Nonmonotonic relationship between the degradation of black phosphorus and its bioactivity in suppressing the centrosome polo-like kinase 1†
Received
3rd July 2023
, Accepted 26th November 2023
First published on 29th November 2023
Abstract
Black phosphorus (BP) nanomaterials are promising for biomedical applications owing to their extraordinary biodegradability. Although previous research has demonstrated changes in the physicochemical properties of BP due to degradation, the impact of degradation on BP interactions with biological systems remains unclear. In this study, we designed a set of BP nanomaterials with varying degrees of degradation, including pristine BP nanosheets, BP oxide, BP quantum dots, and phosphates, to investigate their interactions with centrosome polo-like kinase 1 (PLK1), a potential target for BP in inhibiting mitosis. Molecular dynamics simulations identified distinctive modes of interactions, unveiling the nonmonotonic relationship between BP degradation and its effectiveness in suppressing PLK1. Pristine BP can bind to the polo-box domain (PBD) of PLK1, but these interactions were relatively weak and caused minor perturbations in the PBD's structure. When BP undergoes oxidation, it generates phosphate groups that enhance the binding of the PBD. These interactions result in the distortion of the PBD's active pocket through a lever-like mechanism. As degradation progresses further, smaller BP quantum dots are formed. These quantum dots can specifically recognize and occupy the PBD's pocket, thereby disrupting the binding of natural phosphopeptide necessary for activation. The final degradation products of BP include phosphates, which have a negligible effect on the structure and function of the PBD. These findings offer new molecular-level insights into how the bioactivity of BP can be modulated through degradation, which will be instrumental in guiding the development of safer and more efficient biomedical applications.
Environmental significance
Black phosphorus (BP) has become one the most widely explored materials due to its exceptional properties. Although BP is known to be degradable, the effect of degradation on BP interactions with biological systems remains unclear. This knowledge gap is hindering the biomedical application and identification of toxic properties of BP. In this computational effort, a set of BP nanomaterials with an increasing degree of degradation were designed to pinpoint their interactions with the centrosome polo-like kinase 1 (PLK1), a potential target for BP to inhibit mitosis. Molecular dynamics simulations identified the distinctive modes of interactions, and revealed the nonmonotonic relationship between the degradation of BP and its bioactivity in suppressing PLK1. These results demonstrated the great potential of harnessing degradation for controlling the BP's bioactivity, which will guide safer biomedical applications.
|
1 Introduction
Many nanomaterials possess the capability to traverse biological barriers and serve as carriers for drug delivery to target organs.1,2 Beyond passive drug delivery,3,4 the ultrasmall size and reactive surfaces of nanomaterials allow them to behave like functional biomolecules within cells.5,6 These nanomaterials can elicit specific biological effects by interacting with particular biomolecules.7,8 In comparison to many traditional molecular drugs, nanomaterials offer advantages such as greater transportation efficiency, lower effective doses, and reduced side effects.9–11 Nevertheless, the clinical translation of nanomedicine remains challenging due to issues related to poor biocompatibility, slow biodegradation rate, and the substantial accumulation of nanomaterials in the body.12–14 Consequently, the search for novel nanomaterials with favorable biocompatibility and satisfactory biodegradability is of paramount importance for advancing nanomedicine.
Black phosphorus (BP) has emerged as a promising 2D nanomaterial with biodegradability for various biomedical applications,15–17 including bacterial treatment,18–20 drug delivery,21 photothermal therapy,22 and cellular imaging.23 Previous research has established that BP nanomaterials can penetrate various types of cells,24–26 a prerequisite for triggering biological effects within cells. For example, studies have shown that BP nanomaterials can elicit immune responses by activating integrins on the cell membrane.27 Furthermore, BP can directly interact with the cell membrane, extracting significant amounts of phospholipids and reducing cell viability.28 Additionally, the interactions between BP and other functional biomolecules, such as proteins and DNA, have been explored through experimental and computational methods.29,30 However, many previous studies have overlooked the impact of degradation on BP interactions with biological molecules. The final products of BP degradation include phosphates, phosphites, and other PxOy compounds, which can play a role in phosphate-related cellular processes, such as base synthesis and protein phosphorylation.31–33 While the mechanisms of BP degradation under different conditions have been investigated,34,35 the effects of degradation on BP interactions with biological systems remain poorly understood. Generally, BP degradation begins with oxidation, which reduces BP's stability, followed by fragmentation into smaller nanosheets and quantum dots.36,37 Notably, oxidation and exposure to water can produce phosphate groups on the BP's surface and edges.38 These phosphate groups may render degraded BP similar to phosphorylated molecules, which are natural substrates for many kinase proteins involved in regulating the cell cycle, apoptosis, and autophagy.39 Thus, it is conceivable that BP nanomaterials can specifically recognize and interact with protein kinases to induce biological effects, and the inherent bioactivity of BP may be tuned by degradation.
Polo-like kinase 1 (PLK1) is a member of the serine/tyrosine kinase protein family,40 found widely in eukaryotic cells, where it plays vital roles in multiple phases of the cell cycle.41 PLK1 is often overexpressed in human cancer cells, making it a potential target for anti-tumor therapies.42 To inhibit PLK1, molecular drugs mimicking phosphopeptides, the natural activators of PLK1, have been designed. Some of these molecules are capable of binding to the active pocket within the polo-box domain (PBD), a crucial region for PLK1 activation.43,44 However, challenges in developing these molecular drugs for clinical use include issues related to specificity and dose-limiting toxicity.45 Degradable BP presents an attractive candidate as a PLK1 inhibitor due to the phosphate groups generated during degradation. As BP degrades and its size decreases, it reaches a scale similar to that of natural substrate molecules, enhancing its ability to occupy the PBD's pocket. Previous experiments have shown that BP can cause centrosome fragmentation during mitosis by targeting and deactivating PLK1.46 However, the molecular mechanisms underlying interactions between BP and PLK1 have remained unclear, and the relationship between BP degradation and its effectiveness in suppressing PLK1 is yet to be established.
Molecular dynamics (MD) simulations offer a valuable tool for designing nanomaterials of specific properties and elucidating the mechanisms of nano–bio interactions.47–50 In this study, we employed MD simulations to design a series of BP nanomaterials that spanned the degradation continuum, from pristine BP (P-BP) to BP oxide (BP-O), BP quantum dots (BP-QD), and the final product phosphates. Our aim was to investigate the impact of BP degradation on the binding interactions with the PBD of PLK1. Depending on the degree of BP degradation, distinct modes of BP–PBD interactions were identified. Our findings unveiled a nonmonotonic relationship between BP degradation and its bioactivity in suppressing PLK1. Specifically, oxidation and decreasing size were identified as the two primary features of BP degradation that dictated its bioactivity. Given the prevalence of protein kinases activated by phosphorylated molecules,51,52 the degradation-dependent bioactivity of BP elucidated in this study holds potential to be applied to other functional kinases, making our findings of fundamental significance for a wide range of biomedical applications.
2 Materials and methods
2.1 Models and simulation system setup
PLK1 is an essential cell cycle kinase involved in regulating a wide array of biological processes.53 Specifically, it plays a critical role in orchestrating cell mitosis by assisting centrosome maturation and separation, as illustrated in Fig. 1a.54,55 Structurally, PLK1 comprises an N-terminal Ser/Thr kinase domain (KD) and a C-terminal repeat of the polo-box domain (PBD).56,57 During the interphase of mitosis, PLK1 is temporarily inactive as the PBD is bound to KD, inhibiting phosphorylation at Thr-210.58 Upon binding of a phosphopeptide to the PBD's pocket, the KD dissociates from the PBD and is immediately phosphorylated at Thr-210. Subsequently, PLK1 is recruited to various cellular locations to phosphorylate downstream proteins. Overall, the PBD plays a pivotal role in activating PLK1 and is regarded a potential target for suppressing PLK1.59 The structural information of the PBD bound with a phosphopeptide was obtained from the Protein Data Bank (PDB: 6GY2). The PBD consists primarily of two polo-boxes (PB1 and PB2) with the same β6α motifs (Fig. 1b). The phosphopeptide sequence, ATPPTLSSpTVLI, occupies the PBD's active pocket situated between PB1 and PB2. Residues His-538 and Lys-540 in the pocket are crucial for maintaining the stable binding of the phosphopeptide via strong electrostatic interactions with phosphorylated serine or threonine residues (Fig. 1c).60
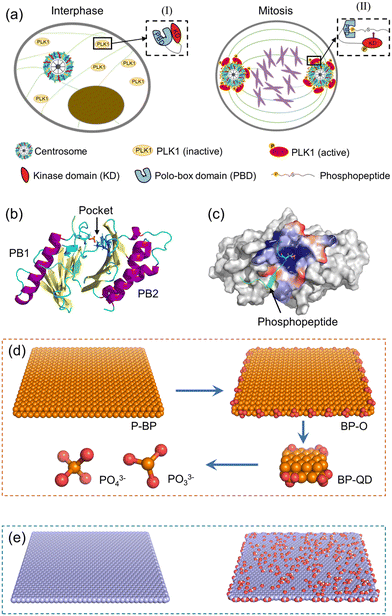 |
| Fig. 1 Simulation system and component models. (a) Schematic of PLK1 activation in mitotic centrosome maturation. (b) Molecular structure of the PBD considered in simulations. The phosphopeptide bound to the PBD is displayed in green with the phosphate group marked in a stick model. Two basic residues namely His-538 and Lys-540 stabilizing the phosphopeptide bound to the PBD's pocket are displayed in blue sticks. (c) The charge distribution near the PBD's pocket. Blue represents positive charge and red represents negative charge. (d) Models of BP degraded from P-BP to BP-O, BP-QD, and final products PO33− and PO43−. Phosphorus and oxygen atoms are displayed in orange and red, respectively. (e) Models of GN and GO nanosheets used for comparison. | |
To elucidate how BP degradation influences its interactions with the PBD, we prepared four representative models of BP with varying degrees of degradation (Fig. 1d). P-BP represents the initial state of BP without degradation. BP-O, on the other hand, simulates the early stage of BP degradation, where oxidation initiates the process. As degradation progresses, the lateral size of BP diminishes. Therefore, we selected BP-QD as a model representing smaller, more degraded BP. At the end of BP degradation, the final products consist of phosphates, phosphites, and other PxOy compounds. We obtained the unit structure of monolayered BP from a previous study, stored at the Cambridge Crystal Data Center (number 1730643).61 Materials Studio 2017 was employed to construct the P-BP nanosheet model with a lateral size of 79.3 Å × 78.6 Å. For the BP-O model, 28 phosphorus atoms at the BP edge were uniformly oxidized to create phosphate groups. BP-QD, significantly smaller in size (12.3 Å × 10 Å), was designed with one phosphate group conjugated at each corner. Interaction parameters for BP nanomaterials were derived from previous density functional theory (DFT) calculations and experimental data.62 These parameters have been widely adopted in studies investigating the interactions between BP and various biomolecules.63–65 The phosphorus atoms of BP were modelled as uncharged Lennard-Jones particles, characterized by a cross section value σPP = 0.330 Å and a depth of potential wall εPP = 0.400 kcal mol−1. Oxygen atoms and phosphorus atoms connected to oxygen were represented as charged Lennard-Jones particles. Atomic charges were computed using the Gaussian 16 software package at the B3LYP/6-311G** level. PO33− and PO43− were considered as two representative models of the final degradation products. The force field parameters for BP are presented in Table S1.†
To compare how BP differs from other 2D nanomaterials in their interactions with the PBD, nanosheets of graphene (GN) and graphene oxide (GO) with the same lateral size as P-BP were also constructed (Fig. 1e). Graphene and its derivatives were among the first 2D nanomaterials to be explored for biomedical applications.66 Additionally, the mechanisms underlying the interactions of GN and GO with biological molecules have been well elucidated.67 GN was constructed using Visual Molecular Dynamics (VMD), with all atoms modeled as neutral sp2 carbon.68 To model GO, 10% of carbon atoms at the basal plane were randomly decorated with hydroxyl and epoxy groups, and 10% of carbon atoms at the edge were randomly grafted with carboxyl groups. Interaction parameters for GN and GO were obtained from previous studies.69 The simulation systems considered in this study are summarized in Table S2.†
2.2 Molecular docking
The ultrasmall size of BP-QD and the presence of phosphate groups could confer properties similar to those of phosphopeptides, allowing BP-QD to potentially bind to the active pocket of the PBD. However, the surface heterogeneity of the PBD makes it challenging to pinpoint the optimal binding site with the lowest free energy using unbiased Molecular Dynamics (MD) simulations. Molecular docking offers an alternative approach that provides a more reliable prediction of protein binding sites by calculating static binding affinity and scores.70 Docking of BP-QD on the PBD was performed using the Molecular Operating Environment (MOE 2019).71 After conducting the docking, eight sets of docking results were obtained, and the top three binding sites with the highest scores were selected as the initial structures for subsequent MD simulations to further refine and optimize conformational changes.
2.3 Molecular dynamics simulation
All-atom MD simulations were performed using the GROMACS software package version 2020.3.72 The OPLS-AA force field was used to describe proteins and phosphopeptides,73 together with the TIP3P water model.74 Simulations of P-BP, BP-O, GN, and GO, as well as their interactions with PBD, were performed in a cubic box of 15 nm × 15 nm × 15 nm. To compare the mode and strength of interactions depending on the initial orientation of the PBD, the PBD was positioned with its pocket respectively adjacent to and distant from the nanosheet surface. Simulations of BP-QD interactions with the PBD were performed in a smaller box of 10 nm × 10 nm × 10 nm. After solvation with water, Cl− and Na+ ions were added to neutralize the system charge and represent the ionic strength of 0.1 M. For each system, the energy was firstly minimized using the steepest descent method to remove bad initial contacts that may impact subsequent equilibrium simulations. Then, a short simulation of 10 ns with the canonical ensemble (constant atom number (N), box volume (V) and temperature (T)) was performed, during which the heavy atoms in protein were restrained to move to allow water to fully dissolve the protein. Using the coordinates and velocities acquired in the previous step, equilibrium simulations of at least 100 ns each were performed with the NPT ensemble. Pressure was kept at P = 1 bar using the Parrinello–Rahman barostat. Temperature was maintained at 310 K by the v-rescale thermostat, with a coupling constant of 2 ps. Nonbonded interactions were truncated at a cutoff of 1.2 nm, and the particle-mesh Ewald summation method was used to deal with the long-range electrostatic interactions. Simulations were performed with a time step of 2 fs, with the neighbor list updated every 10 steps. Periodic boundary conditions were implemented in all three directions.
3 Results
3.1 Comparison of different nanosheets in their interactions with the PBD
To examine the impact of degradation on BP interactions with the PBD, four representative models of BP with varying degrees of degradation were prepared. Surface oxidation, known to be a primary cause of BP degradation,34 is thought to play a central role in this process. An immediate consequence of BP oxidation is the formation of negatively charged phosphate groups at the edges and surface of BP. Given the presence of positively charged residues around the active pocket of the PBD (Fig. 1c), it was hypothesized that BP oxidation would enhance its binding to the PBD. To test this hypothesis, nanosheets of P-BP and BP-O were comparatively studied for their interactions with the PBD. Nanosheets of graphene (GN) and graphene oxide (GO) of the same lateral size as P-BP were considered as typical non-phosphorus 2D nanomaterials for assessing the specificity of interactions between BP and the PBD.
Shown in Fig. 2a–d are the equilibrium states of interactions between the PBD and four different nanosheets. Regardless of oxidation, nanosheets of P-BP, BP-O, GN and GO were capable of binding to the PBD, and residues around the PBD’s pocket formed contacts with these nanosheets. However, it became apparent that the PBD exhibited different conformations on these nanosheets, which appeared to be influenced by the degree of BP oxidation. BP-O and GO, containing negatively charged phosphate and carboxyl groups, exhibited enhanced interactions with the PBD. Residues in contact with the four nanosheets were analyzed (Fig. S1†), and results showed more residues in contact with BP-O and GO compared to their counterparts without oxidation (Fig. 2e). The higher affinity of PBD binding to BP-O compared to P-BP was quantitatively characterized by the lower energy of their interactions and a significantly larger contact surface area (CSA, Fig. 2f). The enhanced binding affinity of the PBD to BP-O was attributed to the lower energy of electrostatic interactions (∼145 kJ mol−1) generated by the phosphate groups and an increase of van der Waals interactions (∼510 kJ mol−1) due to oxidation. In contrast, for GN and GO, oxidation boosted electrostatic interactions by ∼644 kJ mol−1, while weakening van der Waals interactions by ∼290 kJ mol−1. GN and GO displayed similar CSA values to the PBD, suggesting a mild effect of oxidation on the interactions between graphene and the PBD.
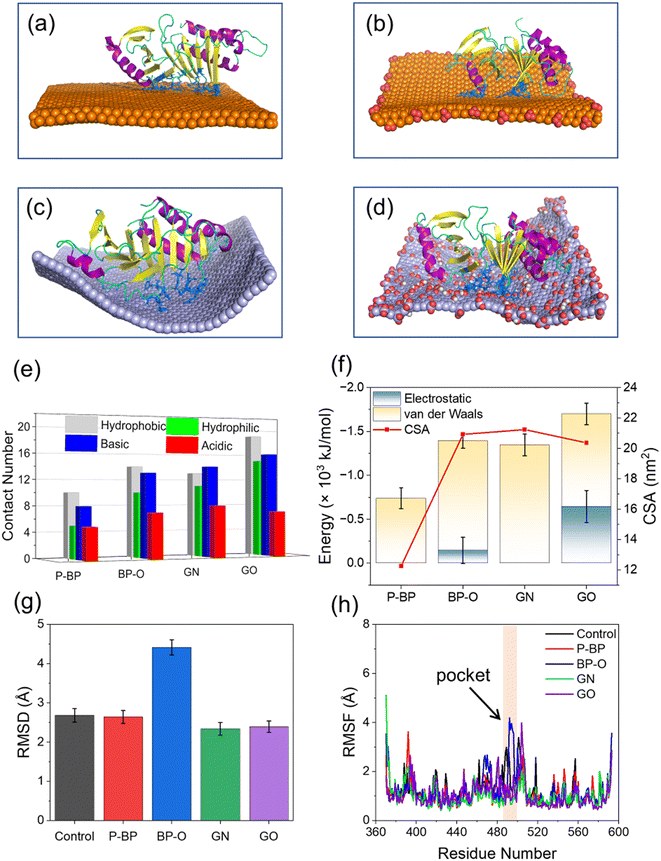 |
| Fig. 2 Comparison of PBD interactions with four different nanosheets. (a–d) Steady states of PBD interactions with P-BP (a), BP-O (b), GN (c), and GO (d). Residues in the PBD's pocket in contact with nanosheets are displayed in the blue stick model. (e) Numbers of different residues in contact with different nanosheets. Residues were defined in contact with nanosheets if the minimal distance between two atoms was less than 0.6 nm. (f) The interaction energy and contact surface area (CSA) between the PBD and four nanosheets. (g) Root-mean-square deviation (RMSD) of the PBD after interactions with four different nanosheets. (h) Root-mean-square fluctuation (RMSF) for residues of the PBD during interactions with four nanosheets. Pocket residues with higher RMSF induced by BP-O are labeled by a yellow band. | |
No significant changes were observed in the secondary structure of the PBD during interactions with the four nanosheets (Fig. S2†). To assess tertiary structural changes, we calculated the root-mean-square deviation (RMSD) of the PBD (Fig. 2g). It is evident that nanosheets of P-BP, GN, and GO induced no substantial changes in the tertiary structure of the PBD. Although GO displayed higher binding affinity to the PBD (Fig. 2f), it did not increase the RMSD of the PBD, possibly due to reduced hydrophobic interactions resulting from oxidation. In addition, nanosheets of GN and GO deformed to establish more contacts with the PBD (Fig. 2c–e). This deformation likely restrained conformational changes and the mobility of the PBD on the GO surface. In contrast, a significant increase in the PBD's RMSD was observed after interactions with BP-O, indicating a noticeable conformational change. The root-mean-square fluctuation (RMSF), calculated for each residue of the PBD, revealed increased fluctuations in the pocket region during interactions with BP-O (Fig. 2h). Given the significance of the PBD's pocket in recognizing phosphopeptides, a critical determinant in whether PLK1 can be activated and appropriately localized to facilitate centrosome maturation and separation,75 this conformational change induced by BP-O could potentially lead to the suppression of PLK1's activity.
3.2 BP-O induces distortion of the PBD's pocket through a lever-like mechanism
Processes of PBD interactions with the four nanosheets were comparatively analyzed (Fig. S3†). The binding of the PBD to P-BP and GN nanosheets was primarily driven by van der Waals interactions (Fig. 2f). GN exhibited stronger binding compared to P-BP due to its higher hydrophobicity and its ability to deform and wrap around the PBD (Fig. S3a and c†).76 The oxidation of graphene introduced carboxyl groups, which enhanced electrostatic interactions with the PBD, while it resulted in a weakening of van der Waals interactions due to reduced surface hydrophobicity. The posture and conformation of the PBD remained nearly unchanged during interactions with nanosheets of P-BP, GN, and GO (Fig. S3a, c and d†). Differently for BP-O, the PBD exhibited a distinct behavior, including migration along the BP-O surface, accompanied by marked conformational changes (Fig. S3b and Video S1†).
In the early stage (t < 140 ns), the PBD attached its pocket region on the planar surface of BP-O and gradually moved toward the BP-O edge, which is rich in phosphate groups (Fig. 3a). At t = 142 ns, residues Arg-392 and Arg-396 in PB2 flipped downward to form electrostatic interactions with the phosphate groups at the BP-O edge (Fig. 3a and S4†). As time progressed, more residues in PB2 were drawn down to establish favorable contacts with BP-O (t = 145 ns). As the PBD's pocket locates between the PB2 and PB1 domains, this flipping movement generated a force that extruded the PBD's pocket from the BP-O edge back to the plane (t = 185 ns). Due to the relative rigidity of the entire protein, a lever-like effect was formed, provoking normal intra-molecular interactions and inducing distortion of the pocket (t = 300 ns, Fig. 3b and S5†). This local structural change was also reflected in the higher RMSD of the whole protein and the pocket region (Fig. S6a and b†). The solvent accessible surface area (SASA) of the PBD's pocket, reflecting the degree of solvent exposure, increased due to this distortion (Fig. S6c†). A comparison of the crystal structure of the PBD with that bound to BP-O revealed a clear change in the PBD's pocket (Fig. S7†). To quantify the degree of pocket distortion, two distances between diagonal residues in the PBD's pocket were calculated (Fig. 3c and d). Compared to the protein in equilibrium, PBD interactions with BP-O caused a significant increase in one distance (Fig. 3c), while the other distance remained nearly unchanged (Fig. 3d). This directional elongation of the pocket was presumed to interfere with the normal binding of phosphopeptides. In contrast, the other nanosheets of P-BP, GN, and GO induced no apparent changes in the pocket structure (Fig. 3c and d and S8†).
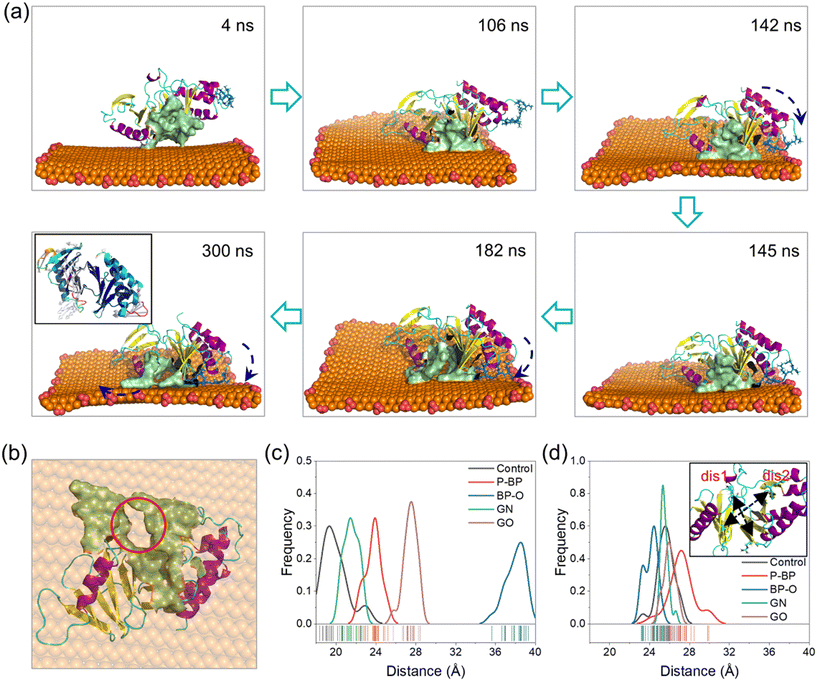 |
| Fig. 3 Distortion of the PBD's pocket induced by interactions with the BP-O nanosheet. (a) Side view of typical snapshots illustrating the conformational change of the PBD on the BP-O nanosheet. Residues in the PBD's pocket are colored in mustard. Residues in PB2 pulled in contact with phosphate groups at the BP-O edge are displayed in a blue stick model. Arrows indicate the rolling motion of the PBD on the BP-O nanosheet by a lever-like effect. The inset of the final frame is a RMSF-vector diagram colored by the values of the b-factor, showing flexibility and movement of residues. White arrows indicate the direction of residues' relative movement. (b) Bottom view of the final snapshot illustrating distortion of the PBD's pocket induced by interactions with BP-O. BP-O is set semi-transparent for viewing the distortion of the PBD's pocket (red circle). (c and d) Frequency distributions of two distances between diagonal residues in the PBD's pocket characterizing the degree of the pocket distortion. The inset of (d) illustrates calculation of the two distances. “dis1” defines the distance between residues Lys-492 and Arg-516 (c), and “dis2” is the distance between Val-415 and Lys-556 (d). | |
The simulation results above strongly suggest that capturing positively charged residues in PB2 by phosphate groups on BP-O represents a critical initiating event that ultimately leads to the distortion of the PBD's pocket. To validate this lever-like mechanism, we further conducted two parallel simulations by positioning the PBD near the phosphate groups at the BP-O edge (Fig. S9†). As the PBD reached the BP-O edge, with residues Arg-392 and Arg-396 captured by the phosphate groups, the PBD's pocket was pulled back and forth, disrupting intra-molecular interactions and, in the end, inducing distortion of the PBD's pocket through a lever-like mechanism. In contrast, PBD interactions with P-BP, in parallel simulations, consistently demonstrated stable binding without any apparent changes in secondary and tertiary structures (Fig. S10 and S11†).
Proteins are heterogeneous in surface structure and properties, making their interactions with nanomaterials influenced by the orientation of proteins getting initial contact with nanomaterials.77,78 To understand the effect of this orientation on PBD interactions with BP, we positioned the PBD with its pocket region distanced from the nanosheets (Fig. S12a and b†). In this reverse orientation, the PBD exhibited lower energy interactions with P-BP (Fig. S12c†), potentially due to the abundance of hydrophobic residues in this region (Fig. S12d†). In contrast, for BP-O, which contains negatively charged phosphate groups, the pocket recognition was more energetically favorable due to electrostatic interactions formed by the positively charged residues surrounding the pocket (Fig. S12c and e†). No apparent changes in the structure of the PBD was observed when the pocket did not come into contact with P-BP and BP-O (Fig. S12f–h†).
3.3 BP-QD occupies the PBD's pocket to inhibit binding of phosphopeptide
Another important feature of BP degradation is the reduction in size until it is fully degraded. When the size of degraded BP approaches the scale range of natural substrate molecules, new patterns of BP interactions with the PBD were anticipated. Specifically, BP-QD can be regarded as an important intermediate of BP degradation. Unlike the larger nanosheet of BP-O, which induced strong interactions leading to distortion of the PBD's pocket through a lever-like mechanism, ultrasmall BP-QD may bind to a specific site of the PBD. Therefore, identifying the optimal binding site is a crucial step in understanding the interactions between BP-QD and the PBD. However, exploring the entire heterogeneous surface of a protein to locate the optimal site can be challenging and time-consuming with traditional MD simulations. Consequently, molecular docking simulations were conducted to initially predict potential binding sites for BP-QD. By consolidating adjacent docking sites that shared residues in contact with BP-QD, three regions of PBD with high affinity to BP-QD were identified (Fig. 4a). In particular, the first region, covering most of the residues in the PBD's pocket, exhibited the highest affinity with a score of −109.5 kcal mol−1 to BP-QD. The other two sites were located further away from the pocket and displayed lower affinity to BP-QD.
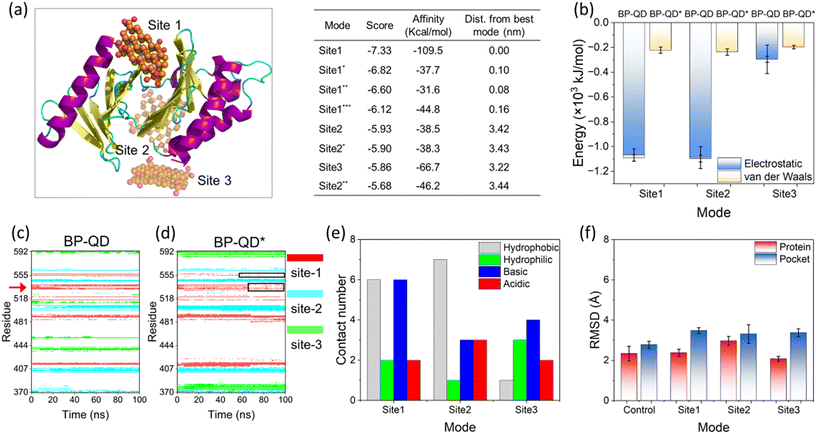 |
| Fig. 4 Specific binding of BP-QD to the PBD's pocket. (a) Top three sites of BP-QD binding to the PBD predicted by molecular docking. Score and binding affinity of these identified regions are given on the right panel. (b) Energy of interactions between BP-QD/BP-QD* and PBD at three sites calculated by MD simulations. (c and d) Time evolutions of residues in contact with BP-QD (c) and BP-QD* (d). Data for top three sites are merged in one figure and displayed in red, blue, and green colors. Red arrow in dictate two residues namely His-538 and Lys-540 in contact with BP-QD. These two residues lose their contacts with BP-QD* as indicated by the black frames. (e) Numbers of different residues in contact with BP-QD at the top three sites. (f) RMSD of the entire PBD and the PBD's pocket after interactions with BP-QD in three different sites. | |
The docking solutions were used as the initial structures for MD simulations to further optimize the interactions and account for conformational flexibility. For each system, three parallel simulations were performed, and the results consistently showed that BP-QD can stably bind to the sites identified previously (Fig. S13†). The interaction energies at these three different sites were calculated (Fig. 4b). As expected, the binding affinity of BP-QD to the PBD was enhanced after equilibration using a flexible protein model. Sites 1 and 2 exhibited similar affinity to BP-QD (−1100 kJ mol−1), with electrostatic interactions being the major driving force. The binding of BP-QD to site 3 was less favorable, with a lower affinity of −300 kJ mol−1. Phosphate groups on BP-QD were found to play a dominant role in PBD recognition and stable binding. To confirm it, all phosphate groups were artificially removed (BP-QD*), and the interaction energies, especially at sites 1 and 2, were significantly reduced (Fig. 4b). Binding of BP-QD* to the three different sites became indistinguishable. The attenuation of BP-QD's binding to the PBD after the removal of phosphate groups was also evident in the contact map, where the binding became less stable (Fig. 4c and d).
Of particular concern was the PBD's pocket being vital for PLK1 activation. His-538 and Lys-540 are key residues for the binding of phosphopeptide to the pocket.57 These two residues were found to remain in contact with BP-QD throughout the simulation period (Fig. 4c and S14 and Video S2†). When the phosphate groups of BP-QD were removed, the binding of BP-QD* to the PBD's pocket became less favorable, and the two critical residues gradually lost their contact with BP-QD* (Fig. 4d and S14†). Although site 2 showed a similar affinity to BP-QD, site 1, which covered the PBD's pocket residues, appeared more promising as the optimal site for BP-QD recognition (Fig. 4e).
No apparent changes were observed in the structure of the PBD induced by interactions with BP-QD or BP-QD*, regardless of the binding site (Fig. 4f, S15 and S16†). Besides, the geometry of the PBD's pocket remained intact during interactions with BP-QD and BP-QD* (Fig. S17 and S18†). Nevertheless, the ultrasmall size of BP-QD allowed it to occupy the active pocket, potentially hindering the normal recognition and binding of the natural phosphopeptide. Fig. 5a depicts the equilibrium structure of the PBD with its pocket bound to a phosphopeptide. Residues around the pocket were colored according to the probability of contact with the phosphopeptide. Through comparison with the contact mode between PBD and BP-QD (Fig. 5b), it can be clearly observed that some important residues such as His-538, Lys-540, and Arg-557 in the pocket were shared by both BP-QD and the phosphopeptide. This indicates that BP-QD may compete with the phosphopeptide for binding to the pocket, disrupting the normal binding of the phosphopeptide. When the PBD's pocket was pre-occupied by BP-QD with a high affinity, the normal recognition of the PBD by the natural phosphopeptide could be hindered, potentially inhibiting PLK1 activation.
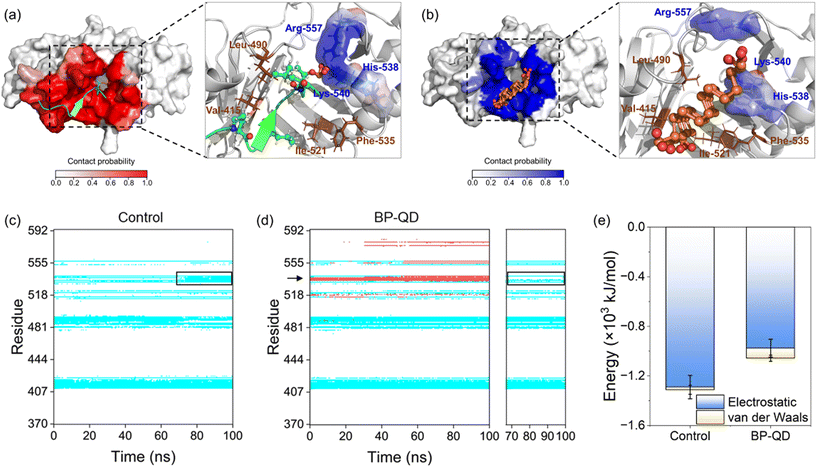 |
| Fig. 5 Competition between BP-QD and phosphopeptide in their binding to the PBD's pocket. (a) Equilibrium structure of the PBD bound to a phosphopeptide colored in green. Residues around the pocket were colored in red according to the probability of contact with the phosphopeptide. Several residues in direct contact with the phosphopeptide are labeled in the right panel. (b) Probability of residues in the PBD's pocket in contact with BP-QD. Residues in contact with BP-QD are labeled in the right panel. (c) Time evolutions of residues in contact with phosphopeptide. The black frame indicates four basic residues in the pocket acquiring favorable contacts with the phosphopeptide. (d) Time evolution of residues in contact with BP-QD with a phosphopeptide bound to the PBD's pocket. Red and blue bands are residues in contact with BP-QD and phosphopeptide, respectively. Under perturbation of BP-QD, fewer residues in the PBD's pocket remain in contact with the phosphopeptide, as indicated by the black frame in the right panel. (e) Energies of interactions between the phosphopeptide and PBD before and after interactions with BP-QD. | |
Another important scenario involves BP-QD binding to the PBD when the pocket is already occupied by a phosphopeptide. A 100 ns simulation demonstrated that the phosphopeptide can tightly bind to the PBD's pocket (Fig. 5c). In the pocket, three basic residues, namely His-538, Lys-540, and Arg-557, formed electrostatic interactions with the phosphate group of the phosphopeptide (Fig. S19a†). Notably, BP-QD can still bind to the region near the pocket occupied by the phosphopeptide (Fig. 5d and S19b†). As the simulation proceeded, BP-QD gradually inserted deep into the PBD's pocket and competed for residues with phosphopeptide within the pocket. As a result of this competition, some residues (e.g., His-538) were observed to lose their contact with the phosphopeptide (Fig. 5d and S19b†). By the end of simulation, the strength of electrostatic interactions between the phosphopeptide and PBD was reduced by approximately 310 kJ mol−1 (Fig. 5e). Given the abundance of positively charged residues in the PBD's pocket, BP-QD appeared to have an advantage in pocket recognition due to the enrichment of phosphate groups. These findings suggested that the phosphopeptide may dissociate from the PBD's pocket due to competition with BP-QD.
3.4 Phosphate ions fail to recognize the PBD's pocket
The final products of BP degradation are quite complex, including but not limited to phosphate, phosphite, and other PxOy compounds.34 It is known that these compounds are metabolizable in vivo,79–81 which suggests the potential biodegradability of BP nanomaterials. However, the biodegradability of BP nanomaterials can have both advantages and disadvantages in biomedical applications. On the one hand, degradation can help prevent the massive accumulation of BP nanomaterials in the body, thereby improving their biocompatibility. On the other hand, rapid degradation may reduce the effectiveness of BP treatment, necessitating frequent dosing. The excess phosphates generated by BP degradation could potentially lead to side effects related to hyperphosphatemia.82 Furthermore, other products, such as superoxide anions and hydroxyl radicals, may also be generated through the oxidative degradation of BP.83 It is crucial to investigate whether the final products of BP degradation are effective in specifically binding to the PBD's pocket for PLK1 deactivation. Among the complex compounds formed during BP degradation, PO33− and PO43− have been identified as the primary products of BP degradation in oxygenated water, constituting 48% and 39% of the products, respectively.84 These compounds, PO33− and PO43−, were thus selected as representative models of the final products of BP degradation for further investigation.
PBD interactions with PO33− and PO43− ions were simulated under the same conditions as in previous simulations. A total of 30 phosphate ions were used to ensure the equivalent phosphorus amount to that of BP-QD for easy comparison. Time sequences of typical snapshots illustrated that several PO33− and PO43− ions could occasionally bind to the PBD's pocket (Fig. 6a and b), but their interactions were weak and experienced drastic fluctuations (Fig. S20†). As a result, there were frequent associations and dissociations of phosphate ions throughout the simulation period (Fig. 6c and S21 and Video S3†). This behavior contrasted with BP-QD, which demonstrated the ability to specifically recognize and stably bind to the PBD's pocket (Fig. 4 and 5). Energetically, the interactions of the PBD with PO33− and PO43− ions were considerably weaker than those with BP-QD (Fig. 6d). In this context, no discernible structural changes in the protein were observed over the finite simulation period (Fig. S22 and S23†). Furthermore, when a phosphopeptide was initially bound to the PBD's pocket, subsequent interactions with phosphate ions did not impact the binding state and energy (Fig. S24†). Consequently, the primary products namely PO33− and PO43− were found to be largely ineffective in suppressing PLK1. Similarly, hypophosphite, another product of BP degradation,85 may also be inactive in suppressing PLK1. It is worth noting that other products of BP degradation, such as hydroxyl radicals, might influence the structure and function of the PBD by inducing protein oxidation.
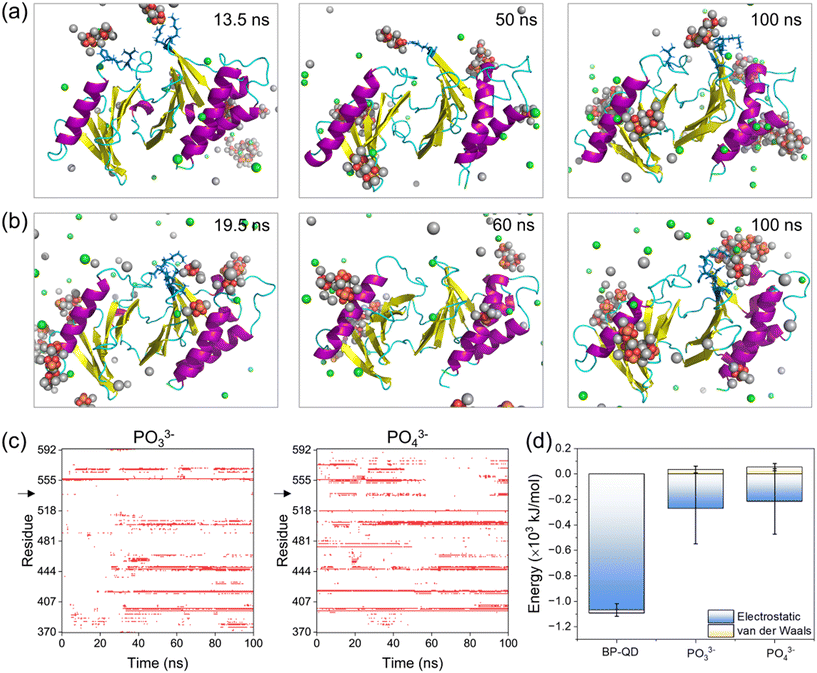 |
| Fig. 6 Non-specific binding of phosphate ions to the PBD. (a and b) Typical snapshots of PBD interactions with PO33− (a) and PO43− (b) ions. Cl− and Na+ ions are displayed in green and silver spheres, respectively. Residues in the PBD's pocket occasionally bound to phosphate ions are displayed in a blue stick model. (c) Time evolutions of residues in contact with PO33− and PO43− ions. Black arrows point to critical basic residues in the pocket that were not recognized by phosphate ions. (d) Energies of PBD interactions with BP-QD, PO33− and PO43−. | |
4 Discussion
Nanomaterials developed for biomedical applications were mostly based on their drug delivery and controlled release capacities, often overlooking their intrinsic bioactivities. In fact, the unique properties of nanomaterials, including their ultrasmall size and reactive surfaces, enable them to elicit specific biological effects through interactions with target biomolecules. Understanding how nanomaterials interact with biological systems is crucial for the development of nanomedicine and the assessment of nanotoxicity. Nevertheless, nanomaterials are subject to continuous transformations, leading to changes in their properties. This dynamic nature makes it challenging to identify the molecular targets of nanomaterials and predict their biological outcomes. Importantly, harnessing degradation as a means to tune the bioactivity of nanomaterials has been rarely considered in previous studies. For degradable BP nanomaterials, cellular uptake is a fundamental prerequisite for BP to express the bioactivity within cells. Previous experiments have demonstrated that BP nanomaterials can be internalized by cells through endocytosis,86 and within the acid environment of lysosomes, BP can undergo degradation, releasing various products that can trigger biological effects through interactions with specific biomolecules. A recent experimental study unveiled the intrinsic bioactivity of BP in mitotic suppression, identifying centrosome PLK1 as the molecular target for BP.46 Given the understanding that the recruitment of PLK1 to mitotic centrosomes for activation is stimulated by the specific binding of a phosphopeptide to the PBD's pocket, it can be inferred that BP's bioactivity in suppressing PLK1 can be modulated by degradation from two key aspects. One aspect is oxidation, which generates phosphate groups that may enhance BP interactions with the PBD, while the other is the decreasing size, allowing degraded BP to occupy the PBD's pocket.
The pathway of BP degradation, as elucidated by DFT calculations,85,87 guided the examination of interactions between the PBD and various models representing different states of BP degradation, including P-BP, BP-O, BP-QD and phosphate ions. MD simulations unveiled degradation-dependent bioactivity of BP in suppressing PLK1, as illustrated in Fig. 7. Before degradation, P-BP exhibited mild interactions with the PBD, binding to it without causing significant structural changes in the protein (stage I). For oxidized BP, oxygen may occupy both edge and surface sites and the formed phosphate groups can enhance the BP interactions with the PBD. Besides, various defects can be generated during the fabrication and degradation of BP, and can further tune the interactions.88 The heterogeneous distribution of defects and oxygen on BP created an asymmetric force, acting as a lever-like mechanism on the PBD. This disrupted intra-molecular interactions and induced distortion of the PBD's pocket (stage II). Larger sheets of BP may also experience distortion to form negatively curved surfaces,89 which was previously found to enhance the dispersive interactions with various biomolecules.90 As the degradation progressed to produce smaller BP-QD, a new pattern of interactions emerged. The ultrasmall size and phosphate groups on BP-QD allowed them to specifically bind to the PBD's pocket. This pre-occupancy hindered the recognition of phosphopeptides by the PBD's pocket (stage III). BP-QD outperformed phosphopeptide in binding to the PBD's pocket due to the abundance of phosphate groups. Consequently, even when the PBD's pocket has been occupied by a phosphopeptide, subsequent interactions with BP-QD could competitively replace the phosphopeptide, suppressing PLK1 activation (stage IV). The final degradation products, phosphates, showed negligible effects on the PBD's structure and function, as they were unable to recognize the PBD's pocket (stage V).
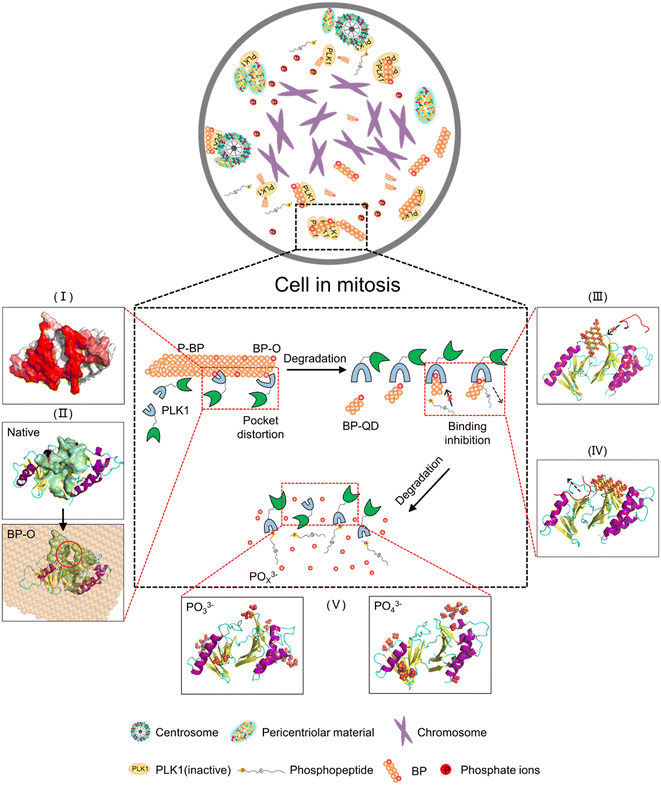 |
| Fig. 7 Schematic of the degradation dependent bioactivity of BP in suppressing PLK1. The top bubble illustrates a cell experiencing mitosis initiated by phosphorylation of PLK1. Inside the cell, BP nanomaterials can experience degradation, with different products exhibiting different activities in suppressing PLK1. (I) Pristine P-BP exhibited mild interactions with PBD. (II) Oxidized BP induced distortion of the PBD's pocket. (III and IV) BP-QDs specifically bind to the PBD's pocket to interfere with binding of phosphopeptide. | |
The bioactivity of BP in suppressing PLK1 can be applied to other kinases that are essential in regulating various biological processes through phosphorylation cascades.91 Harnessing BP degradation to modulate its bioactivity opens opportunities for a wide range of biomedical applications. Various strategies, such as capping layers,92 covalent and noncovalent functionalization,93,94 solvent passivation,95 and polymer-based protection,96 have been developed to control BP degradation. These strategies may cause changes in BP's physicochemical properties and activity that should be carefully considered. Additionally, the size and shape effects of BP nanomaterials should be explored further for better matching the geometry of target biomolecules. Furthermore, any biomedical application of nanomaterials should consider the influence of protein coronas formed on nanomaterials upon entering biological systems. While degraded BP nanomaterials have demonstrated their bioactivity in suppressing PLK1, the effects of protein coronas on BP degradation and bioactivity remain to be fully understood.97
5 Conclusions
In summary, by means of MD simulation, we have designed a set of BP nanomaterials with various degrees of degradation to unveil the nonmonotonic relationship between BP degradation and its bioactivity in suppressing PLK1. In comparison to P-BP, which exhibited weak and nonspecific interactions that minimally impacted the structure of the PBD, oxidation of BP generated phosphate groups that enhanced the binding affinity of BP-O to the PBD's pocket. To reduce the interaction free energy, the PBD underwent flipping on the surface of BP-O with heterogeneous distribution of phosphate groups. This resulted in a lever-like effect, inducing distortion of the PBD's pocket. As the size of degraded BP decreased, eventually reaching the scale range of natural substrate molecules, a new pattern of interactions emerged. Specifically, BP-QD demonstrated specific binding to the PBD's pocket. The occupation of the pocket by BP-QD interfered with normal recognition of phosphopeptides or competitively displaced phosphopeptides already bound to the pocket. Finally, as the ultimate product of BP degradation, phosphate ions were unable to effectively recognize the pocket, thus exerting minimal influence on the structure and function of the PBD. Overall, this study highlights the potential of harnessing degradation to control the bioactivity of BP, offering insights that can guide the development of safer and more effective nanomedicine.
Author contributions
Chaofan Deng: investigation, methodology, writing – original draft. Yanhui Dai: methodology, writing – review & editing. Luyao Ren: methodology. Lin Yang: writing – review & editing. Xia Liu: writing – review & editing. Jian Zhao: supervision, writing – review & editing. Tongtao Yue: conceptualization, methodology, writing – review & editing, supervision.
Conflicts of interest
There are no conflicts of interest to declare.
Acknowledgements
This work was supported by the National Natural Science Foundation of China (Grant No. 32371459, 31871012, 42192572, 42277200), the Natural Science Foundation of Shandong Province (ZR2021YQ23, ZR2020QD128), the Fundamental Research Funds for the Central Universities (202112008), and Guangdong Provincial Key Laboratory of Environmental Pollution and Health (No. GZKLEPH201802).
References
- R. H. Fang, W. Gao and L. Zhang, Targeting drugs to tumours using cell membrane-coated nanoparticles, Nat. Rev. Clin. Oncol., 2023, 20, 33–48 CrossRef PubMed.
- L. Wang, Y. Shi, J. Jiang, C. Li, H. Zhang, X. Zhang, T. Jiang, L. Wang, Y. Wang and L. Feng, Micro-Nanocarriers Based Drug Delivery Technology for Blood-Brain Barrier Crossing and Brain Tumor Targeting Therapy, Small, 2022, 18, 2203678 CrossRef PubMed.
- S. A. Dilliard and D. J. Siegwart, Passive, active and endogenous organ-targeted lipid and polymer nanoparticles for delivery of genetic drugs, Nat. Rev. Mater., 2023, 8, 282–300 CrossRef PubMed.
- D. Zhang, S. Tian, Y. Liu, M. Zheng, X. Yang, Y. Zou, B. Shi and L. Luo, Near infrared-activatable biomimetic nanogels enabling deep tumor drug penetration inhibit orthotopic glioblastoma, Nat. Commun., 2022, 13, 6835 CrossRef PubMed.
- X. Fu, X. Yu, J. Jiang, J. Yang, L. Chen, Z. Yang and C. Yu, Small molecule-assisted assembly of multifunctional ceria nanozymes for synergistic treatment of atherosclerosis, Nat. Commun., 2022, 13, 6528 CrossRef PubMed.
- Y. Zhu, R. Zhao, L. Feng, C. Wang, S. Dong, M. V. Zyuzin, A. Timin, N. Hu, B. Liu and P. Yang, Dual Nanozyme-Driven PtSn Bimetallic Nanoclusters for Metal-Enhanced Tumor Photothermal and Catalytic Therapy, ACS Nano, 2023, 17, 6833–6848 CrossRef PubMed.
- X. Liu, Q. Zhang, W. Knoll, B. Liedberg and Y. Wang, Rational Design of Functional Peptide-Gold Hybrid Nanomaterials for Molecular Interactions, Adv. Mater., 2020, 32, 2000866 CrossRef PubMed.
- Q. Feng, N. Wang, X. Zhang, Y. Mei, R. Fu, J. Chen, X. Yuan, S. Yang, Z. Zhang, H. Zhao and L. Wang, Nanoparticle cluster depolymerizes and removes amyloid fibrils for Alzheimer's disease treatment, Nano Today, 2023, 48, 101756 CrossRef.
- S. Ding, A. I. Khan, X. Cai, Y. Song, Z. Lyu, D. Du, P. Dutta and Y. Lin, Overcoming blood-brain barrier transport: Advances in nanoparticle-based drug delivery strategies, Mater. Today, 2020, 37, 112–125 CrossRef PubMed.
- Z. He, F. Ye, C. Zhang, J. Fan, Z. Du, W. Zhao, Q. Yuan, W. Niu, F. Gao, B. He, P. Cao, L. Zhao, X. Gao, X. Gao, B. Sun, Y. Dong, J. Zhao, J. Qi, X. J. Liang, H. Jiang, Y. Gong, W. Tan and X. Gao, A comparison of Remdesivir versus gold cluster in COVID-19 animal model: A better therapeutic outcome of gold cluster, Nano Today, 2022, 44, 101468 CrossRef CAS PubMed.
- L. Liu, F. Hu, H. Wang, X. Wu, A. S. Eltahan, S. Stanford, N. Bottini, H. Xiao, M. Bottini, W. Guo and X. Liang, Secreted Protein Acidic and Rich in Cysteine Mediated Biomimetic Delivery of Methotrexate by Albumin-Based Nanomedicines for Rheumatoid Arthritis Therapy, ACS Nano, 2019, 13, 5036–5048 CrossRef CAS PubMed.
- R. Foulkes, E. Man, J. Thind, S. Yeung, A. Joy and C. Hoskins, The regulation of nanomaterials and nanomedicines for clinical application: current and future perspectives, Biomater. Sci., 2020, 8, 4653–4664 RSC.
- R. Mohammadpour, M. A. Dobrovolskaia, D. L. Cheney, K. F. Greish and H. Ghandehari, Subchronic and chronic toxicity evaluation of inorganic nanoparticles for delivery applications, Adv. Drug Delivery Rev., 2019, 144, 112–132 CrossRef CAS PubMed.
- G. Jamalipour Soufi and S. Iravani, Eco-friendly and sustainable synthesis of biocompatible nanomaterials for diagnostic imaging: current challenges and future perspectives, Green Chem., 2020, 22, 2662–2687 RSC.
- J. R. Choi, K. W. Yong, J. Y. Choi, A. Nilghaz, Y. Lin, J. Xu and X. Lu, Black Phosphorus and its Biomedical Applications, Theranostics, 2018, 8, 1005–1026 CrossRef CAS PubMed.
- S. Gao, Y. Wang, J. Sun and Z. Zhang, Recent development in black phosphorus nanomaterials for anticancer applications, Front. Biomater. Sci., 2023, 2, 1172524 CrossRef.
- X. Zhang, I. S. Donskyi, W. Tang, S. Deng, D. Liu, S. Zhang, Q. Zhao and B. Xing, Biological Effects of Black Phosphorus Nanomaterials on Mammalian Cells and Animals, Angew. Chem., Int. Ed., 2023, 62, e202213336 CrossRef CAS.
- E. P. Virgo, H. Haidari, Z. L. Shaw, L. Z. Y. Huang, T. L. Kennewell, L. Smith, T. Ahmed, S. J. Bryant, G. S. Howarth, S. Walia, A. J. Cowin, A. Elbourne and Z. Kopecki, Layered Black Phosphorus Nanoflakes Reduce Bacterial Burden and Enhance Healing of Murine Infected Wounds, Adv. Ther., 2023, 2300235 CrossRef.
- Z. L. Shaw, S. Kuriakose, S. Cheeseman, E. L. H. Mayes, A. Murali, Z. Y. Oo, T. Ahmed, N. Tran, K. Boyce, J. Chapman, C. F. Mcconville, R. J. Crawford, P. D. Taylor, A. J. Christofferson, V. K. Truong, M. J. S. Spencer, A. Elbourne and S. Walia, Broad-Spectrum Solvent-free Layered Black Phosphorus as a Rapid Action Antimicrobial, ACS Appl. Mater. Interfaces, 2021, 13, 17340–17352 CrossRef CAS.
- Z. L. Shaw, S. Cheeseman, L. Z. Y. Huang, R. Penman, T. Ahmed, S. J. Bryant, G. Bryant, A. J. Christofferson, R. Orrell-Trigg, C. Dekiwadia, V. K. Truong, J. P. Vongsvivut, S. Walia and A. Elbourne, Illuminating the biochemical interaction of antimicrobial few-layer black phosphorus with microbial cells using synchrotron macro-ATR-FTIR, J. Mater. Chem. B, 2022, 10, 7527–7539 RSC.
- H. Zheng, H. Li, H. Deng, W. Fang, X. Huang, J. Qiao and Y. Tong, Near infrared light-responsive and drug-loaded black phosphorus nanosheets for antibacterial applications, Colloids Surf., B, 2022, 214, 112433 CrossRef CAS.
- H. Li, Y. Liu, S. Li, S. Zhang, B. Huang, R. Cui, Y. Liu and P. Jiang, Cu-Doped black phosphorus quantum dots as multifunctional Fenton nanocatalyst for boosting synergistically enhanced H2O2-guided and photothermal chemodynamic cancer therapy, Nanoscale, 2022, 14, 3788–3800 RSC.
- Y. Wu, Z. Chen, Z. Yao, K. Zhao, F. Shao, J. Su and S. Liu, Black Phosphorus Quantum Dots Encapsulated Biodegradable Hollow Mesoporous MnO2: Dual-Modality Cancer Imaging and Synergistic Chemo-Phototherapy, Adv. Funct. Mater., 2021, 31, 2104643 CrossRef.
- Q. Wu, L. Yao, X. Zhao, L. Zeng, P. Li, X. Yang, L. Zhang, Z. Cai, J. Shi, G. Qu and G. Jiang, Cellular Uptake of Few-Layered Black Phosphorus and the Toxicity to an Aquatic Unicellular Organism, Environ. Sci. Technol., 2020, 54, 1583–1592 CrossRef.
- Y. C. Shin, S. Song, Y. B. Lee, M. S. Kang, H. U. Lee, J. Oh and D. Han, Application of black phosphorus nanodots to live cell imaging, Biomater. Res., 2018, 22, 31 CrossRef.
- J. Hou, H. Wang, Z. Ge, T. Zuo, Q. Chen, X. Liu, S. Mou, C. Fan, Y. Xie and L. Wang, Treating Acute Kidney Injury with Antioxidative Black Phosphorus Nanosheets, Nano Lett., 2020, 20, 1447–1454 CrossRef.
- Z. Liao, X. Ma, J. Kai and J. Fan, Molecular mechanisms of integrin αvβ8 activation regulated by graphene, boron nitride and black phosphorus nanosheets, Colloids Surf., B, 2023, 222, 113139 CrossRef PubMed.
- W. Zhang, Y. Chen, T. Huynh, Y. Yang, X. Yang and R. Zhou, Directional extraction and penetration of phosphorene nanosheets to cell membranes, Nanoscale, 2020, 12, 2810–2819 RSC.
- W. Zhang, T. Huynh, P. Xiu, B. Zhou, C. Ye, B. Luan and R. Zhou, Revealing the importance of surface morphology of nanomaterials to biological responses: Adsorption of the villin headpiece onto graphene and phosphorene, Carbon, 2015, 94, 895–902 CrossRef.
- D. Cortés-Arriagada, Phosphorene as a Template Material for Physisorption of DNA/RNA Nucleobases and Resembling of Base Pairs: A Cluster DFT Study and Comparisons with Graphene, J. Phys. Chem. C, 2018, 122, 4870–4880 CrossRef.
- M. Yadav, R. Kumar and R. Krishnamurthy, Chemistry of Abiotic Nucleotide Synthesis, Chem. Rev., 2020, 120, 4766–4805 CrossRef.
- A. E. Parnell, S. Mordhorst, F. Kemper, M. Giurrandino, J. P. Prince, N. J. Schwarzer, A. Hofer, D. Wohlwend, H. J. Jessen, S. Gerhardt, O. Einsle, P. C. F. Oyston, J. N. Andexer and P. L. Roach, Substrate recognition and mechanism revealed by ligand-bound polyphosphate kinase 2 structures, Proc. Natl. Acad. Sci. U. S. A., 2018, 115, 3350–3355 CrossRef PubMed.
- M. L. Romero Romero, F. Yang, Y. Lin, A. Toth-Petroczy, I. N. Berezovsky, A. Goncearenco, W. Yang, A. Wellner, F. Kumar-Deshmukh, M. Sharon, D. Baker, G. Varani and D. S. Tawfik, Simple yet functional phosphate-loop proteins, Proc. Natl. Acad. Sci. U. S. A., 2018, 115, 11943–11950 Search PubMed.
- T. Zhang, Y. Wan, H. Xie, Y. Mu, P. Du, D. Wang, X. Wu, H. Ji and L. Wan, Degradation Chemistry and Stabilization of Exfoliated Few-Layer Black Phosphorus in Water, J. Am. Chem. Soc., 2018, 140, 7561–7567 CrossRef CAS.
- M. J. Eslamibidgoli and M. H. Eikerling, Mechanical and Chemical Stability of Monolayer Black Phosphorous Studied by Density Functional Theory Simulations, J. Phys. Chem. C, 2018, 122, 22366–22373 CrossRef CAS.
- G. Wang, W. J. Slough, R. Pandey and S. P. Karna, Degradation of phosphorene in air: understanding at atomic level, 2D Mater., 2016, 3, 25011 CrossRef.
- Q. Zhou, Q. Chen, Y. Tong and J. Wang, Light-Induced Ambient Degradation of Few-Layer Black Phosphorus: Mechanism and Protection, Angew. Chem., 2016, 128, 11437–11441 CrossRef PubMed.
- H. Wang and X. F. Yu, Few-Layered Black Phosphorus: From Fabrication and Customization to Biomedical Applications, Small, 2018, 14, 1702830 CrossRef.
- J. Periasamy, V. Kurdekar, S. Jasti, M. B. Nijaguna, S. Boggaram, M. A. Hurakadli, D. Raina, L. M. Kurup, C. Chintha, K. Manjunath, A. Goyal, G. Sadasivam, K. Bharatham, M. Padigaru, V. Potluri and A. R. Venkitaraman, Targeting Phosphopeptide Recognition by the Human BRCA1 Tandem BRCT Domain to Interrupt BRCA1-Dependent Signaling, Cell Chem. Biol., 2018, 25, 677–690 CrossRef CAS PubMed.
- D. M. Lowery, D. Lim and M. B. Yaffe, Structure and function of Polo-like kinases, Oncogene, 2005, 24, 248–259 CrossRef CAS.
- S. Zitouni, C. Nabais, S. C. Jana, A. Guerrero and M. Bettencourt-Dias, Polo-like kinases: structural variations lead to multiple functions, Nat. Rev. Mol. Cell Biol., 2014, 15, 433–452 CrossRef CAS.
- Z. Liu, Q. Sun and X. Wang, PLK1, A Potential Target for Cancer Therapy, Transl. Oncol., 2017, 10, 22–32 CrossRef.
- D. Chapagai, G. Ramamoorthy, J. Varghese, E. Nurmemmedov, C. Mcinnes and M. D. Wyatt, Nonpeptidic, Polo-Box Domain-Targeted Inhibitors of PLK1 Block Kinase Activity, Induce Its Degradation and Target-Resistant Cells, J. Med. Chem., 2021, 64, 9916–9925 CrossRef CAS PubMed.
- S. Ryu, J. Park, Y. J. Ham, D. C. Lim, N. P. Kwiatkowski, D. Kim, D. Bhunia, N. D. Kim, M. B. Yaffe, W. Son, N. Kim, T. Choi, P. Swain, C. Kim, J. Lee, N. S. Gray, K. S. Lee and T. Sim, Novel Macrocyclic Peptidomimetics Targeting the Polo-Box Domain of Polo-Like Kinase 1, J. Med. Chem., 2022, 65, 1915–1932 CrossRef CAS PubMed.
- V. Archambault and K. Normandin, Several inhibitors of the Plk1 Polo-Box Domain turn out to be non-specific protein alkylators, Cell Cycle, 2017, 16, 1220–1224 CrossRef CAS PubMed.
- X. Shao, Z. Ding, W. Zhou, Y. Li, Z. Li, H. Cui, X. Lin, G. Cao, B. Cheng, H. Sun, M. Li, K. Liu, D. Lu, S. Geng, W. Shi, G. Zhang, Q. Song, L. Chen, G. Wang, W. Su, L. Cai, L. Fang, D. T. Leong, Y. Li, X. Yu and H. Li, Intrinsic bioactivity of black phosphorus nanomaterials on mitotic centrosome destabilization through suppression of PLK1 kinase, Nat. Nanotechnol., 2021, 16, 1150–1160 CrossRef CAS.
- M. Cao, R. Cai, L. Zhao, M. Guo, L. Wang, Y. Wang, L. Zhang, X. Wang, H. Yao, C. Xie, Y. Cong, Y. Guan, X. Tao, Y. Wang, S. Xu, Y. Liu, Y. Zhao and C. Chen, Molybdenum derived from nanomaterials incorporates into molybdenum enzymes and affects their activities in vivo, Nat. Nanotechnol., 2021, 16, 708–716 CrossRef CAS PubMed.
- X. Lu, P. Xu, H. Ding, Y. Yu, D. Huo and Y. Ma, Tailoring the component of protein corona via simple chemistry, Nat. Commun., 2019, 10, 4520 CrossRef PubMed.
- L. Ou, H. Chen, B. Yuan and K. Yang, Membrane-Specific Binding of 4 nm Lipid Nanoparticles Mediated by an Entropy-Driven Interaction Mechanism, ACS Nano, 2022, 16, 18090–18100 CrossRef CAS.
- Z. Yan, L. Li, S. Li, Y. Xu and T. Yue, Extracellular interactions between graphene nanosheets and E-cadherin, Environ. Sci.: Nano, 2021, 8, 2152–2164 RSC.
- N. G. Anderson, J. L. Maller, N. K. Tonks and T. W. Sturgill, Requirement for integration of signals from two distinct phosphorylation pathways for activation of MAP kinase, Nature, 1990, 343, 651–653 CrossRef.
- V. Sauvé, G. Sung, N. Soya, G. Kozlov, N. Blaimschein, L. S. Miotto, J. Trempe, G. L. Lukacs and K. Gehring, Mechanism of parkin activation by phosphorylation, Nat. Struct. Mol. Biol., 2018, 25, 623–630 CrossRef.
- G. S. Elowe, I. Alharbi, L. G. Braga and S. Elowe, Playing polo during mitosis: PLK1 takes the lead, Oncogene, 2017, 36, 4819–4827 CrossRef.
- L. Macůrek, A. Lindqvist, D. Lim, M. A. Lampson, R. Klompmaker, R. Freire, C. Clouin, S. S. Taylor, M. B. Yaffe and R. H. Medema, Polo-like kinase-1 is activated by aurora A to promote checkpoint recovery, Nature, 2008, 455, 119–123 CrossRef PubMed.
- I. A. Asteriti, F. De Mattia and G. Guarguaglini, Cross-Talk between AURKA and Plk1 in Mitotic Entry and Spindle Assembly, Front. Oncol., 2015, 5, 283 Search PubMed.
- A. E. H. Elia, L. C. Cantley and M. B. Yaffe, Proteomic screen finds pSer/pThr-binding domain localizing Plk1 to mitotic substrates, Science, 2003, 299, 1228–1231 CrossRef PubMed.
- A. E. H. Elia, P. Rellos, L. F. Haire, J. W. Chao, F. J. Ivins, K. Hoepker, D. Mohammad, L. C. Cantley, S. J. Smerdon and M. B. Yaffe, The Molecular Basis for Phosphodependent Substrate Targeting and Regulation of Plks by the Polo-Box Domain, Cell, 2003, 115, 83–95 CrossRef.
- A. Seki, J. A. Coppinger, C. Y. Jang, J. R. Yates and G. Fang, Bora and the kinase Aurora a cooperatively activate the kinase Plk1 and control mitotic entry, Science, 2008, 320, 1655–1658 CrossRef PubMed.
- A. Scharow, M. Raab, K. Saxena, S. Sreeramulu, D. Kudlinzki, S. Gande, C. Dötsch, E. Kurunci-Csacsko, S. Klaeger, B. Kuster, H. Schwalbe, K. Strebhardt and T. Berg, Optimized Plk1 PBD Inhibitors Based on Poloxin Induce Mitotic Arrest and Apoptosis in Tumor Cells, ACS Chem. Biol., 2015, 10, 2570–2579 CrossRef PubMed.
- F. A. Barr, H. H. W. Silljé and E. A. Nigg, Polo-like kinases and the orchestration of cell division, Nat. Rev. Mol. Cell Biol., 2004, 5, 429–441 CrossRef PubMed.
- S. Lange, P. Schmidt and T. Nilges, Au3SnP7@Black Phosphorus: An Easy Access to Black Phosphorus, Inorg. Chem., 2007, 46, 4028–4035 CrossRef PubMed.
- P. Ballone and R. O. Jones, A reactive force field simulation of liquid-liquid phase transitions in phosphorus, J. Chem. Phys., 2004, 121, 8147–8157 CrossRef.
- Y. Liu, X. Song, Y. Yang, Y. Q. Li, M. Zhao, Y. Mu and W. Li, Anisotropic protein diffusion on nanosurface, Nanoscale, 2020, 12, 5209–5216 RSC.
- B. Li, X. Xie, G. Duan, S. H. Chen, X. Meng and R. Zhou, Binding patterns and dynamics of double-stranded DNA on the phosphorene surface, Nanoscale, 2020, 12, 9430–9439 RSC.
- W. Zhang, Y. Gou, L. Cheng, K. Dong, Y. Sheng, C. Ye, X. Yang and Y. Mu, Revealing the biotoxicity of phosphorene oxide nanosheets based on the villin headpiece, Phys. Chem. Chem. Phys., 2023, 25, 3100–3109 RSC.
- Y. Xiao, Y. X. Pang, Y. Yan, P. Qian, H. Zhao, S. Manickam, T. Wu and C. H. Pang, Synthesis and Functionalization of Graphene Materials for Biomedical Applications: Recent Advances, Challenges, and Perspectives, Adv. Sci., 2023, 10, 2205292 CrossRef.
- V. Castagnola, W. Zhao, L. Boselli, M. C. Lo Giudice, F. Meder, E. Polo, K. R. Paton, C. Backes, J. N. Coleman and K. A. Dawson, Biological recognition of graphene nanoflakes, Nat. Commun., 2018, 9, 1577 CrossRef PubMed.
- W. Humphrey, A. Dalke and K. Schulten, VMD: Visual molecular dynamics, J. Mol. Graphics, 1996, 14, 33–38 CrossRef PubMed.
- H. Tang, Y. Zhao, S. Shan, X. Yang, D. Liu, F. Cui and B. Xing, Wrinkle- and Edge-Adsorption of Aromatic Compounds on Graphene Oxide as Revealed by Atomic Force Microscopy, Molecular Dynamics Simulation, and Density Functional Theory, Environ. Sci. Technol., 2018, 52, 7689–7697 CrossRef PubMed.
- M. A. Unal, F. Bayrakdar, H. Nazir, O. Besbinar, C. Gurcan, N. Lozano, L. M. Arellano, S. Yalcin, O. Panatli, D. Celik, D. Alkaya, A. Agan, L. Fusco, S. Suzuk Yildiz, L. G. Delogu, K. C. Akcali, K. Kostarelos and A. Yilmazer, Graphene Oxide Nanosheets Interact and Interfere with SARS-CoV-2 Surface Proteins and Cell Receptors to Inhibit Infectivity, Small, 2021, 17, 2101483 CrossRef PubMed.
- S. Vilar, G. Cozza and S. Moro, Medicinal chemistry and the molecular operating environment (MOE): application of QSAR and molecular docking to drug discovery, Curr. Top. Med. Chem., 2008, 8, 1555–1572 CrossRef PubMed.
- M. J. Abraham, T. Murtola, R. Schulz, S. Páll, J. C. Smith, B. Hess and E. Lindahl, GROMACS: High performance molecular simulations through multi-level parallelism from laptops to supercomputers, SoftwareX, 2015, 1-2, 19–25 CrossRef.
- G. A. Kaminski, R. A. Friesner, J. Tirado-Rives and W. L. Jorgensen, Evaluation and Reparametrization of the OPLS-AA Force Field for Proteins via Comparison with Accurate Quantum Chemical Calculations on Peptides, J. Phys. Chem. B, 2001, 105, 6474–6487 CrossRef.
- W. L. Jorgensen, J. Chandrasekhar, J. D. Madura, R. W. Impey and M. L. Klein, Comparison of simple potential functions for simulating liquid water, J. Chem. Phys., 1983, 79, 926–935 CrossRef.
- J. Kim, K. Lee and K. Rhee, PLK1 regulation of PCNT cleavage ensures fidelity of centriole separation during mitotic exit, Nat. Commun., 2015, 6, 10076 CrossRef PubMed.
- J. Kang, S. A. Wells, J. D. Wood, J. Lee, X. Liu, C. R. Ryder, J. Zhu, J. R. Guest, C. A. Husko and M. C. Hersam, Stable aqueous dispersions of optically and electronically active phosphorene, Proc. Natl. Acad. Sci. U. S. A., 2016, 113, 11688–11693 CrossRef.
- L. Hao, T. Li, L. Wang, X. Shi, Y. Fan, C. Du and Y. Wang, Mechanistic insights into the adsorption and bioactivity of fibronectin on surfaces with varying chemistries by a combination of experimental strategies and molecular simulations, Bioact. Mater., 2021, 6, 3125–3135 Search PubMed.
- X. Wang, X. Wang, M. Wang, D. Zhang, Q. Yang, T. Liu, R. Lei, S. Zhu, Y. Zhao and C. Chen, Probing Adsorption Behaviors of BSA onto Chiral Surfaces of Nanoparticles, Small, 2018, 14, 1703982 CrossRef.
- S. J. Humphrey, D. E. James and M. Mann, Protein Phosphorylation: A Major Switch Mechanism for Metabolic Regulation, Trends Endocrinol. Metab., 2015, 26, 676–687 CrossRef PubMed.
- D. Mehta, M. Ghahremani, M. Pérez Fernández, M. Tan, P. Schläpfer, W. C. Plaxton and R. G. Uhrig, Phosphate and phosphite have a differential impact on the proteome and phosphoproteome of Arabidopsis suspension cell cultures, Plant J., 2021, 105, 924–941 CrossRef PubMed.
- A. N. Lane and T. W. Fan, Regulation of mammalian nucleotide metabolism and biosynthesis, Nucleic Acids Res., 2015, 43, 2466–2485 CrossRef PubMed.
- M. G. Vervloet, S. Sezer, Z. A. Massy, L. Johansson, M. Cozzolino and D. Fouque, The role of phosphate in kidney disease, Nat. Rev. Nephrol., 2017, 13, 27–38 CrossRef CAS PubMed.
- X. Niu, Y. Li, Y. Zhang, Q. Li, Q. Zhou, J. Zhao and J. Wang, Photo-oxidative Degradation and Protection Mechanism of Black Phosphorus: Insights from Ultrafast Dynamics, J. Phys. Chem. Lett., 2018, 9, 5034–5039 CrossRef CAS PubMed.
- J. Plutnar, Z. Sofer and M. Pumera, Products of Degradation of Black Phosphorus in Protic Solvents, ACS Nano, 2018, 12, 8390–8396 CrossRef CAS.
- S. Zhang, X. Zhang, L. Lei, X. F. Yu, J. Chen, C. Ma, F. Wu, Q. Zhao and B. Xing, pH-Dependent Degradation of Layered Black Phosphorus: Essential Role of Hydroxide Ions, Angew. Chem., Int. Ed., 2019, 58, 467–471 CrossRef CAS.
- W. Tao, X. Zhu, X. Yu, X. Zeng, Q. Xiao, X. Zhang, X. Ji, X. Wang, J. Shi, H. Zhang and L. Mei, Black Phosphorus Nanosheets as a Robust Delivery Platform for Cancer Theranostics, Adv. Mater., 2017, 29, 1603276 CrossRef PubMed.
- M. van Druenen, F. Davitt, T. Collins, C. Glynn, C. O'Dwyer, J. D. Holmes and G. Collins, Evaluating the Surface Chemistry of Black Phosphorus during Ambient Degradation, Langmuir, 2019, 35, 2172–2178 CrossRef CAS PubMed.
- J. Zhao, X. Zhang, Q. Zhao, X. Yu, S. Zhang and B. Xing, Unique Interaction between Layered Black Phosphorus and Nitrogen Dioxide, Nanomaterials, 2022, 12, 2011 CrossRef CAS PubMed.
- Q. Zhao, W. Ma, B. Pan, Q. Zhang, X. Zhang, S. Zhang and B. Xing, Wrinkle-induced high sorption makes few-layered black phosphorus a superior adsorbent for ionic organic compounds, Environ. Sci.: Nano, 2018, 5, 1454–1465 RSC.
- Z. Luo, S. Li, Y. Xu, H. Ren, X. Zhang, G. Hu, F. Huang and T. Yue, Extracting pulmonary surfactants to form inverse micelles on suspended graphene nanosheets, Environ. Sci.: Nano, 2018, 5, 130–140 RSC.
- M. Frodin, T. L. Antal, B. A. Dummler, C. J. Jensen, M. Deak, S. Gammeltoft and R. M. Biondi, A phosphoserine/threonine-binding pocket in AGC kinases and PDK1 mediates activation by hydrophobic motif phosphorylation, EMBO J., 2002, 21, 5396–5407 CrossRef PubMed.
- Y. Y. Illarionov, M. Waltl, G. Rzepa, J. S. Kim, S. Kim, A. Dodabalapur, D. Akinwande and T. Grasser, Long-Term Stability and Reliability of Black Phosphorus Field-Effect Transistors, ACS Nano, 2016, 10, 9543–9549 CrossRef CAS PubMed.
- R. Gusmao, Z. Sofer and M. Pumera, Functional Protection of Exfoliated Black Phosphorus by Noncovalent Modification with Anthraquinone, ACS Nano, 2018, 12, 5666–5673 CrossRef CAS PubMed.
- H. Hu, H. Gao, L. Gao, F. Li, N. Xu, X. Long, Y. Hu, J. Jin and J. Ma, Covalent functionalization of black phosphorus nanoflakes by carbon free radicals for durable air and water stability, Nanoscale, 2018, 10, 5834–5839 RSC.
- G. Abellan, S. Wild, V. Lloret, N. Scheuschner, R. Gillen, U. Mundloch, J. Maultzsch, M. Varela, F. Hauke and A. Hirsch, Fundamental Insights into the Degradation and Stabilization of Thin Layer Black Phosphorus, J. Am. Chem. Soc., 2017, 139, 10432–10440 CrossRef CAS.
- Y. Cao, X. Tian, J. Gu, B. Liu, B. Zhang, S. Song, F. Fan and Y. Chen, Covalent Functionalization of Black Phosphorus with Conjugated Polymer for Information Storage, Angew. Chem., Int. Ed., 2018, 57, 4543–4548 CrossRef CAS.
- J. Mo, Q. Xie, W. Wei and J. Zhao, Revealing the immune perturbation of black phosphorus nanomaterials to macrophages by understanding the protein corona, Nat. Commun., 2018, 9, 2480 CrossRef PubMed.
Footnote |
† Electronic supplementary information (ESI) available: Models and parameters of four nanosheets used in simulations, summary of simulation systems, identification of residues in contact with different nanosheets, characterization of the PBD's structure induced by interactions with BP nanomaterials, repeated simulations of PBD interactions with BP, BP-O, and BP-QD, effect of the initial contact mode, and additional results of PBD interactions with BP-QD and phosphate ions. See DOI: https://doi.org/10.1039/d3en00446e |
|
This journal is © The Royal Society of Chemistry 2024 |
Click here to see how this site uses Cookies. View our privacy policy here.