.
- B. Garg and T. Bisht, Carbon Nanodots as Peroxidase Nanozymes for Biosensing, Molecules, 2016, 21, 1653 CrossRef CAS PubMed.
- H. Li, J. Huang, Y. Liu, F. Lu, J. Zhong, Y. Wang, S. Li, Y. Lifshitz, S.-T. Lee and Z. Kang, Enhanced RuBisCO activity and promoted dicotyledons growth with degradable carbon dots, Nano Res., 2019, 12, 1585–1593 CrossRef CAS.
- R. Shi, H. Li, E. Wu, L. Xiong, R. Lv, R. Guo, Y. Liu, G. Xu, Z. Kang and J. Liu, Simultaneous enzymatic activity modulation and rapid determination of enzyme kinetics by highly crystalline graphite dots, Nanoscale, 2017, 9, 8410–8417 RSC.
- H. Li, S. Guo, C. Li, H. Huang, Y. Liu and Z. Kang, Tuning Laccase Catalytic Activity with Phosphate Functionalized Carbon Dots by Visible Light, ACS Appl. Mater. Interfaces, 2015, 7, 10004–10012 CrossRef CAS PubMed.
- V. Biju, Chemical modifications and bioconjugate reactions of nanomaterials for sensing, imaging, drug delivery and therapy, Chem. Soc. Rev., 2014, 43, 744–764 RSC.
- M. Zhang, H. Wang, Y. Song, H. Huang, M. Shao, Y. Liu, H. Li and Z. Kang, Pristine Carbon Dots Boost the Growth of Chlorella vulgaris by Enhancing Photosynthesis, ACS Appl. Bio Mater., 2018, 1, 894–902 CrossRef CAS.
- H. Li, J. Huang, F. Lu, Y. Liu, Y. Song, Y. Sun, J. Zhong, H. Huang, Y. Wang, S. Li, Y. Lifshitz, S.-T. Lee and Z. Kang, Impacts of Carbon Dots on Rice Plants: Boosting the Growth and Improving the Disease Resistance, ACS Appl. Bio Mater., 2018, 1, 663–672 CrossRef CAS.
- M. Zhang, L. Hu, H. Wang, Y. Song, Y. Liu, H. Li, M. Shao, H. Huang and Z. Kang, One-step hydrothermal synthesis of chiral carbon dots and their effects on mung bean plant growth, Nanoscale, 2018, 10, 12734–12742 RSC.
- H. Huang, S. Yang, Y. Liu, Y. Yang, H. Li, J. A. McLeod, G. Ding, J. Huang and Z. Kang, Photocatalytic Polymerization from Amino Acid to Protein by Carbon Dots at Room Temperature, ACS Appl. Bio Mater., 2019, 2, 5144–5153 CrossRef CAS.
- M. Zhang, H. Wang, P. Liu, Y. Song, H. Huang, M. Shao, Y. Liu, H. Li and Z. Kang, Biotoxicity of degradable carbon dots towards microalgae Chlorella vulgaris, Environ. Sci.: Nano, 2019, 6, 3316–3323 RSC.
- M. Nurunnabi, Z. Khatun, K. M. Huh, S. Y. Park, D. Y. Lee, K. J. Cho and Y.-k. Lee, In Vivo Biodistribution and Toxicology of Carboxylated Graphene Quantum Dots, ACS Nano, 2013, 7, 6858–6867 CrossRef CAS PubMed.
- W. Kong, J. Liu, R. Liu, H. Li, Y. Liu, H. Huang, K. Li, J. Liu, S.-T. Lee and Z. Kang, Quantitative and real-time effects of carbon quantum dots on single living HeLa cell membrane permeability, Nanoscale, 2014, 6, 5116–5120 RSC.
- T. H. Kim, J. P. Sirdaarta, Q. Zhang, E. Eftekhari, J. S. John, D. Kennedy, I. E. Cock and Q. Li, Selective toxicity of hydroxyl-rich carbon nanodots for cancer research, Nano Res., 2018, 11, 2204–2216 CrossRef CAS.
- P. Roy, A. P. Periasamy, C.-Y. Lin, G.-M. Her, W.-J. Chiu, C.-L. Li, C.-L. Shu, C.-C. Huang, C.-T. Liang and H.-T. Chang, Photoluminescent graphene quantum dots for in vivo imaging of apoptotic cells, Nanoscale, 2015, 7, 2504–2510 RSC.
- Y. Qin, Z.-W. Zhou, S.-T. Pan, Z.-X. He, X. Zhang, J.-X. Qiu, W. Duan, T. Yang and S.-F. Zhou, Graphene quantum dots induce apoptosis, autophagy, and inflammatory response via p38 mitogen-activated protein kinase and nuclear factor-kappa B mediated signaling pathways in activated THP-1 macrophages, Toxicology, 2015, 327, 62–76 CrossRef CAS PubMed.
- A. Chandra, S. Deshpande, D. B. Shinde, V. K. Pillai and N. Singh, Mitigating the Cytotoxicity of Graphene Quantum Dots and Enhancing Their Applications in Bioimaging and Drug Delivery, ACS Macro Lett., 2014, 3, 1064–1068 CrossRef CAS.
- Y. Segawa, D. R. Levine and K. Itami, Topologically Unique Molecular Nanocarbons, Acc. Chem. Res., 2019, 52, 2760–2767 CrossRef CAS PubMed.
- Y. S. Rim, S. H. Bae, H. J. Chen, N. De Marco and Y. Yang, Recent Progress in Materials and Devices toward Printable and Flexible Sensors, Adv. Mater., 2016, 28, 4415–4440 CrossRef CAS PubMed.
- E. Jang, S. Jun, H. Jang, J. Llim, B. Kim and Y. Kim, White-Light-Emitting Diodes with Quantum Dot Color Converters for Display Backlights, Adv. Mater., 2010, 22, 3076–3080 CrossRef CAS PubMed.
- H. Zhu, Y. Yang and T. Lian, Multiexciton Annihilation and Dissociation in Quantum Confined Semiconductor Nanocrystals, Acc. Chem. Res., 2013, 46, 1270–1279 CrossRef CAS PubMed.
- J. A. McGuire, J. Joo, J. M. Pietryga, R. D. Schaller and V. I. Klimov, New Aspects of Carrier Multiplication in Semiconductor Nanocrystals, Acc. Chem. Res., 2008, 41, 1810–1819 CrossRef CAS PubMed.
- D. Vanmaekelbergh and P. Liljeroth, Electron-conducting quantum dot solids: novel materials based on colloidal semiconductor nanocrystals, Chem. Soc. Rev., 2005, 34, 299–312 RSC.
- Y. Liu, M. Gibbs, J. Puthussery, S. Gaik, R. Ihly, H. W. Hillhouse and M. Law, Dependence of Carrier Mobility on Nanocrystal Size and Ligand Length in PbSe Nanocrystal Solids, Nano Lett., 2010, 10, 1960–1969 CrossRef CAS PubMed.
- C. P. Collier, R. J. Saykally, J. J. Shiang, S. E. Henrichs and J. R. Heath, Reversible Tuning of Silver Quantum Dot Monolayers Through the Metal-Insulator Transition, Science, 1997, 277, 1978–1981 CrossRef CAS.
- M. V. Kovalenko, M. Scheele and D. V. Talapin, Colloidal Nanocrystals with Molecular Metal Chalcogenide Surface Ligands, Science, 2009, 324, 1417–1420 CrossRef CAS PubMed.
- C. R. Kagan and C. B. Murray, Charge transport in strongly coupled quantum dot solids, Nat. Nanotechnol., 2015, 10, 1013–1026 CrossRef CAS PubMed.
- D. S. Dolzhnikov, H. Zhang, J. Jang, J. S. Son, M. G. Panthani, T. Shibata, S. Chattopadhyay and D. V. Talapin, Composition-matched molecular “solders” for semiconductors, Science, 2015, 347, 425–428 CrossRef CAS PubMed.
- V. L. Colvin, M. C. Schlamp and A. P. Alivisatos, Light-emitting diodes made from cadmium selenide nanocrystals and a semiconducting polymer, Nature, 1994, 370, 354–357 CrossRef CAS.
- X. L. Dai, Z. X. Zhang, Y. Z. Jin, Y. Niu, H. J. Cao, X. Y. Liang, L. W. Chen, J. P. Wang and X. G. Peng, Solution-processed, high-performance light-emitting diodes based on quantum dots, Nature, 2014, 515, 96–99 CrossRef CAS PubMed.
- S. A. Crooker, T. Barrick, J. A. Hollingsworth and V. I. Klimov, Multiple temperature regimes of radiative decay in CdSe nanocrystal quantum dots: Intrinsic limits to the dark-exciton lifetime, Appl. Phys. Lett., 2003, 82, 2793–2795 CrossRef CAS.
- A. L. Efros, M. Rosen, M. Kuno, M. Nirmal, D. J. Norris and M. Bawendi, Band-edge exciton in quantum dots of semiconductors with a degenerate valence band: dark and bright exciton states, Phys. Rev. B: Condens. Matter Mater. Phys., 1996, 54, 4843 CrossRef CAS PubMed.
- M. Chamarro, C. Gourdon, P. Lavallard, O. Lublinskaya and A. I. Ekimov, Enhancement of electron-hole exchange interaction in CdSe nanocrystals: a quantum confinement effect, Phys. Rev. B: Condens. Matter Mater. Phys., 1996, 53, 1336–1342 CrossRef CAS PubMed.
- J. W. Stouwdam and R. A. J. Janssen, Red, green, and blue quantum dot LEDs with solution processable ZnO nanocrystal electron injection layers, J. Mater. Chem., 2008, 18, 1889–1894 RSC.
- I. J. Kramer and E. H. Sargent, Colloidal Quantum Dot Photovoltaics: A Path Forward, ACS Nano, 2011, 5, 8506–8514 CrossRef CAS PubMed.
- M. R. Kim and D. L. Ma, Quantum-Dot-Based Solar Cells: Recent Advances, Strategies, and Challenges, J. Phys. Chem. Lett., 2015, 6, 85–99 CrossRef CAS PubMed.
- J. Tian, Q. Zhang, L. Zhang, R. Gao, L. Shen, S. Zhang, X. Qu and G. Cao, ZnO/TiO2 nanocable structured photoelectrodes for CdS/CdSe quantum dot co-sensitized solar cells, Nanoscale, 2013, 5, 936–943 RSC.
- Q. Zhang, G. Chen, Y. Yang, X. Shen, Y. Zhang, C. Li, R. Yu, Y. Luo, D. Li and Q. Meng, Toward highly efficient CdS/CdSe quantum dots-sensitized solar cells incorporating ordered photoanodes on transparent conductive substrates, Phys. Chem. Chem. Phys., 2012, 14, 6479 RSC.
- M. Semeniuk, Z. Yi, V. Poursorkhabi, J. Tjong, S. Jaffer, Z.-H. Lu and M. Sain, Future Perspectives and Review on Organic Carbon Dots in Electronic Applications, ACS Nano, 2019, 13, 6224–6255 CrossRef CAS PubMed.
- T. Yuan, T. Meng, P. He, Y. X. Shi, Y. C. Li, X. H. Li, L. Z. Fan and S. H. Yang, Carbon quantum dots: an emerging material for optoelectronic applications, J. Mater. Chem. C, 2019, 7, 6820–6835 RSC.
- K. Hola, Y. Zhang, Y. Wang, E. P. Giannelis, R. Zboril and A. L. Rogach, Carbon dots-Emerging light emitters for bioimaging, cancer therapy and optoelectronics, Nano Today, 2014, 9, 590–603 CrossRef CAS.
- V. Gupta, N. Chaudhary, R. Srivastava, G. D. Sharma, R. Bhardwaj and S. Chand, Luminscent Graphene Quantum Dots for Organic Photovoltaic Devices, J. Am. Chem. Soc., 2011, 133, 9960–9963 CrossRef CAS PubMed.
- F. Yuan, Z. Wang, X. Li, Y. Li, Z. a. Tan, L. Fan and S. Yang, Bright Multicolor Bandgap Fluorescent Carbon Quantum Dots for Electroluminescent Light-Emitting Diodes, Adv. Mater., 2017, 29, 1604436 CrossRef PubMed.
- H. R. Jia, Z. B. Wang, T. Yuan, F. L. Yuan, X. H. Li, Y. C. Li, Z. A. Tan, L. Z. Fan and S. H. Yang, Electroluminescent Warm White Light-Emitting Diodes Based on Passivation Enabled Bright Red Bandgap Emission Carbon Quantum Dots, Adv. Sci., 2019, 6, 1900397 CrossRef PubMed.
- X. Yan, X. Cui, B. Li and L.-s. Li, Large, Solution-Processable Graphene Quantum Dots as Light Absorbers for Photovoltaics, Nano Lett., 2010, 10, 1869–1873 CrossRef CAS PubMed.
- R. D. Costa, F. Lodermeyer, R. Casillas and D. M. Guldi, Recent advances in multifunctional nanocarbons used in dye-sensitized solar cells, Energy Environ. Sci., 2014, 7, 1281 RSC.
- Q. Guo, F. L. Yuan, B. Zhang, S. J. Zhou, J. Zhang, Y. M. Bai, L. Z. Fan, T. Hayat, A. Alsaedi and Z. A. Tan, Passivation of the grain boundaries of CH3NH3PbI3 using carbon quantum dots for highly efficient perovskite solar cells with excellent environmental stability, Nanoscale, 2019, 11, 115–124 RSC.
- J. Owens and L. Brus, Chemical Synthesis and Luminescence Applications of Colloidal Semiconductor Quantum Dots, J. Am. Chem. Soc., 2017, 139, 10939–10943 CrossRef PubMed.
- Z. H. Jin, P. Owour, S. D. Lei and L. H. Ge, Graphene, graphene quantum dots and their applications in optoelectronics, Curr. Opin. Colloid Interface Sci., 2015, 20, 439–453 CrossRef CAS.
- G. X. Ni, A. S. McLeod, Z. Sun, L. Wang, L. Xiong, K. W. Post, S. S. Sunku, B. Y. Jiang, J. Hone, C. R. Dean, M. M. Fogler and D. N. Basov, Fundamental limits to graphene plasmonics, Nature, 2018, 557, 530–533 CrossRef CAS PubMed.
- H. Zhu, N. Song, H. Lv, C. L. Hill and T. Lian, Near unity quantum yield of light-driven redox mediator reduction and efficient H2 generation using colloidal nanorod heterostructures, J. Am. Chem. Soc., 2012, 134, 11701–11708 CrossRef CAS PubMed.
- N. Razgoniaeva, P. Moroz, S. Lambright and M. Zamkov, Photocatalytic Applications of Colloidal Heterostructured Nanocrystals: What's Next?, J. Phys. Chem. Lett., 2015, 6, 4352–4359 CrossRef CAS PubMed.
- K. Wu, Z. Chen, H. Lv, H. Zhu, C. L. Hill and T. Lian, Hole Removal Rate Limits Photodriven H-2 Generation Efficiency in CdS-Pt and CdSe/CdS-Pt Semiconductor Nanorod-Metal Tip Heterostructures, J. Am. Chem. Soc., 2014, 136, 7708–7716 CrossRef CAS PubMed.
- K. F. Wu and T. Q. Lian, Quantum confined colloidal nanorod heterostructures for solar-to-fuel conversion, Chem. Soc. Rev., 2016, 45, 3781–3810 RSC.
- J. Liu, Y. Liu, N. Liu, Y. Han, X. Zhang, H. Huang, Y. Lifshitz, S.-T. Lee, J. Zhong and Z. Kang, Metal-free efficient photocatalyst for stable visible water splitting via a two-electron pathway, Science, 2015, 347, 970–974 CrossRef CAS.
- S. Guo, S. Zhao, X. Wu, H. Li, Y. Zhou, C. Zhu, N. Yang, X. Jiang, J. Gao, L. Bai, Y. Liu, Y. Lifshitz, S.-T. Lee and Z. Kang, A Co3O4-CDots-C3N4 three component electrocatalyst design concept for efficient and tunable CO2 reduction to syngas, Nat. Commun., 2017, 8, 1828 CrossRef PubMed.
- M. B. Wilker, K. J. Schnitzenbaumer and G. Dukovic, Recent Progress in Photocatalysis Mediated by Colloidal II-VI Nanocrystals, Isr. J. Chem., 2012, 52, 1002–1015 CrossRef CAS PubMed.
- G. Gong, Y. Liu, B. Mao, L. Tan, Y. Yang and W. Shi, Ag doping of Zn-In-S quantum dots for photocatalytic hydrogen evolution: Simultaneous bandgap narrowing and carrier lifetime elongation, Appl. Catal., B, 2017, 216, 11–19 CrossRef CAS.
- L. Tan, Y. Liu, B. Mao, B. Luo, G. Gong, Y. Hong, B. Chen and W. Shi, Effective bandgap narrowing of Cu-In-Zn-S quantum dots for photocatalytic H2 production via cocatalyst-alleviated charge recombination, Inorg. Chem. Front., 2018, 5, 258–265 RSC.
- Y. Yang, B. Mao, G. Gong, D. Li, Y. Liu, W. Cao, L. Xing, J. Zeng, W. Shi and S. Yuan, In-situ growth of Zn–AgIn5S8 quantum dots on g-C3N4 towards 0D/2D heterostructured photocatalysts with enhanced hydrogen production, Int. J. Hydrogen Energy, 2019, 44, 15882–15891 CrossRef CAS.
- K. Wu, J. Chen, J. R. McBride and T. Lian, Efficient hot-electron transfer by a plasmon-induced interfacial charge-transfer transition, Science, 2015, 349, 632–635 CrossRef CAS PubMed.
- K. P. Acharya, R. S. Khnayzer, T. O'Connor, G. Diederich, M. Kirsanova, A. Klinkova, D. Roth, E. Kinder, M. Imboden and M. Zamkov, The role of hole localization in sacrificial hydrogen production by semiconductor-metal heterostructured nanocrystals, Nano Lett., 2011, 11, 2919–2926 CrossRef CAS.
- X. Chen, S. Shen, L. Guo and S. S. Mao, Semiconductor-based Photocatalytic Hydrogen Generation, Chem. Rev., 2010, 110, 6503 CrossRef CAS PubMed.
- Y. Yang and T. Lian, Multiple exciton dissociation and hot electron extraction by ultrafast interfacial electron transfer from PbS QDs, Coord. Chem. Rev., 2014, 263–264, 229–238 CrossRef CAS.
- W. A. Tisdale and X. Y. Zhu, Artificial atoms on semiconductor surfaces, Proc. Natl. Acad. Sci. U. S. A., 2011, 108, 965–970 CrossRef CAS PubMed.
- M. J. Berr, A. Vaneski, C. Mauser, S. Fischbach, A. S. Susha, A. L. Rogach, F. Jäckel and J. Feldmann, Delayed Photoelectron Transfer in Pt-Decorated CdS Nanorods under Hydrogen Generation Conditions, Small, 2012, 8, 291–297 CrossRef CAS PubMed.
- K. Wu, Z. Chen, H. Lv, H. Zhu, C. L. Hill and T. Lian, Hole removal rate limits photodriven H2 generation efficiency in CdS-Pt and CdSe/CdS-Pt semiconductor nanorod-metal tip heterostructures, J. Am. Chem. Soc., 2014, 136, 7708 CrossRef CAS PubMed.
- T. O'Connor, M. S. Panov, A. Mereshchenko, A. N. Tarnovsky, R. Lorek, D. Perera, G. Diederich, S. Lambright, P. Moroz and M. Zamkov, The effect of the charge-separating interface on exciton dynamics in photocatalytic colloidal heteronanocrystals, ACS Nano, 2012, 6, 8156–8165 CrossRef PubMed.
- E. Khon, K. Lambright, R. S. Khnayzer, P. Moroz, D. Perera, E. Butaeva, S. Lambright, F. N. Castellano and M. Zamkov, Improving the catalytic activity of semiconductor nanocrystals through selective domain etching, Nano Lett., 2013, 13, 2016 CrossRef CAS PubMed.
- N. Bao, L. Shen, T. Takata and K. Domen, Self-Templated Synthesis of Nanoporous CdS Nanostructures for Highly Efficient Photocatalytic Hydrogen Production under Visible Light, Chem. Mater., 2008, 39, 110–117 CrossRef.
- H. Yan, J. Yang, G. Ma, G. Wu, Z. Xu, Z. Lei, J. Shi and C. Li, Visible-light-driven hydrogen production with extremely high quantum efficiency on Pt–PdS/CdS photocatalyst, J. Catal., 2009, 266, 165–168 CrossRef CAS.
- L. Huang, X. Wang, J. Yang, G. Liu, J. Han and C. Li, Dual Cocatalysts Loaded Type I CdS/ZnS Core/Shell Nanocrystals as Effective and Stable Photocatalysts for H2 Evolution, J. Phys. Chem. C, 2013, 117, 11584–11591 CrossRef CAS.
- M. Liu, D. Jing, Z. Zhou and L. Guo, Twin-induced one-dimensional homojunctions yield high quantum efficiency for solar hydrogen generation, Nat. Commun., 2013, 4, 2278 CrossRef PubMed.
- J. Yang, H. Yan, X. Wang, F. Wen, Z. Wang, D. Fan, J. Shi and C. Li, Roles of cocatalysts in Pt–PdS/CdS with exceptionally high quantum efficiency for photocatalytic hydrogen production, J. Catal., 2012, 290, 151–157 CrossRef CAS.
- T. Simon, N. Bouchonville, M. J. Berr, A. Vaneski, A. Adrović, D. Volbers, R. Wyrwich, M. Döblinger, A. S. Susha and A. L. Rogach, Redox shuttle mechanism enhances photocatalytic H2 generation on Ni-decorated CdS nanorods, Nat. Mater., 2014, 13, 1013–1018 CrossRef CAS.
- P. Kalisman, Y. Nakibli and L. Amirav, Perfect Photon-to-Hydrogen Conversion Efficiency, Nano Lett., 2016, 16, 1776 CrossRef CAS PubMed.
- G. A. M. Hutton, B. C. M. Martindale and E. Reisner, Carbon dots as photosensitisers for solar-driven catalysis, Chem. Soc. Rev., 2017, 46, 6111–6123 RSC.
- Q. Li, R. Cao, J. Cho and G. Wu, Nanocarbon Electrocatalysts for Oxygen Reduction in Alkaline Media for Advanced Energy Conversion and Storage, Adv. Energy Mater., 2014, 4, 1301415 CrossRef.
- C. Tang and Q. Zhang, Nanocarbon for Oxygen Reduction Electrocatalysis: Dopants, Edges, and Defects, Adv. Mater., 2017, 29, 1604103 CrossRef PubMed.
- H. T. Li, R. H. Liu, W. Q. Kong, J. Liu, Y. Liu, L. Zhou, X. Zhang, S. T. Lee and Z. H. Kang, Carbon quantum dots with photo-generated proton property as efficient visible light controlled acid catalyst, Nanoscale, 2014, 6, 867–873 RSC.
- Y. Z. Han, H. Huang, H. C. Zhang, Y. Liu, X. Han, R. H. Liu, H. T. Li and Z. H. Kang, Carbon Quantum Dots with Photoenhanced Hydrogen-Bond Catalytic Activity in Aldol Condensations, ACS Catal., 2014, 4, 781–787 CrossRef CAS.
- X. Wang, L. Cao, F. Lu, M. J. Meziani, H. Li, G. Qi, B. Zhou, B. A. Harruff, F. Kermarrec and Y.-P. Sun, Photoinduced electron transfers with carbon dots, Chem. Commun., 2009, 3774–3776 RSC.
- H. Li, W. Kong, J. Liu, N. Liu, H. Huang, Y. Liu and Z. Kang, Fluorescent N-doped carbon dots for both cellular imaging and highly-sensitive catechol detection, Carbon, 2015, 91, 66–75 CrossRef CAS.
- D. Qu, M. Zheng, P. Du, Y. Zhou, L. Zhang, D. Li, H. Tan, Z. Zhao, Z. Xie and Z. Sun, Highly luminescent S, N co-doped graphene quantum dots with broad visible absorption bands for visible light photocatalysts, Nanoscale, 2013, 5, 12272–12277 RSC.
- K. A. Fernando, S. Sahu, Y. Liu, W. K. Lewis, E. A. Guliants, A. Jafariyan, P. Wang, C. E. Bunker and Y. P. Sun, Carbon quantum dots and applications in photocatalytic energy conversion, ACS Appl. Mater. Interfaces, 2015, 7, 8363–8376 CrossRef CAS PubMed.
- S.-J. Jeon, T.-W. Kang, J.-M. Ju, M.-J. Kim, J. H. Park, F. Raza, J. Han, H.-R. Lee and J.-H. Kim, Modulating the Photocatalytic Activity of Graphene Quantum Dots via Atomic Tailoring for Highly Enhanced Photocatalysis under Visible Light, Adv. Funct. Mater., 2016, 26, 8211–8219 CrossRef CAS.
- C. Zhu, M. Zhu, Y. Sun, Y. Zhou, H. Huang, Y. Lifshitz, S.-T. Lee, J. Zhong, Y. Liu and Z. Kang, Defects induced efficient overall water splitting on a carbon-based metal free photocatalyst, Appl. Catal., B, 2018, 237, 166–174 CrossRef CAS.
- Y.-Q. Zhang, D.-K. Ma, Y.-G. Zhang, W. Chen and S.-M. Huang, N-doped carbon quantum dots for TiO2-based photocatalysts and dye-sensitized solar cells, Nano Energy, 2013, 2, 545–552 CrossRef CAS.
- K. W. Chu, S. L. Lee, C. J. Chang and L. Liu, Recent Progress of Carbon Dot Precursors and Photocatalysis Applications, Polymers, 2019, 11, 689 CrossRef CAS PubMed.
- C. Zhu, C. a. Liu, Y. Fu, J. Gao, H. Huang, Y. Liu and Z. Kang, Construction of CDs/CdS photocatalysts for stable and efficient hydrogen production in water and seawater, Appl. Catal., B, 2019, 242, 178–185 CrossRef CAS.
- H. Yu, Y. Zhao, C. Zhou, L. Shang, Y. Peng, Y. Cao, L.-Z. Wu, C.-H. Tung and T. Zhang, Carbon quantum dots/TiO2 composites for efficient photocatalytic hydrogen evolution, J. Mater. Chem. A, 2014, 2, 3344–3351 RSC.
- W.-J. Ong, L. K. Putri, Y.-C. Tan, L.-L. Tan, N. Li, Y. H. Ng, X. Wen and S.-P. Chai, Unravelling charge carrier dynamics in protonated g-C3N4 interfaced with carbon nanodots as co-catalysts toward enhanced photocatalytic CO2 reduction: A combined experimental and first-principles DFT study, Nano Res., 2017, 10, 1673–1696 CrossRef CAS.
- Z. Zeng, S. Chen, T. T. Y. Tan and F.-X. Xiao, Graphene quantum dots (GQDs) and its derivatives for multifarious photocatalysis and photoelectrocatalysis, Catal. Today, 2018, 315, 171–183 CrossRef CAS.
- X. Zhang, Y. Liu, S. T. Lee, S. H. Yang and Z. H. Kang, Coupling surface plasmon resonance of gold nanoparticles with slow-photon-effect of TiO2 photonic crystals for synergistically enhanced photoelectrochemical water splitting, Energy Environ. Sci., 2014, 7, 1409–1419 RSC.
- X. Zhang, F. Wang, H. Huang, H. T. Li, X. Han, Y. Liu and Z. H. Kang, Carbon quantum dot sensitized TiO2 nanotube arrays for photoelectrochemical hydrogen generation under visible light, Nanoscale, 2013, 5, 2274–2278 RSC.
- F. Nan, Z. Kang, J. Wang, M. Shen and L. Fang, Carbon quantum dots coated BiVO4 inverse opals for enhanced photoelectrochemical hydrogen generation, Appl. Phys. Lett., 2015, 106, 153901 CrossRef.
- P. Zhang, T. Wang, X. Chang, L. Zhang and J. Gong, Synergistic Cocatalytic Effect of Carbon Nanodots and Co3O4 Nanoclusters for the Photoelectrochemical Water Oxidation on Hematite, Angew. Chem., Int. Ed., 2016, 55, 5851–5855 CrossRef CAS PubMed.
- H. T. Li, R. H. Liu, S. Y. Lian, Y. Liu, H. Huang and Z. H. Kang, Near-infrared light controlled photocatalytic activity of carbon quantum dots for highly selective oxidation reaction, Nanoscale, 2013, 5, 3289–3297 RSC.
- Z. Y. Jiang, X. H. Zhang, W. Sun, D. R. Yang, P. N. Duchesne, Y. G. Gao, Z. Y. Wang, T. J. Yan, Z. M. Yuan, G. H. Yang, X. X. Ji, J. C. Chen, B. B. Huang and G. A. Ozin, Building a Bridge from Papermaking to Solar Fuels, Angew. Chem., Int. Ed., 2019, 58, 14850–14854 CrossRef CAS PubMed.
- F. F. Zhao, G. J. Zhang, H. Dong, W. B. Ji, L. Zhou, H. Li, H. L. Hu, Y. Liu and Z. H. Kang, Transition metal-directed assembly of diverse coordination polymers based on multifunctional ligand 2,4-dichloro-5-sulfamoylbenzoic acid, CrystEngComm, 2013, 15, 8483–8492 RSC.
- X. Wang, Y. Feng, P. Dong and J. Huang, A Mini Review on Carbon Quantum Dots: Preparation, Properties, and Electrocatalytic Application, Front. Chem., 2019, 7, 671 CrossRef PubMed.
- J. Liu, S. Zhao, C. Li, M. Yang, Y. Yang, Y. Liu, Y. Lifshitz, S.-T. Lee and Z. Kang, Carbon Nanodot Surface Modifications Initiate Highly Efficient, Stable Catalysts for Both Oxygen Evolution and Reduction Reactions, Adv. Energy Mater., 2016, 6, 1502039 CrossRef.
- Y. M. Yang, J. Liu, Y. Z. Han, H. Huang, N. Y. Liu, Y. Liu and Z. H. Kang, Porous cobalt, nitrogen-codoped carbon nanostructures from carbon quantum dots and VB12 and their catalytic properties for oxygen reduction, Phys. Chem. Chem. Phys., 2014, 16, 25350–25357 RSC.
- D. Tang, J. Liu, X. Y. Wu, R. H. Liu, X. Han, Y. Z. Han, H. Huang, Y. Liu and Z. H. Kang, Carbon Quantum Dot/NiFe Layered Double-Hydroxide Composite as a Highly Efficient Electrocatalyst for Water Oxidation, ACS Appl. Mater. Interfaces, 2014, 6, 7918–7925 CrossRef CAS PubMed.
- Y. Yang, J. Liu, S. Guo, Y. Liu and Z. Kang, A nickel nanoparticle/carbon quantum dot hybrid as an efficient electrocatalyst for hydrogen evolution under alkaline conditions, J. Mater. Chem. A, 2015, 3, 18598–18604 RSC.
- S. Guo, Y. Yang, N. Liu, S. Qiao, H. Huang, Y. Liu and Z. Kang, One-step synthesis of cobalt, nitrogen-codoped carbon as nonprecious bifunctional electrocatalyst for oxygen reduction and evolution reactions, Sci. Bull., 2016, 61, 68–77 CrossRef CAS.
- Y. Liu, X. Li, Q. Zhang, W. Li, Y. Xie, H. Liu, L. Shang, Z. Liu, Z. Chen and L. Gu, A General Route to Prepare Low-Ruthenium-Content Bimetallic Electrocatalysts for pH-Universal Hydrogen Evolution Reaction by Using Carbon Quantum Dots, Angew. Chem., Int. Ed., 2020, 59, 1718–1726 CrossRef CAS PubMed.
- Y. Liu, Y. Yang, Z. Peng, Z. Liu, Z. Chen, L. Shang, S. Lu and T. Zhang, Self-crosslinking carbon dots loaded ruthenium dots as an efficient and super-stable hydrogen production electrocatalyst at all pH values, Nano Energy, 2019, 65, 104023 CrossRef CAS.
- L. Liu and A. Corma, Metal Catalysts for Heterogeneous Catalysis: From Single Atoms to Nanoclusters and Nanoparticles, Chem. Rev., 2018, 118, 4981–5079 CrossRef CAS PubMed.
- M. Han, S. Zhu, S. Lu, Y. Song, T. Feng, S. Tao, J. Liu and B. Yang, Recent progress on the photocatalysis of carbon dots: Classification, mechanism and applications, Nano Today, 2018, 19, 201–218 CrossRef CAS.
- D. Qu, J. Liu, X. Miao, M. Han, H. Zhang, Z. Cui, S. Sun, Z. Kang, H. Fan and Z. Sun, Peering into water splitting mechanism of g-C3N4-carbon dots metal-free photocatalyst, Appl. Catal., B, 2018, 227, 418–424 CrossRef CAS.
|
This journal is © the Partner Organisations 2020 |
Click here to see how this site uses Cookies. View our privacy policy here.