DOI:
10.1039/D2VA00161F
(Critical Review)
Environ. Sci.: Adv., 2023,
2, 39-54
Biofouling phenomena in membrane distillation: mechanisms and mitigation strategies
Received
16th July 2022
, Accepted 29th October 2022
First published on 3rd November 2022
Abstract
Membrane distillation (MD) is envisaged as a cost-effective water desalination technology. When operated by low-grade energy, MD surpasses the cost challenges faced by other water desalination technologies. Although MD operates under conditions that minimize the survival of biofilm causing microorganisms, their development and succession is being increasingly reported. This is believed to be caused by the presence of halophiles and thermophiles in the feed solution, inducing significant efficiency losses. Therefore, biofouling mitigation remains crucial. This study reports current developments toward MD biofouling and mitigation strategies. Also, effects of membrane biofouling on process performance are briefly highlighted. This provides an in-depth understanding of measures required to minimize biofouling of MD systems.
Environmental significance
While membrane distillation (MD) is a promising technology in wastewater desalination, its commercial growth is harnessed by various factors including membrane fouling. To address this problem, various studies have extensively reported on organic and inorganic fouling. Biofouling was rarely reported on the basis that MD operational conditions prevent growth of microorganisms on membrane surfaces. However, this problem persists. The current study provides comprehensive and yet concise biofouling phenomenon in MD, its formation, mechanisms, specific microorganisms responsible for membrane fouling and control measures. Deposition and growth of these microorganisms on membrane surfaces is influenced by membrane properties, hydrodynamic conditions, feed solution properties and membrane module designs. To promote its industrial realization, MD requires significant experimental work to mitigate the existing fouling challenges.
|
Introduction
The unprecedented rise in climate change and an increase in population growth have resulted in significant global water shortages.1,2 Over the last century, water consumption has increased by approximately 600%, largely due to urbanization.3 Furthermore, based on scholarly reports, nearly 1.8 billion people are projected to live in water stressed areas by 2025.4 These alarming statistics and the rise in demand for potable water have stimulated the need to explore freshwater supply alternatives. Currently, scientists and engineers are developing sustainable solutions through research to address existing water shortages.
Membrane-based separation technologies have emerged as promising approaches to supply water to coastal areas. These include reverse osmosis (RO), forward osmosis (FO), nanofiltration (NF) and membrane distillation (MD).5 Although RO is the leading water desalination technology, its high energy consumption and extreme operating pressure increases operational costs. These costs are significantly high compared to the volume of water produced. Therefore, alternative membrane technologies of low-cost should be considered. MD is envisaged as a cost-effective membrane technology, not only because of its ability to exploit solar thermal energy but its capacity to operate under moderate conditions, requiring inexpensive equipment.6 The driving force behind MD is the vapor pressure difference existing across the membrane, induced by differences in temperature (Fig. 1a).7 As illustrated in Fig. 1b, both mass (vapor) and heat are transferred through the membrane. The mechanism of mass transport is well explained by Poisseuille flow, molecular diffusion, and Knudsen diffusion, conditional to the existence of trapped air in the pores and the membrane pore size.8 Mass transport depends on the type of membrane used, its properties, the concentration of the feed solution, and the mass transfer coefficient. Further advantages of MD include high salt rejection (≥99%), minimal sensitivity to salinity of the feed solution, low hydrostatic temperature and pressure requirements and the feasible utilization of renewable energy and low-grade/residual energy.9 Schwantes et al. (2013)10 used the waste heat generated from a diesel power station to drive a pilot-scale MD system. The waste heat supplied feed temperatures of 70–80 °C, enabling water production of 4 m3 per day. Although MD displays attractive features, its industrial application has been hindered by low performance module design, production of low water fluxes (compared to current technologies), and membrane fouling.11,12
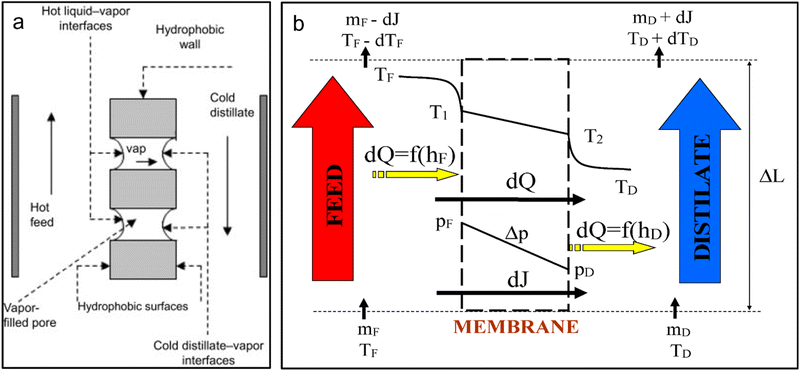 |
| Fig. 1 .Mechanism of operation of MD: (a) process mechanism and (b) heat and mass transfer, where TF, TD, T1, T2, PF, PD, Δp, dQ represent feed, distillate, evaporation and condensation temperatures, feed and distillate vapour pressure, vapor partial pressure difference and change in energy respectively.13–15 | |
Membrane fouling refers to the deposition and growth of inorganic, organic, colloidal and biological substances on the membrane surface.14 Inorganic fouling involves the deposition of mineral salts on the membrane surface following precipitation whereas organic fouling refers to the deposition of organic matter on the membrane surface.16 Membrane fouling is reported to increase membrane wetting, causing a reduction in distillate quality.17 Moreover, membrane fouling introduces regular membrane cleaning and frequent membrane replacements, further increasing costs of water production.18 Current reported studies mostly focus on inorganic and organic fouling, with limited information on the dynamics and succession of biofouling.19 By definition, biofouling refers to the deposition and accumulation of microorganisms on the surface of the membrane and within its pores, leading to the formation of a biofilm.20 The biofilm consists of microbial cells and extracellular polymeric substances (EPS), covering the surface of the membrane. Biofouling of MD systems was overlooked with the perceptions that its operating conditions would minimize biofilm development. However, an in-depth study of fouling layers has revealed the increased presence of biofilms.21 Formation of biofilms induces membrane wetting22 and vapor pressure decline23 causing decay in salt rejection22 and water flux.21,24–26 Krivorot et al., (2011)24 studied biofouling occurrence in MD, using coastal seawater as the feed. Reportedly, microbial attachment increased quantitatively as a function of time, thus affecting process performance. A 34% reduction in water flux was recorded, markedly due to membrane pore blockage caused by biofilm formation. Furthermore, flux decline was caused by the growth of crystal salts on the membrane surface. Another phenomenon contributing to biofouling in MD is temperature polarization (TP), referring to the difference in temperature between the bulk feed stream and the membrane surface.6 The TP stimulates microbial growth at the cooler membrane interface.24 Furthermore, TP decreases the driving force in MD.27 A detailed review of TP and its holistic effects on MD is reported elsewhere.28 Membrane wetting and a subsequent reduction in salt rejection induced by microbial accumulates on the membrane surface was reported by Bogler and Bar-Zeev (2018).22 Membrane wetting was clearly observed at feed temperature of 65 °C using optical coherence tomography (OCT) and facilitated penetration of bacteria and endospores through the membrane towards the distillate. Microbial deposition was dependent on the composition of feed solution and nutrient availability.26 To establish high performing MD with excellent resistance to biofouling, elucidation of biofouling mechanism and its effects is essential. A recent review presenting biofilm development in MD systems was reported.6 However, specific illustration and collective reporting of microorganisms causing biofouling in MD was not highlighted. Therefore, the current review provides detailed insights of MD biofouling with particular focus on the survival of microbes under harsh MD operating conditions. Additionally, the mechanism of biofouling, its formation, causes, effects, and prevention for efficient MD operation are discussed.
Biofilm formation
Biofilm formation follows a pathway of successive steps including; (1) conditioning film formation with migration and adhesion of bacterial cells to the membrane surface, (2) EPS secretion, growth and maturation of bacterial cells, and (3) proliferation and cell detachment for colonization of new areas.30 For control and efficient mitigation of biofouling, insights into its mechanism must be expounded and understood.
Conditioning film formation and surface attachment
A conditioning film composed of macromolecules, organic matter, proteins, amino acids, and nucleic acids initially covers the surface of the membrane. Alongside, dead bacteria and soluble microbial products (SMP's) further adhere on the membrane. The presence of conditioning film alters the membrane surface, promoting better adhesion of cells. Planktonic (free-floating) cells migrate from the bulk solution to the membrane surface through the Brownian motion, where movement is induced by the collision of particles.15 However, attachment is not permanent, since planktonic cells preferentially select sites for adhesion through cell locomotion using flagella and pili.31 Cells exhibiting better motility and microbial activity attach first on membrane surfaces.32 Following attachment, cells undergo proliferation, where constant multiplication and division take place. Furthermore, transparent exopolymer substances (TEP) and gel-like polysaccharides (aggregates) adsorb on the membrane surface to influence fouling.33 The TEPs generate a viscous surface, thus providing a transport for microbial cells to the membrane surface. However, due to their complex chemical composition, specific contribution of TEPs to fouling is not known. According to Zhang et al. 2018,34 interaction between cells and the membrane (cell-to-surface) occur largely through electrostatic and hydrophobic–hydrophobic processes. Although positively charged bacterial cells adhere readily on membrane surfaces, negatively charged bacterial cells tend to adhere less, especially in cases where the membrane surface is predominantly negatively charged and the ionic strength of the feed solution is low.35 Solutions of low ionic strength contain less ions to counter-act the electronegativity of bacterial cells, resulting in increased repulsion between the membrane and the cell. However, at high ionic strength, cells adhere irreversibly on membrane surfaces. Abu-Lail and Camesano (2003)36 evaluated the bio-adhesion of Pseudomonas putida KT2442 and noted low adhesion rates at low ionic strength. This was attributed to the high energy barrier required for cell adhesion to take place.37
Additionally, hydrophobic surfaces tend to adsorb hydrophobic microorganisms. Bacteria contain hydrophobins (known as adhesins), promoting adhesion and formation of conditioning layer. This layer initiates biofilm development.27 Hori and Matsumoto (2010)35 studied the binding mechanism of Staphylococcus aureus on hydrophilic and hydrophobic surfaces. Notably, macromolecules tethered more on hydrophobic surfaces than hydrophilic surfaces. This was attributed to cell-surface contact time and adhesion force. According to Fabre et al. (2018),38 large quantities of protein adhered on hydrophobic surfaces compared to hydrophilic surfaces. This phenomenon was described by reduction in free energy and a rise in entropy of the system.39 However, full assurance of absolute adherence/non-adherence of cells is not well understood since bacteria exhibit endless mechanisms enabling their adaptation under different conditions.40 For instance, hydrophilic bacteria adsorb on hydrophilic surfaces too, further promoting membrane fouling. Theoretically, polysaccharides (the major constituent of microbial cell exopolymer) favourably adhere on hydrophilic surfaces and form a gel-like firm matrix layer. Also, membrane roughness increases microbial attachment on membrane surfaces.41 Bernstein, Belfer, and Freger (2011)42 reported conflicting findings where fewer cells were deposited at high membrane surface roughness. In contrast, other studies have found no correlation between surface roughness and bacterial deposition. Hence, extensive research is required to ascertain these contradictions.
The presence of high organic material in the feed solution is reported to increase the deposition of microbial contaminants on surfaces (Bogler, Lin, and Bar-zeev 2017)27 (Fig. 2). According to Chen et al., (2021),25 deposition of organic matter on membrane surface induced the formation of a conditioning layer, enabling more bacteria to adsorb on the surface. Other forces driving the adhesion of cells on membrane surfaces include thermodynamic and hydrodynamic interactions, polarity, oxygen content, bacteria abundance, process cross flow velocity and permeate flux. Furthermore, process parameters such as feed spacers and surface charge enhance biofilm development. Accumulation of microorganisms on feed spacers reduces the flow of the feed stream, thus affecting water flux.27
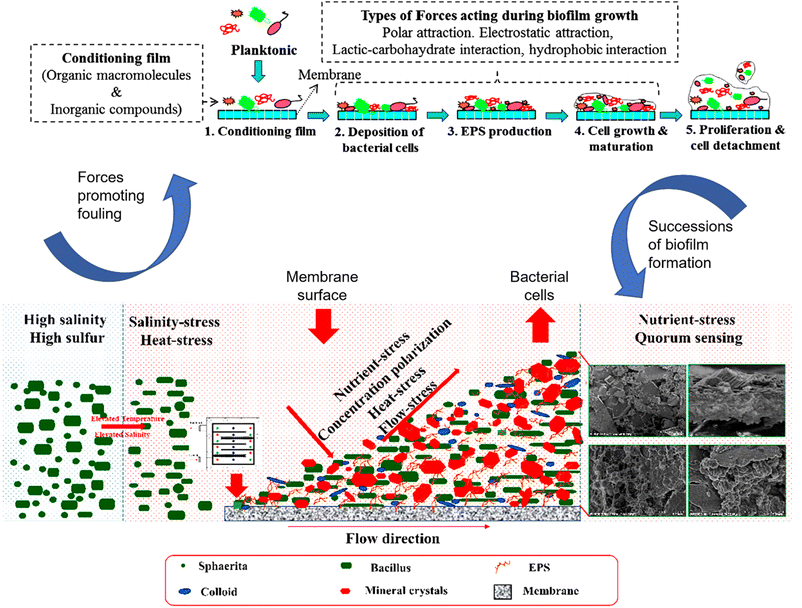 |
| Fig. 2 .Mechanistic relationship of the salinity, heat, nutrient, and flow stresses on the distribution of bacteria on membrane surfaces during MD operation.30,32 | |
Biofilm formation and EPS secretion
Following attachment, bacterial cells secrete EPS. The EPS and bacteria form a highly organised 3-dimensional structure known as a biofilm (Fig. 3). The biofilm forms between the solid and the liquid phase, where ultimate separation takes place. The EPS immobilizes and encapsulates bacterial cells, enhancing the firmness of the biofilm. Though biofilms vary in composition, they consist of bacteria (dead and alive), fungi, eukaryotic organisms, EPS, microalgae, and archaea.43,44 The biofilm structure contains interstitial water channels, facilitating the movement of oxygen, nutrients, and genetic material.27 Bacteria are usually larger than other biofilm microorganisms and typically range from 0.5–2 μm.35 Movement of bacteria through the biofilm results in the colonization of new areas. Also, EPS contains polysaccharides, lipoproteins, proteins, glycoproteins, and carbohydrates contributing towards bacteria resistance against inhibitors.45,46 Furthermore, EPS bind the cells to the surface and maintain a stable environment. The EPS enhances communication within cells and act as a source of nutrients for the bacteria.6 Bacteria are known to release significant EPS under thermal stress to overcome destruction by MD operational conditions.32 During membrane colonization, bacteria produce an exopolymer matrix, which grows and multiplies through the help of nutrients to adapt to the new environment (Fig. 3).
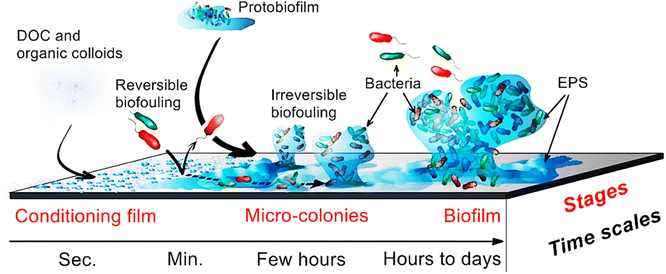 |
| Fig. 3 .Biofilm progression on membrane surfaces. Organic matter forms a conditioning layer enabling attachment of bacterial cells. The EPS are subsequently secreted to further promote attachment of bacterial cells and their colonies. During this process, the biofilm forms thus promoting dispersion and continuous growth of bacterial clusters.15,27 | |
Notably, different biofilm layers contain different pore-size distributions. Goh et al., (2013)23 used evapoporometry to study the particle-size distribution of the biofilm layer caused by two sludge solutions of different hydrophobicities. Reportedly, the hydrophilic sludge containing smaller pores was responsible for vapor pressure reduction. Moreover, smaller pores depressed the vapor flux, owing to the Kelvin effect rather than the effect of an increase in hydraulic resistance. During biofilm development, bacteria senses each other within the vicinity, a process known as quorum sensing (QS). Bacteria secrete autoinducers during QS development, enhancing their communication.47 The QS further promotes microbial social activities and enhance community behaviour through the expression of certain genes.48 The dispersion of the biofilm is augmented by QS.49 Thus, QS further encourages biofilm development. According to Zheng et al., (2022),32 the development and succession of the microbial community is exerted by QS, amongst other factors. The obstinacy of biofilm development and EPS secretion have deemed membrane biofouling a key research concern.
Enumeration and identification of microbial cells on the membrane surface
Biofilm identification on the membrane surface is evaluated using a variety of techniques including scanning electron microscopy coupled with energy dispersive spectroscopy (SEM-EDS), optical coherence tomography (OCT) and confocal laser scanning microscopy (CLSM).50,51 Zodrow et al., (2014)52 evaluated membrane biofilm formation using CLSM, assessing the architecture, heterogeneity and biovolume of the microbial community. The biofilms were heterogeneous and contained several colonies, with a plethora of Burkholderiales, Rhodobacterales, and Flavobacteriales. Bogler & Bar-Zeev, (2018)22 identified the presence of polysaccharides and detected dead and live bacterial cells using CLSM, with a further evaluation of the average biofilm thickness and total biovolume. Overall, feed operating temperatures of 55 °C led to a slightly thicker biovolume compared to 47 °C. This was attributed to provision of the optimum growth temperature of Anoxybacillus sp. Krivorot et al., (2011)24 evaluated the progression of biofilm development on the membrane surface using SEM. The experiment was conducted over a period of 19 days. Reportedly, deposition of microbial cells increased qualitatively as a function of time. Initial bacterial attachment was detected after 28 h, with the conditioning film seen only after 20 h of operation. From 48 h towards 19 days, the biofilm formed, thus covering the membrane surface. This later caused a reduction in process performance.
Physiochemical characteristics and viscoelastic properties of the biofilm are largely determined via UV/vis spectrometry, flow cytometry, atomic force microscopy (AFM) and Fourier Transform Infrared Spectroscopy (FTIR).27 Phattaranawik et al., (2009)21 utilized UV/vis spectrometry to quantify the concentration and composition of the deposited biofilm on the surface of polyvinylidene fluoride (PVDF) and polytetrafluoroethylene (PTFE) membranes. Reportedly, the fouling layer was composed of protein, polysaccharides, and EPS. Since the concentration of polysaccharides on PVDF was higher than PTFE, it was envisaged that polysaccharides played a crucial role in flux decay. The flux of PVDF decreased considerably while that of PTFE remained stable. In another study, Krivorot et al., (2011)24 determined the protein content of the biofilm developed on the membrane surface using the Lowry protein assay. Notably, the protein concentration deposited on the membrane gave an estimation of the biofilm thickness on the surface, further confirming SEM results. In another study, Goh et al., (2013)23 employed FTIR to study and confirm the presence of polysaccharides and proteins, indicating the presence of bacteria. Specifically, peaks at 1500–1700 cm−1, 950–1170 cm−1 and 2800–3000 cm−1 were attributed to proteins, polysaccharides, and fatty acids, respectively. The use of highly specialized sequencing techniques for identification of bacterial species within the biofilm structure has gained significant attraction. For instance, Goh et al., (2013),23 identified bacterial species on fouled membranes through DNA amplification using polymerase chain reaction (PCR). Bacterial organisms were predominantly hydrophilic, thermophilic, and halotolerant species. These included Rubrobacter taiwanensis, Caldalkalibacillus uzonensis, Caldalkalibacillus uzonensis, Tepidimonas sp, and Meiothermus hypogaeus. More recently, specialized techniques such as 16S rDNA and gene sequencing have gained research consideration. With these, bacterial species, growth patterns and behavioural changes of specific microorganisms are identified. Liu et al., (2020a)55 studied the bacterial composition of the biofilm on the membrane surface using 16S rRNA and gene sequencing following DNA extraction. The abundance of live bacteria was higher during initial biofilm development stage which sharply declined as a function of time. This decrease was associated with an increase in salt crystal deposition on the membrane. A further increase in organic and inorganic substances contributed to the remarkable succession and evolution of biofilm bacterial community.
Microorganisms responsible for membrane biofouling in MD
Biofouling in MD is predominantly exacerbated by thermophilic, mesophilic, and halophilic bacteria present in the feed solution. Since feed solutions sourced from different environmental locations contain microorganisms of different identity, analytical assessment of feed solution composition is imperative. For example, feed solutions sourced from marine environments cause biofouling through deposition of phyla Firmicutes, Bacteroidetes and Proteobacteria, largely at high temperatures and water salinity.53 From a general perspective, bacteria enhancing biofilm development in MD include genera Mycobacterium, Bacillus, Lactobacillus, Cytophaga, and Flavobacterium.45 Notably, not all microorganisms present in the feed solution cause biofouling in MD. This uncertainty of events emphasises the importance of extensive biofouling assessment in MD systems.26,29 Gryta (2002)26 evaluated biofouling occurrence in MD. Prior to MD operation, the feed solution was characterized by genera Pseudomonas, Penicillium bacteria, Aspergillus fungi and species S. faecalis. Evaluation of biofilm on membrane surface displayed the presence of S. faecalis and Aspergillus fungi only. Genera Pseudomonas growth was hindered by oxygen deficiency and high-water salinity. However, S. faecalis presented resistance to process conditions, thuspromoting membrane fouling. In another study, Zheng et al. (2022)32 reported a change of plump sphere or short rod to lankier rhabditiform with a microbial community transformation from Algoriphagus, Marinobater, Sulfurihydrogenibium to Chelativorans, Acinetobater, Idiomarina. Change in microbial community was attributed to elevated temperatures and high saline conditions. Moreover, the latter strains notably survived due to their high motility, good quorum sensing effect and EPS secretion. Microbial succession is detrimental to MD as it leads to production of more EPS, thus decreasing membrane life span and process performance. In certain instances, microbial communities present in the feed solution do not evolve, but deposit on the membrane surface. Zodrow et al., (2014)52 evaluated the biofouling impact of seawater, predominantly characterized by Octadecabacter, Sediminicola, Loktanella, and Pelagibacteraceae. These strains were detected on the membrane surface, although in varying concentrations. The most abundant strain was mesophilic Octadecabacter, due to its high temperature and salinity resistance. Other strains detected included thermophilic Bacillales and spore self-protective Ralstonia. Additional microbes identified from the biofilm are presented in Table 1.
Table 1 Biofouling-causing microbial organisms in MDa
Feed source |
Operating conditions |
Microorganism |
Ref. |
T
F and TP are the feed and permeate temperatures, CV is the crossflow velocity, and the letters G, F, O, P and S represent the taxonomic classification of the microorganisms namely: G = genus, F = family, O = order, P = phylum, and S = species, respectively.
|
Saline wastewater (from animal intestines) |
Temp: TF – 80 °C |
S. faecalis (S) |
26
|
T
P – 25 °C |
CV: 0.367 m s−1 |
Seawater (Long Island Sound) |
Temp: TF – 50.4 °C |
Ralstonia (G), Octadecabacter (G), Pelagibacteraceae (F), Loktanella (G), Sediminicola (G), Vibrionaceae (F), Rhodobacteraceae (F) Cryomorphaceae (F), Flavobacteriaceae (F), Bacillales (O) |
52
|
T
P – 18.1 °C |
CV: 4.3 cm s−1 |
Xuanwu Lake (China) |
Temp: TF – 60 °C |
Anoxybacillus (G), Meiothermus (G), Schlegelella (G), Tepidimona (G), Vulcaniibacterium (G), Proteobacteria (P), Deinococcus-Thermus (P) |
55
|
T
P – 10 °C |
CV: — |
Xuanwu Lake, Nan Lake and Qinhuai, River (Nanjing, China) |
Temp: TF – 60 °C |
Tepidimonas (G), Meiothermus (G), OLB14_norank (G), Schlegelella (G), Hydrogenophilaceae (F), Env.OPS 17_norank (G), Armatimonadetes_norank (G) |
25
|
T
P – 15 °C |
CV: 10.5 mm s−1 |
Wastewater from power plant |
Temp: TF – 55 °C |
Idiomarina (G), Chelativorans (G), Phenylobacterium (G), Methyloversatilis (G), Schlegelella (G), Aeribacillus (G), Bacillus (G), Actinobacteria (P), Chloroflexi (P), Microgenomates (P) |
32
|
T
P – 25 °C |
CV: — |
Xuanwu Lake (China) |
Temp: TF – 60 °C |
Tepidimonas (G), Meiothermus (G), Sphingobium (G), Env.OPS 17_norank (G), Curvibacter (G), OLB14_norank (G), Pelomonas (G), Novosphingobium (G), Sphingomonas (G), Bradyrhizobium (G), Chelatococcus (G), Geobacillus (G) |
55
|
T
P – 10 °C |
CV: – 10.5 mm s−1 |
Artificial sterile wastewater |
Temp: TF – 55 °C |
Anoxybacillus sp (G) |
22
|
T
P — |
CV: — |
Different modes of MD operations (closed and open loop) affect biofilm development differently.19,32 Liu et al., (2020a)55 used lake water (Xuanwu Lake) characterized by Proteobacteria, Actinobacteria, Bacteroidetes, and Cyanobacteria to assess biofilm development in MD. Early biofilm development consisted of genera Acidovorax and Acetobacteraceae, which was replaced by thermophilic Methyloversatilis at stage 2 of flux decay. Only Gammaproteobacteria and Deinococcus-Thermus were detected from the biofilm under closed loop operation. The viability of these strains was explained by their halotolerant mechanism induced by a change in morphology to withstand heat.32 Under open loop operation, the membrane biofilm was dominated by Anoxybacillus, Meiothermus, Schlegelella, Tepidimonas, and Vulcaniibacterium. These examples show the dependence of microorganisms on process parameters. The impact of feed salinity was further evaluated by Chen et al., (2021),25 where the membrane biofilm was composed of Proteobacteria, Bacteroidetes, Deinococcus-Thermus, Tepidimonas, Meiothermus, OLB14_norank, Env.OPS 17_norank and Schlegelella. The increase in feed concentration induced by continuous MD operation inhibited the growth of OLB14_norank, Schlegelella, and Tepidimonas. Evidently, MD conditions affect microbial diversity, mobility and activity, leading to a biological selection of cells.54
Effects of biofouling on process performance
Microbial accumulation on membrane surface produces undesirable effects such as permeate flux decays, salt rejection decays and a reduction in membrane lifespan. These effects are largely caused by vapor pressure depression/pore blockage, membrane wetting, and the accumulation of EPS and protein. Microbial accumulates further adsorb on feed spacers, thus exacerbating fouling. However, it is worth noting that decay in MD process performance is complex and may not be attributed to biofouling alone. Table 2 summarizes the impact of biofouling on water flux and salt rejection.
Table 2 A summarized impact of biofouling on MD efficiencya
Configuration of MD |
Type of membrane |
Feed type |
Temperature (°C) |
Flux decay (L m−2 h−1) |
Salt rejection decay |
Ref. |
Where TF, TP, JI, JF, kI and kF represent the feed and permeate temperature, initial and final water flux, initial and final permeate conductivity respectively.
|
DCMD |
PP |
Coastal seawater |
T
F – 40 |
J
I – 3.85 |
No effect on salt rejection efficiency |
24
|
T
P – 20 |
J
F – 2.55 |
MDBR |
PVDF |
Sludge suspension |
T
F – 55 |
J
I – 8.42 |
k
I – 217 μS cm−1 |
23
|
T
P – 19.5 |
J
F – 3.36 |
k
F – <600 μS cm−1 |
DCMD |
— |
Artificial sterile wastewater |
T
F – 65 |
J
I – 23.0 |
k
I – 1000 μS cm−1 |
22
|
T
P — |
J
F – 15.6 |
k
F – 90 000 μS cm−1 |
MDBR |
PVDF/PTFE |
Sludge |
T
F – 56 |
J
I – 12.7 |
k
I – 1.6 g L−1 |
21
|
T
P – 25 |
J
F – 1.90 |
k
F — |
DCMD |
PTFE |
Estuarine water |
T
F – 50.4 |
J
I – 20.0 |
No effect on salt rejection |
52
|
T
P – 18.1 |
J
F – 10.0 |
DCMD |
PTFE |
Lake water |
T
F – 60 |
J
I – 9.67 |
k
I – 2.33 μS cm−1 |
19
|
T
P – 10 |
J
F – 4.28 |
k
F – <12.7 μS cm−1 |
DCMD |
PTFE |
Qinhuai River |
T
F – 60 |
J
I – 8.10 |
k
I – 324.7 μS cm−1 |
25
|
T
P – 15 |
J
F – 4.30 |
k
F – <23.0 μS cm−1 |
DCMD |
PVDF |
Effluent water |
T
F – 60 |
J
I – 40 |
k
I – 4.5 μS cm−1 |
56
|
T
P – 20 |
J
F – 21 |
k
F – 5.8 μS cm−1 |
Permeate flux decay
Membrane biofilm development notably reduces water flux. The water flux decreases due to: (1) membrane pore-blockage, (2) vapor pressure reduction, and (3) temperature and concentration polarization effects (Fig. 4). Commonly, permeate flux decay is caused by membrane pore blockage induced by the biofouling layer.17,52 However, degree of pore blockage determines the extent at which vapour pressure decays, hence flux decline. Goh et al., (2013)23 noted a 60% decrease in water flux caused by the accumulation of microbial contaminants on the surface of the membrane. These contaminants essentially caused a 32% decrease in the vapor pressure, thus reducing the rate of water recovery. This was ascribed to an increase in heat and mass transfer resistance across the boundary layer. Resistance to heat transfer minimizes water vaporization, thus reducing process performance.6 Liu et al., (2020a)55 evaluated the occurrence of biofouling on a closed loop direct contact membrane distillation (DCMD). Reportedly, the flux decreased by 55.79% over time due to the increased deposition of foulants.
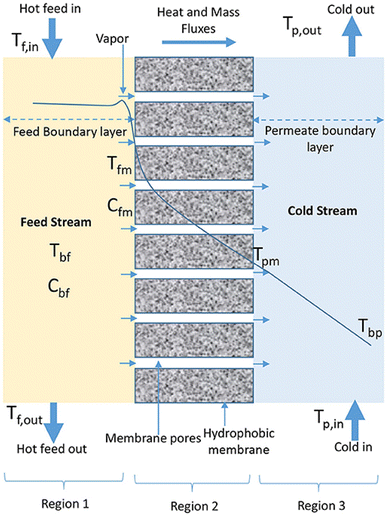 |
| Fig. 4 .Temperature polarization phenomenon in MD. Tf,in, Tfm, Tpm and Tpb represent temperatures in the bulk feed solution, near the membrane surface (feed side), near the membrane surface (permeate side) and in the bulk permeate, respectively.58 | |
Permeate flux decay of MD systems vary depending on feed temperature. This phenomenon was reported by Bogler & Bar-Zeev, (2018).22 The feed stream temperatures were controlled at 47 °C, 55 °C and 65 °C with corresponding flux declines of 30%, 78% and 32%, respectively. Flux decay was attributed to; (1) rapid growth of Anoxybacillus sp at the operating temperatures, (2), membrane pore wetting, (3) TP and CP, and (4) vapor pressure depression and hydraulic resistance. Though temperature may be increased beyond bacterial optimum growth temperature, cell adaptability at new operating conditions continue to threaten MD performance. For these reasons, the growth of biofilms increase at higher operating temperatures (Elcik et al., 2022).57
Salt rejection decline
Membrane wetting is a fundamental aspect requiring significant attention in MD systems. Wetting promotes the passage of feed in liquid form, thus reducing salt rejection.5,55,59 Deposition of hydrophilic biofilms causes membrane wetting. Bogler & Bar-Zeev, (2018)22 reported a reduced salt rejection caused by EPS-induced membrane wetting. A significant difference in salt rejection was obtained for different feed operating temperatures. 30-fold and 90-fold increase in distillate salinity was recorded at 55 °C and 65 °C respectively. 90-fold increase was attributed to the improved bacterial growth conditions causing rapid biofilm formation. Evidently, salt rejection and water flux declined upon inoculation of feed water with bacteria (Anoxybacillus sp.). However, bacterial film conditioning occurring on membrane surface may not affect process performance, especially in cases where hydrophobicity of membrane pores is not altered. This phenomenon was reported by Zodrow et al., (2014)52. Although the contact angle of the hydrophobic membrane decreased from 134 ± 4° to 32 ± 6° due to deposition of foulant layer, no membrane wetting occurred. The hydrophilic layer grew on the membrane surface without changing pore hydrophobicity.
Frequency of membrane replacement
A decrease in membrane lifespan caused by the accumulation and persistence of biofilm development leads to frequent membrane replacements. This causes significant increases in operational costs.5 The frequency of membrane replacement largely depends on the type of feed characteristics and hydrodynamic conditions.60 In desalination plants, membrane replacement occurs within 4–5 years whereas industries treating dairy products replace membranes within 3 years.60 In general, membrane replacements are not recommended due to interruptions in the process and significant amount of labour requirements.61
Mitigation of biofouling in MD
Major strategies used to mitigate biofouling in MD include pre-treatment of the feed solution, membrane modification and cleaning (Fig. 5). Detailed impact of these strategies towards water flux and salt rejection stabilities is presented in Table 3.
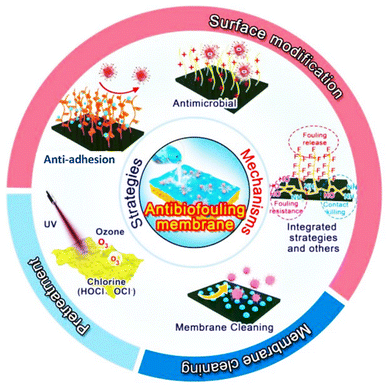 |
| Fig. 5 .Latest developments of biofouling control strategies in MD.62 | |
Table 3 Mitigation strategies of biofouling in MDa
Membrane configuration |
Mitigation strategy |
Mitigation process |
Feed solution |
Process duration |
Impact on water flux and salt rejection |
Change in flux and salt rejection |
Ref. |
J
I and JF are initial and final water flux, kI and kF are initial and final permeate conductivity, RI and RF are initial and final salt rejection respectively.
|
DCMD |
Pre-treatment |
Chlorination (addition of HCl in feed stream) |
Tap-water |
58 days |
Stable water flux and salt rejection |
J
I = 19.8 L m−2 h−1 |
26
|
J
F = 19.5 L m−2 h−1 |
k
I = 3 μS cm−1 |
k
F = 10 μS cm−1 |
DCMD |
Pre-treatment and membrane cleaning |
Magnetic coagulation and HCl cleaning |
Wastewater |
65 days |
97% flux and salt rejection were restored after cleaning (at high HCl concentration) |
J
I = 19.2 L m−2 h−1 |
32
|
J
F = 17.7 L m−2 h−1 |
R
I = 99.5% |
R
F = 98% |
DCMD |
Pre-treatment |
Microfiltration |
Estuarine water |
4 days |
50% decline in flux |
J
I = 20 L m−2 h−1 |
52
|
J
F = 11 L m−2 h−1 |
DCMD |
Membrane cleaning |
NaOH, distilled water, 70% ethanol cleaning |
Coastal seawater |
14 days |
Original flux was restored with minimal increase in distillate conductivity |
J
I = 3.9 L m−2 h−1 |
24
|
J
F = 3.8 L m−2 h−1 |
k
I = 6 μS cm−1 |
k
F = 34 μS cm−1 |
AGMD |
Backwash |
Reversion of direction of flow |
Pond water |
91 days |
Original flux was restored |
J
I = 0.8 kg m−2 h−1 |
97
|
J
F = 0.7 kg m−2 h−1 |
DCMD |
Membrane modification |
Coating membrane with hydrophilic active layer |
Effluent water |
2.5 days |
47% flux decline and slight changes in salt rejection |
J
I = 13.1 L m−2 h−1 |
56
|
J
F = 6.8 L m−2 h−1 |
R
I = 99.8% |
R
F = 97.7% |
DCMD |
Membrane modification |
Membrane modification (f-MWCNTs and AgNPs) |
Scheldt estuary water |
2 days |
20.8% flux decline with minimal salt rejection decay |
J
I = 37.1 L m−2 h−1 |
88
|
J
F = 95.4 L m−2 h−1 |
R
I = 99.99% |
R
F = 95.4% |
DCMD |
Membrane modification |
Membrane modification (f-MWCNTs and AgNPs) |
Effluent water |
2 days |
Stable flux and salt rejection |
J
I = 16.7 L m−2 h−1 |
85
|
J
F = 15.2 L m−2 h−1 |
R
I = 94.8% |
R
F = 94.6% |
Pre-treatment of the feed solution
Pre-treatment of the feed solution is a useful tool to minimize biofouling. This involves removal of potential foulants from the feed solution before MD processing. Pre-treated feed improves resistance to flux decay and reduces frequency of membrane cleaning.36,62 Pre-treatment methods are largely dependent on the composition of the feed solution.64 There are three types of pre-treatment methods widely applied in membrane-based technologies. These are physical, chemical and hybrid pre-treatment processes.
Physicochemical pre-treatment processes.
Physical and chemical pre-treatment processes include filtration (ultra/micro), sonication, coagulation, and chlorination, amongst others. Ultrafiltration (UF) and microfiltration (MF) remove foulants from the feed solution using size-exclusion mechanism. The MF and UF minimize nutrients required for microbial growth. The UF was reported to effectively remove algal cells (96%) and non-algal cells (98%) from the feed solution in a seawater reverse osmosis (SWRO) plant in Saudi Arabia.65 It is worth noting that planktonic cells passing through UF reproduce, thus enhancing biofilm development.66 For example, Zodrow et al., (2014)52 evaluated MF pre-treatment of RO and MD processes. Notably, inefficient removal of organic and biological matter from the feed stream caused blockage of membrane pores, thus a decline in water flux within 12 h of operation. Pre-treatment processes are currently evaluated for MD systems with limited literature reported.56
Alternatively, sonication (with ultrasound frequencies of ≥18 kHz) is strategically used to manage bacterial growth.67 Fouling is controlled through cavitation, where production of strong convective currents is triggered.68 However, this pre-treatment process is rarely reported. Mathieu et al. (2019)69 applied ultrasound (frequency of 46 kHz) to inhibit bacterial growth in bulk drinking water “N” spiked with 100 mg L−1 of Ca(OH)2. Evidently, a 7-fold reduction in bacterial growth was reported. Ultraviolet (UV) treatment is another alternative, where hydroxyl radicals are produced to inhibit bacterial growth.44 During UV pre-treatment, bacterial DNA is broken down while proteins are effectively denatured.44 However, UV is costly, thus limiting its application in MD water purification.70 Due to prevention of UV rays through the feed solution by contaminants, UV treatment is limited to feed turbidity. Generally, wavelength of 200–400 nm is required to initiate cell death.6 To minimize reactivation of microbial cells, UV is applied in conjunction with other mitigation strategies.
Coagulation, chlorine and ozone injection are some of the chemical processes used during pre-treatment. Coagulation is a cost-effective and most convenient process, involving stabilization of particulates through the addition of a coagulant.71 Inorganic coagulants include ferric chloride (FeCl3), aluminium chloride (AlCl3) and polymeric aluminium chloride (PAC) whereas organic coagulants include polyacrylamide (PAM), poly dimethyl diallyl ammonium chloride (PDMDAAC) and microbial flocculants.72,73 Since higher doses of inorganic flocculants are known to cause secondary pollution due to the presence of residual metal ions, a hybrid system of organic and inorganic coagulants is used to improve process performance. Zhang et al. (2022)63 used a hybrid system to evaluate the performance of microbial flocculants modified with PAC (MMF/PAC) to minimize membrane fouling. Cake layer formation on the membrane surface was effectively minimized. Management of biofilm development has been predominantly realized through chlorine dosing.6 Although chlorination of the feed stream effectively prevents biofilm development, it produces harmful mutagenic and carcinogenic by-products.74 Moreover, chlorination damages polymeric membranes due to its high oxidizing ability.63 Spore-forming microbes such as Bacillus are reported to resist chlorination, thus making pre-treatment ineffective.44 Also, ozone (O3) is used to control the growth of bacterial cells in MD systems. Owing to its high oxidizing properties, ozone inactivates viruses, bacteria and organic contaminants.44 Yong Zhang et al. (2016)75 coupled a DCMD system with ozone injection to treat organic pollutants from wastewater. 49% flux recovery was attained. Although ozonation minimizes membrane fouling, its application is limited to process costs.6 Similarly, ozone is insoluble at high temperature, thus making it unsuitable for MD applications. Other oxidative chemicals used to reduce biofouling include peracetic acid and hydrogen peroxide.
Hybrid pre-treatment processes.
The complete removal of microorganisms require hybrid rather than a standalone process. Therefore, a combination of these processes can be chosen from a wide range including ozonation, chlorination and UV irradiation. Stand-alone processes portray a certain level of limitation, hence the need for hybridization. Chen et al. (2022)76 evaluated a combination of UV and chlorine to mitigate the growth of Staphylococcus aureus. Notably, the generation of free radicals such as OH˙ and Cl˙ minimized bacterial growth and inactivated the photo-reactivation of the bacteria. In another study, O3, UV/O3 and UV-assisted peroxidation (UV/H2O2) were used to pre-treat the feed solution (wastewater) in DCMD systems (Kumar et al. 2020).77 A combination of UV/O3 and UV/H2O2 achieved a 99% and 53% bacterial removal effeciency, respectively. Improved performance of UV/H2O2 relative to stand-alone UV was attributed to the cleavage of H2O2 under UV irradiation to produce hydroxyl radicals responsible for destruction of bacterial cells. Notably, UV/H2O2 is recommended for pre-treatment of feed solutions characterized by low dissolved organic carbon (DOC).
Membrane cleaning
Membrane cleaning involves removal of microbial accumulates from membrane surfaces. The concept of cleaning was introduced to restore the original performance of the fouled membranes.6 Furthermore, membrane cleaning reduces frequency of membrane replacements.78 There are two types of cleaning methods widely applied in membrane-based technologies: (1) physical cleaning and (2) chemical cleaning.
Physical cleaning.
Physical cleaning involves the use of deionized water during MD operation to facilitate foulant detachment.15 The use of water guarantees minimal damage to the membrane structure. During cleaning, the feed solution is pumped into the module, followed by deionised water through the system at higher flowrates. These flowrates ensure increased shear forces to enable detachment of foulants from the membrane surface and spacers.27 In other instances, high pressure water is utilized.67 Lyly et al. (2021)79 used deionised water to restore the performance of PTFE membranes during treatment of synthetic seawater spiked with BSA and microalgae (Cylindrotheca fusiformis). The following steps were implemented for physical cleaning: (1) flow of pure cold-water (at 25 °C) for 10 min, followed by (2) hot water (at 60 °C) for 10 min and finally (3) cold-water for 5 min. Reportedly, 62.69% of water flux was restored, displaying promising results for future applications.
Chemical cleaning.
Chemical cleaning involves the use of chemicals to continuously remove foulants from the membrane surface. To minimize membrane damage, frequency and concentration of the cleaning agent should be optimized.64 Dominating chemical cleaning involves removal of inorganic and organic foulants using acidic and basic solutions respectively with limited information on biofouling treatment.80 Zheng et al., (2022)32 used HCl and water to remove microbial accumulates from the membrane surface. The process ensured complete removal of Euryarchaeota. However, full restoration of the flux was not achieved, largely due to Idiomarina cells' affinity for the membrane surface. Krivorot et al., (2011)24 investigated the removal of biofoulants from the membrane surface through flushing with a series of steps: (1) NaOH (pH 12 at 40 °C), (2) distilled water, and (3) 70% ethanol. Notably, the original flux of the membrane was fully restored. To minimize cleaning requirements, modification of the membranes is recommended to render them resistant to biofouling (Bogler et al., 2017).27 This approach ensures minimal waste disposal, thus ensuring environmental safety.81
Membrane modification
Modification involves the alteration of membrane properties to render them resistant to biofouling. Membranes are modified through various approaches; including incorporation of metal nanoparticles (MNPs) (Fig. 6) and alteration of membrane surface properties such as surface roughness, membrane hydrophobicity and surface charge.59,82
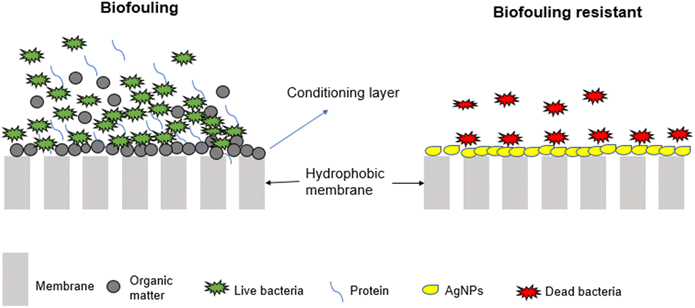 |
| Fig. 6 .Membrane modification using MNPs. | |
Incorporation of MNPs.
Attachment and proliferation of microbial cells on membrane surfaces is largely minimized through the incorporation of biocidal MNPs. Upon oxidation, ionic counterparts of biocidal MNPs are released to react with microbial cells. Biocidal MNPs capable of inactivating bacterial cells include Ag, Ti, Zn and Fe.15,83 Inactivation occurs due to the electrostatic interaction between positively charged metal ions and negatively charged thiol groups on bacterial DNA.84 Nthunya et al., (2020)85 modified polyvinylidene fluoride (PVDF) membrane using silver nanoparticles (AgNPs) and functionalized carbon nanotubes (f-MWCNTs), achieving successful biofouling, colloidal and organic fouling control in MD. Although fouling mitigation is evident, biocidal MNPs tend to leach from the membrane surface, thus reducing fouling control.27 In addition, leaching of MNPs reduces the quality of produced water. Hence, ensuring the stability of these MNPs on membrane surfaces is crucial.
Alteration of membrane surface properties.
Rendering MD membranes superhydrophobic is a useful approach to mitigate biofouling, where the surface energy of the membrane is reduced.85 Ideally, superhydrophobic membranes are characterized by contact angles ≥150°. Teoh et al. (2022)86 enhanced the surface hydrophobicity of PVDF membranes using nylon taffeta substrate for MD treatment of aquaculture wastewater. Self-cleaning microtextured membrane was characterized by water contact angle of 153° and a sliding angle of 8.9°. Notably, the water flux (30 kg m−2 h−1) remained stable. Also, hydrophilic coating of hydrophobic membranes is reported to minimize biofouling. This is achieved through fabrication of Janus membranes. During the process, membranes of asymmetric wettabilities, i.e. hydrophobic/hydrophilic combinations are produced.27 Yang et al. (2010)87 grafted zwitterionic polysulfobetaine methacrylate (polySBMA) to polypropylene (PP) membrane. Water contact angle of the active site was reduced from 145° to 15°. Reportedly, grafting density of 560 μg cm−2 provided resistance to bacterial adhesion. This was attributed to a decrease in interaction between hydrophilic bacteria (E. coli) and membrane active surface. Furthermore, hydrophobic membranes are coated with a thin layer containing hydrophilic MWCNTs and AgNPs to reduce combined fouling of organic, inorganic and biofoulants. While uncoated membranes face flux decays of approximately 90% and salt rejection decays of 6%, hydrophilic coating of membranes minimized flux and salt rejection decays to 24% and 0.75% respectively. Therefore, hydrophilic modification of membranes maintains process resistance to fouling.88
Other biofouling mitigation strategies
Other techniques used to control biofouling of MD systems include air-sparging (bubbling), quorum quenching (QQ), nitric oxide (NOx) injection and alteration of hydrodynamic conditions. The NOx enhances biofilm dispersal by decreasing 60% of membrane biofilm coverage.89 However, due to NOx instability and insolubility, their application in water treatment is minimal. The QQ involves the use of quorum quenching enzymes to deactivate signalling molecules required for biofilm development.6 The quorum sensing system regulates the communication and aggregation of bacterial species. Its suppression assists in managing biofouling in membrane systems. Gram-negative and Gram-positive bacteria use signalling molecules such as N-acyl-homoserine lactones (AHLs) and autoinducing peptides (AIPS) to promote biofilm development and successions.90 To minimise these developments, QQ enzymes such as AHLs lactases damage the lactone rings of the cells. This reduces effective communication between cells, thus slowing biofilm progression.47,91,92 Air-sparging involves the bubbling of air in the feed stream to increase flow rate, shear stress and turbulence, thus preventing membrane clogging.27 Chang et al. (2021)93 studied air bubbling to minimize biofilm development. Reportedly, membrane deposition of inorganic, organic and microalgae was significantly reduced leading to a recovery of 16.95% water flux. This was attributed to an increase in turbulence flow at the feed-membrane interface. Comparatively, shear turbulence of gas bubbling removes foulants better than shear turbulence of feed flowrates.94 However, the increase in air-bubbling beyond a certain threshold reduces the permeate flux.95 Therefore, optimization of air–water ratio is imperative.27 Lastly, hydrodynamic conditions affect MD process performance. Silva et al. (2018)96 evaluated different operating conditions such as solute concentration, flowrates, and different membrane modules on process performance. Reportedly, water flux was higher in a perpendicular flow (W-cell) of the feed to the membrane compared to a parallel flow (H-cell). This was ascribed to a decrease in temperature and concentration polarization. Remarkably, feed flowrate should be optimised to maintain high water flux while simultaneously preventing biofouling. According to Zheng et al. (2022)32 bacterial cells got thinner and longer as feed flow passed through the membrane. The inlet was less fouled by Chelativorans, Acinetobater, and Sphingobium. On one hand, temperature tolerant bacteria such as Idiomarina and Phenylobacterium were detected in abundance at the inlet where process velocity is high. Although a high feed flowrate is desired to minimize the feed boundary layer through high shear stress,96 caution must be exercised to minimize bacterial succession and abundance.
Conclusions and future outlook
MD remains a promising technology to purify high saline wastewater and seawater. However, hydrophobic membranes used in MD are susceptible to biofouling, a process involving the deposition and accumulation of microorganisms. Requiring nutrient availability to mature into a biofilm, these microorganisms largely originate from the feed solution. Several factors affecting microbial deposition include membrane properties, hydrodynamic conditions, feed solution properties and membrane module designs. Mitigation of biofouling is essential as it affects membrane lifespan, increases operational costs, and diminishes permeate water quality. Moreover, large scale application of MD has been hindered by fouling, amongst other factors. Various approaches minimizing biofouling are proposed. These include pre-treatment of the feed solution, membrane cleaning, and metal nanoparticle and surface alteration-based modification. Current reported studies largely focus on the characterization of the microbial community within the biofilm structure. Although this is essential, research work focusing on different approaches to mitigate biofouling is essential. Moreover, various module designs need to be explored. To establish the sustainable application of MD systems at pilot scale, fouling experiments should be carried over long operating periods (600 h and more). Elucidating cell-to-surface interactions using various mathematical models to simulate real operating conditions requires experimental attention. Directing efforts toward lessening attachment will ensure minimal EPS secretion and hinder biofilm progression. Optimization of MNPs concentration on membranes to determine a balance between optimum activity over long periods and the rate of leachability is required. Indeed, membrane biofouling in MD requires significant experimental work to mitigate the existing challenges.
Author contributions
Tshepiso Mpala investigated, wrote the manuscript draft, and analysed, Lebea Nthunya, Anita Etale, Heidi Richards conceptualized, validated manuscript and curated data as well as supervised the study.
Conflicts of interest
Authors declare no conflict of interest.
Acknowledgements
The authors would like to thank the University of the Witwatersrand and National Research Foundation (NRF-grant number: 132724) for funding this research work.
References
- C. He, Z. Liu, J. Wu, X. Pan, Z. Fang, J. Li and B. A. Bryan, Future global urban water scarcity and potential solutions, Nat. Commun., 2021, 12, 1–11, DOI:10.1038/s41467-021-25026-3.
- A. C. Barrios, D. Carrillo, T. R. Waag, D. Rice, Y. Bi, R. Islam and F. Perreault, Prolonging the antibacterial activity of nanosilver-coated membranes through partial sulfidation, Environ. Sci.: Nano, 2020, 7, 2607–2617, 10.1039/d0en00300j.
- J. Davis and P. Horwitz, A bridge and a voice for the water sectors, PLOS Water, 2022, 1, e0000018 CrossRef.
- A. W. Kandeal, A. Joseph, M. Elsharkawy, M. R. Elkadeem, M. A. Hamada, A. Khalil, M. Eid Moustapha and S. W. Sharshir, Research progress on recent technologies of water harvesting from atmospheric air: A detailed review, Sustain. Energy Technol. Assessments, 2022, 52, 102000, DOI:10.1016/j.seta.2022.102000.
- L. N. Nthunya, M. F. Bopape, O. T. Mahlangu, B. B. Mamba, B. Van der Bruggen, C. A. Quist-Jensen and H. Richards, Fouling, performance and cost analysis of membrane-based water desalination technologies: A critical review, J. Environ. Manage., 2022, 301, 113922, DOI:10.1016/j.jenvman.2021.113922.
- F. C. R. Costa, B. C. Ricci, B. Teodoro, K. Koch, J. E. Drewes and M. C. S. Amaral, Biofouling in membrane distillation applications - a review, Desalination, 2021, 516, 115241, DOI:10.1016/j.desal.2021.115241.
- Y. Choi, G. Naidu, S. Lee and S. Vigneswaran, Effect of inorganic and organic compounds on the performance of fractional-submerged membrane distillation-crystallizer, J. Membr. Sci., 2019, 582, 9–19, DOI:10.1016/j.memsci.2019.03.089.
- M. Khayet, Membranes and theoretical modeling of membrane distillation: A review, Adv. Colloid Interface Sci., 2011, 164, 56–88, DOI:10.1016/j.cis.2010.09.005.
- K. Nakoa, A. Date and A. Akbarzadeh, A research on water desalination using membrane distillation, Desalin. Water Treat., 2015, 56, 2618–2630, DOI:10.1080/19443994.2014.972731.
- R. Schwantes, A. Cipollina, F. Gross, J. Koschikowski, D. Pfeifle, M. Rolletschek and V. Subiela, Membrane distillation: Solar and waste heat driven demonstration plants for desalination, Desalination, 2013, 323, 93–106, DOI:10.1016/j.desal.2013.04.011.
- E. Drioli, A. Ali and F. Macedonio, Membrane distillation: Recent developments and perspectives, Desalination, 2015, 356, 56–84, DOI:10.1016/j.desal.2014.10.028.
- K. C. Chong and S. O. Lai, Recent progress in membrane distillation, Jurnal Teknologi, 2014, 70, 97–103, DOI:10.11113/jt.v70.3445.
- P. Pal and A. K. Manna, Removal of arsenic from contaminated groundwater by solar-driven membrane distillation using three different commercial membranes, Water Res., 2010, 44, 5750–5760, DOI:10.1016/j.watres.2010.05.031.
- M. Gryta, The Application of Submerged Modules for Membrane Distillation, Membranes, 2020, 10(25), 1–22, DOI:10.3390/membranes10020025.
- Y. Guo, C. Liu, H. Liu, J. Zhang, H. Li and C. Zhang, Contemporary antibiofouling modifications of reverse osmosis membranes: State-of-the-art insights on mechanisms and strategies, Chem. Eng. J., 2022, 429, 132400, DOI:10.1016/j.cej.2021.132400.
- M. El Batouti, N. F. Alharby and M. M. Elewa, Review of new approaches for fouling mitigation in membrane separation processes in water treatment applications, Separations, 2022, 9(1), 1–44, DOI:10.3390/separations9010001.
- J. W. Chew, W. B. Krantz and A. G. Fane, Effect of a macromolecular- or bio-fouling layer on membrane distillation, J. Membr. Sci., 2014, 456, 66–76, DOI:10.1016/j.memsci.2014.01.025.
- S. Shirazi, C. J. Lin and D. Chen, Inorganic fouling of pressure-driven membrane processes - A critical review, Desalination, 2010, 250, 236–248, DOI:10.1016/j.desal.2009.02.056.
- C. Liu, L. Zhu and L. Chen, Biofouling phenomenon of direct contact membrane distillation (DCMD) under two typical operating modes: Open-loop mode and closed-loop mode, J. Membr. Sci., 2010, 601, 11792, DOI:10.1016/j.memsci.2020.117952.
- M. Zahid, A. Rashid, S. Akram, H. M. Fayzan Shakir, Z. A. Rehan, T. Javed, R. Shabbir and M. M. Hessien, Fabrication and characterization of sulfonated graphene oxide-doped polymeric membranes with improved anti-biofouling behavior, Membranes, 2021, 11, 11080563, DOI:10.3390/membranes11080563.
- J. Phattaranawik, A. G. Fane, A. C. S. Pasquier, W. Bing and F. S. Wong, Experimental study and design of a submerged membrane distillation bioreactor, Chem. Eng. Technol., 2009, 32, 38–44, DOI:10.1002/ceat.200800498.
- A. Bogler and E. Bar-Zeev, Membrane Distillation Biofouling: Impact of Feedwater Temperature on Biofilm Characteristics and Membrane Performance, Environ. Sci. Technol., 2018, 52, 10019–10029, DOI:10.1021/acs.est.8b02744.
- S. Goh, Q. Zhang, J. Zhang, D. McDougald, W. B. Krantz, Y. Liu and A. G. Fane, Impact of a biofouling layer on the vapor pressure driving force and performance of a membrane distillation process, J. Membr. Sci., 2013, 438, 140–152, DOI:10.1016/j.memsci.2013.03.023.
- M. Krivorot, A. Kushmaro, Y. Oren and J. Gilron, Factors affecting biofilm formation and biofouling in membrane distillation of seawater, J. Membr. Sci., 2011, 376, 15–24, DOI:10.1016/j.memsci.2011.01.061.
- L. Chen, Y. Wang, Z. Chen and Z. Cai, The fouling layer development on MD membrane for water treatments: An especial focus on the biofouling progress, Chemosphere, 2021, 264, 128458, DOI:10.1016/j.chemosphere.2020.128458.
- M. Gryta, The assessment of microorganism growth in the membrane distillation system, Desalination, 2002, 142, 79–88, DOI:10.1016/S0011-9164(01)00427-1.
- A. Bogler, S. Lin and E. Bar-zeev, Biofouling of membrane distillation , forward osmosis and pressure retarded osmosis : Principles , impacts and future directions, J. Membr. Sci., 2017, 542, 378–398, DOI:10.1016/j.memsci.2017.08.001.
- A. Anvari, A. Azimi Yancheshme, K. M. Kekre and A. Ronen, State-of-the-art methods for overcoming temperature polarization in membrane distillation process: A review, J. Membr. Sci., 2020, 616, 118413, DOI:10.1016/j.memsci.2020.118413.
- M. Gryta, Concentration of NaCl solution by membrane distillation integrated with crystallization, Sep. Sci. Technol., 2002, 37, 3535–3558, DOI:10.1081/SS-120014442.
- M. Aslam, R. Ahmad and J. Kim, Recent developments in biofouling control in membrane bioreactors for domestic wastewater treatment, Sep. Purif. Technol., 2018, 206, 97–315, DOI:10.1016/j.seppur.2018.06.004.
- A. Uneputty, A. Dávila-Lezama, D. Garibo, A. Oknianska, N. Bogdanchikova, J. F. Hernández-Sánchez and A. Susarrey-Arce, Strategies applied to modify structured and smooth surfaces: A step closer to reduce bacterial adhesion and biofilm formation, Colloids and Interface Science Communications, 2012, 46, 100560, DOI:10.1016/j.colcom.2021.100560.
- L. Zheng, C. Zhang, S. Kang, C. Li, D. Hou, S. Wu, J. Wang and Y. Wei, Insight into the microbial distribution and succession and biofouling mechanism in membrane distillation for desulfurization wastewater treatment, Chem. Eng. J., 2022, 428, 131097, DOI:10.1016/j.cej.2021.131097.
- S. Meng, R. Wang, K. Zhang, X. Meng, W. Xue, H. Liu, D. Liang, Q. Zhao and Y. Liu, Transparent exopolymer particles (TEPs)-associated protobiofilm: A neglected contributor to biofouling during membrane filtration, Front. Environ. Sci. Eng., 2021, 15, 1–10, DOI:10.1007/s11783-020-1361-7.
- Y. Zhang, Y. Wan, G. Pan, H. Yan, X. Yao, H. Shi, Y. Tang, X. Wei and Y. Liu, Surface modification of polyamide reverse osmosis membrane with organic-inorganic hybrid material for antifouling, Appl. Surf. Sci., 2018, 433, 139–148, DOI:10.1016/j.apsusc.2017.10.043.
- K. Hori and S. Matsumoto, Bacterial adhesion: From mechanism to control, Biochem. Eng. J., 2010, 48, 424–434, DOI:10.1016/j.bej.2009.11.014.
- N. I. Abu-Lail and T. A. Camesano, Role of ionic strength on the relationship of biopolymer conformation, DLVO contributions, and steric interactions to bioadhesion of pseudomonas putida KT2442, Biomacromolecules, 2003, 4, 1000–1012, DOI:10.1021/bm034055f.
- J. H. Adair, E. Suvaci and J. Sindel, Derjaguin, Landau, Verwey and Overbeek (DLVO) theory, Surf. Colloid Chem. - from, Encyclopedia of Materials: Science and Technology, 2001, 1–10 Search PubMed.
- H. Fabre, D. Mercier, A. Galtayries, D. Portet, N. Delorme and J. F. Bardeau, Impact of hydrophilic and hydrophobic functionalization of flat TiO 2/Ti surfaces on proteins adsorption, Appl. Surf. Sci., 2018, 432, 15–21, DOI:10.1016/j.apsusc.2017.08.138.
- M. A. Daeschel and J. McGuire, Interrelationships between Protein Surface Adsorption and Bacterial Adhesion, Biotechnol. Genet. Eng. Rev., 1998, 15, 413–438, DOI:10.1080/02648725.1998.10647964.
- Y. Luan, S. Liu, M. Pihl, H. C. van der Mei, J. Liu, F. Hizal, C. H. Choi, H. Chen, Y. Ren and H. J. Busscher, Bacterial interactions with nanostructured surfaces, Curr. Opin. Colloid Interface Sci., 2018, 38, 170–189, DOI:10.1016/j.cocis.2018.10.007.
- O. Habimana, A. J. C. Semião and E. Casey, The role of cell-surface interactions in bacterial initial adhesion and consequent biofilm formation on nanofiltration/reverse osmosis membranes, J. Membr. Sci., 2014, 454, 82–96, DOI:10.1016/j.memsci.2013.11.043.
- R. Bernstein, S. Belfer and V. Freger, Bacterial attachment to RO membranes surface-modified by concentration-polarization-enhanced graft polymerization, Environ. Sci. Technol., 2011, 45, 5973–5980, DOI:10.1021/es1043694.
- Q. She, R. Wang, A. G. Fane and C. Y. Tang, Membrane fouling in osmotically driven membrane processes: A review, J. Membr. Sci., 2016, 499, 201–233, DOI:10.1016/j.memsci.2015.10.040.
- M. Al-Abri, B. Al-Ghafri, T. Bora, S. Dobretsov, J. Dutta, S. Castelletto, L. Rosa and A. Boretti, Chlorination disadvantages and alternative routes for biofouling control in reverse osmosis desalination, npj Clean Water, 2019, 2, 1–15, DOI:10.1038/s41545-018-0024-8.
- A. Matin, Z. Khan, S. M. J. Zaidi and M. C. Boyce, Biofouling in reverse osmosis membranes for seawater desalination : Phenomena and prevention, Desalination, 2011, 281, 1–16, DOI:10.1016/j.desal.2011.06.063.
- K. Manzoor, S. J. Khan, M. Yasmeen, Y. Jamal and M. Arshad, Assessment of anaerobic membrane distillation bioreactor hybrid system at mesophilic and thermophilic temperatures treating textile wastewater, J. Water Proc. Eng., 2022, 46, 102603, DOI:10.1016/j.jwpe.2022.102603.
- S. Tabraiz, B. Shamurad, E. Petropoulos, M. Quintela-Baluja, A. Charlton, J. Dolfing and P. J. Sallis, Mitigation of membrane biofouling in membrane bioreactor treating sewage by novel quorum quenching strain of Acinetobacter originating from a full-scale membrane bioreactor, Bioresour. Technol., 2021, 334, 125242, DOI:10.1016/j.biortech.2021.125242.
- M. Pagliero, A. Comite, O. Soda and C. Costa, Effect of support on PVDF membranes for distillation process, J. Membr. Sci., 2021, 635, 119528, DOI:10.1016/j.memsci.2021.119528.
- R. Ruhal and R. Kataria, Biofilm patterns in gram-positive
and gram-negative bacteria, Microbiol. Res., 2021, 251, 126829, DOI:10.1016/j.micres.2021.126829.
- H. Yoon, Y. Baek, J. Yu and J. Yoon, Biofouling occurrence process and its control in the forward osmosis, Desalination, 2013, 325, 30–36, DOI:10.1016/j.desal.2013.06.018.
- R. Valladares Linares, S. S. Bucs, Z. Li, M. AbuGhdeeb, G. Amy and J. S. Vrouwenvelder, Impact of spacer thickness on biofouling in forward osmosis, Water Res., 2014, 57, 223–233, DOI:10.1016/j.watres.2014.03.046.
- K. R. Zodrow, E. Bar-Zeev, M. J. Giannetto and M. Elimelech, Biofouling and microbial communities in Membrane Distillation and Reverse Osmosis, Environ. Sci. Technol., 2014, 48, 13155–13164, DOI:10.1021/es503051t.
- A. Belila, J. El-Chakhtoura, N. Otaibi, G. Muyzer, G. Gonzalez-Gil, P. E. Saikaly, M. C. M. van Loosdrecht and J. S. Vrouwenvelder, Bacterial community structure and variation in a full-scale seawater desalination plant for drinking water production, Water Res., 2016, 94, 62–72, DOI:10.1016/j.watres.2016.02.039.
- I. Vaz-Moreira, O. C. Nunes and C. M. Manaia, Bacterial diversity and antibiotic resistance in water habitats: Searching the links with the human microbiome, FEMS Microbiol. Rev., 2014, 38, 761–778, DOI:10.1111/1574-6976.12062.
- C. Liu, L. Zhu and L. Chen, Effect of salt and metal accumulation on performance of membrane distillation system and microbial community succession in membrane biofilms, Water Res., 2020, 177, 115805, DOI:10.1016/j.watres.2020.115805.
- L. N. Nthunya, L. Gutierrez, N. Khumalo, S. Derese, B. B. Mamba, A. R. Verliefde and S. D. Mhlanga, Superhydrophobic PVDF nanofibre membranes coated with an organic fouling resistant hydrophilic active layer for direct-contact membrane distillation, Colloids Surf., A, 2019, 575, 363–372, DOI:10.1016/j.colsurfa.2019.05.031.
- H. Elcik, A. Alpatova, G. Gonzalez-Gil, B. Blankert, N. Farhat, N. A. Amin, J. S. Vrouwenvelder and N. Ghaffour, Elucidating biofouling over thermal and spatial gradients in seawater membrane distillation in hot climatic conditions, Water Res., 2022, 223, 118983, DOI:10.1016/j.watres.2022.118983.
- S. O. Olatunji and L. M. Camacho, Heat and mass transport in modeling membrane distillation configurations: A review, Front. Energy Res., 2018, 6, 1–18, DOI:10.3389/fenrg.2018.00130.
- L. Tjale, H. Richards, O. Mahlangu and L. N. Nthunya, Silica nanoparticle modified polysulfone/polypropylene membrane for separation of oil-water emulsions, Results Eng., 2022, 16, 100623, DOI:10.1016/j.rineng.2022.100623.
- S. A. Avlonitis, K. Kouroumbas and N. Vlachakis, Energy consumption and membrane replacement cost for seawater RO desalination plants, Desalination, 2003, 157, 151–158, DOI:10.1016/S0011-9164(03)00395-3.
- A. Agrawal, A. Sharma, K. K. Awasthi and A. Awasthi, Metal oxides nanocomposite membrane for biofouling mitigation in wastewater treatment, Mater. Today Chem., 2021, 21, 100532, DOI:10.1016/j.mtchem.2021.100532.
- H. Zhang, S. Zhu, J. Yang and A. Ma, Advancing Strategies of Biofouling Control in Water-Treated Polymeric Membranes, Polymers, 2022, 14, 1–23, DOI:10.3390/polym14061167.
- B. Zhang, X. Mao, X. Tang, H. Tang, Y. Shen and W. Shi, Pre-coagulation for membrane fouling mitigation in an aerobic granular sludge membrane bioreactor: A comparative study of modified microbial and organic flocculants, J. Membr. Sci., 2022, 644, 120129, DOI:10.1016/j.memsci.2021.120129.
- M. R. Choudhury, N. Anwar, D. Jassby and M. S. Rahaman, Fouling and wetting in the membrane distillation driven wastewater reclamation process – A review, Adv. Colloid Interface Sci., 2019, 269, 370–399, DOI:10.1016/j.cis.2019.04.008.
- A. R. Guastalli, F. X. Simon, Y. Penru, A. de Kerchove, J. Llorens and S. Baig, Comparison of DMF and UF pre-treatments for particulate material and dissolved organic matter removal in SWRO desalination, Desalination, 2013, 322, 144–150, DOI:10.1016/j.desal.2013.05.005.
- J. Mansouri, S. Harrisson and V. Chen, Strategies for controlling biofouling in membrane filtration systems: Challenges and opportunities, J. Mater. Chem., 2010, 20, 4567–4586, 10.1039/b926440j.
- G. Gizer, U. Önal, M. Ram and N. Sahiner, Biofouling and Mitigation Methods: A Review, Biointerface Res. Appl. Chem., 2023, 13, 1–25, DOI:10.33263/BRIAC132.185.
- S. Aghapour Aktij, A. Taghipour, A. Rahimpour, A. Mollahosseini and A. Tiraferri, A critical review on ultrasonic-assisted fouling control and cleaning of fouled membranes, Ultrasonics, 2020, 108, 106228, DOI:10.1016/j.ultras.2020.106228.
- L. Mathieu, A. Keraval, N. F. Declercq and J. C. Block, Assessment of a low-frequency ultrasound device on prevention of biofilm formation and carbonate deposition in drinking water systems, Ultrason. Sonochem., 2019, 52, 41–49, DOI:10.1016/j.ultsonch.2018.10.029.
- H. Zhou and D. W. Smith, Advanced technologies in water and wastewater treatment, Can. J. Civ. Eng., 2001, 28, 49–66, DOI:10.1139/cjce-28-s1-49.
- A. Alkhatib, M. A. Ayari and A. H. Hawari, Fouling mitigation strategies for different foulants in membrane distillation, Chem. Eng. Process., 2021, 167, 108517, DOI:10.1016/j.cep.2021.108517.
- B. Ren, C. Li, X. Zhang and Z. Zhang, Fe(II)-dosed ceramic membrane bioreactor for wastewater treatment: Nutrient removal, microbial community and membrane fouling analysis, Sci. Total Environ., 2019, 664, 116–126, DOI:10.1016/j.scitotenv.2019.02.019.
- C. Zhao, J. Zhou, Y. Yan, L. Yang, G. Xing, H. Li, P. Wu, M. Wang and H. Zheng, Application of coagulation/flocculation in oily wastewater treatment: A review, Sci. Total Environ., 2021, 765, 142795, DOI:10.1016/j.scitotenv.2020.142795.
- M. Zemite, L. Mezule, K. Gruskevica, K. Kokina, J. Rubulis, T. Juhna, N. Gottschalk, F. Dömer, R. Jagau, K. Röwe, W. Augustin, S. Scholl, A. Pereira, A. C. Barros, I. Machado and L. F. Melo, Affordable Pretreatment Strategy for Mitigation of Biofouling in Drinking-Water Systems, J. Environ. Eng., 2022, 148(2) DOI:10.1061/(asce)ee.1943-7870.0001968.
- Y. Zhang, P. Zhao, J. Li, D. Hou, J. Wang and H. Liu, A hybrid process combining homogeneous catalytic ozonation and membrane distillation for wastewater treatment, Chemosphere, 2016, 160, 134–140, DOI:10.1016/j.chemosphere.2016.06.070.
- X. Chen, Z. Chen, H. Liu, N. Huang, Y. Mao, K. Cao, Q. Shi, Y. Lu and H. Y. Hu, Synergistic effects of UV and chlorine in bacterial inactivation for sustainable water reclamation and reuse, Sci. Total Environ., 2022, 845, 157320, DOI:10.1016/j.scitotenv.2022.157320.
- R. Vinoth Kumar, M. O. Barbosa, A. R. Ribeiro, S. Morales-Torres, M. F. R. Pereira and A. M. T. Silva, Advanced oxidation technologies combined with direct contact membrane distillation for treatment of secondary municipal wastewater, Process Saf. Environ. Prot., 2020, 140, 111–123, DOI:10.1016/j.psep.2020.03.008.
- S. Alzahrani, A. W. Mohammad, N. Hilal, P. Abdullah and O. Jaafar, Identification of foulants, fouling mechanisms and cleaning efficiency for NF and RO treatment of produced water, Sep. Purif. Technol., 2013, 118, 324–341, DOI:10.1016/j.seppur.2013.07.016.
- L. H. T. Lyly, B. S. Ooi, W. J. Lim, Y. S. Chang, C. J. C. Derek and J. K. Lim, Desalinating microalgal-rich water via thermoresponsive membrane distillation, J. Environ. Chem. Eng., 2021, 9, 105897, DOI:10.1016/j.jece.2021.105897.
- E. Guillen-Burrieza, A. Ruiz-Aguirre, G. Zaragoza and H. A. Arafat, Membrane fouling and cleaning in long term plant-scale membrane distillation operations, J. Membr. Sci., 2014, 468, 360–372, DOI:10.1016/j.memsci.2014.05.064.
- M. Ben-Sasson, X. Lu, E. Bar-Zeev, K. R. Zodrow, S. Nejati, G. Qi, E. P. Giannelis and M. Elimelech, In situ formation of silver nanoparticles on thin-film composite reverse osmosis membranes for biofouling mitigation, Water Res., 2014, 62, 260–270, DOI:10.1016/j.watres.2014.05.049.
- R. R. Choudhury, J. M. Gohil, S. Mohanty and S. K. Nayak, Antifouling, fouling release and antimicrobial materials for surface modification of reverse osmosis and nanofiltration membranes, J. Mater. Chem. A, 2018, 6, 313–333, 10.1039/c7ta08627j.
- Y. N. Slavin, J. Asnis, U. O. Häfeli and H. Bach, Metal nanoparticles: Understanding the mechanisms behind antibacterial activity, J. Nanobiotechnol., 2017, 15, 1–20, DOI:10.1186/s12951-017-0308-z.
- A. Politano, P. Argurio, G. Di Profio, V. Sanna, A. Cupolillo, S. Chakraborty, H. A. Arafat and E. Curcio, Photothermal Membrane Distillation for Seawater Desalination, Adv. Mater., 2017, 29, 1–6, DOI:10.1002/adma.201603504.
- L. N. Nthunya, L. Gutierrez, E. N. Nxumalo, A. R. Verliefde, S. D. Mhlanga and M. S. Onyango, f-MWCNTs/AgNPs-coated superhydrophobic PVDF nanofibre membrane for organic, colloidal, and biofouling mitigation in direct contact membrane distillation, J. Environ. Chem. Eng., 2020, 8, 103654, DOI:10.1016/j.jece.2020.103654.
- G. H. Teoh, Z. A. Jawad, B. S. Ooi, Y. S. Chang and S. C. Low, Surface-templating of rough interface to efficiently recover aquaculture wastewater using membrane distillation, Desalination, 2022, 522, 115419, DOI:10.1016/j.desal.2021.115419.
- Y. F. Yang, Y. Li, Q. L. Li, L. S. Wan and Z. K. Xu, Surface hydrophilization of microporous polypropylene membrane by grafting zwitterionic polymer for anti-biofouling, J. Membr. Sci., 2010, 362, 255–264, DOI:10.1016/j.memsci.2010.06.048.
- L. N. Nthunya, L. Gutierrez, L. Lapeire, K. Verbeken, N. Zaouri, E. N. Nxumalo, B. B. Mamba, A. R. Verliefde and S. D. Mhlanga, Fouling resistant PVDF nanofibre membranes for the desalination of brackish water in membrane distillation, Sep. Purif. Technol., 2019, 228, 115793, DOI:10.1016/j.seppur.2019.115793.
- N. Barraud, M. V. Storey, Z. P. Moore, J. S. Webb, S. A. Rice and S. Kjelleberg, Nitric oxide-mediated dispersal in single- and multi-species biofilms of clinically and industrially relevant microorganisms, Microb. Biotechnol., 2009, 2, 370–378, DOI:10.1111/j.1751-7915.2009.00098.x.
- S. Ge, Y. Zhao, D. Liu, X. Dong, Y. Zhang, H. Yang and Y. Li, Characterization of a N-acylhomoserine lactonase from Serratia sp. and its biofouling mitigation in a membrane bioreactor, Microbiol. Res., 2022, 264, 127175, DOI:10.1016/j.micres.2022.127175.
- Y. Gu, J. Huang, G. Zeng, Y. Shi, Y. Hu, B. Tang, J. Zhou, W. Xu and L. Shi, Quorum quenching activity of indigenous quorum quenching bacteria and its potential application in mitigation of membrane biofouling, J. Chem. Technol. Biotechnol., 2018, 93, 1394–1400, DOI:10.1002/jctb.5507.
- N. N. Shu, H. Park, S. S. A. Shah, N. Mameda, H. J. Yoo, J. Min, I. Angelidaki and K. H. Choo, Probiotic strategy for biofouling control through direct injection of quorum-quenching bacteria into membrane bioreactors, Chem. Eng. J., 2022, 438, 135572, DOI:10.1016/j.cej.2022.135572.
- Y. S. Chang, Y. T. Cheah, L. H. T. Lyly, N. I. I. Ishak, Y. S. Ng, A. L. Ahmad, C. P. Leo, C. J. C. Derek and B. S. Ooi, Microalgal exopolymeric substances fouling in submerged vacuum membrane distillation and its mitigation via enhanced air bubbling, Desalination, 2021, 508, 115047, DOI:10.1016/j.desal.2021.115047.
- S. J. Judd, P. Le-Clech and B. Jefferson, A comparison of submerged and sidestream tubular membrane bioreactor configurations, Desalination, 2005, 173, 113–122, DOI:10.1016/j.desal.2004.08.029.
- Z. Ding, L. Liu, Z. Liu and R. Ma, The use of intermittent gas bubbling to control membrane fouling in concentrating TCM extract by membrane distillation, J. Membr. Sci., 2011, 372, 172–181, DOI:10.1016/j.memsci.2011.01.063.
- T. L. S. Silva, S. Morales-Torres, C. M. P. Esteves, A. R. Ribeiro, O. C. Nunes, J. L. Figueiredo and A. M. T. Silva, Desalination and removal of organic micropollutants and microorganisms by membrane distillation, Desalination, 2018, 437, 121–132, DOI:10.1016/j.desal.2018.02.027.
- G. W. Meindersma, C. M. Guijt and A. B. de Haan, Desalination and water recycling by air gap membrane distillation, Desalination, 2006, 187, 291–301, DOI:10.1016/j.desal.2005.04.088.
|
This journal is © The Royal Society of Chemistry 2023 |
Click here to see how this site uses Cookies. View our privacy policy here.