DOI:
10.1039/D4TB00478G
(Paper)
J. Mater. Chem. B, 2024,
12, 5157-5161
Lysosomal-targeted fluorescent probe based pH regulating reactivity for tracking cysteine dynamics under oxidative stress†
Received
6th March 2024
, Accepted 17th April 2024
First published on 18th April 2024
Abstract
The ability to detect and visualize cellular events and associated biological analytes is essential for the understanding of their physiological and pathological functions. Cysteine (Cys) plays a crucial role in biological systems and lysosomal homeostasis. This puts forward higher requirements on the performance of the probe. Herein, we rationally designed a coumarin-based probe for the reversible, specific, sensitive, and rapid detection of Cys based on pH regulating reactivity. The obtained probe (ECMA) introduces a morpholine moiety to target lysosomes, and α,β-unsaturated-ketone with an electron-withdrawing CN group served as a reversible reaction site for Cys. Importantly, ECMA was successfully applied to the real-time monitoring of Cys dynamics in living cells. Furthermore, cell imaging clearly revealed that exogenous Cys could induce the up-regulation of lysosomal ROS, which provided a powerful tool for investigating the relationship between oxidative stress and lysosomal Cys.
Introduction
As one of the vital organelles, lysosomes can receive signals from other organelles or metabolic pathways within the cell as well as send signals to the cytoplasm and nucleus to regulate specific metabolic processes.1–3 To maintain their physiological function, lysosomes require a relatively reducing environment. It has been reported that intracellular reactive sulfur species (RSS) are important for maintaining such a reducible environment, stabilizing lysosomal membranes and stimulating protein degradation.4–7 However, ROS-induced oxidation that occurs within the lysosomal compartment always disturbs the oxidant-antioxidant balance, which could be a possible indicator of diseased states.8,9 The sulfhydryl (SH) group in cysteine (Cys) is chemically relatively active and strongly nucleophilic under physiological conditions. Studies have suggested that Cys is essential for lysosomes because of its crucial role in regulating the redox state.10–12 Normal intracellular levels of Cys (30–200 μM) maintain the synthesis of various proteins, and abnormal levels of Cys are always associated with diseases.13–16
Cystinosis is the first documented genetic disorder induced by abnormal lysosomal transport function,17,18 wherein lysosomal cystine is rapidly reduced to cysteine.19 Lysosomal cystine transporter protein (cystinosin) dysfunction leads to the abnormal accumulation of cystine and formation of intracellular crystals, which progressively damage cells and organs.20–22 As a novel imaging method, in recent years, fluorescence imaging technology has become the “eye” to reveal the relationship between a signal molecule and the specific disease because of its advantages of visualization and endogenous imaging.23–28 For timely detection of lysosomal cystine transport abnormalities, it is important to develop a reversible fluorescent probe for the real-time detection of the concentration changes of Cys in lysosomes.
Intramolecular charge transfer (ICT), as one of the commonly used sensing mechanisms, has been widely used for developing ratiometric fluorescent probes. Its corresponding sensing behavior is dependent on two different wavelengths as a signal parameter, which effectively reduces the interference of the probe's concentration and instrumental variations.29–31 For a better understanding of the role of Cys in lysosomes, a reversible reaction between the probe and the target is highly demanded.32 Considering that the reactions between α,β-unsaturated ketone and thiols are generally reversible,33,34 we selected α,β-unsaturated ketone as a Cys recognition site and the morpholine group served as a lysosome-targeted group (Fig. S1, ESI†). It was found that the acidic lysosomal environment limited the reactivity of GSH towards the Michael acceptor of ECMA. However, the presence of Cys shows obvious fluorescence responses with physiological concentrations of Cys (0–200 μM) (Scheme 1). Thus, we speculated that ECMA could specifically and reversibly react with Cys, which might be suitable for real-time imaging of lysosomal Cys in living cells. Further confocal fluorescent imaging indicated that ECMA exhibited excellent lysosome-targetable ability and was able to image the fluctuation of Cys in living cells under oxidative stress.
 |
| Scheme 1 Design mechanism and molecular structure of ECMA. | |
Experimental section
Apparatus and materials
The substances and equipment involved in the experiment are in the ESI.†
Synthesis and characterization
The specific synthesis of the probe ECMA is shown in Fig. S1 (ESI†). 1H and 13C NMR spectra of all compounds are shown in the ESI† (Fig. S2–S7).
General fluorescence spectra measurements
Stock solutions (20 mM) of amino acids were prepared using distilled water. The stock solution of ECMA was prepared in DMSO, and the solutions for fluorescence emission spectra were obtained by diluting the stock solution with PBS at pH 5 to a final concentration of 10 μM. The changes in the spectral signal with increasing cysteine (Cys) were recorded.
Cell cytotoxicity assays
Cytotoxicity was assessed by performing a CCK-8 assay with the HeLa cells. HeLa cells were seeded into a group 96-well plate at 2 × 103/well and were cultured at 37 °C and 5% CO2 for 24 h. Different concentrations of ECMA (0, 1, 2.5, 5, 10, 20, and 30 μM) were then added to the wells. After incubation for 6 h, CCK-8 was added to each well, and the plate was incubated for another 1 h. The optical densities at 450 nm were measured.
Cell culture and fluorescence imaging
HeLa cells grown in 1640 medium containing 10% FBS and 1% antibiotics at 37 °C in an atmosphere of 5% CO2. Fluorescence signals were collected for channel 1: 460–520 nm (λex = 405 nm) and channel 2: 542–602 nm (λex = 488 nm).
Results and discussion
Spectral properties of ECMA toward Cys
The photochemical characterization of ECMA and the reaction between the probe and Cys were studied in PBS buffer solution at pH 5. Obviously, as the concentration of Cys increased (0–200 μM), the fluorescence signal at 572 nm slightly diminished (Fig. S8, ESI†), and a new fluorescent emission at 490 nm significantly increased (Fig. 1a). This phenomenon indicated that the sulfhydryl group of Cys can undergo a Michael addition reaction with the α,β-unsaturated ketone of the probe, which resulted in the disruption of the conjugation system of ECMA, the blocking of the ICT process, and the blue shift of the emission peak. By fluorescence titration experiment (Fig. 2a), it is noteworthy that the fluorescence intensity ratio of the probe at 490 nm and 572 nm exhibited a relatively good linear relationship (R2 = 0.971) with increasing Cys concentration. In addition, the corresponding detection limits were calculated (k = 3) to be 0.127 μM for Cys.
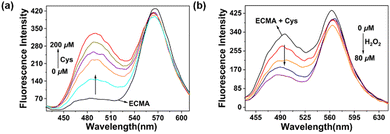 |
| Fig. 1 Spectral properties of ECMA were investigated in PBS (pH = 5). (a) Fluorescence emission titration of ECMA in the presence of different concentrations of Cys (0–200 μM), λex = 400 nm, λem = 490 nm. Slit = 5 nm/5 nm. (b) Spectral response of ECMA with Cys upon the addition of H2O2 (0–80 μM), λex = 400 nm, λem = 490 nm. Slit = 5 nm/5 nm. | |
 |
| Fig. 2 (a) A linear relationship of the fluorescence intensity ratio at 490 nm and 572 nm with Cys concentration in the range of 0–200 μM. (b) Fluorescence kinetics of ECMA (10 μM) with 200 μM of Cys in PBS (pH 5) within 500 s. (c) Change in fluorescence intensity of ECMA (10 μM) at 490 nm upon the addition of various analytes (10 equiv.) in PBS (pH 5), λex = 400 nm. (d) Effect of pH on the fluorescence signal at 490 nm of ECMA. | |
Spectral response of the Cys addition system to hydrogen peroxide
Subsequently, we explored the spectral response of the ECMA and Cys addition products to H2O2. As mentioned above, the addition of H2O2 to the reaction system resulted in a gradual reduction of the fluorescence peak at 490 nm (Fig. 1b) and a considerable increase in the fluorescence intensity at 572 nm (Fig. S9, ESI†). That is when H2O2 is added to the reaction system, it causes the ECMA to recover its original structure and spectral property. In addition, we performed a reversible cyclic response test and found that ECMA remained sensitive after 5 reversible cycles (Fig. S13, ESI†).
Kinetic studies and selectivity studies
In order to study the reaction kinetics, time-dependent fluorescence spectra change of ECMA with Cys was evaluated. When 200 μM of Cys was added to the solution of ECMA (10 μM), the fluorescence intensity enhancement at 490 nm was recorded. As shown in Fig. 2b, the intensity plateaued after 300 s. It indicates that ECMA is able to respond rapidly to Cys. The selectivity of ECMA was investigated in the presence of various amino acids. The ECMA solution was incubated with Cys, other amino acids (Gly, Hcy, Glu, Pro, Arg, Asp, Met, Tyr, Lys, lle, Trp, Ser, Thr, His, Leu, GSH) and H2S for 5 minutes. The fluorescence signals at 490 nm were measured. As depicted in Fig. 2c, only Cys was able to cause significant fluorescence enhancement at 490 nm in ECMA under the excitation at 400 nm, while none of the other analytes significantly interfered with the assay. We found that ECMA recognized glutathione (GSH) in the PBS system (Fig. S10, ESI†) but did not interfere with GSH under acidic conditions at pH = 5. The results demonstrate that the probe ECMA is highly selective toward Cys at pH 5 and exist good anti-interference ability in the complex bio-system.
Reaction mechanism and suitable pH
According to the changes in the fluorescence spectra before and after the addition of Cys, a possible sensing mechanism of ECMA for detecting Cys is proposed in Scheme 1. In order to confirm the sensing mechanism, Cys was added to the DMSO-d6 solution of ECMA, and the corresponding reaction product was studied using ESI-MS. As shown in Fig. S11, we found that when Cys reacted with the ECMA, the dominant peak at m/z = 503.1954 was attributed to [ECMA + Cys]+. Considering the further application in living cells, we measured the influence of pH from 4 to 8 on the fluorescence of ECMA with Cys (Fig. 2d). The emission intensity at 490 nm of ECMA enhanced gradually with the pH value. It is obvious that ECMA was enough to work as a probe in cytoplasm and lysosome.
Fluorescence imaging in living cells
Encouraged by the above results, we investigated the capability of the probe ECMA to detect intracellular Cys. Before the application of ECMA in cellular imaging, we carried out the CCK-8 assay to study its cytotoxicity (Fig. S14, ESI†). After co-incubation with 10 μM ECMA for 6 h, the survival rate of HeLa cells was 96.3%, which showed relatively low cytotoxicity, so we chose 10 μM ECMA for the subsequent cell imaging experiments. In this study, morpholine was considered as a lysosomal targeting group, thus increasing the distribution of ECMA in lysosomes. To study the ability of subcellular localization, the probe ECMA with a commercial Lyso-Tracker Red (LTR) was applied to HeLa cells. As shown in Fig. 3, the orange channel of ECMA overlaid well with the red channel of Lyso-Tracker Red (Pearson's colocalization coefficient was 0.9). The finding confirms that ECMA can specifically aggregate on the lysosome of living cells.
 |
| Fig. 3 The co-localization images of the probe in HeLa cells. (a) Fluorescence image of the probe on the orange channel. (b) Fluorescence image of LTR on the red channel. (c) Merged image and (d) co-localization image (0.90). Orange channel, λex = 488 nm, λem = 572 ± 30 nm; red channel, λex = 561 nm, λem = 620 ± 30 nm. Scale bars = 20 μm. | |
Subsequently, the real-time imaging of ECMA for endogenous and exogenous Cys in HeLa cells was studied. After incubating at 37 °C for 5 min and 20 min with 10 μM ECMA, it was observed that the fluorescence signal in the orange channel largely decreased, while the blue channel was remarkably enhanced (Fig. 4). This result suggests that ECMA is capable of monitoring endogenous Cys.
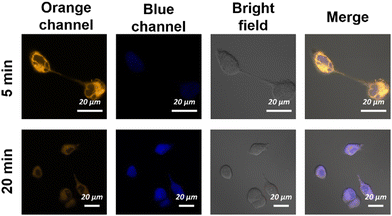 |
| Fig. 4 Real-time fluorescence images of endogenous Cys in HeLa cells. Orange channel: (λem = 542–602 nm, λex = 488 nm). Blue channel: (λem = 460–520 nm, λex = 405 nm). Scale bars = 20 μm. | |
Next, HeLa cells were pretreated with ECMA and then incubated with exogenous Cys (50 μM), as shown in Fig. 5, there was a significant enhancement of the fluorescence signal in the orange channel and disappearance in the blue channel within 6 min. One can notice that this unusual response was reversed with our previous fluorescence titration results. Hence, we speculated that the exogenous Cys may induce cellular oxidative stress, further leading to an up-regulation of ROS, such as H2O2. Besides, the intensity ratio of the orange channel and blue channel tended to decrease after about 6 min. It was probably due to the fact that the probe ECMA reacts with exogenous Cys.
 |
| Fig. 5 (a) Real-time fluorescence images of exogenous Cys in HeLa cells within 10 min and (b) the average fluorescence intensity ratio of the orange channel and the blue channel every 2 min (from 0–10 min). Orange channel: (λem = 542–602 nm, λex = 488 nm). Blue channel: (λem = 460-520 nm, λex = 405 nm). Scale bars = 20 μm. | |
To verify whether the probe ECMA is capable of reversible intracellular recognition of Cys, we pretreated HeLa cells with 10 μM ECMA, then H2O2 was added and co-incubated for another 10 min (Fig. 6). We observed that the fluorescence signal of 542–602 nm in the orange channel gradually increased, while the blue fluorescence of 460–520 nm decreased, and the intensity ratio of the orange channel to the blue channel tended to increase within 10 min. All these suggest that ECMA is capable of reversibly tracking intracellular Cys, and real-time responses to changes in the intracellular redox balance status.
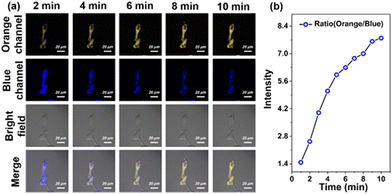 |
| Fig. 6 (a) Fluorescence imaging with the probe in HeLa cells under oxidative stress status mediated by H2O2 and (b) the average fluorescence intensity ratio of the orange channel and the blue channel every 2 min (from 0–10 min). Orange channel: (λem = 542–602 nm, λex = 488 nm). Blue channel: (λem = 460–520 nm, λex = 405 nm). Scale bars = 20 μm. | |
Next, HeLa cells pretreated with the probe were incubated at 37 °C and 39 °C for 10 min, and a significant increase in the fluorescence intensity ratio of the orange channel to that of the blue channel was observed at elevated temperatures, as shown in Fig. 7. The main reason for the significant increase in orange/blue values is due to the oxidative stress caused by the cellular heat stroke. During this process, levels of oxide substances spike (e.g., H2O2), which causes the probe to return to its initial state, showing an enhanced signal in the orange channel and a diminished signal in the blue channel. Therefore, ECMA can dynamically monitor the fluctuations of Cys in living cells under oxidative stress.
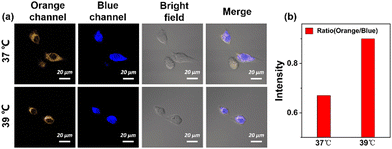 |
| Fig. 7 (a) Confocal images of ECMA (10 μM) with endogenous Cys at 37 °C and 39 °C and (b) the average fluorescence intensity ratio of the orange channel and the blue channel. Orange channel: (λem = 542–602 nm, λex = 488 nm). Blue channel: (λem = 460–520 nm, λex = 405 nm). Scale bars = 20 μm. | |
Conclusions and outlook
To sum up, here we have designed and synthesized a highly selective and reversible fluorescent probe (ECMA) for real-time lysosomal Cys imaging. The construction of ECMA was equipped with α,β-unsaturated ketone as the thiols reaction site, and a morpholine unit as a lysosome-targetable group. It is worth noting that ECMA shows excellent lysosome-targeted ability and capability for real-time imaging of the fluctuation of Cys in the living cells under oxidative stress status. As a result, we believe that the probe ECMA will be a powerful tool for the study of lysosome-related diseases such as cystinosis induced by abnormal Cys.
Conflicts of interest
There are no conflicts to declare.
Acknowledgements
We thank the Program of Graduate Education and Teaching Reformthe of Shanxi (2022YJJG302), the Applied Basic Research Programs of Shanxi (201801D221106, 202203021221228), the Key R&D Project of Lvliang (2023GXYF04), and Scientific Instrument Center of Shanxi University (201512). The authors sincerely thank Suzhou Deyo Bot Advanced Materials Co., Ltd and Professor Wentai Wang for providing support on material characterization.
Notes and references
- M. Abu-Remaileh, G. A. Wyant, C. Kim, N. N. Laqtom, M. Abbasi, S. H. Chan, E. Freinkman and D. M. Sabatini, Science, 2017, 358, 807–813 CrossRef CAS PubMed.
- Q. L. Liu, X. Y. Niu, Y. Zhang, Y. Zhao, K. X. Xie, B. Yang, Q. He, S. Y. Lv and L. Li, Nanoscale, 2020, 12, 13010–13016 RSC.
- X. Zhang, A. D. Rietz, J. Hu, C. Brommesson, X. Y. Wu, K. Uvdal and Z. J. Hu, Sens. Actuators, B, 2021, 345, 130350 CrossRef CAS.
- J. M. Fukuto and A. J. Hobbs, Nitric oxide, 2021, 107, 46–57 CrossRef CAS PubMed.
- K. Surendran, S. P. Vitiello and D. A. Pearce, Pediatr. Nephrol., 2014, 29, 2253–2261 CrossRef PubMed.
- D. Kand, T. Saha, M. Lahiri and P. Talukdar, Org. Biomol. Chem., 2015, 13, 8163–8168 RSC.
- C. Y. Chen, L. Q. Zhou, W. Liu and W. S. Liu, Anal. Chem., 2018, 90, 6138–6143 CrossRef CAS PubMed.
- D. R. Balce, E. R. O. Allan, N. McKenna and R. M. Yates, J. Biol. Chem., 2014, 289, 31891–31904 CrossRef CAS PubMed.
- Y. K. Yue, F. J. Huo, P. Yue, X. M. Meng, J. C. Salamanca, J. O. Escobedo, R. M. Strongin and C. X. Yin, Anal. Chem., 2018, 90, 7018–7024 CrossRef CAS PubMed.
- L. W. He, X. L. Yang, K. X. Xu and W. Y. Lin, Anal. Chem., 2017, 89, 9567–9573 CrossRef CAS PubMed.
- Y. L. Huang, Q. F. Ren, S. Q. Li, Y. Feng, W. Zhang, G. S. Fang, L. K. Li, C. Sun, X. Wang and X. M. Meng, Sens. Actuators, B, 2019, 293, 247–255 CrossRef CAS.
- S. T. Cai, C. Liu, X. J. Jiao, L. C. Zhao and X. S. Zeng, J. Mater. Chem. B, 2020, 8, 2269–2274 RSC.
- L. Y. Niu, Y. Z. Chen, H. R. Zheng, L. Z. Wu, C. H. Tung and Q. Z. Yang, Chem. Soc. Rev., 2015, 44, 6143–6160 RSC.
- Y. K. Yue, F. J. Huo, P. Ning, Y. B. Zhang, J. B. Chao, X. M. Meng and C. X. Yin, J. Am. Chem. Soc., 2017, 139, 3181–3185 CrossRef CAS PubMed.
- B. Sarkar, M. Kulharia and A. K. Mantha, Int. J. Exp. Pathol., 2017, 98, 52–66 CrossRef CAS PubMed.
- R. S. Na, M. Q. Zhu, S. S. Fan, Z. Wang, X. W. Wu, J. Tang, J. Liu, Y. Wang and R. M. Hua, Molecules, 2016, 21, 1023 CrossRef PubMed.
- W. A. Gahl, N. Bashan, F. Tietze, I. Bernardini and J. D. Schulman, Science, 1982, 217, 1263–1265 CrossRef CAS PubMed.
- S. Cherqui and P. J. Courtoy, Nat. Rev. Nephrol., 2017, 13, 115–131 CrossRef CAS PubMed.
- X. Guo, P. Schmiege, T. E. Assafa, R. Wang, Y. Xu, L. Donnelly, M. Fine, X. D. Ni, J. S. Jiang, G. Millhauser, L. Feng and X. C. Li, Cell, 2022, 185, 3739–3752 CrossRef CAS PubMed.
- W. A. Gahl, J. G. Thoene and J. A. Schneider, N. Engl. J. Med., 2002, 347, 111–121 CrossRef PubMed.
- L. R. Rega, E. Polishchuk, S. Montefusco, G. Napolitano, G. Tozzi, J. Z. Zhang, F. Bellomo, A. Taranta, A. Pastore, R. Polishchuk, F. Piemonte, D. L. Medina, S. D. Catz, A. Ballabio and F. Emma, Kidney Int., 2016, 89, 862–873 CrossRef CAS PubMed.
- J. A. Hollywood, A. Przepiorski, R. F. D’Souza, S. Sreebhavan, E. J. Wolvetang, P. T. Harrison, A. J. Davidson and T. M. Holm, J. Am. Soc. Nephrol., 2020, 31, 962–982 CrossRef CAS PubMed.
- Y. J. Wang, H. X. Shen, Z. Li, S. Y. Liao, B. L. Yin, R. Y. Yue, G. Q. Guan, B. D. Chen and G. S. Song, J. Am. Chem. Soc., 2024, 146, 6252–6265 CrossRef CAS PubMed.
- Y. Ma, J. H. Shang, L. H. Liu, M. H. Li, X. Y. Xu, H. Cao, L. Xu, W. Sun, G. S. Song and X. B. Zhang, J. Am. Chem. Soc., 2023, 145, 17881–17891 CrossRef CAS PubMed.
- L. L. Lei, F. R. Yang, X. Meng, L. Xu, P. Liang, Y. Ma, Z. Dong, Y. J. Wang, X. B. Zhang and G. S. Song, J. Am. Chem. Soc., 2023, 145, 24386–24400 CrossRef CAS PubMed.
- C. Lu, Z. Li, N. Wu, D. Y. Lu, X. B. Zhang and G. S. Song, Chem., 2023, 9, 3185–3211 CAS.
- Y. C. Liu, L. L. Teng, X. F. Lou, X. B. Zhang and G. S. Song, J. Am. Chem. Soc., 2023, 145, 5134–5144 CrossRef CAS PubMed.
- N. Wu, C. Lu, Y. J. Wang, L. H. Liu, D. Y. Lu, Y. Zhou, M. He, X. B. Zhang and G. S. Song, Anal. Chem., 2023, 95, 6603–6611 CrossRef CAS PubMed.
- X. Q. Jiang, Y. Yu, J. W. Chen, M. K. Zhao, H. Chen, X. Z. Song, A. J. Matzuk, S. L. Carroll, X. Tan, A. Sizovs, N. H. Cheng, M. C. Wang and J. Wang, ACS Chem. Biol., 2015, 10, 864–874 CrossRef CAS PubMed.
- S. H. Han, H. Zhang, X. X. Yue, J. P. Wang, L. Yang, B. H. Wang and X. Z. Song, Anal. Chem., 2021, 93, 10934–10939 CrossRef CAS PubMed.
- T. Liu, W. J. Zhao, Z. L. Guo, Y. Z. Zhai, W. J. Zhang, X. F. Yang, D. D. Chen and C. X. Yin, Sens. Actuators, B, 2022, 368, 132098 CrossRef CAS.
- W. J. Zhang, F. J. Huo, F. Q. Cheng and C. X. Yin, J. Am. Chem. Soc., 2020, 142, 6324–6331 CrossRef CAS PubMed.
- J. W. Chen, X. Q. Jiang, S. L. Carroll, J. Huang and J. Wang, Org. Lett., 2015, 17, 5978–5981 CrossRef CAS PubMed.
- J. B. Chao, J. M. Zhao, J. P. Jia, Y. B. Zhang, F. J. Huo and C. X. Yin, Spectrochim. Acta, Part A, 2021, 263, 120173 CrossRef CAS PubMed.
|
This journal is © The Royal Society of Chemistry 2024 |
Click here to see how this site uses Cookies. View our privacy policy here.